- 1Department of Forestry and Wildlife Ecology, University of Wisconsin—Madison, Madison, WI, United States
- 2Hitchcock Center for Chemical-Ecology, Department of Chemistry, University of Nevada—Reno, Reno, NV, United States
- 3Truckee Meadows Community College, Reno, NV, United States
A novel meroterpenoid cabagranin D was isolated with related neolignans cabagranins A–C from the leaves of Piper cabagranum (Costa Rica). Cabagranins A–C represent the first examples of 3,3′-neolignans isolated from the plant genus Piper, and the meroterpenoid cabagranin D displays an unprecedented Diels–Alder conjugate of an unsubstituted phenylpropenone and α-phellandrene. Details of the full structural elucidation of these compounds and a discussion of their potential biosynthetic relationships are presented.
1 Introduction
The Piper genus of plants (Piperaceae) is the source of a diversity of compounds isolated from over 2,600 accepted species distributed across the tropics (Parmar et al., 1997; Gutierrez et al., 2013; Mgbeahuruike et al., 2017; Gomez-Calvario and Rios, 2019; Salehi et al., 2019; Fan et al., 2023). Numerous studies have characterized the role of these compounds in various ecological interactions and uncovered novel compounds with a wide diversity of biological activities, including antimicrobial and anti-herbivore activities (Xu and Li, 2011).
In a phytochemical survey of Piper species within the Radula clade, we identified Piper cabagranum as having a unique chemistry based on GC-MS and 1H NMR analysis of crude extracts (Uckele et al., 2021). We observed that general categories of natural products like lignans, sesquiterpenes, and flavonoids were shared among closely related species; however, 1H NMR analysis of crude leaf extracts revealed that specific structural motifs varied widely (Richards et al., 2018; Uckele et al., 2021). This divergence in functional motifs likely stems from the distinct evolutionary paths of these plant species, creating fertile ground for the discovery of new natural products. The unique spectral features encountered in the crude methanolic extract of P. cabagranum (Costa Rica) distinguished it from the other 70 species in our study and motivated the phytochemical characterization of this species, with the goal of understanding the role of specialized metabolites in mediating ecological interactions. Our work led to the discovery of an unprecedented meroterpene Diels–Alder conjugate cabagranin D, 5 (Figure 1). Furthermore, this work identified a new series of dehydrodieugenol-derived 3,3′-neolignans (cabagranins A–C; 1–3) which involve novel biosynthetic connections between cabagranin D (5) and the co-isolated neolignans (Figure 1). We here report the isolation, structural, and stereochemical characterization of new meroterpenoid 5 and neolignans 1–3—natural products from P. cabagranum (Costa Rica).
2 Materials and methods
2.1 Plant material
Leaf samples of P. cabagranum were collected from La Selva Biological Station and the Tirimbina Biological Reserve and verified (voucher # EJT3531) in March 2012. The leaves were oven-dried (35°C–40°C) and ground to a fine powder.
2.2 Extraction and isolation
The ground leaf material (1 g) was twice extracted with 400 mL of HPLC-grade hexanes for 2 h under mechanical agitation. The supernatants were pooled and evaporated under reduced pressure. The spent plant material was then twice extracted with HPLC-grade (Fisher Scientific, Hampton, NH) acetone under the same conditions, and the supernatants were combined and evaporated under reduced pressure, resulting in 200 mg of crude acetone extract and 100 mg of hexane extract. The crude acetone extract (180 mg) was dissolved in methanol (3 mL) and then purified via RP-HPLC (Poroshell C18, 21.2 mm × 150 mm, Agilent, Santa Clara, California, United States) using a 20 min gradient of 30%–100% acetonitrile:water (Optima grade: Fisher Scientific, Hampton, NH) and held for 7 min at 100% acetonitrile using an Agilent 1260/1290 Infinity II equipped with an Agilent 6140 Quadrupole LC/MS (Santa Clara, California, United States). This separation yielded compounds 1 (63 mg, elution time = 8.1 min), 2 (3 mg, elution time = 9.4 min), 3 (4 mg, elution time = 10.4 min), 4 (2 mg, elution time = 13.6 min), and 5 (2 mg, elution time = 18.9 min). The hexane extract (100 mg) was further purified through solid phase extraction (C-18 Sep-Pak) using a 10% step gradient of acetone:water from 50% to 100% acetone, yielding a 70% acetone:water fraction that was enriched in compound 5. Further purification using the preparatory HPLC methods described above yielded an additional 3 mg of compound 5.
