- Univ. Lille, Centre National de la Recherche Scientifique (CNRS), Institut National de la Santé et de la Recherche Médicale (Inserm), Centre Hospitalier Universitaire de Lille (CHU Lille), Institut Pasteur de Lille, U1019 - UMR 9017 - Center for Infection and Immunity of Lille (CIIL), Lille, France
Although the adipose tissue (AT) is a central metabolic organ in the regulation of whole-body energy homeostasis, it is also an important endocrine and immunological organ. As an endocrine organ, AT secretes a variety of bioactive peptides known as adipokines – some of which have inflammatory and immunoregulatory properties. As an immunological organ, AT contains a broad spectrum of innate and adaptive immune cells that have mostly been studied in the context of obesity. However, overwhelming evidence supports the notion that AT is a genuine immunological effector site, which contains all cell subsets required to induce and generate specific and effective immune responses against pathogens. Indeed, AT was reported to be an immune reservoir in the host’s response to infection, and a site of parasitic, bacterial and viral infections. In addition, besides AT’s immune cells, preadipocytes and adipocytes were shown to express innate immune receptors, and adipocytes were reported as antigen-presenting cells to regulate T-cell-mediated adaptive immunity. Here we review the current knowledge on the role of AT and AT’s immune system in host defense against pathogens. First, we will summarize the main characteristics of AT: type, distribution, function, and extraordinary plasticity. Second, we will describe the intimate contact AT has with lymph nodes and vessels, and AT immune cell composition. Finally, we will present a comprehensive and up-to-date overview of the current research on the contribution of AT to host defense against pathogens, including the respiratory viruses influenza and SARS-CoV-2.
Introduction
Adipose tissues (ATs) are organized to form one of the largest organ in the body that contributes to several essential functions of our organism including e.g., mechanical support, thermoregulation, energy storage and release, regulation of appetite, and modulation of immunity (1–4). There are three major types of adipose tissues – the white adipose tissue (WAT), the brown adipose tissue (BAT), and the beige adipose tissue. WAT is the most prevalent of the tissue types and its primary role is to control the storage and release of energy to supply the bioenergetic needs to other organs. BAT is the major site of sympathetically activated nonshivering thermogenesis during cold exposure and after spontaneous hyperphagia, thereby controlling whole-body energy expenditure and body fat (5). Remarkably, WAT has the ability to reversibly acquire thermogenic characteristics in response to certain stimuli in a process called “browning” or “beiging”, corresponding to the beige (or brite) adipose tissue (6).
Since 20 years, it has been shown that WAT contains nearly every innate and adaptive immune cell types (7–10). Thus, WAT can now be considered as a true immunological organ, with important roles in anti-microbial defense, wound healing, and inflammation (11). Akin to WAT, immune cells have been recently reported to also infiltrate and reside within thermogenic ATs (i.e., BAT and beige adipose tissue) where they contribute to tissue’s homeostasis and activation (12–14). Besides, not only do ATs contain immune cells, but even adipocytes (ATs’ main constituent cells) can produce factors with immunoregulatory and antimicrobial activities (15–18), and can act as antigen-presenting cells to initiate adaptive immune responses (19).
Here, after a description of the key features of ATs: their type, distribution, function and remarkable flexibility, we review the key literature illustrating the immune properties of ATs and describe the important, yet underestimated, role ATs may play in the host defense against pathogens, notably in influenza and SARS-CoV-2 respiratory infections.
Key features of adipose tissues: Type, distribution, function and plasticity
ATs are specialized connective tissues that are organized to form a large organ with discrete anatomy, specific vascular and nerve supplies, complex cytology, and high physiological plasticity (1, 20, 21). ATs contribute to many of an organism’s basic needs, including energy metabolism, thermogenesis, and protection against pathogens (1, 2, 4, 22).
Below, we will evoke the main characteristics of ATs: their type, location, function and exceptional capacity to adapt to various nutritional, hormonal and environmental changes; these have been extensively reviewed elsewhere (21, 23–28).
The different types, locations, and functions of adipose tissues
In mammals, two broad categories of ATs i.e., WAT and BAT, function antagonistically to control systemic energy homeostasis (29, 30). The anabolic WAT collects, stores, and releases energy in the form of lipids (31), whereas the catabolic BAT oxidizes lipids to produce heat – a process known as adaptive thermogenesis (32–34). Functionally distinct from WAT and BAT, the bone marrow adipose tissue [also referred to as “yellow adipose tissue” or “marrow adipose tissue” (MAT)] is a key regulator of bone metabolism (35, 36). Although less well-documented than WAT and BAT, MAT has also been acknowledged as contributing to systemic energy metabolism regulation (37–40).
WAT is the most abundant form of ATs. It can be subdivided into subcutaneous (SCAT) and visceral (VAT) adipose tissue (4, 41, 42), although smaller depots are scattered throughout the body, surrounding organs and lymph nodes (Figure 1A). SCAT and VAT differ significantly with regard to their cellular, molecular, and physiological characteristics, and oppositely contribute to the metabolic syndrome (2, 47, 48). Indeed, while increased amount of SCAT is associated with improved insulin sensitivity (49), VAT accumulation is commonly associated with increased insulin resistance, high-risk of type 2 diabetes, and high mortality (50, 51). SCAT is located beneath the skin, and should be distinguished from the less-characterized dermal WAT (DAT), which resides directly below the reticular dermis (i.e., above the SCAT), and is involved in thermal insulation, hair regeneration, wound healing and protection against skin infections (17, 18, 52–55). VAT includes intrathoracic fat depots (surrounding the heart: epicardial and pericardial fat, situated within the mediastinum: mediastinal fat), and intraabdominal fat depots (mesenteric, omental, perigonadal, perirenal and retroperitoneal fat). In addition, under certain physiological (e.g., aging) or pathological (e.g., metabolic syndrome) conditions, fat depots can also develop around or within skeletal muscles (56) and in liver (57, 58).
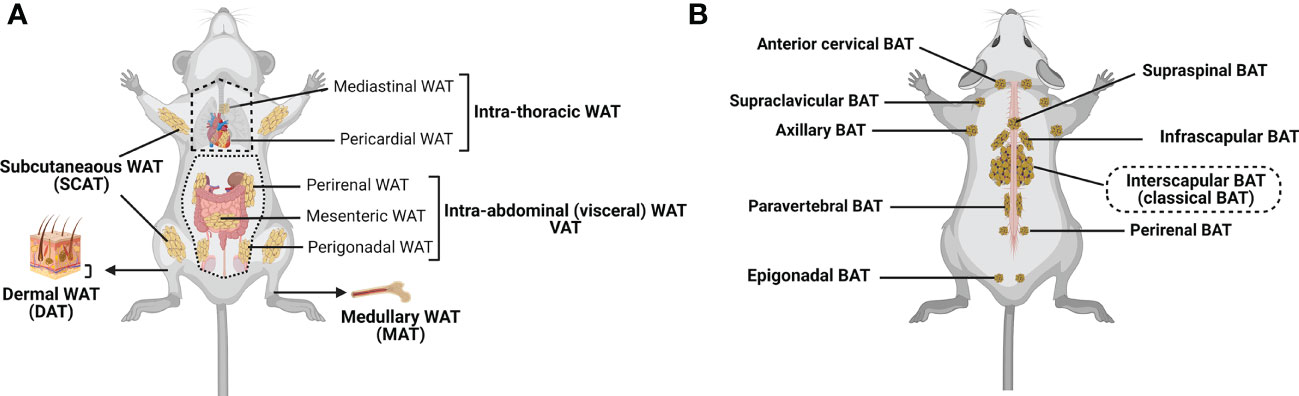
Figure 1 Major adipose tissue depots and anatomical locations in the mouse. There are two major types of adipose tissue: lipid-rich white adipose tissue (WAT; involved in energy storing) and mitochondria-rich brown adipose tissue (BAT; involved in energy burning) (24, 29, 30). Besides, WAT can convert to metabolically active fat through the process of browning (Beige/brite adipose tissue that is considered as the third adipose tissue type; inducible, energy expending) (6, 43). (A) WAT is found in many anatomical locations. The largest WAT depots are subcutaneous (SCAT; for example, inguinal, gluteal and femoral) and visceral (VAT; within the abdominal cavity, between the organs; for example, perirenal, mesenteric and perigonadal VAT). Intrathoracic adipose tissue is an extra-abdominal WAT depot located in the thoracic cavity (for example, pericardial and mediastinal WAT). Smaller WAT depots are also found around blood vessels (not shown), within the bone marrow (medullary WAT: MAT), in the dermis (dermal WAT: DAT), or as ectopic depots within specific organs (pancreas, skeletal muscle, liver (not shown)). (B) Classical brown adipocytes are contained in the interscapular BAT depot. Clusters of brown adipocytes are also found in other locations, including infrascapular, cervical, supraclavicular, axillary, paravertebral, epigonadal, perirenal and supraspinal depots (24, 41, 44–46). Created with BioRender.com.
Compared to WAT, BAT is located in more specific areas (41, 44, 45). In rodents, the largest and most investigated BAT depot is the subcutaneous interscapular BAT, but BAT depots can also be found in the supraclavicular region of the neck (46), and in perirenal region (45) (Figure 1B). In humans, the supraclavicular region contains the highest proportion of total body BAT volume, followed by the mediastinal, thoracic paravertebral, perinephric, and adrenal loci (59). While previously believed to be nonexistent or nonfunctional in adult humans, several reports provide unequivocal evidence of the presence and activity of BAT in this population (60, 61).
In stark contrast to WAT and BAT, MAT has a specific location in the bone marrow of certain long bones and vertebrae, where it resides side-by-side with hematopoietic and bone cells (36, 40, 62, 63). MAT includes two distinct subtypes that have different locations in bones – the “constitutive MAT”, concentrated in the distal skeletal bones, and the “regulated MAT” that is diffusely distributed in the spine and proximal limb bones, and is regulated by several environmental factors (40, 64).
The remarkable plasticity and remodeling capacity of adipose tissues: Introducing the beige adipose tissue
A momentous property of ATs is their high degree of plasticity. To meet the organism’s needs, ATs’ size, metabolism, structure, and phenotype can change rapidly (25–27, 65).
The potential for ATs to grow and regress in size is substantial. WAT can quickly expand its volume within days after initiation of an obesogenic diet through an increase in the size (hypertrophy, due to enhanced lipid storage capacity) and/or the number (hyperplasia) of adipocytes, whereas it rapidly shrinks by lipolysis upon fasting or cold exposure (66–69). This illustrates (i) the metabolic plasticity of WAT i.e., the ability to switch between two opposing metabolic programs: nutrient storage (lipogenesis) vs. nutrient release (lipolysis), and (ii) the cellular plasticity of WAT since newly generated adipocytes can be recruited during obesity development and relapse, as well as during cold exposure (65, 70–73).
As observed in obesity, aging is also associated with expanded WAT mass (resulting from decreased SCAT and DAT mass, and marked increased VAT mass), and reduced BAT mass and activity (74–77). At the molecular level, transcription control through forkhead box protein A3 (FOXA3) has been proposed as a potential regulatory factor for WAT accretion and BAT decline during aging and obesity (78). In addition, it has been reported that aging and obesity are also associated with increased MAT mass (79, 80). Most importantly, the age- and obesity-related changes in ATs’ mass and location are associated with progressive dysfunction of these tissues, ultimately leading to systemic inflammation and occurrence of metabolic disorders; this defines the concept of “adipaging” (77, 81, 82).
Another striking example of ATs’ remarkable metabolic plasticity is that, under various physiological, pathological and pharmacological conditions, WAT can acquire oxidative BAT-like features and, conversely, that BAT can convert into WAT-like tissue, in processes respectively termed “WAT browning” (or “beiging”) (6, 43) and “BAT whitening” (83). Thermogenic adaptation of ATs has been initially described during environmental cold exposure: BAT mass increases together with elevated expression of thermogenic genes (84). In WAT, especially in rodents, cold exposure induces the development of thermogenic beige adipocytes within the tissue (84, 85). Results from several studies further support that “inducible” brown adipocytes (aka “beige” or “brite”) can emerge within classical WAT depots in response to cold acclimatation and other stimuli such as β 3-adrenergic stimulation (28, 86). The exact mechanism is currently unclear (41, 87). Some have proposed that the browning/beiging of WAT is a result of de novo production of beige adipocytes (88), while other studies support that beige adipocytes derive from preexisting adipocytes (89). It is noteworthy that both aging and obesity are associated with impaired WAT browning (75, 90), and enhanced BAT whitening (91). Very few studies have focused on mechanisms involved in BAT whitening. For optimal thermogenic activity, BAT requires an extensive vascularization to ensure efficient supplies of oxygen needed to support the high energy consumption (92). It has been proposed that BAT whitening may partly rely on decreased vascularity of the tissue, leading to functional hypoxia and decreased thermogenic activity (93). Moreover, beige adipocytes can transform into energy-storing white adipocytes within days after external stimuli are withdrawn (94, 95).
The biomedical interest in beige AT – now viewed as the third type of ATs, is currently centered on the capacity of this oxidative tissue to counteract obesity via induction of energy expenditure, and to mitigate the vast array of obesity-associated diseases, including type 2 diabetes, heart disease, insulin resistance, hyperglycemia, dyslipidemia, hypertension, and many types of cancer (96, 97).
Adipose tissues: A proper and legitimate component of the immune system
Immune organs represent sites of exclusive immunological function, however, the definition of an immune tissue has been extended to organs such as the liver (98), uterus (99), skeletal muscle (100), hypothalamus (101), and small intestine (102). Similarly, ATs (mostly described for WAT) are now defined as proper immune organs (3, 11, 103, 104).
The adipose-lymphatic crosstalk
The lymphatic system is distributed throughout the body and consists of lymphoid nodes and organs, and lymphatic vessels (105, 106). The lymphatic system forms a one-direction transit pathway from the extracellular space toward the venous circulation to maintain fluid homeostasis by removing the protein-rich lymph from the extracellular space among tissues and returning it to the bloodstream (107). In addition to its fluid homeostasis function, the lymphatic system is also important for transport of pathogens [bacteria, viruses, prions (108)], antigens, exosomes and immune cells (including antigen-presenting cells) to regional lymph nodes and lymphoid structures, and management of immune cell trafficking and inflammation (107, 109–114). Besides, it has been reported that lymphatic endothelial cells regulate immune responses more directly by controlling entry of immune cells into lymphatic capillaries, presenting antigens on major histocompatibility complex proteins, and modulating antigen presenting cells (115).
In humans, lymphatic density varies greatly from AT depot to depot, but local lymphatic vessel function may still impact local adipose health (116, 117). In mice, lymphatics are absent in BAT and present in WAT (rare in gonadal VAT, sparse in SCAT). For instance, SCAT lies in proximity to the dermal lymphatic vasculature, and VAT surrounds the collecting lymphatic vessels of the mesentery, cisterna chyli and thoracic duct, as well as the efferent and afferent lymphatic vessels of intra-abdominal lymph nodes (118).
In addition, a close relationship exists between ATs and lymph nodes – the organizing centers of immune surveillance and response. Indeed, even in the leanest animals, lymph nodes are always found surrounded by WAT (119). Extensive work by Pond and colleagues further revealed the bidirectional functional partnership connecting WAT and lymph nodes: through increasing its rate of lipolysis, perinodal WAT serves as a reservoir of energy that is deployed to power local immune responses while, vice-versa, chronic lymph node activation results in increased WAT mass (119–124).
All immune-cell-types are present in adipose tissues and functionally active
Despite a rather simple histological appearance, ATs’ cellular composition is complex (125). Although contributing to more than 90% of ATs’ volume, mature, lipid-filled adipocytes represent less than 50% of adipose cells (126). Other cell types in ATs (collectively referred to as stromal-vascular cells) include e.g., multipotent mesenchymal progenitor cells, preadipocytes, and a broad spectrum of innate and adaptive immune cells – which all spatially and functionally interact. Interest in adipose immune cells was significantly accelerated by the discovery that ATs’ immune cell composition is highly sensitive to metabolic and nutritional states (7, 8); this exemplifies another aspect of ATs’ cellular adaptability. Although mainly described for WAT, in which they contribute to the regulation of systemic metabolism by modulating the inflammatory tone of the tissue, immune cells are also present in thermogenic ATs (i.e., BAT and beige adipose tissue) where they have been proposed to support and regulate tissue remodeling and thermogenic function (12–14, 127, 128).
