- 1Applied and Analytical Palaeontology, Institute of Geosciences, Johannes Gutenberg University, Mainz, Germany
- 2Department of Natural Environmental Studies, Graduate School of Frontier Sciences, The University of Tokyo, Kashiwa, Chiba, Japan
- 3Leibniz Institute for the Analysis of Biodiversity Change, Zoological Museum, Hamburg, Germany
- 4Clinic for Zoo Animals, Exotic Pets and Wildlife, Vetsuisse Faculty, University of Zurich, Zurich, Switzerland
- 5Department of Cariology, Endodontology and Periodontology, University of Leipzig, Leipzig, Germany
- 6Granovit Zoofeed, Granovit AG, Kaiseraugst, Switzerland
- 7Department of Veterinary and Biosciences, Faculty of Veterinary Medicine, Ghent University, Merelbeke, Belgium
- 8The University Museum, The University of Tokyo, Tokyo, Japan
In mammals, complex dental microwear textures (DMT) representing differently sized and shaped enamel lesions overlaying each other have traditionally been associated with the seeds and kernels in frugivorous diets, as well as with sclerotized insect cuticles. Recently, this notion has been challenged by field observations as well as in vitro experimental data. It remains unclear to what extent each food item contributes to the complexity level and is reflected by the surface texture of the respective tooth position along the molar tooth row. To clarify the potential of seeds and other abrasive dietary items to cause complex microwear textures, we conducted a controlled feeding experiment with rats. Six individual rats each received either a vegetable mix, a fruit mix, a seed mix, whole crickets, whole black soldier fly larvae, or whole day-old-chicks. These diets were subjected to material testing to obtain mechanical properties, such as Young’s modulus, yield strength, and food hardness (as indicated by texture profile analysis [TPA] tests). Seeds and crickets caused the highest surface complexity. The fruit mix, seed mix, and crickets caused the deepest wear features. Moreover, several diets resulted in an increasing wear gradient from the first to the second molar, suggesting that increasing bite force along the tooth row affects dental wear in rats on these diets. Mechanical properties of the diets showed different correlations with DMT obtained for the first and second molars. The first molar wear was mostly correlated with maximum TPA hardness, while the second molar wear was strongly correlated with maximum yield stress, mean TPA hardness, and maximum TPA hardness. This indicates a complex relationship between chewing mechanics, food mechanical properties, and observed DMT. Our results show that, in rats, seeds are the main cause of complex microwear textures but that hard insect body parts can also cause high complexity. However, the similarity in parameter values of surface textures resulting from seed and cricket consumption did not allow differentiation between these two diets in our experimental approach.
Introduction
The reconstruction of paleodiets is a central aspect of paleoecology. Diet provides insights not only to an animal’s ecology but also, indirectly, to aspects of its habitat and predominant climatic conditions (e.g., closed vs. open, arid vs. humid) (e.g., Merceron et al., 2007a,b; Rivals et al., 2008) and on how these are linked to the evolution of different taxa (e.g., Teaford and Ungar, 2000). Thus, methods for paleodietary inference have always been of interest to the scientific community, but even more so when they allowed inferences about our ancestors and human evolution (e.g., Walker, 1981). However, when hominids are involved, not only is the interest but also the potential for debate high, as new results may challenge long-standing paradigms (Lee-Thorp, 2011). One important question is whether seeds played a prominent role as a key dietary component responsible for distinct morphological adaptations like thick enamel, enlarged, bunodont teeth, and complex microwear patterns. It applies to primates in general and hominids in particular.
Extant primates (Kay, 1981; Dumont, 1995; Shellis et al., 1998; Martin et al., 2003) and bats (Dumont, 1995) that incorporate a large proportion of so-called hard objects into their diet possess thick enamel. Hence, the presence of thick enamel has also been interpreted as an adaptation toward hard-object feeding in fossil species (e.g., Alba et al., 2010), including hominids (e.g., Martin et al., 2003). The relationship is, however, not straightforward, as thinner enamel may also be suitable for processing hard objects, as seen, for instance, in sea otters (Constantino et al., 2011). It seems that tooth crown morphology, enamel distribution, and enamel microstructure also need to be considered to predict the resistance of teeth during hard-object feeding (Constantino et al., 2011; Ungar, 2011; Schwartz et al., 2020).
Microwear and dental microwear texture (DMT) are often-applied “proxies” that are analyzed to infer the mechanical properties of the foods consumed (Calandra and Merceron, 2016). By directly observing the two-dimensional (pits and scratches) (Rivals et al., 2007; Semprebon and Rivals, 2007) or three-dimensional (roughness, complexity, anisotropy) patterns on enamel wear facets in extant species, inferences can be drawn about the dietary habits of extinct species (e.g., Scott et al., 2005; Ungar et al., 2008, 2010; DeSantis et al., 2012). One long-standing paradigm regarding specifically primate and hominid diets has been that hard object feeding (i.e., seeds and nuts) results in pitted enamel surfaces (Grine and Kay, 1988) with high complexity (Scott et al., 2005, 2012; Daegling et al., 2011).
However, there are cases when dental morphology and observed microwear features seem to be incongruent. The genus Paranthropus is an excellent example of how similar morphologies may have been used in different dietary specializations (Ungar et al., 2008; Sponheimer et al., 2022). There is an ongoing debate whether their enlarged molars with thick enamel caps were an adaptation for processing bulk low-quality forage (e.g., grasses). The similarity of the enlarged molars to extant hard object-feeders (e.g., otters, peccary) suggests that fracture resistance while processing nuts and seeds was the driving force behind the evolution of this particular tooth morphology [see Sponheimer et al. (2022) for a comprehensive review].
Both eastern (Paranthropus boisei) and southern (Paranthropus robustus) members of the genus Paranthropus possessed enlarged, bunodont molars with thick enamel caps, yet their reconstructed diet differs drastically. Dental micrwoear texture analysis (DMTA) (Ungar et al., 2008; Ungar, 2012) and isotope evidence (van der Merwe et al., 2008) suggest that P. boisei was primarily feeding on C4/CAM plants (and/or meat of animals that consumed C4 grasses), which is considered tough but not hard food—an interpretation not easily reconciled with their thick enamel cusps (Sponheimer et al., 2022). By contrast, P. robustus is reconstructed as a hard-object feeder (Constantino et al., 2018), based on microwear and DMTA that reveal high complexity but low anisotropy values, both typically associated with hard-object feeding (Scott et al., 2005; Ungar et al., 2008; Ungar and Berger, 2018). Until recently, the diet of P. robustus was less subject to debate, as most studies (microwear, DMTA, tooth macrowear) suggested hard objects as an important dietary component. When talking about hard objects in an herbivorous diet, we usually imply seeds and nuts (Daegling et al., 2011; Ungar and Sponheimer, 2011).
Recently, this notion has been challenged by an experimental in vitro study by van Casteren et al. (2020). In vitro, they brought endocarps into contact with isolated human teeth and found that the endocarp per se was not able to cause deep lesions but only rubbing (plastic deformation) of the enamel. Thus, they concluded that seeds could not be the source of the high complexity in surface textures that were conventionally associated with hard-object feeding. Moreover, they suggested that this would bring seeds back on the table as a possible diet for Paranthropus boisei, as the lack of complexity in microwear textures would no longer be indicative of an absence of seeds in the diet. This is not the first debate that has arisen in the dental wear community. Similarly, the discussion of whether phytoliths (amorphous silica bodies inside the plant tissue) or external grit and mineral dust are more responsible for abrasive wear has been a long one as controlled feeding experiments provided abundant evidence for the importance of both as dietary abrasives (Merceron et al., 2016; Winkler et al., 2019a, 2020a; Schulz-Kornas et al., 2020a). Similarly, controlled feeding experiments demonstrated that hard-object (nut) feeding results in new dental microwear features after only one feeding bout in capuchin monkeys (Teaford et al., 2020), and the inclusion of seeds or nuts into otherwise identical diets results in distinct DMT in pigs (Louail et al., 2021), but without resolving whether high complexity is linked to seed consumption.
In the present study, we conducted a feeding experiment with rats to better determine which naturally ingested animal and plant diets can produce high complexity (in absence of external abrasives). We chose rats because they are omnivorous and can deal with various diets. Rat molar teeth have served as models for human molars in numerous studies, although evident differences in masticatory behavior and dental morphology are acknowledged (Ohba, 1974; Nishijima et al., 2007, 2009; Dammaschke, 2010). Rats cannot serve as a model organism for replication of the Paranthropus masticatory behavior. What rats can do, however, is to show what kind of diets do result in more complex microwear textures that are traditionally associated with herbivorous hard-object feeding.