2.3 Spectroscopic acquisition methods
High-resolution mass spectrometry data were collected using an Agilent TOF LC/MS (model G6230B, Santa Clara, California, United States). NMR spectra were gathered using a two-channel 400 MHz Varian VNMRS spectrometer (399.78 MHz 1H and 100.53 MHz 13C) equipped with an ATB automation probe (400 ATB PFG) (Agilent, Santa Clara, California, United States). Circular dichroism experiments were performed on a Jasco J-1500 CD-spectrometer (model J-1500-150, Jasco Corporation, Tokyo, Japan). Polarimetry experiments were conducted on a Jasco P-2000 polarimeter (Jasco Corporation, Tokyo, Japan).
2.3.1 High-resolution mass spectrometry measurements
High-resolution mass spectrometry (HRMS) analysis was performed using an Agilent TOF LC/MS (Santa Clara, California, United States) fitted with an electrospray ionization source (ESI). The isolated compound was taken up into methanol (1 μg/mL) and injected directly into the ionization source. Instrument parameters were: gas temperature, 325°C; gas flow, 5 L/min; nebulizer, 20 psig; and ion polarity, positive.
2.3.2 Nuclear magnetic resonance measurements
Reported chemical shifts were recorded in parts per million (δ) using CD3OD as a standard for 1H and 13C (δH 3.31; δC 49.0). Coupling constants (J) are reported in Hz. Nuclear magnetic resonance (NMR) assignments were made based on 1H and 13C spectra, as well as various 2D experimental spectra (COSY, HMBC, HSQC, and NOESY). For individual compounds, 1H spectra were acquired using the parameters set automatically by the instrument with the number of transients (nt = 128), 13C spectra with the number of transients (nt = 15,000), 1H–1H gCOSY (nt = 4 × 128), 1H–13C gHMBCAD (nt = 8 × 512), 1H–13C gHSQCAD (nt = 4 × 256), and 1H–1H NOESY (nt = 32 × 256).
2.3.3 Polarimetry measurements
Polarimetry measurements were taken on a Jasco P-2000 polarimeter. Each compound was dissolved in 10 mL of dichloromethane and placed into a 10-cm polarimeter cell along with a dichloromethane blank. The samples were placed in the polarimeter to obtain the optical rotation in degrees.
2.3.4 Electronic circular dichroism measurements
Electronic circular dichroism (ECD) measurements were obtained on a Jasco J-1500 CD spectrometer. The isolated compound was dissolved in methanol (0.5 mM) and placed into the CD spectrometer along with a methanol blank. The acquisition parameters were as follows: photometric mode, CD, HT; measure range, 400–200 nm; data pitch, 0.5 nm; CD scale, 200 mdeg/0.1 dOD; FL scale, 200 mdeg/0.1 dOD; D.I.T., 1 s; bandwidth, 1.00 nm; accumulations, 1; and scanning speed, 10 nm/min.
3 Results and discussion
Cabagranin A (1) was purified from the 50% acetone:water eluent as a colorless oil, which was found to have the formula C21H24O5 from HRESIMS m/z = 379.1551 [M + Na]+, corresponding to an oxygenated dehydrodieugenol derivative. 1H NMR analysis revealed the clear presence of a bis-phenylpropanoid with differing propenyl units (Table 1). One of these units was hydroxylated at C-7, indicated by the resonance δH 5.07 (d, J = 5.6 Hz)/δC 76.0, which was coupled to the C-8 vinylic methine δH 6.05 (ddd, J = 17.1, 10.3, 5.9 Hz)/δC 142.3 based on COSY and HMBC analyses. HMBC correlations to quaternary oxygenated aromatic carbons led to the assignment of the three different methoxy singlets as aryl methyl ethers (Figure 2).