AT’s innate and adaptive immune cell types and functions have been extensively documented, mainly for WAT and predominantly in the context of obesity (7–10, 129). In the lean state, WAT contains anti-inflammatory M2-like macrophages, regulatory T cells (Treg cells), type 2 innate lymphoid cells (ILC2s), invariant natural killer cells (iNKT cells), natural killer cells (NK cells), eosinophils, dendritic cells (DCs), and γδ T cells, which all cooperate to prevent inflammation and coordinate metabolic responses. In the obese state, the profile of adipose immune cells shifts towards a proinflammatory-type of cells: the number of neutrophils, inflammatory M1 macrophages, mast cells, B cells, DCs, CD8+ T cells, T helper (Th) 1 cells and Th17 cells increases, while the number of eosinophils, iNKT cells, ILC2s and Treg cells decreases (9, 129). Interestingly, in the context of mouse and human obesity it has been reported that immune cell composition differ between SCAT and VAT (130–132).
A summary of immune cells identified in ATs is presented in Table 1.
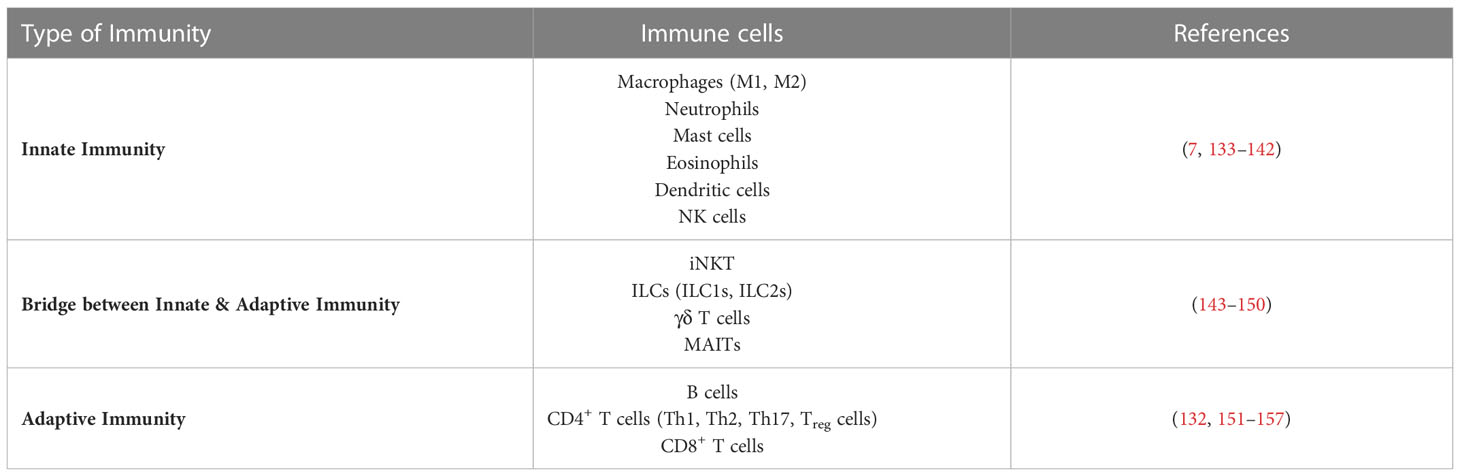
Table 1 Immune cells (innate, adaptive and bridging innate & adaptive immunity) found in adipose tissues.
In addition, it has to be mentioned that some intraabdominal and intrathoracic VAT depots are rich in immune cell clusters called fat-associated lymphoid clusters (FALCs). FALCs correspond to inducible, atypical lymphoid tissues that were first identified in the omentum where they were termed “milky spots” (158). Later, the presence of FALCs was reported in other VAT depots (i.e., mesenteric, mediastinal, gonadal and pericardial) (159–161). FALCs are in direct contact with adipocytes, lack fibrous capsule (unlike lymph nodes), and contain B cells, T cells, macrophages, dendritic cells, NKT cells, iNKT cells and ILC2s (159). Importantly, FALCs can give rise to germinal centers under certain conditions (162). These small clusters of immune cells behave as a secondary lymphoid organ and are responsible for modulating both innate and adaptive immune responses (163). The presence of FALCs, together with the aforementioned resident adipose immune cells, have led some authors to propose that ATs can be considered as a tertiary lymphoid organ, with hallmarks of innate and adaptive immune responses (164).
The mechanisms by which ATs contribute to immune responses may be via direct effects of adipose immune cells, and/or via indirect effects whereby adipocytes modulate immunity and inflammation through endocrine, paracrine, autocrine or juxtacrine mechanisms of action. As endocrine organs, ATs communicate with other organs (including immunological organs) via the synthesis and secretion of a multitude (>600) of molecules typically referred to as “adipokines”, such as TNFα, IL-6, chemerin, resistin, visfatin, vaspin, irisin, omentin-1, lipocalin-2, apelin, adiponectin and leptin, to name but a few (15, 50, 165–167). In ATs, adipokines can be released by adipocytes, preadipocytes, adipose resident and -infiltrated immune cells, or other cell types. In addition to their regulation of ATs’ metabolic homeostasis, some adipokines have immunoregulatory and inflammatory functions. For example, adiponectin has anti-inflammatory properties (168) and can negatively regulate macrophage function (169). In contrast, leptin is a proinflammatory factor (170) with broad actions on both the innate (activation of monocytes/macrophages, neutrophils and NK cells) and adaptive (promotion of CD4+ T cell proliferation and IL-2 secretion) immunity (171, 172).
In addition, accumulating evidence indicates that adipocytes can behave as immune cells and sense inflammatory cues; thereby playing an important role in shaping immune responses. Indeed, adipocytes have the ability to express innate immune receptors (173), produce proinflammatory cytokines (50), express chemokines (174), and present antigens (175–177). Most recently, Caputa et al., reported that adipocytes can be licensed by adipose innate immune cells (i.e., NK cells and iNKT cells) to acquire anti-bacterial functions during Listeria monocytogenes infection; this highlights the extra-metabolic capacity of adipocytes to actively participate in the immune response to bacterial infection (178). Such fascinating findings highlight the intricacy and potential for adipocytes to robustly modulate ATs and systemic inflammation that in turn impact global immune responsiveness under homeostatic and disease state conditions.
Adipose tissues and host defense against microbial infections
Unprecedented changes are occurring worldwide: populations age and the prevalence of obesity and related comorbidities continue to increase. This represents a major health challenge since obesity and aging both predispose to health complications (including diabetes, cardiovascular diseases, and cancer), and increase the risk of infections [including respiratory infections, such as influenza (179, 180) and COVID-19 (181)] and premature death. Both obesity and aging are characterized by enhanced low‐grade chronic inflammation and altered innate and adaptive immune cell functions, which contribute to the impaired immune surveillance and host defense in obese and aged individuals (182). Another feature is shared by obesity and aging i.e., the pathological expansion of WAT in general and VAT depot in particular, together with changes in adipose immune cell composition (48, 183); this suggests that WAT remodeling occurring in aged and obese individuals may contribute to the markedly increased vulnerability of these at-risk populations.
Next, we will show that ATs can be targeted by several pathogens that can eventually accumulate and persist in the tissue, and even be a site of active immune responses to infection.
Adipose tissues can be “the place-to-be-and-stay” for some pathogens
Pathogens that have been reported to infect ATs, and eventually persist within, are listed in Table 2.
Bacteria
Mycobacterium tuberculosis (Mtb), the etiological agent of tuberculosis, has the ability to persist in its host for a long time. However, aside from the lungs and regional lymph nodes (which are the main targets for Mtb), the other locations of the bacilli in latently infected patients remain incompletely identified (228). WAT may constitute, among others, an important reservoir for M. tuberculosis. Indeed, Mtb could be isolated from the SCAT and several VAT depots (i.e., perigonadal, perirenal, and mesenteric) of infected mice (184). In individuals with active or latent tuberculosis, Mtb was found in perinodal WAT (active tuberculosis) and in perirenal VAT, abdominal SCAT, and perinodal WAT (latent tuberculosis) (185). Furthermore, Neyrolles and colleagues showed that Mtb, after binding to scavenger receptors, can infect adipocytes in vitro, where it persists in a non-replicating, dormant, state (185). Importantly, the presence and persistence of Mtb in WAT was shown to modulate adipose tissue’s physiology. M. tuberculosis infection drives the recruitment of Mtb-specific IFNγ+ CD8+ T cells and IFNγ+ NK cells into the WAT, indicative of local inflammation (186). More recently, it has been reported that M. tuberculosis infection also stimulates the infiltration of inflammatory immune cells in BAT (229). Interestingly, infection was associated with adipocyte hypertrophy in both WAT and BAT, thereby modulating whole-body energy metabolism (229). The important contribution of WAT to the host’s pathophysiological response to M. tuberculosis infection was confirmed through the use of transgenic inducible “fatless” mice (230). Using this elegant approach, the authors demonstrated that the loss of fat cells during the course of Mtb infection promote the severity of pulmonary pathogenesis. Of note that Mycobacterium canettii, a rare representative of the M. tuberculosis complex, has been reported to successfully infect preadipocytes and adipocytes in vitro (231). However, while WAT is undoubtedly a reservoir for Mtb, it is an unlikely sanctuary for M. canettii, and it is still an open question whether M. canettii and M. tuberculosis can persist in BAT.
Coxiella burnetii (C. burnetti), the agent of Q fever, is known to persist in humans and rodents (232). Bechah and colleagues identified WAT (SCAT and VAT) and BAT as tissue reservoirs for the bacterium: C. burnetii can persist in mouse WAT and BAT for at least four months after infection, while it was not detected in the blood, spleen, liver or lungs as early as 30 days of infection (187). Importantly, the transfer of VAT from convalescent mice to naive immunodeficient mice resulted in the infection of the recipient animals. Analysis of C. burnetii localization in WAT demonstrated that the bacterium targets macrophages and adipocytes. In vitro infection of mouse adipocytes showed that C. burnetii can infect and replicate within adipocytes, where it resided in late phagosomes (187). Whether ATs are reservoirs for C. burnetii in humans remains to be shown.
Rickettsia prowazekii (R. prowazekii) is the causative agent of epidemic typhus, also called louse-borne typhus. Until recently, epidemic typhus was considered a disease of the past, however re-emergences have been reported (233). R. prowazekii can infect and replicate in adipocytes in vitro, and the bacterium was detected in murine WAT, but not in liver, spleen, lung, or central nervous system, up to four months after recovery from the primary infection, suggesting a role for WAT as a potential reservoir for dormant infections with R. prowazekii (188). Whether ATs are reservoirs for R. prowazekii in humans remains to be shown.
Listeria monocytogenes (L. monocytogenes) is a food-borne bacterium responsible for a disease called listeriosis, which is potentially lethal in immunocompromised individuals (234). It has been reported that L. monocytogenes can infect adipocytes in vitro, inducing the upregulation of the expression of the genes coding monocyte chemoattractant protein-1 (MCP-1, involved in macrophage recruitment) and adiponectin (an anti-inflammatory, insulin-sensitizing adipokine) (189). In addition, L. monocytogenes was detected in the WAT of infected obese mice (189). Recently, Caputa and colleagues explored the role of perinodal WAT in the immune response to L. monocytogenes (178). Contrary to their expectations, perinodal WAT was not required for the development of the adaptive immune response during infection. However, the authors found that, over the course of infection, perinodal WAT adipocytes became infected and were rapidly cleared of bacteria. Importantly, the authors demonstrated that infected adipocytes initiated a transcriptional response to IFN-γ (produced by adipose NK and iNKT cells) and shifted away from lipid metabolism toward anti-bacterial functions (178). These major results clearly demonstrate the repurposing of adipocytes away from lipid metabolism toward fighting infection.
Chlamydophila pneumoniae (C. pneumonia), renamed from Chlamydia pneumoniae in 1999, causes respiratory tract infections such as pneumonia. The potential effect of C. pneumoniae infection on fat cells was first investigated by Shi and colleagues in 2008: C. pneumonia can successfully infect preadipocytes and adipocytes in vitro. Strikingly, infection of preadipocytes impaired their differentiation towards fully mature and insulin-sensitive adipocytes through a TNFα-mediated inflammatory mechanism (190). Later on, it was reported that C. pneumoniae proliferated in in-vitro-infected mouse adipocytes by inducing lipolysis, thereby acquiring energy for its own replication to the detriment of host’s lipid metabolism pathway (191). The same authors further reported that C. pneumoniae infection robustly induces fatty acid-binding protein 4 (FABP4, an intracellular lipid chaperone) secretion from adipocytes partly by stimulating the endoplasmic reticulum stress/unfolded protein response (235). However, no reports are available yet on the detection of C. pneumonia in ATs.
Staphylococcus aureus (S. aureus) is a commensal bacterium and opportunistic pathogen, causing potentially fatal disease. It represents the most frequently isolated human bacterial pathogen from a range of diseases including e.g., sepsis, pneumonia, and skin infections (236, 237). S. aureus is able to infect adipocytes in vitro, and to survive inside these cells (192). Adipocyte infection with S. aureus decreases adiponectin and resistin release whereas visfatin, monocyte chemoattractant protein-1 (MCP-1), and IL-6 secretion are increased (192). Because adipocyte viability is not affected during infection, it has been proposed that ATs (and adipocytes within) might function as hosts for S. aureus chronic infection. In vivo, Zhang and colleagues reported massive expansion of the DAT in response to a S. aureus subdermal infection in mice, resulting from both increased adipocyte size (hypertrophy) and number (hyperplasia) (18). Importantly, the authors showed that the local expansion of dermal fat produces the antimicrobial peptide cathelicidin [which has been described to inhibit bacterial growth, stimulate neutrophils and exert proinflammatory activities (238)], but this response appears to decline as adipocytes mature (18). The defective cathelicidin production by mature adipocytes may explain observations of elevated susceptibility to S. aureus infection during obesity and insulin resistance that have been reported in experimental mouse models (239, 240) and in humans (241, 242). Another possible explanation for this apparent discrepancy is that insulin resistance, leptin resistance and/or other aspects of the metabolic syndrome may perturb the infection/adipogenesis/cathelicidin pathway identified by Zhang and colleagues (18). Data on the regulation of cathelicidin are still scarce but signaling by adipose-derived hormones such as resistin could potentially influence its expression; Hochberg and colleagues indeed reported a positive correlation between circulating cathelicidin and resistin levels in obese subjects undergoing a bariatric surgery (243). Besides, cathelicidin is post-translationally cleaved to its active form (244), however, whether this process could also be influenced by factors that are modulated in obesity and metabolic syndrome remains to be seen. In contrast to WAT, S. aureus infection has no impact on BAT (245). However, the relevance of these observations during S. aureus infection in humans still needs to be elucidated and has to be investigated in future studies.
Parasites
Three parasite species are uniquely associated with ATs during their life cycle: Trypanosoma cruzi, the causative agent of Chagas disease; Trypanosoma brucei, the causative agent of African sleeping sickness; and Plasmodium spp., the causative agents of malaria. Recently, it has been reported that Leishmania infantum, responsible of visceral leishmaniasis, can also reside in ATs.
In 1970, Shoemaker and colleagues first reported the presence of Trypanosoma cruzi (T. cruzi) in the BAT of infected mice (194). Later on, a high number of parasites was also reported in the WAT of infected mice (195, 196). Using acute and chronic mouse models of T. cruzi infection, it was subsequently demonstrated that the parasite directly infects ATs (both WAT and BAT) where it can persist, notably in adipocytes (246, 247). In humans, T. cruzi was found in the SCAT of patients with chronic Chagas disease, confirming the role of ATs as tissue reservoirs for the parasite from which recrudescence may occur during immunosuppression (248). The presence of T. cruzi in ATs is associated with local inflammation (notably increased production of IL-6 and TNFα), macrophage recruitment and oxidative stress, as well as with a reduction in lipid accumulation, adipocyte size, and fat mass partly resulting from increased expression of lipolytic enzymes (247, 249, 250).
African trypanosomiasis (also known as “sleeping sickness”), caused by Trypanosoma brucei (T. brucei), is transmitted by tsetse flies. In mammalian hosts, trypanosomes are thought to exist in two major niches: early in infection, they populate the blood and later, they breach the blood-brain barrier. Trindade and colleagues uncover ATs (both WAT and BAT) as the third, and major, niches where parasites can accumulate and replicate through their functional adaptation to lipid-rich environment (197). Since skin is the entry site for the parasite, many parasites can be found in the vicinity of SCAT adipocytes, but not in adipocytes (198, 199). Thus, like for T. cruzi, there is no doubt that ATs are major T. brucei reservoirs. However, while T. cruzi resides inside adipocytes, T. brucei is found in the interstitial spaces between adipocytes.
Five species of genus Plasmodium are known to cause malaria in humans: Plasmodium falciparum, P. vivax, P. malariae, P. ovale, and P. knowlesi. However, infection with P. falciparum is being accounted for more than 90% of the world’s malaria mortality (251). In addition, P. berghei causes malaria in certain rodents, such as mice (252). Examination of post-mortem tissues obtained from individuals who died from severe malaria revealed that P. falciparum accumulate in WAT (mainly SCAT), although the lung and the spleen are the main sites of parasite accumulation (200, 201). In WAT, the sequestration of P. berghei-infected-red-blood-cells (iRBCs) largely depends on CD36 (202) – a scavenger receptor that regulates the process of lipid storage and lipolysis (253). Importantly, it has been recently reported that iRBCs sequestration in WAT microvasculature increases the production of leptin (254), the circulating levels of which are associated with severe (i.e., cerebral) malaria in mice (255, 256). Thus far, there is no report of Plasmodium spp.-iRBCs sequestration in BAT.