Diets of omnivores are naturally diverse and complex in composition and are thus likely diverse in mechanical properties, and we did not aim at mimicking the natural diet of either rats or primates. Rather, we aimed to design experimental diets that resemble a specialized feeding type. Thus, we used several herbivore and faunivore diets with different components that remain constant over the duration of the experiment: a vegetable mix, a fruit mix (whole fruits with seeds), a seed mix (legume, C3 and C4 grass, and sunflower seeds), whole house crickets (hereafter: crickets), whole black soldier fly larvae (hereafter: BSFL) and whole day-old chicks (hereafter: daychicks). We subjected these diets to tests of mechanical properties that can be obtained through compression tests to relate them to the observed DMT. The mechanical properties we focused on were as follows: Young’s modulus (E), yield stress, and texture profile analysis (TPA) hardness, because they are well-represented in biomechanical anthropological studies (Young’s modulus, yield stress) (e.g., Lucas et al., 2009; Thompson et al., 2014; Berthaume, 2016) or food texture studies (TPA hardness) (e.g., Nishinari et al., 2019). In addition, they can be obtained with a relatively simple analytical setup. We note that this approach is simplified and does not cover other commonly used measures of mechanical properties (e.g., obtained through wedge or scissor tests) or potential cuticle hardness in insects (Evans and Sanson, 2005) and have discussed the benefits and limitations.
In a previous study on guinea pigs, we found that, for hard and brittle, pelleted diets, a DMTA gradient indicating increasing wear from front to rear along the molar tooth row could be observed (Winkler et al., 2021), while natural plant diets displayed no such gradient or even showed an opposite trend of decreasing wear from the fourth premolar toward the third molar. As the rats in the present study were subjected to diets of very different mechanical properties, we were interested to see if they would also show tooth position-specific differences that reflected specific mastication behavior on different diets. We thus compared DMTA not only between diet groups but also between the first and second upper molars within each diet group.
Materials and methods
The feeding experiment was approved by the Swiss Cantonal Animal Care and Use Committee Zurich (animal experiment license No. ZH135/16) and was conducted during February/March 2018 at the Vetsuisse Faculty, University of Zurich. All animals in this experiment were female adult WISTAR (RjHan:WI) rats (Rattus norvegicus forma domestica; n = 36; initial body mass = 216.5 ± 16.5 g; initial age 12–14 weeks). Rats were kept in groups of 3 in indoor stables (0.58 m2 each), each equipped with one or two large food dishes containing their assigned experimental diet and two nipple drinkers of tap water. Stables were enriched with dust-free softwood granulate, a shelter, a climbing frame, two tubes, and a running plate (Frei et al., 2021). Two groups (i.e., 6 animals in total) received the same diet for 37 consecutive days. Initially, the breeder diet was provided in addition to the experimental diet and phased out until the 5th day of the experiment. Following the conclusion of the experiment, animals were euthanized with carbon dioxide and enzymatic maceration of the skulls was conducted at LIB Hamburg [former Center of Natural History (CeNak) of the University of Hamburg]. For the vegetable and fruit groups, one specimen each lost its label during maceration and could not be assigned without uncertainty to the correct diet group. Thus, the groups of vegetable and fruit eaters are only comprised of five individuals each.
Experimental diets
The six experimental diets consisted of a vegetable mix, a fruit mix, a seed mix, crickets, black soldier fly larvae, and day-old chicks. Weight percentages of each main dietary component are given in Table 1. Diets were designed to be isocaloric and to contain all nutrients to sustain healthy growth in a 250 g rat. Rats received their designated diets one time per day in the morning. Each diet was supplemented by a specifically balanced supplement powder that was admixed to a quark (a type of dairy product, also known as curd or curd cheese) or a quark and sunflower oil base (Table 1), which were chosen as additional protein and fat supply that could not be chewed but had to be lapped up by the animals. The composition of each supplement powder is given in the Supplementary Table 1.
Mechanical properties testing and limitations
Several diets included a mixture of food items, with likely different mechanical properties. Thus, rats could theoretically choose foods that they preferred over foods they disliked. Such behavior was observed in the group receiving a vegetable mix, where several rats would avoid eating root celery (pers. observation DEW). The groups receiving crickets and black soldier fly larvae had no such choice, except for avoiding eating body parts of the crickets (e.g., legs). Similarly, the daychick group could selectively feed on certain parts of the whole daychicks, but only left the beak and parts of the integument with feathers (pers. observation DEW). In the groups receiving fruit mixture and seed mixture, no preferences were observed, as food was consumed completely. However, we cannot rule out that individual rats had specific preferences and avoided specific items, while other rats consumed them. Therefore, we expect more variability of DMT parameters in groups that received a mixture of food items (vegetables, fruits, and seeds) as compared to more homogenous diets (crickets, BSFL, and daychicks).
Although the behavior and individual preferences of the rats could likely affect the items they consumed (and thus the tooth wear they developed), we conducted compression tests to derive quantitative mechanical properties of the items. Through the compression tests, we obtained stress-strain curves from which representative mechanical properties, i.e., Young’s modulus, yield stress, and TPA hardness, were calculated. Due to limitations in the preparation of analyzed samples, these three mechanical properties could not be obtained simultaneously for all items but had to be derived from linear regressions with other highly correlated mechanical properties (see Statistics section).
For obtaining Young’s modulus and yield stress, samples of fruit and vegetables were cut into rectangular, circular, or cubic pieces; seeds were tested as whole grains and insects as whole specimens. In crickets, we additionally tested the head capsule and the gizzard separately. For both, an approximately circular shape was assumed. The surface area of whole insects and grains was approximated from a rectangular shape. For daychicks, it was not possible to obtain circular or rectangular pieces, thus we only subjected them to TPA hardness tests (see below). The vegetable diet included two leafy greens (parsley, spinach) which are not suitable for compression tests. We did not assess the mechanical properties of these two items. Similarly, for the fruit mix, we restricted testing of figs to the isolated fig seeds, as the available figs were too soft to be cut into circular or rectangular pieces. Fig seeds are expected to be the hardest component of the fruit mix diet and, consequently, to contribute more to the observed wear differences than the soft parts of the figs.
Testing was conducted over several days of one week, in the same laboratory environment, to reduce variations in humidity and temperature. Each sample was placed on a circular platform of 60 mm diameter under a force gauge and compressed in a cutoff setup until breaking or until the force gauge registered an overload (due to contact with the platform). We used circular solid testers of different diameters (60 mm, 15 mm, 3 mm) but ensured that the tester was larger than the sample for obtaining Young’s modulus and yield stress so that compression would be evenly exerted over the complete surface area of the sample. The movement speed of the circular solid tester was set to 600 mm/min and the test speed (from contact point on) to 120 mm/min. We used two different force gauges, HF-100 and HF-10 (JISC, Japan Instrumental Systems Inc., Nara, Japan), attached to an automated servo stand (JSV-H1000, JISC, Japan Instrumental Systems Inc., Nara, Japan). The HF-100 has a maximum load capacity of 1000 N and a resolution of 0.1 N, while the HF-10 has a maximum load capacity of 100 N and a resolution of 0.01 N. Thus, for soft, easily deformable samples, the force gauge HF-10 with a higher precision was employed. For fruit and vegetables that were fed including the skin, we conducted multiple compression tests both from the skin side or from the side without skin. A representative stress-strain diagram and the results of mechanical properties testing are shown in Figure 1. Detailed results of mechanical properties (Young’s modulus, yield stress, false yield stress, TPA hardness) are illustrated in Supplementary Tables 2–4. We note that values obtained for Young’s modulus are negative, because we used a compression setup. However, the sign is usually omitted in the literature, thus when we are referring to larger Young’s modulus, we would refer to a more negative value. To avoid confusion, we presented all values as positive numbers.