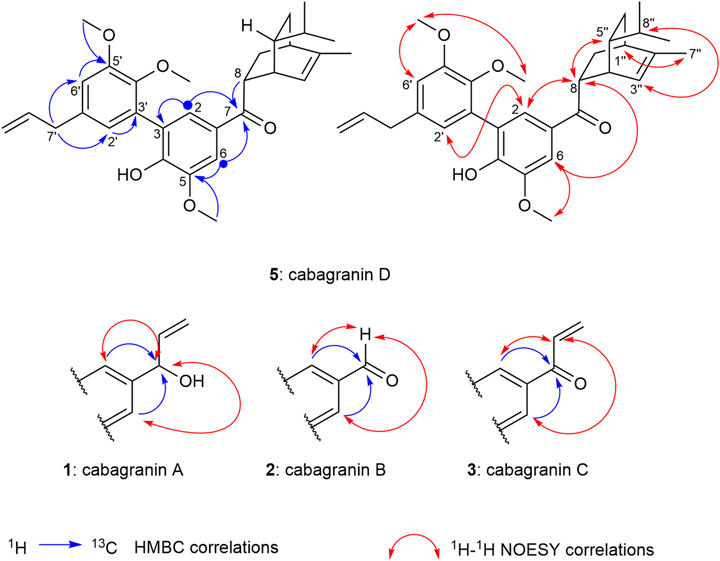
FIGURE 2. 2D NMR correlations establishing the proposed structures and relative configurations of cabagranins A–D.
Proton resonances in the aromatic region indicate the presence of two pairs of meta-coupled protons (δH 6.96/6.74 and δH 6.83/6.65, J ∼ 2 Hz), each pair displaying HMBC correlations with each set of the two aromatic O-substituted carbons (δC 144–154) and one of the benzylic carbons (δC 76.0 and 40.8, respectively). NOESY correlations between the most shielded protons in each ring supported the proximity of the two rings through direct linkage. Lastly, NOESY correlations were used to assign the location of three methoxy groups across the aromatic rings, which supported the lone phenol being para to the modified propenyl moiety.
Attempts to evaluate the enantiopurity of cabagranin A (1) and assign the absolute configuration of the alcohol were unsuccessful due to decomposition of the material under a variety of derivatization conditions. ECD analysis demonstrated no Cotton effects and a low optical rotation value {
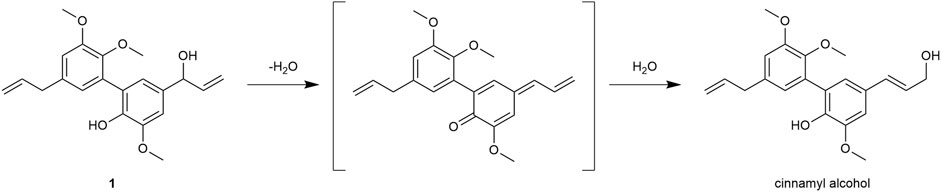
FIGURE 3. Proposed rearrangement of alcohol 1 to the cinnamyl alcohol derivative through a p-quinone methide intermediate.
Further purification of the 50% acetone:water fractions resulted in four minor components that retained most of the structural features in 1, including 4, which is presumed to be the biosynthetic precursor to 1, and a coumarin (Scheme 1, see SI). Two new compounds were isolated from this fraction which bore the identical bis-aryl phenol moiety of 1 but differed in their modified propenyl moieties. These compounds were assigned as cabagranin B (2), which contains an aldehyde substituent, and cabagranin C (3), which contains a 1-propenone substituent (Figure 1). It is important to note that neolignans containing the vinyl ketone substituent of 3 have only been isolated in a few cases and that most reports suggest that this product is the result of lignin pyrolysis.
Cabagranin D (5) was isolated as the predominant component of the 70% acetone:water fractions and found to have the formula C31H38O5 from HRESIMS m/z = 513.2653 [M + Na]+. NMR spectral analysis indicated the presence of the 3,3′-biaryl structure analogous to 1–4 in addition to an iso-propyl group (δH 0.85), an allylic methyl (δH 1.76 and δC 20.0), and a vinylic proton [δH 5.49 (dt, J = 6.5 and 2.0 Hz), δC 121.9] (Table 2). 1H–1H COSY correlations were consistent with a [2.2.2] bicyclic structure, which was supported by key HMBC correlations between H-2 and H-6 aryl methines and the H-8 methine with the carbonyl carbon at δC 202. Relative configuration of C-8 and C-5″ were assigned from NOESY correlations between H-8 to H-5″ and H-8″ to H-3''. Further 2-D NMR correlations were consistent with the structural assignment of 5, which is postulated to be the endo product of a Diels–Alder cycloaddition between the enone of 3 and the monoterpene α-phellandrene (6, Figure 1). This new molecule seems to represent a novel late-stage merger between a terpene and a neolignan, presumably through a Diels–Alder reaction.