Leishmania spp. are the causative agents of a spectrum of clinical diseases, all termed leishmaniasis. Two species of Leishmania are known to give rise to the visceral form of the disease, which is the most severe form of leishmaniasis: L. donovani and L. infantum. The report of several cases of relapses, even post-treatment, has raised the unresolved question of host sites allowing parasite persistence (257). L. infantum persistence in the VAT of intra-peritoneally infected mice was described in 2011 (203). Recently, it has been reported that L. infantum can reside and persist in the VAT and BAT of intravenously infected mice, mostly in adipocytes (204). However, it remains to be determined whether ATs and adipocytes could be a reservoir for L. infantum in humans.
Viruses
Adenoviruses (Advs) are common viruses that typically cause mild cold- or flu-like illness. More than 80 different Adv types can infect humans (258). The possibility for Advs to be associated with WAT in humans was initially considered in 1997 by Dhurandhar and colleagues, who conducted a pioneer study linking a virus to obesity in humans (259). Adv36 was the first human virus to be identified as causing obesity in animals (260), and the only virus that was related to obesity and/or metabolic alterations in naturally infected humans (261). In non-human primates, it was reported that Adv36 reside in VAT (205), an observation that has been repeatedly extended to humans (206–208). In vitro, Adv36 infection promotes preadipocyte differentiation toward mature, lipid-filled adipocytes (262, 263), partly through the regulation of adipogenesis-related genes such as CCAAT/enhancer-binding protein (C/EBP) α and β, peroxisome proliferator-activated γ (PPARγ) and glycerol-3-phosphate dehydrogenase (GPDH) (264). Importantly, Adv36 infection leads to the decreased production of the proinflammatory, immunoregulatory adipokine leptin (263), and the increased production of the anti-inflammatory adipokine adiponectin (265). To our knowledge, there is to date no information regarding the presence of Adv36 in BAT.
Human immunodeficient virus (HIV) is responsible for the progressive immune dysfunction that leads to acquired immunodeficiency syndrome (AIDS). The notion that ATs act as reservoirs for HIV was first put forward by Dupin and colleagues in 2002 (266). However, the identification of ATs (exclusively WAT, both SCAT and VAT) as long-lived sanctuaries for replicative viruses and major sites of inflammation during chronic HIV (and its simian homologue SIV) infection, came later (209–213). Although adipocytes express the CD4, CCR5, and CXCR4 cell surface receptors necessary for HIV entry, viruses seldomly infected cultured adipocytes and, when entry does occur, the production of viral particles is relatively poor (267, 268); adipocytes are thus not likely to be the major cell hosts in WAT. In fact, HIV (and SIV) was detected in WAT’s stromal vascular fraction (209), notably in CD4+ T cells (210, 212). Of note that unambiguous data on adipose macrophages are missing: the virus was found in these cells in the SIV infection model but not in samples from HIV-infected patients (212).
Cytomegalovirus (CMV) is a wide-spread virus, with manifestations ranging from asymptomatic to severe end-organ dysfunction in immunocompromised patients with congenital CMV disease (269). During the last decades, CMV was implicated in the pathogenesis of atherosclerosis and cardiovascular disorders (270) as well as prostate cancer (271). CMV can infect preadipocytes and adipocytes in vitro (272, 273), and it has been recently reported that mouse CMV infects VAT, resulting in the durable enrichment of cytotoxic CMV-specific CD8+ T cells and that carry markers of tissue residence (216). Of note that the presence of CMV in BAT has been reported in an acute model of infection in rodents (217, 218).
Lymphocytic choriomeningitis virus (LCMV) is an important cause of neurologic diseases in humans (274). It has been recently reported that LCMV infects WAT (only reported for the perigonadal VAT depot) and adipocytes (214). Virus-specific T cells accumulate in the WAT and are likely responsible for clearing infection there. These T cells then differentiate into memory T cells that appear transcriptionally distinct from memory T cells in circulation or in lymphoid tissues (214). Whether SCAT and/or BAT can also be targeted by LCMV remains unknown.
Evidences for the contribution of adipose tissues to the host’s memory/recall response to pathogens
Tissue-resident memory CD8+ T cells (TRM) are a distinct memory population that is generated and persists at the infection site (275–277). Upon exposure to the same (or similar) pathogen, TRM cells provide a first line of adaptive cellular defense and are crucial in lethal challenge models (278). In mice, non-human primates and humans, it has been shown that ATs (only reported for WAT) harbor many TRM cells (279). Interestingly, WAT’s TRM cells metabolically adapt to the tissue through up-regulating genes involved in lipid metabolism, indicating that they use fatty acid metabolism for their homeostasis (280).
So far, only few reports have underscored that ATs act as reservoirs for CD8+ TRM cells: after infection (VAT) (193, 214), dietary restriction (MAT) (281), and obesity (VAT) (214, 282, 283), thereby likely participating to adaptive immune responses.
Han and colleagues reported that, following T. gondii or Y. pseudotuberculosis infection, nearly half of all CD8+ T cells within WAT are specific for the administered pathogen, suggesting that CD8+ T cells home to and persist (as CD8+ TRM cells) within WAT after infection (193). Importantly, the authors also demonstrated that the induction of memory T cell responses in WAT results in the remodeling of WAT’s physiology in favor of the activation of antimicrobial response at the expense of lipid metabolism (193). These observations were the first to identify WAT as immune reservoirs sustaining pool of memory T cells and facilitating their reactivation, thus potentially contributing to immunological memory after infection. Whether WAT’s TRM cells are important for recall responses to T. gondii and/or Y. pseudotuberculosis reinfections in humans remains to be seen.
Misumi and colleagues brought the second demonstration of an active anti-infectious role of ATs’ memory T cells (214). Following lymphocytic choriomeningitis virus infection, the authors showed that more memory IFNγ+ CD8+ T cells accumulate in the WAT of obese mice than in the WAT of lean mice. Strikingly, WAT memory T cells of obese mice rapidly caused lethal immunopathology upon re-challenge infection, whereas lean mice remained unaffected and could efficiently control the challenge (214). This demonstrates that obesity gives rise to an unusual form of T-cell-mediated pathogenesis during viral infection that leads to lethality.
Altogether, these major findings illustrate the importance of AT’s T cells to infection and in memory/recall responses and may have implications for the management of infections in individuals having alterations in AT’s immune cell composition and function, such as individuals with obesity and aged adults, who display impaired immune responses to pathogens.
In addition, unlike stereotypical lymphoid organs that primarily contain adaptive immune cells, ATs are enriched with several types of innate immune cells (e.g., macrophages, DCs), as well as with ILCs and innate-like T cells (e.g., iNKT cells, γδ T cells) (Table 1), which are likely contributing to host defense against infection. Indeed, adipose iNKT cells and γδ T cells have been reported to mediate host defense by modulating the number and/or function of other adipose immune cells (e.g., Tregs, DCs, NK cells and macrophages) (284), and DCs were shown to control adaptive immune responses to AT’s infection (133, 134, 285, 286).
The special case of influenza A and SARS-CoV-2 respiratory viruses
Since the last twenty years, a growing number of new viruses has emerged and entered the human population (287). Moreover, influenza A (H1N1)pdm09 virus and severe acute respiratory syndrome coronavirus-2 (SARS-CoV-2) have caused global pandemics (288, 289). Epidemiological evidence establishes obesity and aging – two conditions associated with ATs dysfunctions – as important risk factors for increased susceptibility and severity to viral respiratory pneumonias associated with H1N1 and SARS-CoV-2 pandemics (290–292). This has prompted some researchers to (re)address the role of ATs in influenza and COVID-19. In this section, we will examine the impact of influenza virus and SARS-CoV-2 infection on ATs (Table 2), and comment on the contribution of ATs to influenza and COVID-19 pathogenesis.
Influenza A viruses (IAVs) are very contagious pathogens responsible for severe respiratory illnesses in humans and animals worldwide. IAVs are enveloped, segmented negative-sense, single-stranded RNA viruses that primarily infect respiratory epithelial cells (293). With the exception of the reports showing that IAV can infect adipocytes in vitro (272), and that IAV (H5N1) can target WAT in vivo (219), no study has investigated the impact of influenza infection on the WAT of lean mice until recently. Ayari and colleagues evaluated the metabolic consequences of IAV (H3N2) infection in lean mice, and the impact of infection on SCAT and VAT (220). The authors showed that IAV-infected mice present alterations in whole-body energy metabolism that persisted after the resolution of the infection. Importantly, during the acute phase of infection, viral RNA was detected in SCAT and, at a lower level, in VAT. Concomitantly, viral-antigen-harboring hematopoietic cells (i.e., expressing CD45, a leukocyte common antigen) were found in WAT (mostly in SCAT), and this was associated with the parallel activation of type I IFN signaling pathways and inhibition of cholesterol biosynthesis pathways, suggesting activation of antiviral innate immune responses. In addition, IAV infection induced a transient metabolic rewiring in SCAT (not VAT) – characterized by the emergence of UCP1-expressing thermogenic brown-like/beige adipocytes in the tissue. The latter may partly rely on a direct effect of the virus on SCAT: preadipocytes committed to the thermogenic differentiation program upon in vitro IAV infection (220). Since a growing body of evidence suggests that immune cells are highly sensitive to thermal stress (294, 295), SCAT browning may participate to the initial steps of immune defense against the infection. Thus, influenza-induced SCAT thermogenesis might help controlling antiviral immune defenses, locally. From the virus side, it is conceivable that, since the host expends energy to increase SCAT thermogenesis, it fails to support an efficient immune response against infection locally, thereby allowing the virus to propagate for a longer period of time in the tissue (296). At this stage, many questions remain unanswered about how the virus and/or infected cells reach the WAT, what is the phenotype of these cells, and do they participate to the host’s defense against infection?
In 2021, Zeng and colleagues reported that IAV can also infect the mesenteric VAT. In this fat pad, infection was associated with moderate decreased adipocyte number, increased release of leptin, visfatin and chemerin, and decreased release of adiponectin (221). The dissemination of IAV to ATs has been recently extended to the perivascular AT in a pregnant mouse model of infection (222). In this model, IAV preferentially disseminated into the perivascular AT of the abdominal aorta, and this was associated with the recruitment of proinflammatory monocytes and neutrophils, and the massive infiltration and activation of T cells. This suggests that during pregnancy, the perivascular AT might be a niche that supports IAV dissemination and a site of marked inflammation and vascular dysfunction, which may be central to the maternal and perinatal complications following IAV infection during pregnancy (297).
Altogether, these findings demonstrate that, during the acute phase of infection, IAV can disseminate to different adipose depots (SCAT and epidydimal VAT) (220), mesenteric VAT (221), and perivascular VAT of the abdominal aorta (222). To our knowledge, there is no published report on IAV targeting BAT.
Coronaviruses are responsible for mild to severe respiratory tract infections, similarly to influenza viruses (298). Coronaviruses are enveloped, non-segmented positive sense, single-stranded RNA viruses. To date, seven coronaviruses associated with human infections have been identified, such as the severe acute respiratory syndrome coronavirus-2 (SARS-CoV-2) (299), which caused the coronavirus disease 2019 (COVID-19) pandemic (300). Since adipose cells express ACE2 – the major cell entry receptor for SARS-CoV-2 (301) – and virus replication and its inflammatory insult are favored by the presence of lipid droplets (302), the hypothesis that ATs may serve as reservoir for storing and replicating the virus, as well as a site for cytokine amplification, has emerged as a potential explanation for the strong association between obesity, aging and COVID-19 severity (303, 304). Recent studies have shown that SARS-CoV-2 can indeed infect ATs’ cells, including adipocytes, thus favoring a local inflammatory response and resulting changes in lipid profiles (223–226, 305–307). In turn, these changes are thought to contribute to insulin resistance and to hamper patients’ recovery.
Reiterer and colleagues were the first to suggest that SARS-CoV-2 triggers WAT dysfunction, which in turn contributes to adverse COVID-19 outcomes (223). Indeed, these investigators reported that mouse (but not human) adipocytes infected with SARS-CoV-2 in vitro produced lower amounts of adiponectin – an insulin-sensitizing adipokine (308). Importantly, viral RNA and low adiponectin expression were evidenced in WAT from infected golden hamsters. These changes were associated with a robust inflammatory antiviral response in WAT and a systemic insulin-resistant state, suggesting that hyperglycemia in severe COVID-19 might result (at least in part) from infection-induced WAT dysfunction (223). Zickler and colleagues showed SARS-CoV-2 is frequently detectable in the WAT (SCAT and VAT) of patients deceased from COVID-19 (225). Remarkably, the virus was detected predominantly in the WAT of males who were overweight or obese. In female individuals, SARS-CoV-2 was also detected in WAT, although there was no clear correlation between fat mass and virus mRNA levels. In a hamster model of COVID-19, the authors also showed that SARS-CoV-2 spreads from the respiratory tract into WAT, where it continues to replicate, thereby leading to local inflammation and changes in whole-body metabolism. In in vitro infection experiments, the authors showed that ACE2 expression was strongly induced upon adipocyte differentiation, and provided mechanistic insight that lipid droplet metabolism is critical for SARS-CoV-2 propagation, since blocking lipid breakdown drastically reduced viral replication in mature adipocytes (225). Martinez-Colon and colleagues identified mature, lipid-laden adipocytes and macrophages as the two main cellular targets of SARS-CoV-2 in human WAT (226). Strikingly, preadipocytes are not permissive to infection – reinforcing the notion that lipid droplet metabolism is critical for SARS-CoV-2 propagation (225, 302). Colleluori and colleagues confirmed SARS-CoV-2’s ability to infect mature adipocytes and further showed that infected adipocytes are less viable and have a smaller lipid droplet size and a higher prevalence of pyknotic nuclei, which are suggestive of infection-induced cell delipidation and death (305). In human individuals who deceased from COVID-19, Basolo and colleagues recently confirmed the presence of the SARS-CoV-2 genome in the SCAT, and further showed that SARS-CoV-2 infection activates the IFN-alpha pathway and induces the recruitment of NK cells, macrophages and T cells in the tissue (306). Most recently, Saccon and colleagues confirmed that WAT (i.e., thoracic SCAT) is a frequent extrapulmonary site where SARS-CoV-2 can be detected in patients who died from COVID-19 (307). Using adipocytes differentiated from primary stromal-vascular cells isolated from the SCAT or the VAT of individuals undergoing bariatric surgery, the authors also provided evidence that VAT adipocytes are more susceptible than SCAT adipocytes to SARS-CoV-2 infection in vitro (307). Although these observations were made in vitro, it appears that SARS-CoV-2 infection may have a depot-specific impact on ATs, likely due to the intrinsic differences between SCAT and VAT with regard to their cellular, molecular, and physiological characteristics, notably regarding lipid metabolism (47, 48). In a non-human primate model of COVID-19, SARS-CoV-2 was detected in SCAT but not in VAT or in epicardial AT (309). However, when evaluating the consequences of SARS-CoV-2 infection on WAT T cells, the authors showed that both SCAT and VAT T cells showed a drastic reduction in CD69 expression (309) – a standard marker of T cell activation and residency in tissues (310–312) that is expressed by most tissue resident memory T cells (312–314). Thus, the loss of CD69 expression on SCAT and VAT T cells in SARS-CoV-2-infected animals might reflect a major change in the migratory potential of adipose T cells, and/or functional alterations in adipose-resident-T cells caused by inappropriate activation (315). Besides, it is acknowledged that T cell activation requires a coordinated rewiring of cellular metabolism (to support increase in cell size and clonal expansion, lineage polarization, and acquisition of effector function) – a process that is likely to be dependent upon tissue’s metabolic rates and fuel requirements (316). Importantly, it has been reported that CD69 can function as a metabolic gatekeeper in T cells, notably regarding amino acid uptake (317). This demonstrates that in a model of mild infection, SCAT is selectively infected by SARS-CoV-2 although changes in the immune properties of AT are observed in both SCAT and VAT (309). Finally, while no reports are currently available on the detection of SARS-CoV-2 in BAT, it is noteworthy that ACE2 has been reported to be highly expressed in BAT, where it participates to the maintenance of thermogenesis and energy expenditure through the direct induction of UCP1 and activation of mitochondrial function (318).
Altogether, these findings demonstrated that ATs infection (mainly described for WAT) represents a relevant feature of COVID-19 and thus should be considered when investigating the mechanisms of disease pathogenesis (including immune responses), notably in at risk-populations such as obese and aged individuals.