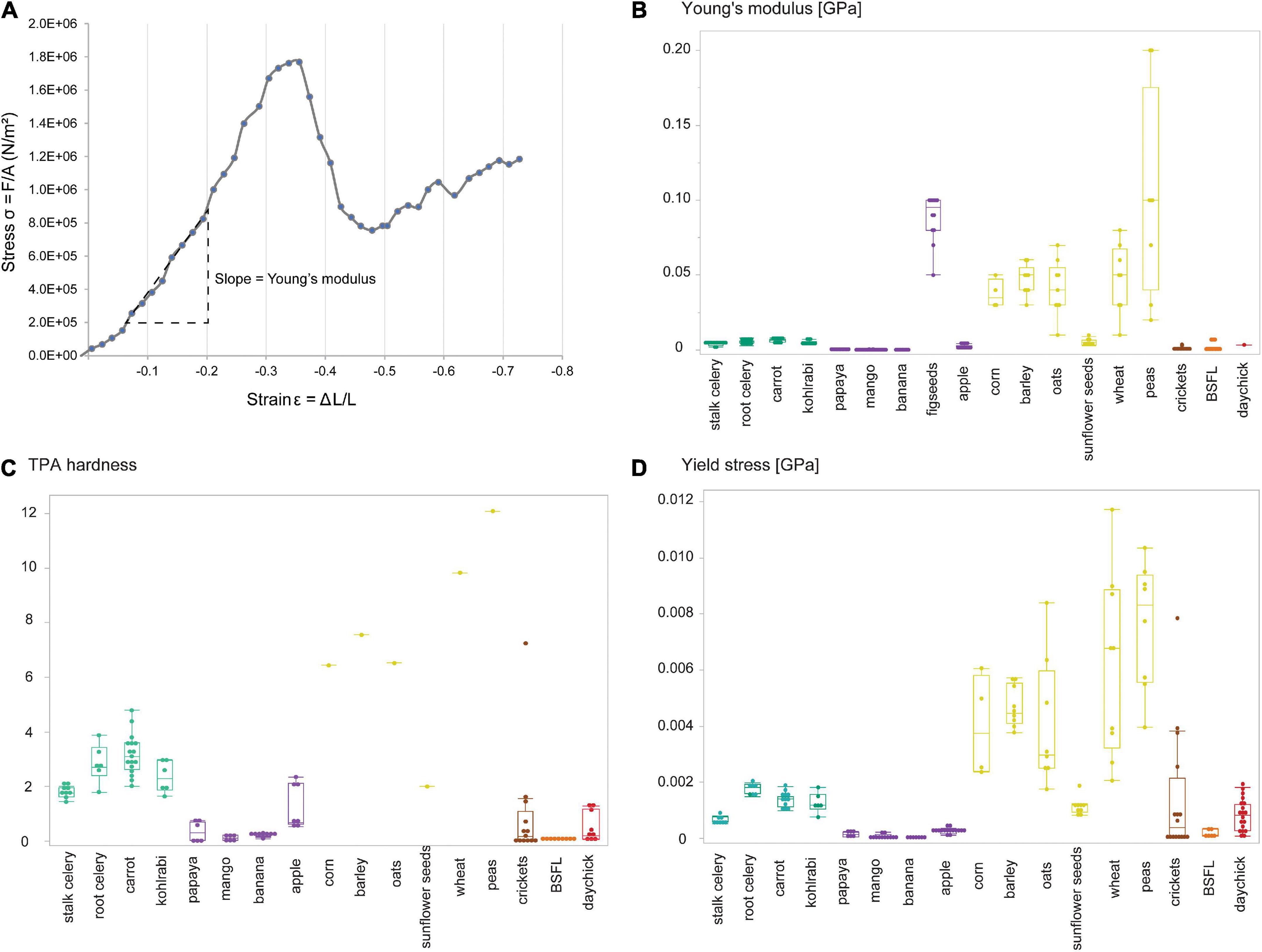
Figure 1. Mechanical properties of components of near natural diets. (A) Exemplary stress-strain curve obtained for a carrot cube. (B) Young’s modulus, (C) TPA hardness, and (D) Yield stress obtained for the different diet components. TPA hardness is commonly reported unitless but would have the unit N/mm2. Colors highlight the different experimental diets to which the individual items belong to. Green = vegetable mix, purple = fruit mix, yellow = seed mix, brown = crickets (including whole crickets, isolated head capsules, and gizzards), orange = black soldier fly larvae (BSFL), red = daychicks.
Additionally, the food texture tester TEX-100 (JISC, Japan Instrumental Systems Inc., Nara, Japan) was used to obtain the standardized parameter TPA hardness. In food science, TPA hardness is measured by compressing a sample two times for the same compression depth or ratio, with the peak force occurring during the first compression divided by the tester area being the hardness (Bourne, 2002; Nishinari et al., 2019). An example curve is represented in Supplementary Figure 1. All raw measurement results are given in Supplementary Table 4. We used a 3-mm circular solid tester (area = 7.069 mm2), at a test movement speed of 120 mm/min, and an indentation depth of 2 mm. We chose this small 3-mm tester following the advice from Nishinari et al. (2019) that the tester should be less than one-third of the diameter of the sample. The hold time between the first and second indentations was set to 3 s. This hardness measure is different from (micro) indentation hardness measures such as Vickers or Knoop hardness. As TPA hardness should only be measured on food items with a certain elasticity so that repeated compression would be possible, we did not test individual items from the seed mix, the cricket head capsules, and the cricket gizzards using this procedure, as they fractured during the first indentation.
Dental microwear texture measurements
Measurements were conducted at the LIB Hamburg [former Center of Natural History (CeNak) of the University of Hamburg], Germany, on a μsurf Custom (NanoFocus AG, Oberhausen, Germany) confocal disc-scanning microscope, equipped with a blue LED (470 nm) and high-speed progressive-scan digital camera (984 × 984 pixel), a 100x long working-distance objective (resolution in x, y = 0.16 μm, step size in z = 0.06 μm), following the published routine for DMT data (Schulz et al., 2010, 2013) with adjustment for rats (Winkler et al., 2016) and guinea pigs (Winkler et al., 2019a, 2020a,2021). Measurements were performed on original tooth surfaces of the first and second upper molars on central anterior enamel bands. Depending on wear stage and preservation, up to four (minimum 2) non-overlapping scans were taken from each specimen. Forty-four DMT parameters were calculated after filtering surfaces [leveling, spatial filtering (denoising median 5 × 5 filter size and Gaussian 3 × 3 filter size with default cut-offs), filling of non-measured points, noise-reduction by thresholding (upper and lower 0.5%), removal of outliers (maximum slope of 85%), 2nd order form removal] and cropping them to 60 × 60 μm in MountainsMap v.9.0.9878. From the two to four scans per specimen, median values were calculated for each parameter to adequately represent the surface texture of the enamel and avoid bias by picking particularly worn or unworn locations. Parameter results for each specimen are given in Supplementary Table 5.
Statistics
Descriptive and test statistics were computed in JMP Pro v. 16.0. A non-parametric, heteroscedastic pairwise comparison test (Wilcoxon test) was performed for DMTA of all dietary pairs. The application of multiple comparison tests increases the probability of Type I error; however, conservative corrections for multiple testing would inflate the probability of Type II error in small samples such as ours. We hence applied the Benjamini–Hochberg procedure with an accepted false discovery rate (FDR) of 0.25. We therefore note that the results of diet group comparisons presented here were prone to include false positives but accepted this on the basis of it being an exploratory study and including both raw p-values and critical p-values using an FDR of 0.25 into the supplements (Supplementary Table 10).
Mechanical properties parameters (Young’s modulus, yield stress, TPA hardness) were repeatedly measured for multiple individual samples (4–20 repetitions) and then averaged for each dietary item. Individual measurements are given in Supplementary Tables 2–4. Some diets were composed of several different items (vegetable mix, fruit mix, seed mix), while others were composed of one item only (BSFL, crickets, and daychicks). To generate representative mechanical properties from each diet, we calculated two values for each mechanical properties parameter: a weighted mean using relative proportions of diet items and a maximum using the largest value obtained for an individual diet component. For crickets, we tested whole specimens, head capsules, and gizzards and calculated a weighted mean based on weight percentages (Supplementary Tables 6, 7). For the diets BSFL and daychicks, the weighted mean and maximum value were identical.
As we could not obtain either Young’s modulus and yield stress for daychicks or TPA hardness for the seed mix, cricket heads, and cricket gizzards, we used linear regressions to establish the nature of the relationships between measurable mechanical properties and used these regressions to infer the parameter values that we were unable to measure.