Compound 5 was found to be optically active and have an optical rotation of
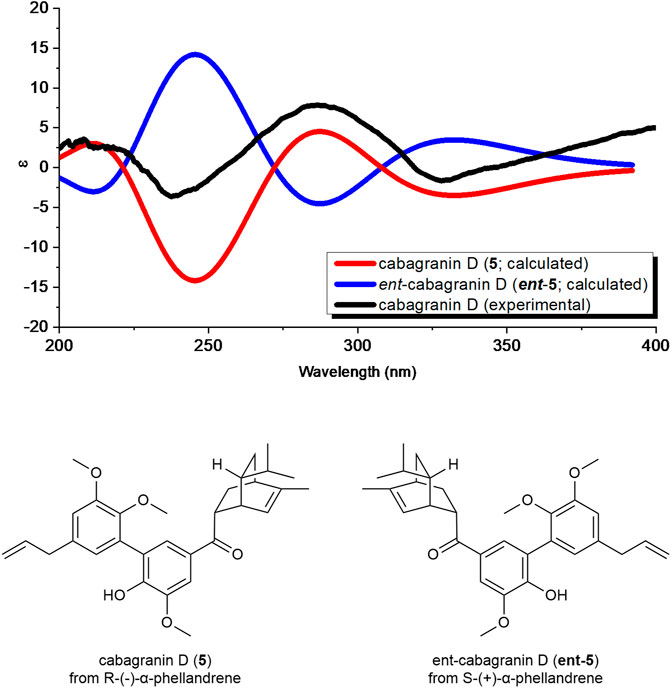
FIGURE 4. Comparison of the calculated ECD spectra of both enantiomers of cabagranin D using time-dependent density functional theory (TDDFT) calculations and experimental ECD spectra.
4 Conclusion
The co-isolation of the series of neolignans 1–4 supports the proposed biosynthetic pathway shown in Scheme 1. This hypothesis suggests that eugenol undergoes oxidative dimerization followed by monomethylation to yield compound 4. The major constituent of the crude extract is formed through the selective oxidation of the allyl group of the phenolic ring. A variety of neolignans have been isolated from other Piper species, but this represents the first example that contains a hydroxylated propenyl side chain (Macedo et al., 2017). While the conversion of the alcohol to ketone 3 is anticipated to be facile, compound 3 was not always present in detectable concentrations in the crude extracts of the leaves. The high electrophilic reactivity of 3, its rare occurrence (Chen et al., 2012; de Sousa et al., 2017; de Sousa et al., 2020), and the presumed toxicity of the vinyl ketone suggest that this compound could be an artifact of isolation and is not present in high concentrations in vivo (Chen et al., 2012; de Sousa et al., 2017; de Sousa et al., 2020).
The discovery of meroterpenoid 5 effectively represents a Diels–Alder cycloaddition reaction between ketone 3 and α-phellandrene (6). Although some similar examples exist, the isolation of 5 provides the first example of a Diels–Alder product between an unsubstituted phenylpropenone and a terpene (Pasfield et al., 2013; Alves et al., 2017; Qiu et al., 2018; Tortora et al., 2022; Zhou et al., 2023). Given the instability of 3, we hypothesize that the ketone precursor could be formed in situ and simultaneously trapped by α-phellandrene in a single enzymatic step. In this scenario, the Diels–Alder product could emerge from the activity of an oxidase enzyme acting on the hydroxyl group of compound 1. This oxidation of 1 would lead to the formation of a vinyl p-quinone methide intermediate, representing the protonated enone, which would produce 5 (Scheme 1) from the reaction with α-phellandrene. Recent research highlights the role of redox-active enzymes that have likely diverged from their ancestral functions to act as Diels–Alderases in the biosynthesis of prenylated phenol and alkaloid natural products (Oikawa and Tokiwano, 2004; Gao et al., 2020; Gao et al., 2022; Liu et al., 2023). Other investigations have shown that phenols and their ethers can act as redox tags in electrocatalytic Diels–Alder reactions and that silver nanoparticles can catalyze related Diels–Alder reactions involving phenolic chalcones and terpenoid dienes (Cong et al., 2008; Cong et al., 2010). When comparing biomimetic Diels–Alder reactions involving a chalcone and a 2,4-disubstituted diene, it was found that the desired reactions with moderate yield require high pressures or temperatures, or strong Lewis acids (ONeill et al., 2006; Tee et al., 2016; Chai et al., 2020; Tangdenpaisal et al., 2022). However, when using enzymatic (Gao et al., 2020) or redox-active catalysts (Cong et al., 2010; Ohmura et al., 2023), nearly identical reactants can undergo the Diels–Alder reaction at room temperature or even below, demonstrating a more efficient and milder process. While these reports support our hypothesis, we cannot distinguish the role of Lewis-acid or single-electron processes in catalyzing the proposed Diels–Alder reaction. Ongoing experimental and computational investigations are evaluating our biosynthetic hypothesis surrounding the formation of 5.