Concluding remarks
Historically viewed as inert sites for energy storage, adipose tissues (ATs) are now appreciated as important regulators of many aspects of whole-body physiology, including immune responses. In this review, we remind that ATs harbor a plethora of innate and adaptive immune cells that shape their endocrine and metabolic functions and contribute to tissue repair and homeostasis as well as to immunosurveillance and immunoregulation. These last years, a wide range of pathogens (bacteria, parasites or viruses such as the respiratory viruses influenza and SARS-CoV-2) have been reported to target ATs, and eventually persist within. Notably, since ATs express ACE2 and SARS-CoV-2 replication is favored by the presence of lipid droplets, the hypothesis that ATs may constitute major reservoirs for viral shedding/spread and potentiators of local and systemic inflammation, has emerged as a possible explanation for the severe forms of COVID-19 in obese and aged individuals as well as for the enduring post-infection symptoms collectively termed long COVID. More generally, the demonstration that both some infections can persist in ATs that act as sources of subsequent disease, expands the knowledge of host-pathogen interactions, in which ATs should not be ignored. Beside inducing metabolic and inflammatory changes in the tissue, we describe how these infections also lead to alterations in the adipose immune landscape. We finally touch upon recent groundbreaking evidence implicating adipose resident memory T cells in host defense against certain pathogens, which show how vital the adipose immune system is for host defense against infection. The translatability of these major breakthroughs to humans still remains to be established. Nevertheless, these fascinating results might allow to understand the differences in disease pathogenesis in fragile populations such as the elderly and obese individuals – who present with quantitative and qualitative changes in adipose immune cells. Increasing our understanding of the role of ATs immune cells might ultimately lead to the development of innovative preventive/therapeutic strategies (targeted immunomodulation) for the treatment of infectious diseases and effective vaccination in at-risk populations.
Author contributions
All authors listed have made a substantial, direct, and intellectual contribution to the work (reviewing of literature, writing and editing the manuscript, designing Figures and Tables), and approved the submitted version of the manuscript.
Funding
This study was supported by the Centre National de la Recherche Scientifique (CNRS), the Institut National de la Santé et de la Recherche Médicale (INSERM), the University of Lille and the Institut Pasteur de Lille. JB received a grant (PhD fellowship) from Lille University and from the Institut Pasteur de Lille. GB received a grant (PhD fellowship) from Lille University. IW received salary support by CNRS.
Acknowledgments
The authors warmly acknowledge Dr. Corinne Grangette for fruitful discussions.
Conflict of interest
The authors declare that the research was conducted in the absence of any commercial or financial relationships that could be construed as a potential conflict of interest.
Publisher’s note
All claims expressed in this article are solely those of the authors and do not necessarily represent those of their affiliated organizations, or those of the publisher, the editors and the reviewers. Any product that may be evaluated in this article, or claim that may be made by its manufacturer, is not guaranteed or endorsed by the publisher.
References
2. Rosen ED, Spiegelman BM. What we talk about when we talk about fat. Cell (2014) 156:20–44. doi: 10.1016/j.cell.2013.12.012
3. Ayari A, Wolowczuk I. Physiology and pathophysiology of adipose tissue-derived cytokine networks. In: Cytokine effector functions in tissues. Academic Press. Elsevier Inc (2017). p. 33–50. doi: 10.1016/B978-0-12-804214-4.00001-4
4. Zwick RK, Guerrero-Juarez CF, Horsley V, Plikus MV. Anatomical, physiological and functional diversity of adipose tissue. Cell Metab (2018) 27:68–83. doi: 10.1016/j.cmet.2017.12.002
5. Gaspar RC, Pauli JR, Shulman GI, Muñoz VR. An update on brown adipose tissue biology: a discussion of recent findings. Am J Physiol Endocrinol Metab (2021) 320:E488–95. doi: 10.1152/ajpendo.00310.2020
6. Wu J, Cohen P, Spiegelman BM. Adaptive thermogenesis in adipocytes: Is beige the new brown? Genes Dev (2013) 27:234–50. doi: 10.1101/gad.211649.112
7. Weisberg SP, McCann D, Desai M, Rosenbaum M, Leibel RL, Ferrante AW Jr.. Obesity is associated with macrophage accumulation in adipose tissue. J Clin Invest (2003) 112:1796–808. doi: 10.1172/JCI200319246
8. Ferrante AW. The immune cells in adipose tissue. Diabetes Obes Metab (2013) 15 Suppl:3, 34–38. doi: 10.1111/dom.12154
9. Kane H, Lynch L. Innate immune control of adipose tissue homeostasis. Trends Immunol (2019) 40:857–72. doi: 10.1016/j.it.2019.07.006
10. Cox AR, Chernis N, Masschelin PM, Hartig SM. Immune cells gate white adipose tissue expansion. Endocrinology (2019) 160:1645–58. doi: 10.1210/en.2019-00266
11. Grant RW, Dixit VD. Adipose tissue as an immunological organ. Obes Silver Spring Md (2015) 23:512–8. doi: 10.1002/oby.21003
12. Sun W, Modica S, Dong H, Wolfrum C. Plasticity and heterogeneity of thermogenic adipose tissue. Nat Metab (2021) 3:751–61. doi: 10.1038/s42255-021-00417-4
13. Knights AJ, Wu J, Tseng Y-H. The heating microenvironment: Intercellular cross talk within thermogenic adipose tissue. Diabetes (2020) 69:1599–604. doi: 10.2337/db20-0303
14. Agueda-Oyarzabal M, Emanuelli B. Immune cells in thermogenic adipose depots: The essential but complex relationship. Front Endocrinol (2022) 13. doi: 10.3389/fendo.2022.839360
15. MacLaren R, Cui W, Cianflone K. Adipokines and the immune system: an adipocentric view. Adv Exp Med Biol (2008) 632:1–21. doi: 10.1007/978-0-387-78952-1_1
16. Schäffler A, Schölmerich J. Innate immunity and adipose tissue biology. Trends Immunol (2010) 31:228–35. doi: 10.1016/j.it.2010.03.001
17. Chen SX, Zhang L-J, Gallo RL. Dermal white adipose tissue: A newly recognized layer of skin innate defense. J Invest Dermatol (2019) 139:1002–9. doi: 10.1016/j.jid.2018.12.031
18. Zhang L, Guerrero-Juarez CF, Hata T, Bapat SP, Ramos R, Plikus MV, et al. Dermal adipocytes protect against invasive staphylococcus aureus skin infection. Science (2015) 347:67–71. doi: 10.1126/science.1260972
19. Song J, Deng T. The adipocyte and adaptive immunity. Front Immunol (2020) 11. doi: 10.3389/fimmu.2020.593058
20. Cinti S. Adipose tissues and obesity. Ital J Anat Embryol Arch Ital Anat Ed Embriol (1999) 104:37–51.
21. Cinti S. The Adipose Organ. In: Fantuzzi G., Mazzone T. (eds) Adipose Tissue and Adipokines in Health and Disease. Nutrition and Health. Humana Press. (2007). doi: 10.1007/978-1-59745-370-7_1
22. Kajimura S. Adipose tissue in 2016: Advances in the understanding of adipose tissue biology. Nat Rev Endocrinol (2017) 13:69–70. doi: 10.1038/nrendo.2016.211
23. Cinti S. The adipose organ. In: Fantuzzi G, Mazzone T, editors. Adipose tissue and adipokines in health and disease. Humana Press (2007). p. 3–19. doi: 10.1007/978-1-59745-370-7_1
24. Zhang F, Hao G, Shao M, Nham K, An Y, Wang Q, et al. An adipose tissue atlas: An image-guided identification of human-like BAT and beige depots in rodents. Cell Metab (2018) 27:252–262.e3. doi: 10.1016/j.cmet.2017.12.004
25. De Fano M, Bartolini D, Tortoioli C, Vermigli C, Malara M, Galli F, et al. Adipose tissue plasticity in response to pathophysiological cues: A connecting link between obesity and its associated comorbidities. Int J Mol Sci (2022) 23:5511. doi: 10.3390/ijms23105511
26. Sakers A, De Siqueira MK, Seale P, Villanueva CJ. Adipose-tissue plasticity in health and disease. Cell (2022) 185:419–46. doi: 10.1016/j.cell.2021.12.016
27. Pellegrinelli V, Carobbio S, Vidal-Puig A. Adipose tissue plasticity: how fat depots respond differently to pathophysiological cues. Diabetologia (2016) 59:1075–88. doi: 10.1007/s00125-016-3933-4
28. Lee Y-H, Mottillo EP, Granneman JG. Adipose tissue plasticity from WAT to BAT and in between. Biochim Biophys Acta BBA Mol Basis Dis (2014) 1842:358–69. doi: 10.1016/j.bbadis.2013.05.011
29. Saely CH, Geiger K, Drexel H. Brown versus white adipose tissue: a mini-review. Gerontology (2012) 58:15–23. doi: 10.1159/000321319
30. Cinti S. Adipose organ development and remodeling. Compr Physiol (2018) 8:1357–431. doi: 10.1002/cphy.c170042
31. Morigny P, Boucher J, Arner P, Langin D. Lipid and glucose metabolism in white adipocytes: pathways, dysfunction and therapeutics. Nat Rev Endocrinol (2021) 17:276–95. doi: 10.1038/s41574-021-00471-8
32. Hull D, Segall MM. Distinction of brown from white adipose tissue. Nature (1966) 212:469–72. doi: 10.1038/212469a0
33. Rothwell NJ, Stock MJ. A role for brown adipose tissue in diet-induced thermogenesis. Obes Res (1997) 5:650–6. doi: 10.1002/j.1550-8528.1997.tb00591.x
34. Klingenspor M. Cold-induced recruitment of brown adipose tissue thermogenesis. Exp Physiol (2003) 88:141–8. doi: 10.1113/eph8802508
35. Scheller EL, Burr AA, MacDougald OA, Cawthorn WP. Inside out: Bone marrow adipose tissue as a source of circulating adiponectin. Adipocyte (2016) 5:251–69. doi: 10.1080/21623945.2016.1149269
36. Scheller EL, Cawthorn WP, Burr AA, Horowitz MC, MacDougald OA. Marrow adipose tissue: Trimming the fat. Trends Endocrinol Metab TEM (2016) 27:392–403. doi: 10.1016/j.tem.2016.03.016
37. Lecka-Czernik B. Marrow fat metabolism is linked to the systemic energy metabolism. Bone (2012) 50:534–9. doi: 10.1016/j.bone.2011.06.032
38. Hardouin P, Rharass T, Lucas S. Bone marrow adipose tissue: To be or not to be a typical adipose tissue? Front Endocrinol (2016) 7:85. doi: 10.3389/fendo.2016.00085
39. Cornish J, Wang T, Lin J. Role of marrow adipocytes in regulation of energy metabolism and bone homeostasis. Curr Osteoporos Rep (2018) 16:116–22. doi: 10.1007/s11914-018-0425-0
40. Suchacki KJ, Cawthorn WP. Molecular interaction of bone marrow adipose tissue with energy metabolism. Curr Mol Biol Rep (2018) 4:41–9. doi: 10.1007/s40610-018-0096-8
41. Gesta S, Tseng Y-H, Kahn CR. Developmental origin of fat: Tracking obesity to its source. Cell (2007) 131:242–56. doi: 10.1016/j.cell.2007.10.004
42. Luong Q, Huang J, Lee KY. Deciphering white adipose tissue heterogeneity. Biology (2019) 8. doi: 10.3390/biology8020023
43. Abdullahi A, Jeschke MG. White adipose tissue browning: A double-edged sword. Trends Endocrinol Metab TEM (2016) 27:542–52. doi: 10.1016/j.tem.2016.06.006
44. Frontini A, Cinti S. Distribution and development of brown adipocytes in the murine and human adipose organ. Cell Metab (2010) 11:253–6. doi: 10.1016/j.cmet.2010.03.004
45. Townsend K, Tseng Y-H. Brown adipose tissue: Recent insights into development, metabolic function and therapeutic potential. Adipocyte (2012) 1:13–24. doi: 10.4161/adip.18951
46. Mo Q, Salley J, Roshan T, Baer LA, May FJ, Jaehnig EJ, et al. Identification and characterization of a supraclavicular brown adipose tissue in mice. JCI Insight (2017) 2(11):e93166. doi: 10.1172/jci.insight.93166
47. Wajchenberg BL. Subcutaneous and visceral adipose tissue: their relation to the metabolic syndrome. Endocr Rev (2000) 21:697–738. doi: 10.1210/edrv.21.6.0415
48. Tchkonia T, Thomou T, Zhu Y, Karagiannides I, Pothoulakis C, Jensen MD, et al. Mechanisms and metabolic implications of regional differences among fat depots. Cell Metab (2013) 17:644–56. doi: 10.1016/j.cmet.2013.03.008
49. Tran TT, Yamamoto Y, Gesta S, Kahn CR. Beneficial effects of subcutaneous fat transplantation on metabolism. Cell Metab (2008) 7:410–20. doi: 10.1016/j.cmet.2008.04.004
50. Kershaw EE, Flier JS. Adipose tissue as an endocrine organ. J Clin Endocrinol Metab (2004) 89(6):2548–56. doi: 10.1210/jc.2004-0395
51. Shuster A, Patlas M, Pinthus JH, Mourtzakis M. The clinical importance of visceral adiposity: a critical review of methods for visceral adipose tissue analysis. Br J Radiol (2012) 85:1–10. doi: 10.1259/bjr/38447238
52. Driskell RR, Jahoda CAB, Chuong C-M, Watt FM, Horsley V. Defining dermal adipose tissue. Exp Dermatol (2014) 23:629–31. doi: 10.1111/exd.12450
53. Schneider MR. Coming home at last: dermal white adipose tissue. Exp Dermatol (2014) 23:634–5. doi: 10.1111/exd.12438
54. Kasza I, Suh Y, Wollny D, Clark RJ, Roopra A, Colman RJ, et al. Syndecan-1 is required to maintain intradermal fat and prevent cold stress. PloS Genet (2014) 10:e1004514. doi: 10.1371/journal.pgen.1004514
55. Alexander CM, Kasza I, Yen CLE, Reeder SB, Hernando D, Gallo RL, et al. Dermal white adipose tissue: a new component of the thermogenic response. J Lipid Res (2015) 56:2061–9. doi: 10.1194/jlr.R062893
56. Hausman GJ, Basu U, Du M, Fernyhough-Culver M, Dodson MV. Intermuscular and intramuscular adipose tissues: Bad vs. good adipose tissues. Adipocyte (2014) 3:242–55. doi: 10.4161/adip.28546
57. Gong Z, Tas E, Yakar S, Muzumdar R. Hepatic lipid metabolism and non-alcoholic fatty liver disease in aging. Mol Cell Endocrinol (2017) 455:115–30. doi: 10.1016/j.mce.2016.12.022
58. Yki-Järvinen H. Non-alcoholic fatty liver disease as a cause and a consequence of metabolic syndrome. Lancet Diabetes Endocrinol (2014) 2:901–10. doi: 10.1016/S2213-8587(14)70032-4
59. Betz MJ, Enerbäck S. Human brown adipose tissue: What we have learned so far. Diabetes (2015) 64:2352–60. doi: 10.2337/db15-0146
60. Saito M, et al. High incidence of metabolically active brown adipose tissue in healthy adult humans: effects of cold exposure and adiposity. Diabetes (2009) 58:1526–31. doi: 10.2337/db09-0530
61. Lee P, Swarbrick MM, Ho KKY. Brown adipose tissue in adult humans: a metabolic renaissance. Endocr Rev (2013) 34:413–38. doi: 10.1210/er.2012-1081
62. Naveiras O, Nardi V, Wenzel PL, Fahey F, Daley GQ. Bone marrow adipocytes as negative regulators of the hematopoietic microenvironment. Nature (2009) 460:259–63. doi: 10.1038/nature08099
63. Miggitsch C, et al. Human bone marrow adipocytes display distinct immune regulatory properties. EBioMedicine (2019) 46:387–98. doi: 10.1016/j.ebiom.2019.07.023