A fourth parameter, false yield strength, was employed to estimate yield stress for daychicks. We calculated false yield strength during compression of irregularly shaped objects as maximum force recorded divided by the area of the circular solid tester (maxF/testerArea). The false yield was found to be significantly correlated with yield stress (R2 = 0.986, p < 0.0001) for the dietary items in which we could obtain both. Yield stress for daychicks was inferred from the regression equation:
Young’s modulus was also found to be significantly correlated with yield stress (R2 = 0.967, p < 0.0001). Young’s modulus for daychicks was obtained from the regression equation:
TPA hardness was significantly correlated with yield stress (R2 = 0.966, p < 0.0001), and TPA hardness values for seeds, cricket heads, and cricket gizzards were obtained from the equation:
It is important to note that, in the case of seeds and cricket gizzards, the extrapolated values were mostly outside of the range of the other measurements used to generate the regression equation and hence were particularly susceptible to error. Therefore, the following evaluation of these data was done using non-parametric tests only.
As mechanical properties parameters could not be normalized by common transformations, and DMTA parameters are also not normally distributed, we subsequently conducted Spearman correlations between all DMTA and mechanical properties parameters (Supplementary Tables 8, 9).
Results
Dietary differences
The vegetable mix, BSFL, and daychick diets caused visibly lower surface roughness and smaller wear features on the M1 (Figure 2). The groups receiving daychicks and BSFL were best distinguished from the other diet groups when the M1 is considered (Table 2 and Figure 3; Supplementary Figure 2). For the M2, the daychick diet was less distinct from the other diet groups. Seeds and crickets were not significantly different from each other for any parameter on both M1 and M2. Seeds and crickets showed the highest complexity values, but these were not significantly larger compared to the other diet groups due to the large variance observed. A detailed description of DMTA parameter results for each diet group is given in the supplementary text in the Supplementary material. There was no trend of an increased variability in DMTA parameters for the more heterogenous diets.
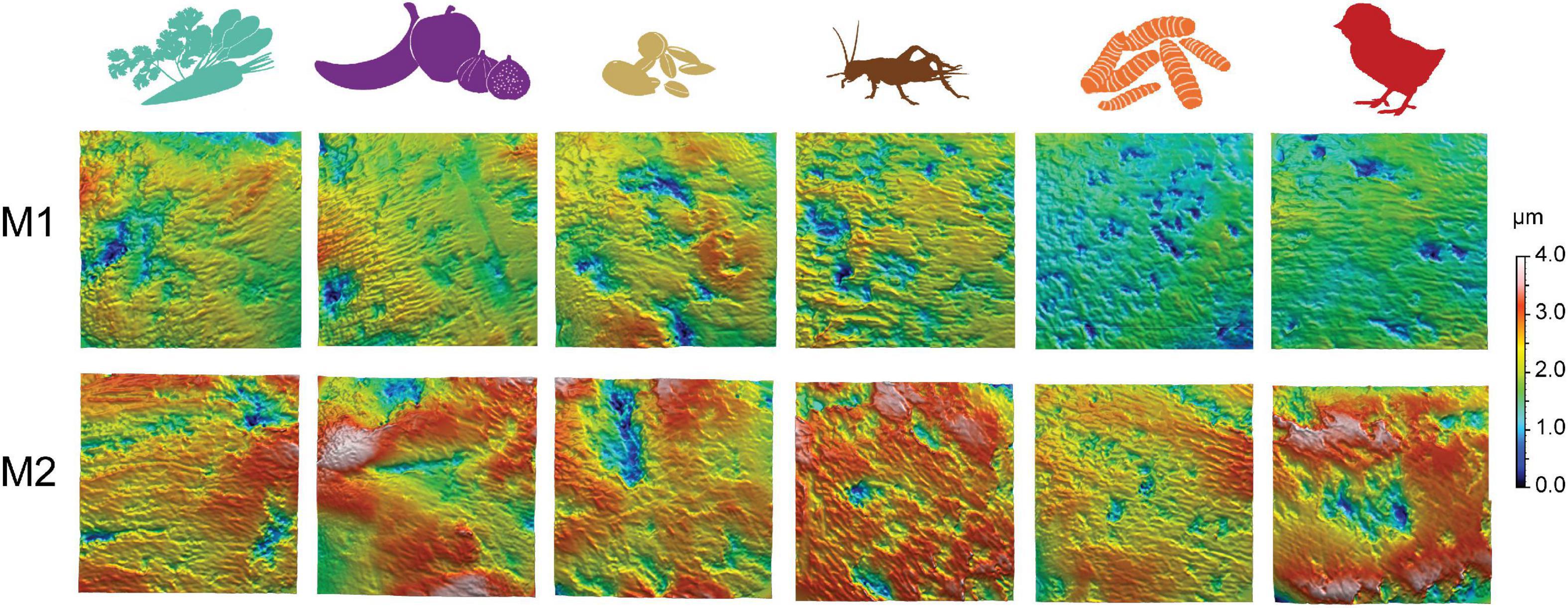
Figure 2. Exemplary 3D photosimulations of representative enamel surface scans for each diet group (from left to right: vegetable mix, fruit mix, seed mix, crickets, BSFL, black soldier fly larvae, and daychicks). Scans of the first (M1) and second upper molars (M2) belong to the same individual and are displayed on the same scale. Each surface scan is 60 × 60 μm.
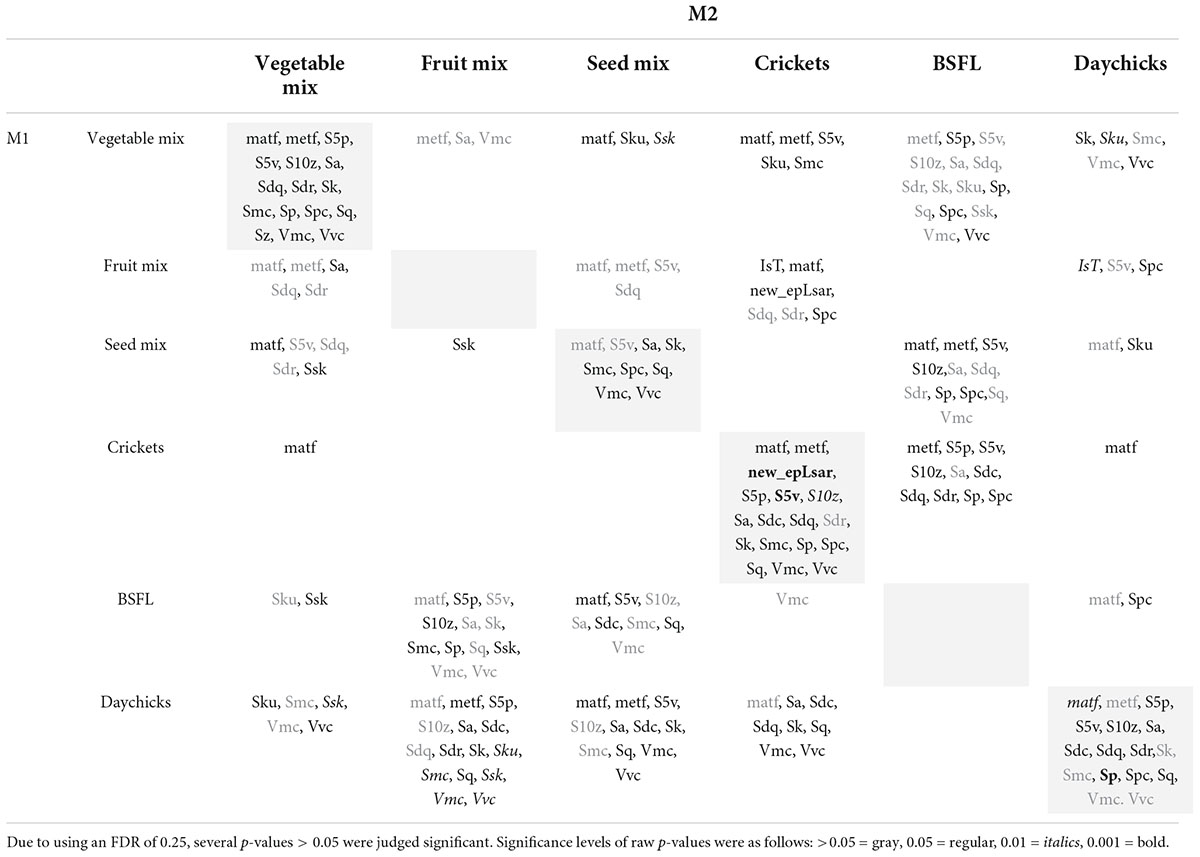
Table 2. DMTA parameters displaying significant differences after the application of the Benjamini–Hochberg procedure between diet groups for the same tooth position (white cells) and between tooth positions within the same diet group (gray shaded cells).