The compounds isolated in this study establish P. cabagranum as a chemically distinct species within its genus, primarily due to the presence of oxidized 3,3′-neolignans and a distinctive neolignan meroterpenoid, cabagranin D, marking the first occurrence of a Diels–Alder between a vinyl ketone dienophile and a terpene diene. It inspires future studies on the biosynthetic origins of this unique compound.
Data availability statement
The original contributions presented in the study are included in the article/Supplementary Material; further inquiries can be directed to the corresponding author.
Author contributions
CO: conceptualization, investigation, methodology, writing–original draft, writing–review and editing, data curation, formal analysis, and validation. ZL: data curation, formal analysis, investigation, validation, and writing–review and editing. ML: data curation, investigation, writing–review and editing, and methodology. SO: formal analysis, investigation, writing–review and editing, and methodology. CD: formal analysis, investigation, writing–review and editing, conceptualization, funding acquisition, and validation. CJ: investigation, methodology, writing–review and editing, conceptualization, funding acquisition, project administration, resources, supervision, and writing–original draft.
Funding
The author(s) declare that financial support was received for the research, authorship, and/or publication of this article. This work was supported by grants from the NSF (EF-2133818) and by the Hitchcock Center for Chemical-Ecology fund. CO was supported by a Hitchcock Center for Chemical-Ecology graduate fellowship. Collection and export permits were granted by Sistema Nacional De Areas De Conservación (SINAC), Costa Rica (113-DGVS-2016).
Acknowledgments
The authors thank Christopher Harris for assistance with collecting the ECD spectra and Eric J. Tepe and Humberto Garcia for their help in identification and collection of the plant tissue.
Conflict of interest
The authors declare that the research was conducted in the absence of any commercial or financial relationships that could be construed as a potential conflict of interest.
The author(s) declared that they were an editorial board member of Frontiers, at the time of submission. This had no impact on the peer review process and the final decision.
Publisher’s note
All claims expressed in this article are solely those of the authors and do not necessarily represent those of their affiliated organizations, or those of the publisher, the editors, and the reviewers. Any product that may be evaluated in this article, or claim that may be made by its manufacturer, is not guaranteed or endorsed by the publisher.
Supplementary material
The Supplementary Material for this article can be found online at: https://www.frontiersin.org/articles/10.3389/fntpr.2023.1332436/full#supplementary-material
References
Alves, H. D. S., Rocha, W., Braz-Filho, R., and Chaves, M. C. O. (2017). Isolation of monoterpene dihydrochalcones from piper montealegreanum yuncker (piperaceae). Molecules 22 (6), 874. doi:10.3390/molecules22060874
Chai, G. L., Qiao, Y., Zhang, P., Guo, R., Wang, J., and Chang, J. (2020). Chiral hydroxytetraphenylene-boron complex catalyzed asymmetric diels-alder cycloaddition of 2’-hydroxychalcones. Org. Lett. 22, 8023–8027. doi:10.1021/acs.orglett.0c02978
Chen, Q. F., Chen, X. Z., Li, G. Y., Wang, C., and Zhang, G. L. (2012). Two new 2-phenylbenzofurans from the bark of Styrax perkinsiae. Chin. J. Nat. Med. 10 (2), 92–97. doi:10.3724/sp.j.1009.2012.00092