64. Scheller EL, Doucette CR, Learman BS, Cawthorn WP, Khandaker S, Benjamin Schell B, et al. Region-specific variation in the properties of skeletal adipocytes reveals regulated and constitutive marrow adipose tissues. Nat Commun (2015) 6:7808. doi: 10.1038/ncomms8808
65. Sun K, Kusminski CM, Scherer PE. Adipose tissue remodeling and obesity. J Clin Invest (2011) 121:2094–101. doi: 10.1172/JCI45887
66. Choe SS, Huh JY, Hwang IJ, Kim JI, Kim JB. Adipose tissue remodeling: Its role in energy metabolism and metabolic disorders. Front Endocrinol (2016) 7:30. doi: 10.3389/fendo.2016.00030
67. Jeffery E, Church CD, Holtrup B, Colman L, Rodeheffer MS. Rapid depot-specific activation of adipocyte precursor cells at the onset of obesity. Nat Cell Biol (2015) 17:376–85. doi: 10.1038/ncb3122
68. Tang HN, Tang CY, Man XF, Tan SW, Guo Y, Tang J, et al. Plasticity of adipose tissue in response to fasting and refeeding in male mice. Nutr Metab (2017) 14:3. doi: 10.1186/s12986-016-0159-x
69. Chouchani ET, Kazak L, Spiegelman BM. New advances in adaptive thermogenesis: UCP1 and beyond. Cell Metab (2019) 29:27–37. doi: 10.1016/j.cmet.2018.11.002
70. Berry R, Jeffery E, Rodeheffer MS. Weighing in on adipocyte precursors. Cell Metab (2014) 19:8–20. doi: 10.1016/j.cmet.2013.10.003
71. Ghaben AL, Scherer PE. Adipogenesis and metabolic health. Nat Rev Mol Cell Biol (2019) 20:242–58. doi: 10.1038/s41580-018-0093-z
72. Rodeheffer MS, Birsoy K, Friedman JM. Identification of white adipocyte progenitor cells In vivo. Cell (2008) 135:240–9. doi: 10.1016/j.cell.2008.09.036
73. MacLean PS, Higgins JA, Giles ED, Sherk VD, Jackman MR. The role for adipose tissue in weight regain after weight loss. Obes Rev (2015) 16:45–54. doi: 10.1111/obr.12255
74. Mancuso P, Bouchard B. The impact of aging on adipose function and adipokine synthesis. Front Endocrinol (2019) 10:137. doi: 10.3389/fendo.2019.00137
75. Zoico E, Rubele S, De Caro A, Nori N, Mazzali G, Fantin F, et al. Brown and beige adipose tissue and aging. Front Endocrinol (2019) 10:368. doi: 10.3389/fendo.2019.00368
76. Alcalá M, Calderon-Dominguez M, Serra D, Herrero L, Viana M. Mechanisms of impaired brown adipose tissue recruitment in obesity. Front Physiol (2019) 10:94. doi: 10.3389/fphys.2019.00094
77. Reyes-Farias M, Fos-Domenech J, Serra D, Herrero L, Sánchez-Infantes D. White adipose tissue dysfunction in obesity and aging. Biochem Pharmacol (2021) 192:114723. doi: 10.1016/j.bcp.2021.114723
78. Ma X, Xu L, Gavrilova O, Mueller E. Role of forkhead box protein A3 in age-associated metabolic decline. Proc Natl Acad Sci U S A (2014) 111:14289–94. doi: 10.1073/pnas.1407640111
79. Ambrosi TH, Scialdone A, Graja A, Gohlke S, Jank AM, Bocian C, et al. Adipocyte accumulation in the bone marrow during obesity and aging impairs stem cell-based hematopoietic and bone regeneration. Cell Stem Cell (2017) 20:771–784.e6. doi: 10.1016/j.stem.2017.02.009
80. Patel VS, Chan ME, Rubin J, Rubin CT. MARROW ADIPOSITY AND HEMATOPOIESIS IN AGING AND OBESITY: EXERCISE AS AN INTERVENTION. Curr Osteoporos Rep (2018) 16:105–15. doi: 10.1007/s11914-018-0424-1
81. Pérez LM, et al. ‘Adipaging’: ageing and obesity share biological hallmarks related to a dysfunctional adipose tissue. J Physiol (2016) 594:3187–207. doi: 10.1113/JP271691
82. Tam BT, Morais JA, Santosa S. Obesity and ageing: Two sides of the same coin. Obes Rev (2020) 21:e12991. doi: 10.1111/obr.12991
83. Kotzbeck P, Giordano A, Mondini E, Murano I, Severi I, Venema W, et al. Brown adipose tissue whitening leads to brown adipocyte death and adipose tissue inflammation. J Lipid Res (2018) 59:784–94. doi: 10.1194/jlr.M079665
84. Cannon B, Nedergaard J. Brown adipose tissue: function and physiological significance. Physiol Rev (2004) 84:277–359. doi: 10.1152/physrev.00015.2003
85. Barbatelli G, Murano I, Madsen L, Hao Q, Jimenez M, Kristiansen K, et al. The emergence of cold-induced brown adipocytes in mouse white fat depots is determined predominantly by white to brown adipocyte transdifferentiation. Am J Physiol Endocrinol Metab (2010) 298:E1244–53. doi: 10.1152/ajpendo.00600.2009
86. Sidossis L, Kajimura S. Brown and beige fat in humans: thermogenic adipocytes that control energy and glucose homeostasis. J Clin Invest (2015) 125:478–86. doi: 10.1172/JCI78362
87. Cinti S. Transdifferentiation properties of adipocytes in the adipose organ. Am J Physiol Endocrinol Metab (2009) 297:E977–986. doi: 10.1152/ajpendo.00183.2009
88. Wang QA, Tao C, Gupta RK, Scherer PE. Tracking adipogenesis during white adipose tissue development, expansion and regeneration. Nat Med (2013) 19:1338–44. doi: 10.1038/nm.3324
89. Lee Y-H, Petkova AP, Konkar AA, Granneman JG. Cellular origins of cold-induced brown adipocytes in adult mice. FASEB J Off Publ Fed Am Soc Exp Biol (2015) 29:286–99. doi: 10.1096/fj.14-263038
90. Lucchini FC, Wueest S, Challa TD, Item F, Modica S, Borsigova M, et al. ASK1 inhibits browning of white adipose tissue in obesity. Nat Commun (2020) 11:1642. doi: 10.1038/s41467-020-15483-7
91. Shimizu I, Walsh K. The whitening of brown fat and its implications for weight management in obesity. Curr Obes Rep (2015) 4:224–9. doi: 10.1007/s13679-015-0157-8
92. Bagchi M, Kim LA, Boucher J, Walshe TE, Kahn CR, D'Amore PA, et al. Vascular endothelial growth factor is important for brown adipose tissue development and maintenance. FASEB J Off Publ Fed Am Soc Exp Biol (2013) 27:3257–71. doi: 10.1096/fj.12-221812
93. Shimizu I, Aprahamian T, Kikuchi R, Shimizu A, Papanicolaou KN, MacLauchlan S, et al. Vascular rarefaction mediates whitening of brown fat in obesity. J Clin Invest (2014) 124:2099–112. doi: 10.1172/JCI71643
94. Rosenwald M, Perdikari A, Rülicke T, Wolfrum C. Bi-directional interconversion of brite and white adipocytes. Nat Cell Biol (2013) 15:659–67. doi: 10.1038/ncb2740
95. Altshuler-Keylin S, Shinoda K, Hasegawa Y, Ikeda K, Hong H, Kang Q, et al. Beige adipocyte maintenance is regulated by autophagy-induced mitochondrial clearance. Cell Metab (2016) 24:402–19. doi: 10.1016/j.cmet.2016.08.002
96. Porter C, Chondronikola M, Sidossis LS. The therapeutic potential of brown adipocytes in humans. Front Endocrinol (2015) 6:156. doi: 10.3389/fendo.2015.00156
97. Bartelt A, Heeren J. Adipose tissue browning and metabolic health. Nat Rev Endocrinol (2014) 10:24–36. doi: 10.1038/nrendo.2013.204
98. Doherty DG, O’Farrelly C. Innate and adaptive lymphoid cells in the human liver. Immunol Rev (2000) 174:5–20. doi: 10.1034/j.1600-0528.2002.017416.x
99. Lynch L, Golden-Mason L, Eogan M, O’Herlihy C, O’Farrelly C. Cells with haematopoietic stem cell phenotype in adult human endometrium: relevance to infertility? Hum Reprod Oxf Engl (2007) 22:919–26. doi: 10.1093/humrep/del456
100. Nielsen S, Pedersen BK. Skeletal muscle as an immunogenic organ. Curr Opin Pharmacol (2008) 8:346–51. doi: 10.1016/j.coph.2008.02.005
101. Soto-Tinoco E, Guerrero-Vargas NN, Buijs RM. Interaction between the hypothalamus and the immune system. Exp Physiol (2016) 101:1463–71. doi: 10.1113/EP085560
102. Camerini V, Panwala C, Kronenberg M. Regional specialization of the mucosal immune system. intraepithelial lymphocytes of the large intestine have a different phenotype and function than those of the small intestine. J Immunol Baltim Md 1950 (1993) 151:1765–76. doi: 10.4049/jimmunol.151.4.1765
103. Exley MA, Hand L, O’Shea D, Lynch L. Interplay between the immune system and adipose tissue in obesity. J Endocrinol (2014) 223:R41–48. doi: 10.1530/JOE-13-0516
104. Vadde R, Gupta MK, Nagaraju GP. Is adipose tissue an immunological organ? Crit Rev Immunol (2019) 39(6):481–90. doi: 10.1615/CritRevImmunol.2020033457
105. Hsu MC, Itkin M. Lymphatic anatomy. Tech Vasc Interv Radiol (2016) 19:247–54. doi: 10.1053/j.tvir.2016.10.003
106. Choi I, Lee S, Hong Y-K. The new era of the lymphatic system: no longer secondary to the blood vascular system. Cold Spring Harb Perspect Med (2012) 2:a006445. doi: 10.1101/cshperspect.a006445
107. Escobedo N, Oliver G. The lymphatic vasculature: Its role in adipose metabolism and obesity. Cell Metab (2017) 26:598–609. doi: 10.1016/j.cmet.2017.07.020
108. Magold AI, Swartz MA. Pathogenic exploitation of lymphatic vessels. Cells (2022) 11:979. doi: 10.3390/cells11060979
109. Card CM, Yu SS, Swartz MA. Emerging roles of lymphatic endothelium in regulating adaptive immunity. J Clin Invest (2014) 124:943–52. doi: 10.1172/JCI73316
110. Dieterich LC, Seidel CD, Detmar M. Lymphatic vessels: new targets for the treatment of inflammatory diseases. Angiogenesis (2014) 17:359–71. doi: 10.1007/s10456-013-9406-1
111. Kerjaschki D. The lymphatic vasculature revisited. J Clin Invest (2014) 124:874–7. doi: 10.1172/JCI74854
112. Randolph GJ, Angeli V, Swartz MA. Dendritic-cell trafficking to lymph nodes through lymphatic vessels. Nat Rev Immunol (2005) 5:617–28. doi: 10.1038/nri1670
113. von der Weid P-Y, Rainey KJ. Review article: lymphatic system and associated adipose tissue in the development of inflammatory bowel disease. Aliment Pharmacol Ther (2010) 32:697–711. doi: 10.1111/j.1365-2036.2010.04407.x
114. Liao S, von der Weid PY. Lymphatic system: An active pathway for immune protection. Semin Cell Dev Biol (2015) 38:83–9. doi: 10.1016/j.semcdb.2014.11.012
115. Kataru RP, Baik JE, Park HJ, Wiser I, Rehal S, Shin JY, et al. Regulation of immune function by the lymphatic system in lymphedema. Front Immunol (2019) 10. doi: 10.3389/fimmu.2019.00470
116. Redondo PAG, Gubert F, Zaverucha-do-Valle C, Pereira Pena Dutra T, De Paula Ayres-Silva J, Fernandes N, et al. Lymphatic vessels in human adipose tissue. Cell Tissue Res (2020) 379:511–20. doi: 10.1007/s00441-019-03108-5
117. Varaliová Z, Vlasák R, Čížková T, Gojda J, Potočková J, Šiklová M, et al. Lymphatic drainage affects lipolytic activity of femoral adipose tissue in women. Int J Obes (2020) 44:1974–8. doi: 10.1038/s41366-020-0559-x
118. Harvey NL. The link between lymphatic function and adipose biology. Ann N Y Acad Sci (2008) 1131:82–8. doi: 10.1196/annals.1413.007
119. Pond CM, Mattacks CA. Interactions between adipose tissue around lymph nodes and lymphoid cells in vitro. J Lipid Res (1995) 36:2219–31. doi: 10.1016/S0022-2275(20)39206-3
120. Pond CM, Mattacks CA. In vivo evidence for the involvement of the adipose tissue surrounding lymph nodes in immune responses. Immunol Lett (1998) 63:159–67. doi: 10.1016/S0165-2478(98)00074-1
121. Pond CM, Mattacks CA. The source of fatty acids incorporated into proliferating lymphoid cells in immune-stimulated lymph nodes. Br J Nutr (2003) 89:375–82. doi: 10.1079/BJN2002784
122. Mattacks CA, Sadler D, Pond CM. Site-specific differences in fatty acid composition of dendritic cells and associated adipose tissue in popliteal depot, mesentery, and omentum and their modulation by chronic inflammation and dietary lipids. Lymphat Res Biol (2004) 2:107–29. doi: 10.1089/lrb.2004.2.107
123. Mattacks CA, Sadler D, Pond CM. The cellular structure and lipid/protein composition of adipose tissue surrounding chronically stimulated lymph nodes in rats. J Anat (2003) 202:551–61. doi: 10.1046/j.1469-7580.2003.00188.x
124. Pond CM. Paracrine relationships between adipose and lymphoid tissues: implications for the mechanism of HIV-associated adipose redistribution syndrome. Trends Immunol (2003) 24:13–8. doi: 10.1016/S1471-4906(02)00004-2
125. Corvera S. Cellular heterogeneity in adipose tissues. Annu Rev Physiol (2021) 83:257–78. doi: 10.1146/annurev-physiol-031620-095446
126. Eto H, Suga H, Matsumoto D, Inoue K, Aoi N, Kato H, et al. Characterization of structure and cellular components of aspirated and excised adipose tissue. Plast Reconstr Surg (2009) 124:1087–97. doi: 10.1097/PRS.0b013e3181b5a3f1
127. Villarroya F, Cereijo R, Villarroya J, Gavaldà-Navarro A, Giralt M. Toward an understanding of how immune cells control brown and beige adipobiology. Cell Metab (2018) 27:954–61. doi: 10.1016/j.cmet.2018.04.006
128. Omran F, Christian M. Inflammatory signaling and brown fat activity. Front Endocrinol (2020) 11:156. doi: 10.3389/fendo.2020.00156
129. Trim WV, Lynch L. Immune and non-immune functions of adipose tissue leukocytes. Nat Rev Immunol (2022) 22:371–86. doi: 10.1038/s41577-021-00635-7
130. Caspar-Bauguil S, et al. Adipose tissues as an ancestral immune organ: site-specific change in obesity. FEBS Lett (2005) 579:3487–92. doi: 10.1016/j.febslet.2005.05.031
131. Duffaut C, Zakaroff-Girard A, Bourlier V, Decaunes P, Maumus M, Chiotasso P, et al. Interplay between human adipocytes and T lymphocytes in obesity. Arterioscler Thromb Vasc Biol (2009) 29:1608–14. doi: 10.1161/ATVBAHA.109.192583
132. McLaughlin T, Liu LF, Lamendola C, Shen L, Morton J, Rivas H, et al. T-Cell profile in adipose tissue is associated with insulin resistance and systemic inflammation in humans. Arterioscler Thromb Vasc Biol (2014) 34:2637–43. doi: 10.1161/ATVBAHA.114.304636
133. Macdougall CE, Longhi MP. Adipose tissue dendritic cells in steady-state. Immunology (2019) 156:228–34. doi: 10.1111/imm.13034
134. Macdougall CE, Wood EG, Loschko J, Scagliotti V, Cassidy FC, Robinson ME, et al. Visceral adipose tissue immune homeostasis is regulated by the crosstalk between adipocytes and dendritic cell subsets. Cell Metab (2018) 27:588–601.e4. doi: 10.1016/j.cmet.2018.02.007
135. Amano SU, Cohen JL, Vangala P, Tencerova M, Nicoloro SM, Yawe JC, et al. Local proliferation of macrophages contributes to obesity-associated adipose tissue inflammation. Cell Metab (2014) 19:162–71. doi: 10.1016/j.cmet.2013.11.017
136. Watanabe Y, Nagai Y, Honda H, Okamoto N, Yanagibashi T, Ogasawara M, et al. Bidirectional crosstalk between neutrophils and adipocytes promotes adipose tissue inflammation. FASEB J Off Publ Fed Am Soc Exp Biol (2019) 33:11821–35. doi: 10.1096/fj.201900477RR
137. Talukdar S, Oh DY, Bandyopadhyay G, Li D, Xu J, McNelis J, et al. Neutrophils mediate insulin resistance in mice fed a high-fat diet through secreted elastase. Nat Med (2012) 18:1407–12. doi: 10.1038/nm.2885