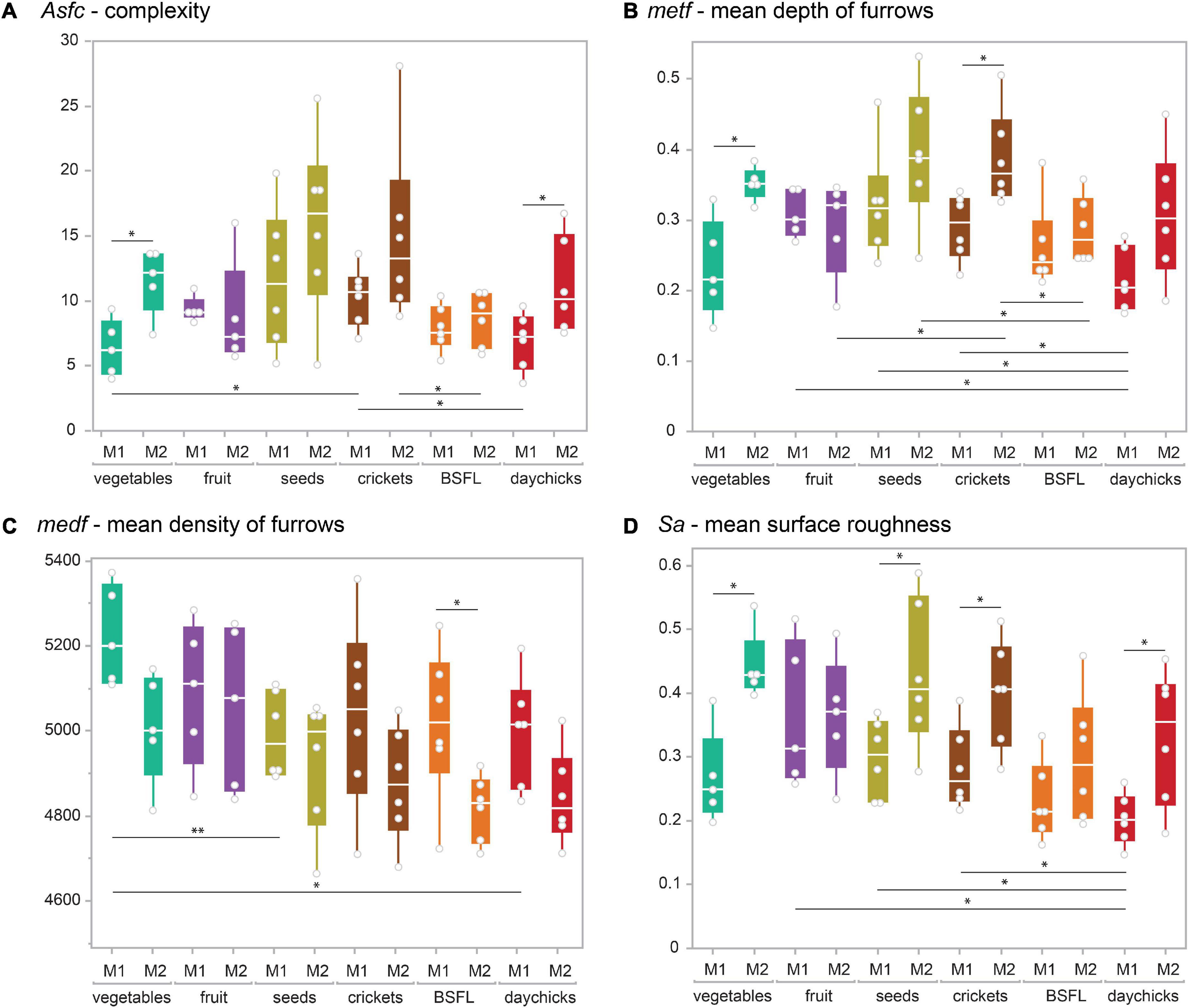
Figure 3. Boxplots depicting representative (A) complexity (Asfc), (B) density (medf), (C) and height (metf), (D) (Sa) parameters for the first (M1) and second upper molars (M2) of all dietary groups. Significant differences between tooth positions within one dietary group are displayed above, while differences between dietary groups for the same tooth position are displayed below the boxplots. Significance levels: *0.05, **0.01, from the Wilcoxon test for multiple comparisons without correction. BSFL, black soldier fly larvae.
Tooth position-specific differences
For all diet groups, except fruit and BSFL, more than one DMTA parameter was significantly different between M1 and M2 within the same diet (Table 2). The differences were mostly due to height and volume parameters, with parameter values consistently increasing from M1 to M2.
Diet mechanical properties
Young’s modulus was largest for dried peas and fig seeds (Figure 1B). Overall, the items from the seed mix had larger Young’s modulus than other diets, resulting in the largest mean per diet. Insects (crickets and BSFL) and fruit mix showed the lowest mean Young’s modulus, followed by vegetables and daychicks.
Using regression equations, it was inferred that TPA hardness was largest in all items from the seed mix except sunflower seeds and cricket gizzards (Figure 1C; Supplementary Table 4). The largest measured TPA hardness values were found for several vegetables (root celery, carrot, kohlrabi), followed by stalk celery and apple. Some individual samples of daychick body parts had higher values that were obtained from whole specimens, while on average, fruits, insects, and daychicks showed the lowest TPA hardness values.
The highest yield stress was found for items of the seed mix, with dried peas exceeding all other seeds, and cricket gizzards (Figure 1D; Supplementary Table 3). Sunflower seeds had much lower yield stress values, comparable to vegetables and daychicks. Fruit and whole insects (crickets and BSFL) had the lowest yield stress, while intermediate values were inferred for daychicks.
Correlations of mechanical properties and dental microwear textures analysis
For the M1, no significant correlation was found between any DMTA and mean mechanical properties parameter (Supplementary Table 8). When considering maximum values for each mechanical property, several significant correlations were found with DMTA parameters. Maximum Young’s modulus was significantly correlated with the volume parameters Vv (void volume) and Vvc (void volume of the core) (Figure 4; Supplementary Table 8). Maximum TPA hardness was significantly correlated with 16 DMTA parameters representing height, complexity, volume, and area parameters (Figure 4; Supplementary Table 8). There were no significant correlations between any DMTA parameter for M1 and yield stress.