Cong, H., Becker, C. F., Elliott, S. J., Grinstaff, M. W., and Porco, J. A. (2010). Silver nanoparticle-catalyzed Diels− alder cycloadditions of 2′-hydroxychalcones. J. Am. Chem. Soc. 132 (21), 7514–7518. doi:10.1021/ja102482b
Cong, H., Ledbetter, D., Rowe, G. T., Caradonna, J. P., and Porco, J. A. (2008). Electron transfer-initiated diels− alder cycloadditions of 2′-hydroxychalcones. J. Am. Chem. Soc. 130 (29), 9214–9215. doi:10.1021/ja803094u
de Sousa, F. S., Baldim, J. L., Azevedo, R. A., Figueiredo, C. R., Pieper, P., Sear, C. E., et al. (2020). Structure-activity relationship study of cytotoxic neolignan derivatives using multivariate analysis and computation-aided drug design. Bioorg Med. Chem. Lett. 30 (16), 127349. doi:10.1016/j.bmcl.2020.127349
de Sousa, F. S., Grecco, S. S., Girola, N., Azevedo, R. A., Figueiredo, C. R., and Lago, J. H. G. (2017). Neolignans isolated from Nectandra leucantha induce apoptosis in melanoma cells by disturbance in mitochondrial integrity and redox homeostasis. Phytochemistry 140, 108–117. doi:10.1016/j.phytochem.2017.04.024
Fan, D., Zhou, C., Chen, C., Li, X., Ma, J., Hu, Y., et al. (2023). Lignans from the genus Piper L. and their pharmacological activities: an updated review. Fitoterapia 165, 105403. doi:10.1016/j.fitote.2022.105403
Gao, L., Su, C., Du, X., Wang, R., Chen, S., Zhou, Y., et al. (2020). FAD-dependent enzyme-catalysed intermolecular [4+2] cycloaddition in natural product biosynthesis. Nat. Chem. 12 (7), 620–628. doi:10.1038/s41557-020-0467-7
Gao, L., Yang, J., and Lei, X. (2022). Enzymatic intermolecular Diels-Alder reactions in synthesis: from nature to design. Tetrahedron Chem. 2, 100013. doi:10.1016/j.tchem.2022.100013
Gomez-Calvario, V., and Rios, M. Y. (2019). 1 H and 13 C NMR data, occurrence, biosynthesis, and biological activity of Piper amides. Magn. Reson Chem. 57 (12), 993–1070. doi:10.1002/mrc.4941
Gutierrez, R. M., Gonzalez, A. M., and Hoyo-Vadillo, C. (2013). Alkaloids from piper: a review of its phytochemistry and pharmacology. Mini Rev. Med. Chem. 13 (2), 163–193. doi:10.2174/138955713804805148
Liu, Z., Rivera, S., Newmister, S. A., Sanders, J. N., Nie, Q., Liu, S., et al. (2023). An NmrA-like enzyme-catalysed redox-mediated Diels-Alder cycloaddition with anti-selectivity. Nat. Chem. 15 (4), 526–534. doi:10.1038/s41557-022-01117-6
Macedo, A. L., Dos Santos, T. C. C., Valverde, A. L., Moreira, D. L., and Vasconcelos, T. R. A. (2017). An overview of neolignans of the genus piper L.: isolation methods and biological activities. Mini Rev. Med. Chem. 17 (8), 693–720. doi:10.2174/1389557516666161130094826
Mgbeahuruike, E. E., Yrjönen, T., Vuorela, H., and Holm, Y. (2017). Bioactive compounds from medicinal plants: focus on Piper species. South Afr. J. Bot. 112, 54–69. doi:10.1016/j.sajb.2017.05.007
Ohmura, S., Katagiri, K., Kato, H., Horibe, T., Miyakawa, S., Hasegawa, J. Y., et al. (2023). Highly enantioselective radical cation [2 + 2] and [4 + 2] cycloadditions by chiral iron(III) photoredox catalysis. J. Am. Chem. Soc. 145, 15054–15060. doi:10.1021/jacs.3c04010
Oikawa, H., and Tokiwano, T. (2004). Enzymatic catalysis of the Diels-Alder reaction in the biosynthesis of natural products. Nat. Prod. Rep. 21 (3), 321–352. doi:10.1039/b305068h
O’Neill, P. M., Verissimo, E., Ward, S. A., Davies, J., Korshin, E. E., Araujo, N., et al. (2006). Diels–Alder/thiol–olefin co-oxygenation approach to antimalarials incorporating the 2,3-dioxabicyclo[3.3.1]nonane pharmacophore. Bioorg. Med. Chem. Lett. 16 (11), 2991–2995. doi:10.1016/j.bmcl.2006.02.059