138. Liu J, Divoux A, Sun J, Zhang J, Clément K, Glickman JN, et al. Genetic deficiency and pharmacological stabilization of mast cells reduce diet-induced obesity and diabetes in mice. Nat Med (2009) 15:940–5. doi: 10.1038/nm.1994
139. Tanaka A, Nomura Y, Matsuda A, Ohmori K, Matsuda H. Mast cells function as an alternative modulator of adipogenesis through 15-deoxy-delta-12, 14-prostaglandin J2. Am J Physiol Cell Physiol (2011) 301:C1360–1367. doi: 10.1152/ajpcell.00514.2010
140. Wu D, Molofsky AB, Liang HE, Ricardo-Gonzalez RR, Jouihan HA, Bando JK, et al. Eosinophils sustain adipose alternatively activated macrophages associated with glucose homeostasis. Science (2011) 332:243–7. doi: 10.1126/science.1201475
141. Brigger D, Riether C, van Brummelen R, Mosher KI, Shiu A, Ding Z, et al. Eosinophils regulate adipose tissue inflammation and sustain physical and immunological fitness in old age. Nat Metab (2020) 2:688–702. doi: 10.1038/s42255-020-0228-3
142. Theurich S, Tsaousidou E, Hanssen R, Lempradl AM, Mauer J, Timper K, et al. IL-6/Stat3-Dependent induction of a distinct, obesity-associated NK cell subpopulation deteriorates energy and glucose homeostasis. Cell Metab (2017) 26:171–184.e6. doi: 10.1016/j.cmet.2017.05.018
143. Lynch L, Michelet X, Zhang S, Brennan PJ, Moseman A, Lester C, et al. Regulatory iNKT cells lack PLZF expression and control treg cell and macrophage homeostasis in adipose tissue. Nat Immunol (2015) 16:85–95. doi: 10.1038/ni.3047
144. Lynch L, Nowak M, Varghese B, Clark J, Hogan AE, Toxavidis V, et al. Adipose tissue invariant NKT cells protect against diet-induced obesity and metabolic disorder through regulatory cytokine production. Immunity (2012) 37:574–87. doi: 10.1016/j.immuni.2012.06.016
145. Boulenouar S, Michelet X, Duquette D, Alvarez D, Hogan AE, Dold C, et al. Adipose type one innate lymphoid cells regulate macrophage homeostasis through targeted cytotoxicity. Immunity (2017) 46:273–86. doi: 10.1016/j.immuni.2017.01.008
146. Wang H, Shen L, Sun X, Liu F, Feng W, Jiang C, et al. Adipose group 1 innate lymphoid cells promote adipose tissue fibrosis and diabetes in obesity. Nat Commun (2019) 10:3254. doi: 10.1038/s41467-019-11270-1
147. Molofsky AB, Nussbaum JC, Liang HE, Dyken SJV, Cheng LE, Mohapatra A, et al. Innate lymphoid type 2 cells sustain visceral adipose tissue eosinophils and alternatively activated macrophages. J Exp Med (2013) 210(3):535–49. doi: 10.1084/jem.20121964
148. Kohlgruber AC, Gal-Oz ST, LaMarche NM, Shimazaki M, Duquette D, Koay HF, et al. γδ T cells producing interleukin-17A regulate adipose regulatory T cell homeostasis and thermogenesis. Nat Immunol (2018) 19:464–74. doi: 10.1038/s41590-018-0094-2
149. Toubal A, Lehuen A. Role of MAIT cells in metabolic diseases. Mol Immunol (2021) 130:142–7. doi: 10.1016/j.molimm.2020.12.014
150. Magalhaes I, Pingris K, Poitou C, Bessoles S, Venteclef N, Kiaf B, et al. Mucosal-associated invariant T cell alterations in obese and type 2 diabetic patients. J Clin Invest (2015) 125:1752–62. doi: 10.1172/JCI78941
151. Camell CD, Günther P, Lee A, Goldberg EL, Spadaro O, Youm YH, et al. Aging induces an Nlrp3 inflammasome-dependent expansion of adipose b cells that impairs metabolic homeostasis. Cell Metab (2019) 30:1024–1039.e6. doi: 10.1016/j.cmet.2019.10.006
152. Frasca D, Ferracci F, Diaz A, Romero M, Lechner S, Blomberg BB. Obesity decreases b cell responses in young and elderly individuals. Obes Silver Spring Md (2016) 24:615–25. doi: 10.1002/oby.21383
153. Kintscher U, Hartge M, Hess K, Foryst-Ludwig A, Clemenz M, Wabitsch M, et al. T-Lymphocyte infiltration in visceral adipose tissue: a primary event in adipose tissue inflammation and the development of obesity-mediated insulin resistance. Arterioscler Thromb Vasc Biol (2008) 28:1304–10. doi: 10.1161/ATVBAHA.108.165100
154. Bertola A, Ciucci T, Rousseau D, Bourlier V, Duffaut C, Bonnafous S, et al. Identification of adipose tissue dendritic cells correlated with obesity-associated insulin-resistance and inducing Th17 responses in mice and patients. Diabetes (2012) 61:2238–47. doi: 10.2337/db11-1274
155. Feuerer M, Herrero L, Cipolletta D, Naaz A, Wong J, Nayer A, et al. Lean, but not obese, fat is enriched for a unique population of regulatory T cells that affect metabolic parameters. Nat Med (2009) 15:930–9. doi: 10.1038/nm.2002
156. Nishimura S, Manabe I, Nagasaki M, Eto K, Yamashita H, Ohsugi M, et al. CD8+ effector T cells contribute to macrophage recruitment and adipose tissue inflammation in obesity. Nat Med (2009) 15:914–20. doi: 10.1038/nm.1964
157. Moysidou M, Karaliota S, Kodela E, Salagianni M, Koutmani Y, Katsouda A, et al. CD8+ T cells in beige adipogenesis and energy homeostasis. JCI Insight (2018) 3:95456. doi: 10.1172/jci.insight.95456
158. Platell C, Cooper D, Papadimitriou JM, Hall JC. The omentum. World J Gastroenterol (2000) 6:169–76. doi: 10.3748/wjg.v6.i2.169
159. Moro K, Yamada T, Tanabe M, Takeuchi T, Ikawa T, Kawamoto H, et al. Innate production of T h 2 cytokines by adipose tissue-associated c-kit + sca-1 + lymphoid cells. Nature (2010) 463:540–4. doi: 10.1038/nature08636
160. Elewa YHA, Ichii O, Otsuka S, Hashimoto Y, Kon Y. Characterization of mouse mediastinal fat-associated lymphoid clusters. Cell Tissue Res (2014) 357:731–41. doi: 10.1007/s00441-014-1889-6
161. Jackson-Jones LH, Duncan SM, Magalhaes MS, Campbell SM, Maizels RM, McSorley HJ, et al. Fat-associated lymphoid clusters control local IgM secretion during pleural infection and lung inflammation. Nat Commun (2016) 7:12651. doi: 10.1038/ncomms12651
162. Rangel-Moreno J, Moyron-Quiroz JE, Carragher DM, Kusser K, Hartson L, Moquin A, et al. Omental milky spots develop in the absence of lymphoid tissue-inducer cells and support b and T cell responses to peritoneal antigens. Immunity (2009) 30:731–43. doi: 10.1016/j.immuni.2009.03.014
163. Cruz-Migoni S, Caamaño J. Fat-associated lymphoid clusters in inflammation and immunity. Front Immunol (2016) 7:612. doi: 10.3389/fimmu.2016.00612
164. Frasca D, Blomberg BB. Adipose tissue: A tertiary lymphoid organ: Does it change with age? Gerontology (2020) 66:114–21. doi: 10.1159/000502036
165. Lago F, Dieguez C, Gómez-Reino J, Gualillo O. Adipokines as emerging mediators of immune response and inflammation. Nat Clin Pract Rheumatol (2007) 3:716–24. doi: 10.1038/ncprheum0674
166. Lafontan M. Historical perspectives in fat cell biology: the fat cell as a model for the investigation of hormonal and metabolic pathways. Am J Physiol Cell Physiol (2012) 302:C327–59. doi: 10.1152/ajpcell.00168.2011
167. Recinella L, Orlando G, Ferrante C, Chiavaroli A, Brunetti L, Leone S. Adipokines: New potential therapeutic target for obesity and metabolic, rheumatic, and cardiovascular diseases. Front Physiol (2020) 11. doi: 10.3389/fphys.2020.578966
168. Ouchi N, Walsh K. Adiponectin as an anti-inflammatory factor. Clin Chim Acta Int J Clin Chem (2007) 380:24–30. doi: 10.1016/j.cca.2007.01.026
169. Yokota T, Oritani K, Takahashi I, Ishikawa J, Matsuyama A, Ouchi N, et al. Adiponectin, a new member of the family of soluble defense collagens, negatively regulates the growth of myelomonocytic progenitors and the functions of macrophages. Blood (2000) 96:1723–32. doi: 10.1182/blood.V96.5.1723
170. Lord GM. Leptin as a proinflammatory cytokine. Contrib Nephrol (2006) 151:151–64. doi: 10.1159/000095326
171. Lord GM, Matarese G, Howard JK, Baker RJ, Bloom SR, Lechler RI, et al. Leptin modulates the T-cell immune response and reverses starvation-induced immunosuppression. Nature (1998) 394(6696):897–901. doi: 10.1038/29795
172. Cava AL, Matarese G. The weight of leptin in immunity. Nat Rev Immunol (2004) 4:371–9. doi: 10.1038/nri1350
173. Kopp A, Buechler C, Neumeier M, Weigert J, Aslanidis C, Schölmerich J, et al. Innate immunity and adipocyte function: ligand-specific activation of multiple toll-like receptors modulates cytokine, adipokine, and chemokine secretion in adipocytes. Obes Silver Spring Md (2009) 17:648–56. doi: 10.1038/oby.2008.607
174. Frasca D, Diaz A, Romero M, Thaller S, Blomberg BB. Secretion of autoimmune antibodies in the human subcutaneous adipose tissue. PloS One (2018) 13:e0197472. doi: 10.1371/journal.pone.0197472
175. Poggi M, Jager J, Paulmyer-Lacroix O, Peiretti F, Gremeaux T, Verdier M, et al. The inflammatory receptor CD40 is expressed on human adipocytes: contribution to crosstalk between lymphocytes and adipocytes. Diabetologia (2009) 52:1152–63. doi: 10.1007/s00125-009-1267-1
176. Huh JY, Kim JI, Park YJ, Hwang IJ, Lee YS, Sohn JH, et al. A novel function of adipocytes in lipid antigen presentation to iNKT cells. Mol Cell Biol (2013) 33:328–39. doi: 10.1128/MCB.00552-12
177. Xiao L, Yang X, Lin Y, Li S, Jiang J, Qian S, et al. Large Adipocytes function as antigen-presenting cells to activate CD4(+) T cells via upregulating MHCII in obesity. Int J Obes 2005 (2016) 40:112–20. doi: 10.1038/ijo.2015.145
178. Caputa G, Matsushita M, Sanin DE, Kabat AM, Edwards-Hicks J, Grzes KM, et al. Intracellular infection and immune system cues rewire adipocytes to acquire immune function. Cell Metab (2022) 34:747–760.e6. doi: 10.1016/j.cmet.2022.04.008
179. Honce R, Schultz-Cherry S. Impact of obesity on influenza a virus pathogenesis, immune response, and evolution. Front Immunol (2019) 10. doi: 10.3389/fimmu.2019.01071
180. Ershler WB. Influenza and Aging. In: Goldstein AL (eds) Biomedical Advances in Aging. GWUMC Department of Biochemistry Annual Spring Symposia. Springer, Boston, MA. (1990). doi: 10.1007/978-1-4613-0513-2_51
181. Cheng WA, Turner L, Marentes Ruiz CJ, Tanaka ML, Congrave-Wilson Z, Lee Y, et al. Clinical manifestations of COVID-19 differ by age and obesity status. Influenza Other Respir Viruses (2022) 16:255–64. doi: 10.1111/irv.12918
182. Thomas AL, Alarcon PC, Divanovic S, Chougnet CA, Hildeman DA, Moreno-Fernandez ME. Implications of inflammatory states on dysfunctional immune responses in aging and obesity. Front Aging (2021) 2. doi: 10.3389/fragi.2021.732414
183. De Carvalho FG, Justice JN, de Freitas EC, Kershaw EE, Sparks LM. Adipose tissue quality in aging: How structural and functional aspects of adipose tissue impact skeletal muscle quality. Nutrients (2019) 11:2553. doi: 10.3390/nu11112553
184. Agarwal P, Khan SR, Verma SC, Beg M, Singh K, Mitra K, et al. Mycobacterium tuberculosis persistence in various adipose depots of infected mice and the effect of anti-tubercular therapy. Microbes Infect (2014) 16:571–80. doi: 10.1016/j.micinf.2014.04.006
185. Neyrolles O, Hernández-Pando R, Pietri-Rouxel F, Fornès P, Tailleux L, Barrios Payán JA, et al. Is adipose tissue a place for mycobacterium tuberculosis persistence? PloS One (2006) 1:e43. doi: 10.1371/journal.pone.0000043
186. Beigier-Bompadre M, Montagna GN, Kühl AA, Lozza L, Weiner J, Kupz A, et al. Mycobacterium tuberculosis infection modulates adipose tissue biology. PloS Pathog (2017) 13. doi: 10.1371/journal.ppat.1006676
187. Bechah Y, et al. Persistence of coxiella burnetii, the agent of q fever, in murine adipose tissue. PloS One (2014) 9:e97503. doi: 10.1371/journal.pone.0097503
188. Bechah Y, Paddock CD, Capo C, Mege J-L, Raoult D. Adipose tissue serves as a reservoir for recrudescent rickettsia prowazekii infection in a mouse model. PloS One (2010) 5:e8547. doi: 10.1371/journal.pone.0008547
189. Sashinami H, Nakane A. Adiponectin is required for enhancement of CCL2 expression in adipose tissue during listeria monocytogenes infection. Cytokine (2010) 50:170–4. doi: 10.1016/j.cyto.2009.12.005
190. Shi Y, Liu Y, Murdin A, Raudonikiene-Mancevski A, Ayach BB, Yu Z, et al. Chlamydophila pneumoniae inhibits differentiation of progenitor adipose cells and impairs insulin signaling. J Infect Dis (2008) 197:439–48. doi: 10.1086/525045
191. Walenna NF, Kurihara Y, Chou B, Ishii K, Soejima T, Itoh R, et al. Chlamydia pneumoniae exploits adipocyte lipid chaperone FABP4 to facilitate fat mobilization and intracellular growth in murine adipocytes. Biochem Biophys Res Commun (2018) 495:353–9. doi: 10.1016/j.bbrc.2017.11.005
192. Hanses F, Kopp A, Bala M, Buechler C, Falk W, Salzberger B, et al. Intracellular survival of staphylococcus aureus in adipocyte-like differentiated 3T3-L1 cells is glucose dependent and alters cytokine, chemokine, and adipokine secretion. Endocrinology (2011) 152:4148–57. doi: 10.1210/en.2011-0103
193. Han S-J, Glatman Zaretsky A, Andrade-Oliveira V, Collins N, Dzutsev A, Shaik J, et al. White adipose tissue is a reservoir for memory T cells and promotes protective memory responses to infection. Immunity (2017) 47:1154–1168.e6. doi: 10.1016/j.immuni.2017.11.009
194. Shoemaker JP, Hoffman RV, Huffman DG. Trypanosoma cruzi: Preference for brown adipose tissue in mice by the tulahuen strain. Exp Parasitol (1970) 27:403–7. doi: 10.1016/0014-4894(70)90045-7
195. Lenzi HL, Oliveira DN, Lima MT, Gattass CR. Trypanosoma cruzi: paninfectivity of CL strain during murine acute infection. Exp Parasitol (1996) 84:16–27. doi: 10.1006/expr.1996.0086
196. Buckner FS, Wilson AJ, Van Voorhis WC. Detection of live trypanosoma cruzi in tissues of infected mice by using histochemical stain for β-galactosidase. Infect Immun (1999) 67:403–9. doi: 10.1128/IAI.67.1.403-409.1999
197. Trindade S, Rijo-Ferreira F, Carvalho T, Pinto-Neves D, Guegan F, Aresta-Branco F, et al. Trypanosoma brucei parasites occupy and functionally adapt to the adipose tissue in mice. Cell Host Microbe (2016) 19:837–48. doi: 10.1016/j.chom.2016.05.002
198. Caljon G, Reet NV, Trez CD, Vermeersch M, Pérez-Morga D, Abbeele JVD. The dermis as a delivery site of trypanosoma brucei for tsetse flies. PloS Pathog (2016) 12:e1005744. doi: 10.1371/journal.ppat.1005744
199. Capewell P, Cren-Travaillé C, Marchesi F, Johnston P, Clucas C, Benson RA, et al. The skin is a significant but overlooked anatomical reservoir for vector-borne African trypanosomes. eLife 5:e17716. doi: 10.7554/eLife.17716
200. Wilairatana P, Riganti M, Puchadapirom P, Punpoowong B, Vannaphan S, Udomsangpetch R, et al. Prognostic significance of skin and subcutaneous fat sequestration of parasites in severe falciparum malaria. Southeast Asian J Trop Med Public Health (2000) 31:203–12.