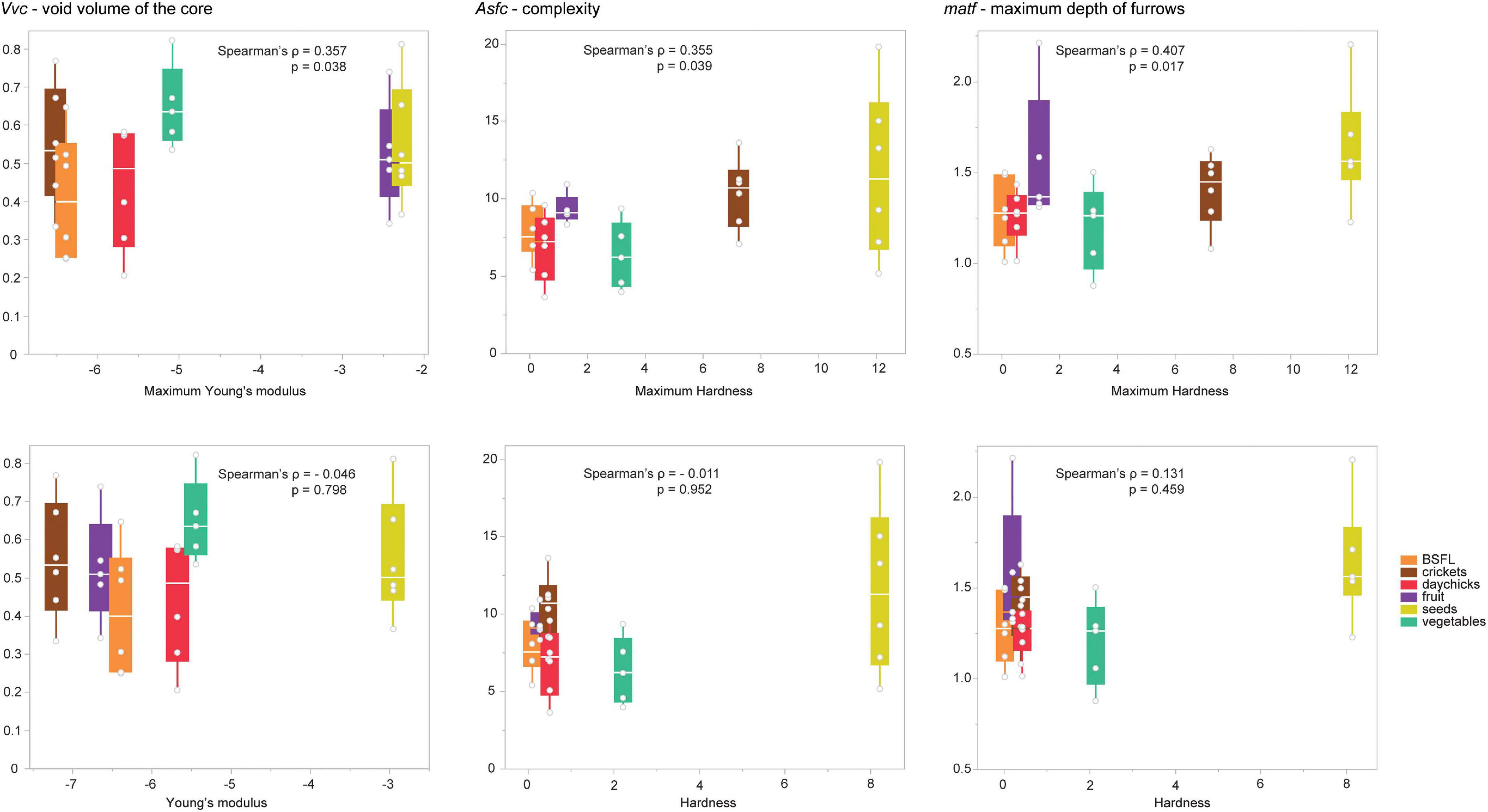
Figure 4. Correlations of selected DMTA parameters were obtained from the first upper molar (M1) of all rat diet groups with Young’s modulus and TPA hardness. The upper row shows a correlation with the maximum Young’s modulus and maximum TPA hardness per diet, and the lower row shows a correlation with the mean Young’s modulus and the mean TPA Hardness per diet. Note that correlations are stronger and significant for maximum mechanical properties (upper row) only. Young’s modulus is shown log-transformed for better visualization. BSFL, black soldier fly larvae.
For the M2, mean TPA hardness and maximum TPA hardness showed multiple significant correlations (17 and 20, respectively) with several height, volume, slope, and complexity parameters (Figure 5; Supplementary Table 9). Mean yield stress showed no significant correlations, while for maximum yield stress, 21 DMTA parameters showed significant correlations (Figure 5; Supplementary Table 9). There were no significant correlations of DMTA parameters with mean Young’s modulus, and only one parameter (IsT − texture isotropy) was significantly correlated with maximum Young’s modulus (Supplementary Table 8).
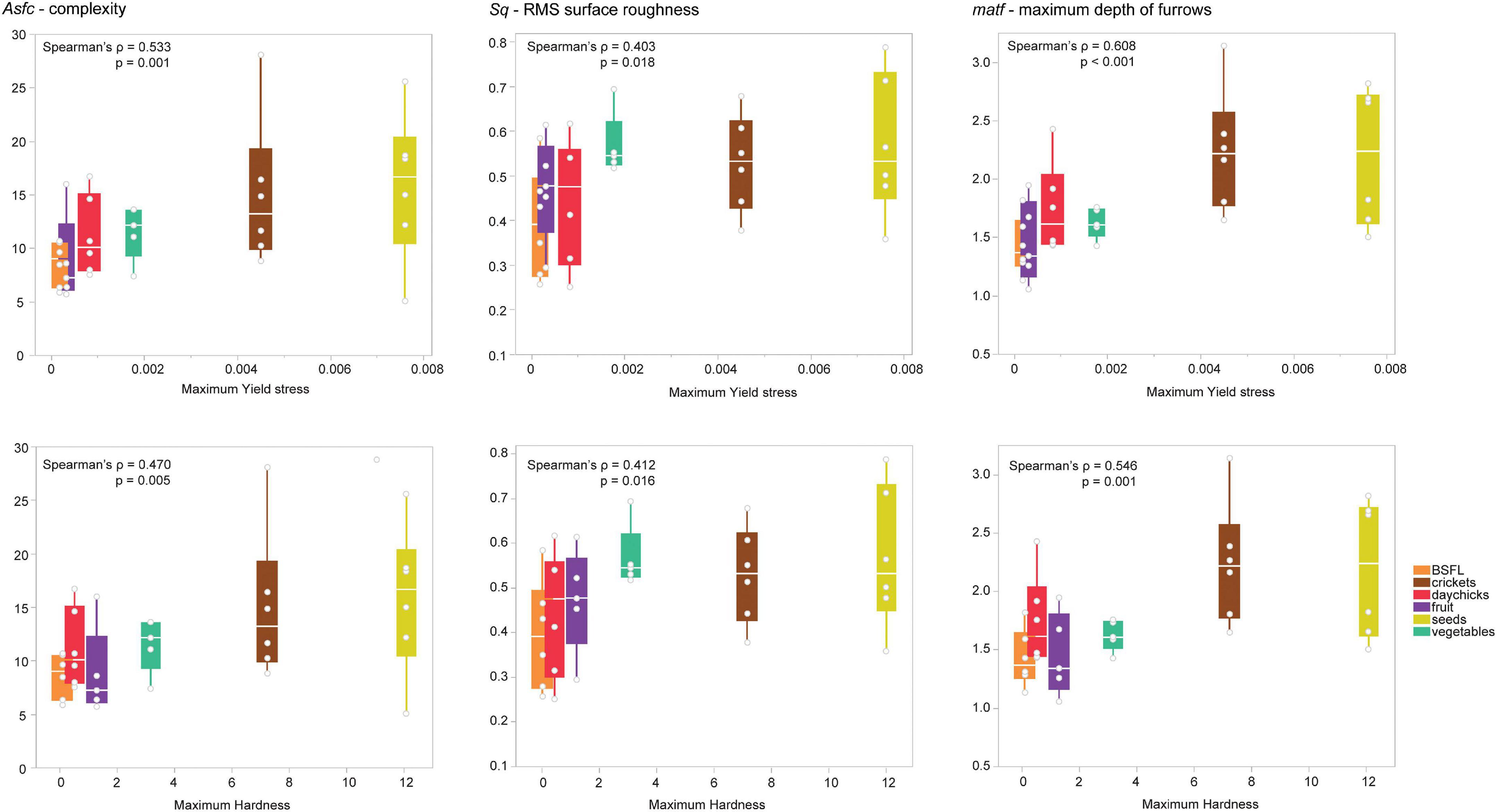
Figure 5. Correlations of selected DMTA parameters were obtained from the second upper molar (M2) of all rat diet groups for maximum yield stress and maximum TPA hardness. The upper row shows a correlation with maximum yield stress per diet, and the lower row shows a correlation with maximum TPA hardness per diet. BSFL, black soldier fly larvae.
Discussion
Limitations of the experimental setup
We have to note that several circumstances of the experimental setup likely introduced a source of variability and uncertainty. Therefore, all results obtained have to be interpreted with caution. Firstly, rats received diets while housed in groups of 3 in bulk one time per day. Hence, for the mixed diets (vegetable mix, fruit mix, seed mix), individual rats were free to choose among the diet items offered. We could not control if each individual consumed equal amounts of each dietary component, as either dietary preferences or dominance of other individuals may have affected food choice. This is likely a source of the observed variability in the DMTA data and may have influenced clearer separation between diet groups. We thus suggest that future feeding experiments should focus on one food item, or more homogenous food mixes, instead of trying to create a more natural diet mix.
Second, the components of the vegetable and fruit mix were purchased on a day-to-day basis, over the course of 37 days, to provide fresh food every day. The source of vegetables and fruits may have differed, as well as their degree of ripeness, water content, or other parameters, which might have added variability to the mechanical properties of these diets and could have influenced dental wear. The measurement of mechanical properties took place after the feeding experiment, hence—except for the seed mix—not on the same batches of food that the rats received. The seed mix was stored in a cool, dry place after the experiment and used for mechanical properties testing. Still, the storage time could potentially have altered the water content of the kernels to some degree (even though it was initially low). We purchased the same dietary items the rats received and treated them the same way (buying frozen crickets, black soldier fly larvae, and daychicks and defrosting them before testing). Still, the mechanical properties obtained may slightly differ from the mechanical properties of the original experimental diets but should nevertheless provide a good approximation of these food items.