Parmar, V. S., Jain, S. C., Bisht, K. S., Jain, R., Taneja, P., Jha, A., et al. (1997). Phytochemistry of the genus piper. Phytochemistry 46 (4), 597–673. doi:10.1016/s0031-9422(97)00328-2
Pasfield, L. A., de la Cruz, L., Ho, J., Coote, M. L., Otting, G., and McLeod, M. D. (2013). Synthesis of (±)-Panduratin A and related natural products using the high pressure diels–alder reaction. Asian J. Org. Chem. 2 (1), 60–63. doi:10.1002/ajoc.201200171
Qiu, Y. W., Hai, P., Xiao, H., Gao, Y., Tao, Y. H., Miao, D. R., et al. (2018). Glabralides A-C, three novel meroterpenoids from Sarcandra glabra. Tetrahedron 74 (2), 341–347. doi:10.1016/j.tet.2017.12.001
Richards, L. A., Oliveira, C., Dyer, L. A., Rumbaugh, A., Urbano-Muñoz, F., Wallace, I. S., et al. (2018). Shedding light on chemically mediated tri-trophic interactions: a 1H-NMR network approach to identify compound structural features and associated biological activity. Front. Plant Sci. 9, 1155. doi:10.3389/fpls.2018.01155
Salehi, B., Zakaria, Z. A., Gyawali, R., Ibrahim, S. A., Rajkovic, J., Shinwari, Z. K., et al. (2019). Piper species: a comprehensive review on their phytochemistry, biological activities and applications. Molecules 24 (7), 1364. doi:10.3390/molecules24071364
Tangdenpaisal, K., Songthammawat, P., Ruchirawat, S., and Ploypradith, P. (2022). Total synthesis of palodesangrens A and C. J. Org. Chem. 87, 386–398. doi:10.1021/acs.joc.1c02417
Tee, J. T., Keane, T., Meijer, AJHM, Khaledi, H., Abd Rahman, N., and Chee, C. F. (2016). A strategy toward the biomimetic synthesis of (±)-Morusalbanol A pentamethyl ether. Synthesis 48, 2263–2270. doi:10.1055/s-0035-1560434
Tortora, C., Pisano, L., Vergine, V., Ghirga, F., Iazzetti, A., Calcaterra, A., et al. (2022). Synthesis, biosynthesis, and biological activity of diels-alder adducts from morus genus: an update. Molecules 27 (21), 7580. doi:10.3390/molecules27217580
Uckele, K. A., Jahner, J. P., Tepe, E. J., Richards, L. A., Dyer, L. A., Ochsenrider, K. M., et al. (2021). Phytochemistry reflects different evolutionary history in traditional classes versus specialized structural motifs. Sci. Rep. 11 (1), 17247. doi:10.1038/s41598-021-96431-3
Xu, W. H., and Li, X. C. (2011). Antifungal compounds from piper species. Curr. Bioact. Compd. 7 (4), 262–267. doi:10.2174/157340711798375822
Keywords: meroterpene, Diels–Alder, neolignan, Piper cabagranum, Piperaceae
Citation: de Oliveira CR Jr, Ledvina ZD, Leonard MD, Odoh SO, Dodson CD and Jeffrey CS (2024) Isolation of new neolignans and an unusual meroterpenoid from Piper cabagranum. Front. Nat. Produc. 2:1332436. doi: 10.3389/fntpr.2023.1332436
Received: 02 November 2023; Accepted: 11 December 2023;
Published: 04 January 2024.
Edited by:
Grzegorz Wegrzyn, University of Gdansk, PolandReviewed by:
Vaderament-A. Nchiozem-Ngnitedem, University of Potsdam, GermanyRachid Chahboun, University of Granada, Spain
Copyright © 2024 de Oliveira, Ledvina, Leonard, Odoh, Dodson and Jeffrey. This is an open-access article distributed under the terms of the Creative Commons Attribution License (CC BY). The use, distribution or reproduction in other forums is permitted, provided the original author(s) and the copyright owner(s) are credited and that the original publication in this journal is cited, in accordance with accepted academic practice. No use, distribution or reproduction is permitted which does not comply with these terms.
*Correspondence: Christopher S. Jeffrey, Y2plZmZyZXlAdW5yLmVkdQ==