201. Franke-Fayard B, Fonager J, Braks A, Khan SM, Janse CJ. Sequestration and tissue accumulation of human malaria parasites: Can we learn anything from rodent models of malaria? PloS Pathog (2010) 6(9):e1001032. doi: 10.1371/journal.ppat.1001032
202. Franke-Fayard B, Janse CJ, Cunha-Rodrigues M, Ramesar J, Büscher P, Que I, et al. Murine malaria parasite sequestration: CD36 is the major receptor, but cerebral pathology is unlinked to sequestration. Proc Natl Acad Sci U S A (2005) 102:11468–73. doi: 10.1073/pnas.0503386102
203. Michel G, Ferrua B, Lang T, Maddugoda MP, Munro P, Pomares C, et al. Luciferase-expressing leishmania infantum allows the monitoring of amastigote population size, In vivo, ex vivo and In vitro. PloS Negl Trop Dis (2011) 5:e1323. doi: 10.1371/journal.pntd.0001323
204. Schwing A, Pisani DF, Pomares C, Majoor A, Lacas-Gervais S, Jager J, et al. Identification of adipocytes as target cells for leishmania infantum parasites. Sci Rep (2021) 11:21275. doi: 10.1038/s41598-021-00443-y
205. Dhurandhar NV, Whigham LD, Abbott DH, Schultz-Darken NJ, Israel BA, Bradley SM, et al. Human adenovirus ad-36 promotes weight gain in Male rhesus and marmoset monkeys. J Nutr (2002) 132:3155–60. doi: 10.1093/jn/131.10.3155
206. Ponterio E, Cangemi R, Mariani S, Casella G, De Cesare A, Trovato FM, et al. Adenovirus 36 DNA in human adipose tissue. Int J Obes (2015) 39:1761–4. doi: 10.1038/ijo.2015.163
207. Salehian B, Forman SJ, Kandeel FR, Bruner DE, He J, Atkinson RL, et al. Adenovirus 36 DNA in adipose tissue of patient with unusual visceral obesity. Emerg Infect Dis (2010) 16:850–2. doi: 10.3201/eid1605.091271
208. Barrera-Alcocer J, García-Benavides L, Muñoz-Valle JF, de la Cruz-Mosso U, González RA, Luquín S, et al. Presence of adenovirus-36 DNA in adipose tissue of women: Relationship with adipocyte morphology and the expression of C/EBPβ and HIF-1α. Diabetes Metab Syndr Obes Targets Ther (2021) 14:477–86. doi: 10.2147/DMSO.S285341
209. Couturier J, Suliburk JW, Brown JM, Luke DJ, Agarwal N, Yu X, et al. Human adipose tissue as a reservoir for memory CD4 T cells and HIV. AIDS Lond Engl (2015) 29:667–74. doi: 10.1097/QAD.0000000000000599
210. Couturier J, Agarwal N, Nehete PN, Baze WB, Barry MA, Sastry KJ, et al. Infectious SIV resides in adipose tissue and induces metabolic defects in chronically infected rhesus macaques. Retrovirology (2016) 13:30. doi: 10.1186/s12977-016-0260-2
211. Couturier J, Lewis DE. HIV Persistence in adipose tissue reservoirs. Curr HIV/AIDS Rep (2018) 15:60–71. doi: 10.1007/s11904-018-0378-z
212. Damouche A, Lazure T, Avettand-Fènoël V, Huot N, Dejucq-Rainsford N, Satie AP, et al. Adipose tissue is a neglected viral reservoir and an inflammatory site during chronic HIV and SIV infection. PloS Pathog (2015) 11(9):e1005153. doi: 10.1371/journal.ppat.1005153
213. Hsu DC, Wegner MD, Sunyakumthorn P, Silsorn D, Tayamun S, Inthawong D, et al. CD4+ cell infiltration into subcutaneous adipose tissue is not indicative of productively infected cells during acute SHIV infection. J Med Primatol (2017) 46:154–7. doi: 10.1111/jmp.12298
214. Misumi I, Starmer J, Uchimura T, Beck MA, Magnuson T, Whitmire JK. Obesity expands a distinct population of T cells in adipose tissue and increases vulnerability to infection. Cell Rep (2019) 27:514–524.e5. doi: 10.1016/j.celrep.2019.03.030
215. Baazim H, Schweiger M, Moschinger M, Xu H, Scherer T, Popa A, et al. CD8+ T cells induce cachexia during chronic viral infection. Nat Immunol (2019) 20:701–10. doi: 10.1038/s41590-019-0397-y
216. Contreras NA, Sitnik KM, Jeftic I, Coplen CP, Čičin-Šain L, Nikolich-Žugich J. Life-long control of cytomegalovirus (CMV) by T resident memory cells in the adipose tissue results in inflammation and hyperglycemia. PloS Pathog (2019) 15:e1007890. doi: 10.1371/journal.ppat.1007890
217. Bruggeman CA, Bruning JH, Grauls G, van den Bogaard AE, Bosman F. Presence of cytomegalovirus in brown fat. study in a rat model. Intervirology (1987) 27:32–7. doi: 10.1159/000149712
218. Price P, Eddy KS, Papadimitriou JM, Robertson TA, Shellam GR. Cytomegalovirus infection of adipose tissues induces steatitis in adult mice. Int J Exp Pathol (1990) 71:557–71.
219. Nishimura H, Itamura S, Iwasaki T, Kurata T, Tashiro M. Characterization of human influenza a (H5N1) virus infection in mice: neuro-, pneumo- and adipotropic infection. J Gen Virol (2000) 81:2503–10. doi: 10.1099/0022-1317-81-10-2503
220. Ayari A, Rosa-Calatrava M, Lancel S, Barthelemy J, Pizzorno A, Mayeuf-Louchart A, et al. Influenza infection rewires energy metabolism and induces browning features in adipose cells and tissues. Commun Biol (2020) 3:1–15. doi: 10.1038/s42003-020-0965-6
221. Zeng M-S, Yu W-D, Wang H-X, Xu P-P, Liu J-Y. Puerarin reduces impairment of intestinal and adipose immune responses to influenza virus infection in mice. Arch Virol (2021) 166:2387–97. doi: 10.1007/s00705-021-05112-z
222. Oseghale O, Liong S, Coward-Smith M, To EE, Erlich JR, Luong R, et al. Influenza a virus elicits peri-vascular adipose tissue inflammation and vascular dysfunction of the aorta in pregnant mice. PloS Pathog (2022) 18:e1010703. doi: 10.1371/journal.ppat.1010703
223. Reiterer M, Rajan M, Gómez-Banoy N, Lau JD, Gomez-Escobar LG, Ma L, et al. Hyperglycemia in acute COVID-19 is characterized by insulin resistance and adipose tissue infectivity by SARS-CoV-2. Cell Metab (2021) 33:2174–2188.e5. doi: 10.1016/j.cmet.2021.09.009
224. Hirschbühl K, Dintner S, Beer M, Wylezich C, Schlegel J, Delbridge C, et al. Viral mapping in COVID-19 deceased in the augsburg autopsy series of the first wave: A multiorgan and multimethodological approach. PloS One (2021) 16:e0254872. doi: 10.1371/journal.pone.0254872
225. Zickler M, Stanelle-Bertram S, Ehret S, Heinrich F, Lange P, Schaumburg B, et al. Replication of SARS-CoV-2 in adipose tissue determines organ and systemic lipid metabolism in hamsters and humans. Cell Metab (2022) 34:1–2. doi: 10.1016/j.cmet.2021.12.002
226. Martínez-Colón GJ, Ratnasiri K, Chen H, Jiang S, Zanley E, Rustagi A, et al. SARS-CoV-2 infection drives an inflammatory response in human adipose tissue through infection of adipocytes and macrophages. Sci Transl Med (2022):eabm9151. doi: 10.1126/scitranslmed.abm9151
227. Tanowitz HB, Scherer PE, Mota MM, Figueiredo LM. Adipose tissue - a safe haven for parasites? Trends Parasitol (2017) 33:276–84. doi: 10.1016/j.pt.2016.11.008
228. Parrish NM, Dick JD, Bishai WR. Mechanisms of latency in mycobacterium tuberculosis. Trends Microbiol (1998) 6:107–12. doi: 10.1016/S0966-842X(98)01216-5
229. Martinez N, Cheng CY, Ketheesan N, Cullen A, Tang Y, Lum J, et al. mTORC2/Akt activation in adipocytes is required for adipose tissue inflammation in tuberculosis. EBioMedicine (2019) 45:314–27. doi: 10.1016/j.ebiom.2019.06.052
230. Ayyappan JP, Ganapathi U, Lizardo K, Vinnard C, Subbian S, Perlin DS, et al. Adipose tissue regulates pulmonary pathology during TB infection. mBio (2019) 10:e02771–18. doi: 10.1128/mBio.02771-18
231. Bouzid F, Brégeon F, Poncin I, Weber P, Drancourt M, Canaan S. Mycobacterium canettii infection of adipose tissues. Front Cell Infect Microbiol (2017) 7. doi: 10.3389/fcimb.2017.00189
232. Raoult D, Marrie T, Mege J. Natural history and pathophysiology of q fever. Lancet Infect Dis (2005) 5:219–26. doi: 10.1016/S1473-3099(05)70052-9
233. Raoult D, Roux V. The body louse as a vector of reemerging human diseases. Clin Infect Dis Off Publ Infect Dis Soc Am (1999) 29:888–911. doi: 10.1086/520454
234. Radoshevich L, Cossart P. Listeria monocytogenes: towards a complete picture of its physiology and pathogenesis. Nat Rev Microbiol (2018) 16:32–46. doi: 10.1038/nrmicro.2017.126
235. Walenna NF, Kurihara Y, Chou B, Ishii K, Soejima T, Hiromatsu K. Chlamydia pneumoniae infection–induced endoplasmic reticulum stress causes fatty acid–binding protein 4 secretion in murine adipocytes. J Biol Chem (2020) 295:2713–23. doi: 10.1074/jbc.RA119.010683
236. Musser JM, Schlievert PM, Chow AW, Ewan P, Kreiswirth BN, Rosdahl VT, et al. A single clone of staphylococcus aureus causes the majority of cases of toxic shock syndrome. Proc Natl Acad Sci U S A (1990) 87:225–9. doi: 10.1073/pnas.87.1.225
237. David MZ, Daum RS. Community-associated methicillin-resistant staphylococcus aureus: epidemiology and clinical consequences of an emerging epidemic. Clin Microbiol Rev (2010) 23:616–87. doi: 10.1128/CMR.00081-09
238. Yamasaki K, Di Nardo A, Bardan A, Murakami M, Ohtake T, Coda A, et al. Increased serine protease activity and cathelicidin promotes skin inflammation in rosacea. Nat Med (2007) 13:975–80. doi: 10.1038/nm1616
239. Wang B, P. CC, Pippin JJ. Leptin- and leptin receptor-deficient rodent models: Relevance for human type 2 diabetes. Curr Diabetes Rev (2014) 10:131–45. doi: 10.2174/1573399810666140508121012
240. Park S, Rich J, Hanses F, Lee JC. Defects in innate immunity predispose C57BL/6J-Leprdb/Leprdb mice to infection by staphylococcus aureus. Infect Immun (2009) 77:1008–14. doi: 10.1128/IAI.00976-08
241. Befus M, Lowy FD, Miko BA, Mukherjee DV, Herzig CTA, Larson EL. Obesity as a determinant of staphylococcus aureus colonization among inmates in maximum-security prisons in new York state. Am J Epidemiol (2015) 182:494–502. doi: 10.1093/aje/kwv062
242. Kang CI, Song JH, Ko KS, Chung DR, Peck KR, Asian Network for Surveillance of Resistant Pathogens (ANSORP) Study Group. Clinical features and outcome of staphylococcus aureus infection in elderly versus younger adult patients. Int J Infect Dis IJID Off Publ Int Soc Infect Dis (2011) 15:e58–62. doi: 10.1016/j.ijid.2010.09.012
243. Hochberg A, Patz M, Karrasch T, Schäffler A, Schmid A. Serum levels and adipose tissue gene expression of cathelicidin antimicrobial peptide (CAMP) in obesity and during weight loss. Horm Metab Res Horm Stoffwechselforschung Horm Metab (2021) 53:169–77. doi: 10.1055/a-1323-3050
244. Gallo RL, Kim KJ, Bernfield M, Kozak CA, Zanetti M, Merluzzi L, et al. Identification of CRAMP, a cathelin-related antimicrobial peptide expressed in the embryonic and adult mouse. J Biol Chem (1997) 272:13088–93. doi: 10.1074/jbc.272.20.13088
245. Schmid A, Karrasch T, Thomalla M, Schlegel J, Salzberger B, Schäffler A, et al. Innate immunity of adipose tissue in rodent models of local and systemic staphylococcus aureus infection. Mediators Inflamm (2017) 2017:5315602. doi: 10.1155/2017/5315602
246. Andrade ZA, Silva HRR, Andrade ZA, Silva HRR. Parasitism of adipocytes by trypanosoma cruzi. Mem Inst Oswaldo Cruz (1995) 90:521–2. doi: 10.1590/S0074-02761995000400018
247. Combs TP, Nagajyothi F, Nagajyothi Mukherjee S, de Almeida CJG, Jelicks LA, William Schubert W, et al. The adipocyte as an important target cell for trypanosoma cruzi infection. J Biol Chem (2005) 280:24085–94. doi: 10.1074/jbc.M412802200
248. Matos Ferreira AV, Segatto M, Menezes Z, Macedo AM, Gelape C, de Oliveira Andrade L, et al. Evidence for trypanosoma cruzi in adipose tissue in human chronic chagas disease. Microbes Infect (2011) 13:1002–5. doi: 10.1016/j.micinf.2011.06.002
249. Nagajyothi F, Desruisseaux MS, Machado FS, Upadhya R, Zhao D, Schwartz GJ, et al. Response of adipose tissue to early infection with trypanosoma cruzi (Brazil strain). J Infect Dis (2012) 205:830–40. doi: 10.1093/infdis/jir840
250. Wen JJ, Nagajyothi F, Machado FS, Weiss LM, Scherer PE, Tanowitz HB, et al. Markers of oxidative stress in adipose tissue during trypanosoma cruzi infection. Parasitol Res (2014) 113:3159–65. doi: 10.1007/s00436-014-3977-7
251. Snow RW. Global malaria eradication and the importance of plasmodium falciparum epidemiology in Africa. BMC Med (2015) 13:23. doi: 10.1186/s12916-014-0254-7
252. Matsuoka H, Yoshida S, Hirai M, Ishii A. A rodent malaria, plasmodium berghei, is experimentally transmitted to mice by merely probing of infective mosquito, anopheles stephensi. Parasitol Int (2002) 51(1):17–23. doi: 10.1016/S1383-5769(01)00095-2
253. Abumrad NA, el-Maghrabi MR, Amri EZ, Lopez E, Grimaldi PA. Cloning of a rat adipocyte membrane protein implicated in binding or transport of long-chain fatty acids that is induced during preadipocyte differentiation. homology with human CD36. J Biol Chem (1993) 268:17665–8. doi: 10.1016/S0021-9258(17)46753-6
254. Mejia P, Treviño-Villarreal JH, De Niz M, Meibalan E, Longchamp A, Reynolds JS, et al. Adipose tissue parasite sequestration drives leptin production in mice and correlates with human cerebral malaria. Sci Adv (2021) 7:eabe2484. doi: 10.1126/sciadv.abe2484
255. Mejia P, Treviño-Villarreal JH, Hine C, Harputlugil E, Lang S, Calay E, et al. Dietary restriction protects against experimental cerebral malaria via leptin modulation and T-cell mTORC1 suppression. Nat Commun (2015) 6:6050. doi: 10.1038/ncomms7050
256. Pulido-Mendez M, De Sanctis J, Rodríguez-Acosta A. Leptin and leptin receptors during malaria infection in mice. Folia Parasitol (Praha) (2002) 49:249–51. doi: 10.14411/fp.2002.046
257. Bogdan C. Mechanisms and consequences of persistence of intracellular pathogens: leishmaniasis as an example. Cell Microbiol (2008) 10:1221–34. doi: 10.1111/j.1462-5822.2008.01146.x
258. Greber UF, Flatt JW. Adenovirus entry: From infection to immunity. Annu Rev Virol (2019) 6:177–97. doi: 10.1146/annurev-virology-092818-015550
259. Dhurandhar NV, Kulkarni PR, Ajinkya SM, Sherikar AA, Atkinson RL. Association of adenovirus infection with human obesity. Obes Res (1997) 5:464–9. doi: 10.1002/j.1550-8528.1997.tb00672.x
260. Dhurandhar NV, Israel BA, Kolesar JM, Mayhew GF, Cook ME, Atkinson RL, et al. Increased adiposity in animals due to a human virus. Int J Obes Relat Metab Disord J Int Assoc Study Obes (2000) 24:989–96. doi: 10.1038/sj.ijo.0801319
261. Atkinson RL, Dhurandhar NV, Allison DB, Bowen RL, Israel BA, Albu JB, et al. Human adenovirus-36 is associated with increased body weight and paradoxical reduction of serum lipids. Int J Obes 2005 (2005) 29:281–6. doi: 10.1038/sj.ijo.0802830
262. Whigham LD, Israel BA, Atkinson RL. Adipogenic potential of multiple human adenoviruses in vivo and in vitro in animals. Am J Physiol Regul Integr Comp Physiol (2006) 290:R190–4. doi: 10.1152/ajpregu.00479.2005
263. Vangipuram SD, Yu M, Tian J, Stanhope KL, Pasarica M, Havel PJ, et al. Adipogenic human adenovirus-36 reduces leptin expression and secretion and increases glucose uptake by fat cells. Int J Obes 2005 (2007) 31:87–96. doi: 10.1038/sj.ijo.0803366
264. Pasarica M, Shin AC, Yu M, Yang HMO, Rathod M, Jen KLC, et al. Human adenovirus 36 induces adiposity, increases insulin sensitivity, and alters hypothalamic monoamines in rats. Obes Silver Spring Md (2006) 14:1905–13. doi: 10.1038/oby.2006.222
265. Jiao Y, Mao X, Chang X, Abudureyimu K, Zhang C, Lu J, et al. Adenovirus36 infection expresses cellular APMI and visfatin genes in overweight uygur individuals. Diagn Pathol (2014) 9:83. doi: 10.1186/1746-1596-9-83
266. Dupin N, Buffet M, Marcelin AG, Lamotte C, Gorin I, Ait-Arkoub Z, et al. HIV And antiretroviral drug distribution in plasma and fat tissue of HIV-infected patients with lipodystrophy. AIDS Lond Engl (2002) 16:2419–24. doi: 10.1097/00002030-200212060-00006
267. Hazan U, Romero IA, Cancello R, Valente S, Perrin V, Mariot V, et al. Human adipose cells express CD4, CXCR4, and CCR5 [corrected] receptors: a new target cell type for the immunodeficiency virus-1? FASEB J Off Publ Fed Am Soc Exp Biol (2002) 16:1254–6. doi: 10.1096/fj.01-0947fje
268. Munier S, Borjabad A, Lemaire M, Mariot V, Hazan U. In vitro infection of human primary adipose cells with HIV-1: a reassessment. AIDS Lond Engl (2003) 17:2537–9. doi: 10.1097/00002030-200311210-00019
269. Gupta M, Shorman M. Cytomegalovirus. [Updated 2022 Aug 8]. In: StatPearls [Internet]. Treasure Island (FL): StatPearls Publishing (2022).