Third, we excluded parsley and spinach from the mechanical properties testing, because their geometric shape does not allow for compression tests. Additionally, for daychicks and the seed mix, not all mechanical property parameters could be obtained but had to be inferred from linear regression equations.
Finally, choosing parameters for mechanical properties testing is similar to opening Pandora’s box, as numerous methods for items with different structural properties (homogenous vs. inhomogeneous, brittle, tough, elastic, etc.) and an equally large number of opinions on which are the best practices exist [see Berthaume (2016) for a review]. The selection of testing methods and parameters presented in our study followed two principles: simplicity and feasibility. By only conducting compression tests, we limited our obtained parameters to Young’s modulus and yield stress, hereby omitting other often measured parameters like energy release rate or toughness (e.g., Strait and Vincent, 1998). Our setup required only a force gauge, stiff testers, and measurement of the sample area and can hence be easily repeated. Regarding hardness testing, several methods are available but require sophisticated equipment such as diamond indenters and thin sections of the material (Vickers and Knoop hardness). Still, it has been questioned whether the observed hardness is an intrinsic mechanical property of the material or a product of the local testing environment, as argued by Berthaume (2016). We did not wish to engage in this discussion but did hope to simply find a hardness measure that was obtainable with our setup. Therefore, we chose TPA hardness. Parameters from food texture studies (TPA) have been subjected to criticism because calculations of these parameters are often not consistently applied by researchers or details of the testing setup are insufficiently reported (e.g., size of the tester, test speed). However, TPA hardness has been evaluated as “probably the most reliable TPA parameter” by Nishinari et al. (2019). The general pitfall is that TPA intends to mimic human masticatory behavior using an instrument and compress (indenting) a food sample two times and that this measure only works for elastic foods. For hard and brittle items, such as the seeds in our experiment, TPA hardness thus had to be inferred, which introduced further uncertainty. This setup is an oversimplification of the actual masticatory process. In the case of our study, we did not intend to mimic masticatory behavior of the rats but to gain quantitative results related to the diet’s intrinsic physical properties. The interaction between tooth morphology, bite force, mastication behavior (movement), lubrication of the food through saliva, and many more aspects cannot be accounted for in our approach but will all affect the observed tooth (micro)wear (Schulz-Kornas et al., 2020b). Therefore, our characterization of diet mechanical properties is not extensive but can still highlight several correlations between observed DMTs and diet mechanical properties.
Tooth position-specific dental microwear texture
The controlled rat feeding experiment of various near-natural diets revealed several systematic dental wear patterns. Comparable to guinea pigs feeding on pelleted diets (Winkler et al., 2021), overall surface roughness, including depth of wear features, increased from M1 to M2 in rats (Figure 3; Supplementary Figure 2). The only exception is the fruit diet, which showed similar roughness values on M1 and M2. The most pronounced increase was seen for vegetables, seeds, crickets, and daychicks. Due to the variable magnitude of increase in surface roughness from M1 to M2, differences observed between the diet groups were more pronounced when only considering the M1 and weaker when considering only the M2. Overall, the number of significantly different DMTA parameters between tooth positions on the same diet is even larger than between diet groups for the same tooth position (Table 2). This finding highlights that DMTA is likely tooth-position-specific—an observation made before for ungulates (Schulz et al., 2010) and macropods (Arman et al., 2019)—and cautions against lumping tooth positions together to increase sample sizes (for example in fossil samples). It might be that, in small mammals, DMTA conducted on anterior cheek tooth positions better reflects dietary differences than when using posterior teeth. This observation is in agreement with Winkler et al. (2021), who found that the fourth premolar showed the best dietary discrimination in guinea pigs receiving natural plant diets of different abrasiveness.
When focusing on the M1, the six experimental diets can be roughly separated into three groups according to their resulting DMT patterns. However, differentiation between diets within these groups is difficult. Group one includes fruit mix, seed mix, and crickets, which are showing the highest surface complexity (Sdr, Asfc) and largest height differences (e.g., depth of furrows, peak height, mean surface roughness). These are the groups containing diet components that are often considered hard objects. Group two is composed of BSFL and daychicks, which had the lowest complexity, height, and volume parameter values. Black soldier fly larvae can be considered, in contrast to crickets, as soft-bodied insects, as they are not covered by a hard, sclerotized cuticle (Chapman, 2013a). Strait and Vincent (1998) tested the toughness of insects through scissor tests. They found beetles to be tough and brittle, while caterpillars were soft and compliant. We would suggest that crickets might be more comparable to beetles, while BSFL bear more resemblance to caterpillars (Evans and Sanson, 2005).
Finally, the vegetable mix diet would fall into group three, which is characterized by intermediate height and volume parameter values, but has distinctly higher anisotropy (new epLsar, Sfrax epLsar) and mean density of furrows. In herbivorous ungulates, high anisotropy values are characteristic of grazing species (Ungar et al., 2007), while in guinea pigs, the differences between browse and grass diets were less pronounced (Winkler et al., 2021). For rats, it seems that mastication of the vegetables required a different mastication mode as compared to the other diets. We believe that the more fibrous vegetables may require more frequent shearing motions, while diets such as fruit, seeds, and insects may require crushing movements. The stronger alignment (anisotropy) and higher density of wear features would hence result from such a repeated shearing motion.
What causes high complexity?
Patterns of complex surfaces have been associated with hard-object feeding not only in primates (Scott et al., 2005, 2012; Ungar et al., 2008, 2010; Ungar and Berger, 2018) and carnivorous mammals (DeSantis et al., 2012), but also in lepidosaurs (Winkler et al., 2019b), archosaurs (Bestwick et al., 2019), and fish (Purnell and Darras, 2015). Rats are no exception, as they also display the highest surface complexity (both by Asfc and Sdr) on the hardest diet (seeds) after feeding on their designated diet for 37 consecutive days. Yet, how complexity develops over longer feeding periods cannot be predicted. van Casteren et al. (2020) argued that “occasional contacts between seeds and irregular spicules of enamel could result in the latter being fractured, but over time this process should result in a decrease, rather than increase, in texture complexity.” We cannot rule out that our experimental animals would have displayed a change in complexity values over time. However, from the current viewpoint of DMTA, it is likely that texture turnover in (small) mammals is fast (Winkler et al., 2020b) and continuously feeding on the same diet will produce similar DMTA over and over again, thus resulting in a short-term dietary proxy that reflects the last few weeks of an individual’s diet. We therefore conclude that the connection between high surface complexity and hard-object feeding is independent of body size, chewing mode, and taxonomic affinity [a similar conclusion was reached by Purnell and Darras (2015) for fishes], and thus a universal wear pattern following the oral processing of stress-limited foodstuffs.
However, we note that our hardness measure yielded low values for crickets, which resulted in the second highest surface complexity observed in this experiment. Overall, the mechanical properties of the two insect diets (crickets, BSFL) were found to be similar when testing whole insects, while the DMT pattern diverged completely. Cricket feeding resulted in high complexity, surface roughness, and deep and voluminous wear features, while BSFL feeding resulted in the lowest complexity, surface roughness, and lowest depth of wear features observed among all diet groups. For insectivorous bats, Purnell et al. (2013) found that species consuming “harder” prey (e.g., coleoptera) showed larger volume parameter values than species consuming “softer” prey, which is consistent with the disparity in our results observed between supposedly harder crickets and softer BSFL. It is therefore evident that our approach toward measuring mechanical properties of foodstuffs is not sufficient to describe the essential differences between these two insect diets. When testing individual cricket body parts, the head capsule displayed much higher TPA hardness than the abdomen, and a part of the digestive system (the gizzard) showed mechanical properties comparable to seeds (see raw data, Supplementary Tables 2–4). The gizzard is lined with strong cuticular plates or “teeth” in house crickets and other Orthoptera (Kirby et al., 1982 and references within, Elzinga, 1996; Chapman, 2013b). When considering maximum yield stress and maximum TPA hardness values for crickets (that were derived from regression equations for the gizzards), we found strong correlations with a variety of DMTA parameters. Although the hardness data were inferred from the regression equation, and the extrapolated values were mostly outside of the range of the other measurements (see Statistics section), these results suggest that crickets are much more heterogenous as compared to BSFL, and likely, their sclerotized body parts greatly exceed less sclerotized body parts (like the abdomen) in hardness. Also, Young’s modulus of individual body parts has been found to differ greatly in locusts (over seven orders of magnitude, Li et al., 2020). Such subtle differences were not well-captured by our experimental approach but are likely responsible for the pronounced differences in DMT observed between crickets and BSFL.