270. Du Y, Zhang G, Liu Z. Human cytomegalovirus infection and coronary heart disease: a systematic review. Virol J (2018) 15:31. doi: 10.1186/s12985-018-0937-3
271. Samanta M, Harkins L, Klemm K, Britt WJ, Cobbs CS. High prevalence of human cytomegalovirus in prostatic intraepithelial neoplasia and prostatic carcinoma. J Urol (2003) 170:998–1002. doi: 10.1097/01.ju.0000080263.46164.97
272. Bouwman JJM, Visseren FLJ, Bouter KP, Diepersloot RJA. Infection-induced inflammatory response of adipocytes in vitro. Int J Obes (2008) 32:892–901. doi: 10.1038/ijo.2008.36
273. Bouwman JJM, Diepersloot RJA, Visseren FLJ. Intracellular infections enhance interleukin-6 and plasminogen activator inhibitor 1 production by cocultivated human adipocytes and THP-1 monocytes. Clin Vaccine Immunol (2009) 16:1222–7. doi: 10.1128/CVI.00166-09
274. Bonthius DJ. Lymphocytic choriomeningitis virus: An under-recognized cause of neurologic disease in the fetus, child, and adult. Semin Pediatr Neurol (2012) 19:89–95. doi: 10.1016/j.spen.2012.02.002
275. Jameson SC, Masopust D. Understanding subset diversity in T cell memory. Immunity (2018) 48:214–26. doi: 10.1016/j.immuni.2018.02.010
276. Topham DJ, Reilly EC. Tissue-resident memory CD8+ T cells: From phenotype to function. Front Immunol (2018) 9:515. doi: 10.3389/fimmu.2018.00515
277. Yenyuwadee S, Sanchez-Trincado Lopez JL, Shah R, Rosato PC, Boussiotis VA. The evolving role of tissue-resident memory T cells in infections and cancer. Sci Adv (2022) 8:eabo5871. doi: 10.1126/sciadv.abo5871
278. Wu T, Hu Y, Lee YT, Bouchard KR, Benechet A, Khanna K, et al. Lung-resident memory CD8 T cells (TRM) are indispensable for optimal cross-protection against pulmonary virus infection. J Leukoc Biol (2014) 95:215–24. doi: 10.1189/jlb.0313180
279. Masopust D, Vezys V, Marzo AL, Lefrançois L. Preferential localization of effector memory cells in nonlymphoid tissue. Science (2001) 291:2413–7. doi: 10.1126/science.1058867
280. Vardam-Kaur T, Sun J, Borges da Silva H. Metabolic regulation of tissue-resident memory CD8+ T cells. Curr Opin Pharmacol (2021) 57:117–24. doi: 10.1016/j.coph.2021.02.004
281. Collins N, Han SJ, Enamorado M, Link VM, Huang B, Moseman EA, et al. The bone marrow protects and optimizes immunological memory during dietary restriction. Cell (2019) 178:1088–1101.e15. doi: 10.1016/j.cell.2019.07.049
282. Porsche CE, Delproposto JB, Geletka L, O’Rourke R, Lumeng CN. Obesity results in adipose tissue T cell exhaustion. JCI Insight (2021) 6. doi: 10.1172/jci.insight.139793
283. Rebeles J, Green WD, Alwarawrah Y, Nichols AG, Eisner W, Danzaki K, et al. Obesity-induced changes in T-cell metabolism are associated with impaired memory T-cell response to influenza and are not reversed with weight loss. J Infect Dis (2019) 219:1652–61. doi: 10.1093/infdis/jiy700
284. LaMarche NM, Kohlgruber AC, Brenner MB. Innate T cells govern adipose tissue biology. J Immunol (2018) 201:1827–34. doi: 10.4049/jimmunol.1800556
285. Kuan EL, Ivanov S, Bridenbaugh EA, Victora G, Wang W, Childs EW, et al. Collecting lymphatic vessel permeability facilitates adipose tissue inflammation and distribution of antigen to lymph node-homing adipose tissue dendritic cells. J Immunol Baltim Md 1950 (2015) 194:5200–10. doi: 10.4049/jimmunol.1500221
286. Ivanov S, Scallan JP, Kim KW, Werth K, Johnson MW, Saunders BT, et al. CCR7 and IRF4-dependent dendritic cells regulate lymphatic collecting vessel permeability. J Clin Invest (2016) 126:1581–91. doi: 10.1172/JCI84518
287. Ryu W-S. New emerging viruses. Mol Virol Hum Pathog Viruses (2017), 289–302. doi: 10.1016/B978-0-12-800838-6.00021-7
288. Viboud C, Simonsen L. Global mortality of 2009 pandemic influenza a H1N1. Lancet Infect Dis (2012) 12:651–3. doi: 10.1016/S1473-3099(12)70152-4
289. Ur Rehmana MF, Farihab C, Anwar A, Shahzad N, Ahmad M, Mukhtar S, et al. Novel coronavirus disease (COVID-19) pandemic: A recent mini review. Comput Struct Biotechnol J (2021) 19:612–23. doi: 10.1016/j.csbj.2020.12.033
290. Van Kerkhove MD, Vandemaele KAH, Shinde V, Jaramillo-Gutierrez G, Koukounari A, Donnelly CA, et al. Risk factors for severe outcomes following 2009 influenza a (H1N1) infection: a global pooled analysis. PloS Med (2011) 8:e1001053. doi: 10.1371/journal.pmed.1001053
291. Wu C, Chen X, Cai Y, Xia J, Zhou X, Xu S, et al. Risk factors associated with acute respiratory distress syndrome and death in patients with coronavirus disease 2019 pneumonia in wuhan, China. JAMA Intern Med (2020) 180:934–43. doi: 10.1001/jamainternmed.2020.0994
292. Kaeuffer C, Le Hyaric C, Fabacher T, Mootien J, Dervieux B, Ruch Y, et al. Clinical characteristics and risk factors associated with severe COVID-19: prospective analysis of 1,045 hospitalised cases in north-Eastern France, march 2020. Eurosurveillance (2020) 25:2000895. doi: 10.2807/1560-7917.ES.2020.25.48.2000895
293. Kalil AC, Thomas PG. Influenza virus-related critical illness: pathophysiology and epidemiology. Crit Care (2019) 23:258. doi: 10.1186/s13054-019-2539-x
294. Evans SS, Repasky EA, Fisher DT. Fever and the thermal regulation of immunity: the immune system feels the heat. Nat Rev Immunol (2015) 15:335–49. doi: 10.1038/nri3843
295. Schieber AMP, Ayres JS. Thermoregulation as a disease tolerance defense strategy. Pathog Dis (2016) 74:ftw106. doi: 10.1093/femspd/ftw106
296. Barthelemy J, Wolowczuk I. Influenza a virus infection induces white adipose tissue browning: A metabolic adaptation to infection? J Cell Immunol (2020) 2:276–83. doi: 10.33696/immunology.2.055
297. Freeman DW, Barno A. Deaths from Asian influenza associated with pregnancy. Am J Obstet Gynecol (1959) 78:1172–5. doi: 10.1016/0002-9378(59)90570-8
298. Flerlage T, Boyd DF, Meliopoulos V, Thomas PG, Schultz-Cherry S. Influenza virus and SARS-CoV-2: pathogenesis and host responses in the respiratory tract. Nat Rev Microbiol (2021) 19:425–41. doi: 10.1038/s41579-021-00542-7
299. Zhu N, Zhang D, Wang W, Li X, Yang B, Song J, et al. A novel coronavirus from patients with pneumonia in China, 2019. N Engl J Med (2020) 382:727–33. doi: 10.1056/NEJMoa2001017
300. Almaghaslah D, Kandasamy G, Almanasef M, Vasudevan R, Chandramohan S. Review on the coronavirus disease (COVID-19) pandemic: Its outbreak and current status. Int J Clin Pract (2020) 74:e13637. doi: 10.1111/ijcp.13637
301. Scialo F, Daniele A, Amato F, Pastore L, Matera MG, Cazzola M, et al. ACE2: The major cell entry receptor for SARS-CoV-2. Lung (2020) 198:867–77. doi: 10.1007/s00408-020-00408-4
302. Dias SSG, Soares VC, Ferreira AC, Sacramento CQ, Fintelman-Rodrigues N, Temerozo JR, et al. Lipid droplets fuel SARS-CoV-2 replication and production of inflammatory mediators. PloS Pathog (2020) 16:e1009127. doi: 10.1371/journal.ppat.1009127
303. Ryan PM, Caplice NM. Is adipose tissue a reservoir for viral spread, immune activation, and cytokine amplification in coronavirus disease 2019? Obes Silver Spring Md (2020) 28:1191–4. doi: 10.1002/oby.22843
304. Kruglikov IL, Scherer PE. The role of adipocytes and adipocyte-like cells in the severity of COVID-19 infections. Obes Silver Spring Md (2020) 28:1187–90. doi: 10.1002/oby.22856
305. Colleluori G, Graciotti L, Pesaresi M, Di Vincenzo A, Perugini J, Di Mercurio E, et al. Visceral fat inflammation and fat embolism are associated with lung’s lipidic hyaline membranes in subjects with COVID-19. Int J Obes 2005 (2022) 46:1009–17. doi: 10.1038/s41366-022-01071-w
306. Basolo A, Poma AM, Bonuccelli D, Proietti A, Macerola E, Ugolini C, et al. Adipose tissue in COVID-19: detection of SARS-CoV-2 in adipocytes and activation of the interferon-alpha response. J Endocrinol Invest (2022) 45:1021–9. doi: 10.1007/s40618-022-01742-5
307. Saccon TD, Mousovich-Neto F, Ludwig RG, Carregari VC, dos Anjos Souza AB, dos Passos ASC, et al. SARS-CoV-2 infects adipose tissue in a fat depot- and viral lineage-dependent manner. Nat Commun (2022) 13(1):5722. doi: 10.1038/s41467-022-33218-8
308. Fisman EZ, Tenenbaum A. Adiponectin: a manifold therapeutic target for metabolic syndrome, diabetes, and coronary disease? Cardiovasc Diabetol (2014) 13:103. doi: 10.1186/1475-2840-13-103
309. Olivo A, Marlin R, Lazure T, Maisonnasse P, Bossevot L, Mouanga C, et al. Detection of SARS-CoV-2 in subcutaneous fat but not visceral fat, and the disruption of fat lymphocyte homeostasis in both fat tissues in the macaque. Commun Biol (2022) 5:1–10. doi: 10.1038/s42003-022-03503-9
310. Koethe JR, McDonnell W, Kennedy A, Abana CO, Pilkinton M, Setliff I, et al. Adipose tissue is enriched for activated and late-differentiated CD8+ T cells, and shows distinct CD8+ receptor usage, compared to blood in HIV-infected persons. J Acquir Immune Defic Syndr 1999 (2018) 77:e14–21. doi: 10.1097/QAI.0000000000001573
311. Wanjalla CN, McDonnell WJ, Barnett L, Simmons JD, Furch BD, Lima MC, et al. Adipose tissue in persons with HIV is enriched for CD4+ T effector memory and T effector memory RA+ cells, which show higher CD69 expression and CD57, CX3CR1, GPR56 Co-expression with increasing glucose intolerance. Front Immunol (2019) 10:408. doi: 10.3389/fimmu.2019.00408
312. Mackay LK, Braun A, Macleod BL, Collins N, Tebartz C, Bedoui S, et al. Cutting edge: CD69 interference with sphingosine-1-phosphate receptor function regulates peripheral T cell retention. J Immunol Baltim Md 1950 (2015) 194:2059–63. doi: 10.4049/jimmunol.1402256
313. Kumar BV, Ma W, Miron M, Granot T, Guyer RS, Carpenter DJ, et al. Human tissue-resident memory T cells are defined by core transcriptional and functional signatures in lymphoid and mucosal sites. Cell Rep (2017) 20:2921–34. doi: 10.1016/j.celrep.2017.08.078
314. Mackay LK, Rahimpour A, Ma JZ, Collins N, Stock AT, Hafon ML, et al. The developmental pathway for CD103+CD8+ tissue-resident memory T cells of skin. Nat Immunol (2013) 14:1294–301. doi: 10.1038/ni.2744
315. Chen Z-Y, Wang L, Gu L, Qu R, Lowrie DB, Hu Z, et al. Decreased expression of CD69 on T cells in tuberculosis infection resisters. Front Microbiol (2020) 11:1901. doi: 10.3389/fmicb.2020.01901
316. Caputa G, Castoldi A, Pearce EJ. Metabolic adaptations of tissue-resident immune cells. Nat Immunol (2019) 20:793–801. doi: 10.1038/s41590-019-0407-0
317. Cibrian D, Saiz ML, de la Fuente H, Sánchez-Díaz R, Moreno-Gonzalo O, Jorge I, et al. CD69 controls the uptake of l-tryptophan through LAT1-CD98 and AhR-dependent secretion of IL-22 in psoriasis. Nat Immunol (2016) 17:985–96. doi: 10.1038/ni.3504
Keywords: adipose tissue, immune cells, pathogens, influenza, SARS-CoV-2
Citation: Barthelemy J, Bogard G and Wolowczuk I (2023) Beyond energy balance regulation: The underestimated role of adipose tissues in host defense against pathogens. Front. Immunol. 14:1083191. doi: 10.3389/fimmu.2023.1083191
Received: 28 October 2022; Accepted: 09 January 2023;
Published: 02 March 2023.
Edited by:
Luzia Teixeira, Institute of Biomedical Sciences Abel Salazar - University of Porto, PortugalReviewed by:
Hiroshi Wakao, Dokkyo Medical University, JapanJason Kyle Whitmire, University of North Carolina at Chapel Hill, United States
Copyright © 2023 Barthelemy, Bogard and Wolowczuk. This is an open-access article distributed under the terms of the Creative Commons Attribution License (CC BY). The use, distribution or reproduction in other forums is permitted, provided the original author(s) and the copyright owner(s) are credited and that the original publication in this journal is cited, in accordance with accepted academic practice. No use, distribution or reproduction is permitted which does not comply with these terms.
*Correspondence: Isabelle Wolowczuk, aXNhYmVsbGUud29sb3djenVrQHBhc3RldXItbGlsbGUuZnI=