Dental microwear texture and mechanical properties
Maximum values of mechanical properties have a stronger impact on the DMTA of M1. We found maximum Young’s modulus to be significantly correlated with 2 DMTA parameters, while mean Young’s modulus was not significantly correlated with any DMTA parameter (Supplementary Table 8). For maximum TPA hardness, M1 showed 16 significant correlations, while mean TPA hardness was not significantly correlated with DMTA parameters at all (Supplementary Table 8). M2 showed more significant correlations between material properties parameters and DMTA than M1. Here, maximum yield stress and maximum TPA hardness showed abundant strong correlations with DMTA parameters, and mean TPA hardness was significantly correlated with 17 DMTA parameters (Supplementary Table 9). In conclusion, differences in (maximum and mean) Young’s modulus between diet items did not explain differences observed in DMTA, while maximum TPA hardness was strongly correlated with DMTA on both M1 and M2. For the M2, also maximum yield stress and mean TPA hardness were equally well-correlated with DMTA parameters, which may result from different chewing behavior when processing harder items. If harder items would be primarily processed using the M2, the observed wear might be more correlated with yield stress, which determines the fracture point of the diet item and overall hardness. Although our methodology has several limitations, this general trend highlights that mechanical properties and tooth position-specific wear are interrelated.
Parameters associated with increased abrasion such as height, volume, and complexity parameters increased from M1 to M2 on most diets. The stronger correlation of maximum mechanical properties indicates that M1 dental microwear may be more sensitive toward detecting inhomogeneous diets, more singular feeding events, and thus potentially fallback feeding events (Lambert et al., 2004). The M2, on the other hand, is strongly affected by overall TPA hardness and maximum TPA hardness of the diet. This may indicate that stiff, resistant food items (commonly termed hard objects) are more frequently and thoroughly processed using the M2 (and hence in the rear of the dentition). This is in accordance with observations from guinea pigs (Winkler et al., 2021), for which it was suggested that increased bite force toward the rear of the dentition results in increased abrasive wear when feeding on hard pellets. Under the previously discussed limitations, our results here need to be treated with caution, but in the context of the increased wear on posterior teeth in guinea pigs, it is plausible that rats also exert stronger bite forces toward the rear of the dentition and utilize the posterior teeth to break harder foodstuff.
Outlook and conclusion
Different foodstuffs can likely cause high complexity of DMT; however, in our controlled feeding experiment seeds, fruit (with seeds) and harder insect body parts resulted in the highest recorded complexity. The findings of this study emphasize that DMT formation in small mammals (i.e., rodents) seems to be strongly connected to mastication behavior and mechanics (likely bite force) and is hence significantly different for different tooth positions. These results call for a more thorough investigation of DMT gradients along the tooth row in other taxa, including large mammals.
We attempted to associate quantitative food mechanical properties with quantitative DMT. We considered this approach relevant to better understand the interrelation between ingesta and observed dental (micro)wear, beyond the detection of differences between diet categories. As noted, the masticatory system is highly complex, and accounting for all possible factors that affect DMT formation is impossible in an experimental setup. However, we can approach this complex system by controlling isolated factors and quantifying them. Future research may further elucidate how food mechanical properties affect dental (micro)wear, but also manage to take into account different occlusal morphologies and chewing mechanics, including movement, speed, bite force, fluid dynamics on the occlusal surface, and many more. At the moment, we are still only scratching the surface of understanding dental wear.
Data availability statement
The dataset presented in this study is permanently stored in the following online repository: ZFDM online repository of the University of Hamburg, doi.org/10.25592/uhhfdm.10235.
Ethics statement
The animal study was reviewed and approved by Swiss Cantonal Animal Care and Use Committee Zurich animal experiment license No. ZH135/16.
Author contributions
DEW, MC, MOK, ES-K, and TT: conceptualization. DEW, MC, MOK, and ADC: methodology. DEW: formal analysis and visualization. MOK, TMK, and AT: resources. DEW, MC, and MOK: writing—original draft. TT, DEW, and TK: funding acquisition. All authors: writing—review and editing and approve the submitted version.
Funding
This project received funding from the European Research Council (ERC) under the European Union’s Horizon 2020 Research and Innovation Programme (Grant Agreement 681450) (ERC Consolidator Grant Agreement to TT) and from the Japan Society for the Promotion of Science (JSPS) under Postdoctoral fellowship awarded to DEW (KAKENHI Grant No. 20F20325) and TK (KAKENHI Grant No. 19J40003).
Acknowledgments
We thank Louise Martin, Nicole Schmid, Sandra Heldstab, and Kathrin Zbinden (from the University of Zurich) for support with animal husbandry, and three reviewers for their helpful comments on the manuscript. We further thank Professor Shinji Nagata (University of Tokyo) for the helpful discussion about potentially mechanically challenging body parts of crickets, including the gizzard.
Conflict of interest
Author AT was employed by Granovit Zoofeed, Granovit AG.
The remaining authors declare that the research was conducted in the absence of any commercial or financial relationships that could be construed as a potential conflict of interest.
Publisher’s note
All claims expressed in this article are solely those of the authors and do not necessarily represent those of their affiliated organizations, or those of the publisher, the editors and the reviewers. Any product that may be evaluated in this article, or claim that may be made by its manufacturer, is not guaranteed or endorsed by the publisher.
Supplementary material
The Supplementary Material for this article can be found online at: https://www.frontiersin.org/articles/10.3389/fevo.2022.957427/full#supplementary-material
References
Alba, D. M., Fortuny, J., and Moyà-Solà, S. (2010). Enamel thickness in the Middle Miocene great apes Anoiapithecus, Pierolapithecus and Dryopithecus. Proc. R. Soc. B Biol. Sci. 277, 2237–2245. doi: 10.1098/rspb.2010.0218
Arman, S. D., Prowse, T. A. A., Couzens, A. M. C., Ungar, P. S., and Prideaux, G. J. (2019). Incorporating intraspecific variation into dental microwear texture analysis. J. R. Soc. Interface 16:20180957. doi: 10.1098/rsif.2018.0957
Berthaume, M. A. (2016). Food mechanical properties and dietary ecology. Am. J. Phys. Anthropol. 159, 79–104. doi: 10.1002/ajpa.22903
Bestwick, J., Unwin, D. M., and Purnell, M. A. (2019). Dietary differences in archosaur and lepidosaur reptiles revealed by dental microwear textural analysis. Sci. Rep. 9:11691. doi: 10.1038/s41598-019-48154-9
Bourne, M. C. (2002). Food texture and viscosity: Concept and measurement, 2nd Edn. San Diego, CA: Academic Press, 182–186.
Calandra, I., and Merceron, G. (2016). Dental microwear texture analysis in mammalian ecology. Mammal Rev. 46, 215–228. doi: 10.1111/mam.12063
Chapman, R. F. (2013a). “The integument, gas exchange and homeostasis,” in The insects: Structure and function, 5th Edn, eds S. J. Simpson and A. E. Douglas (Cambridge: Cambridge University Press), 463–498.
Chapman, R. F. (2013b). “Alimentary canal, digestion and absorption,” in The insects: Structure and function, 5th Edn, eds S. J. Simpson and A. E. Douglas (Cambridge: Cambridge University Press), 46–80. doi: 10.1017/CBO9781139035460.006
Constantino, P. J., Borrero-Lopez, O., and Lawn, B. R. (2018). Mechanisms of tooth damage and Paranthropus dietary reconstruction. Biosurf. Biotribol. 4, 73–78. doi: 10.1049/bsbt.2018.0017
Constantino, P. J., Lee, J. J. W., Morris, D., Lucas, P. W., Hartstone-Rose, A., Lee, W. K., et al. (2011). Adaptation to hard-object feeding in sea otters and hominins. J. Hum. Evol. 61, 89–96. doi: 10.1016/j.jhevol.2011.02.009
Daegling, D. J., McGraw, W. S., Ungar, P. S., Pampush, J. D., Vick, A. E., and Bitty, E. A. (2011). Hard-object feeding in sooty mangabeys (Cercocebus atys) and interpretation of early hominin feeding ecology. PLoS One 6:e23095. doi: 10.1371/journal.pone.0023095
Dammaschke, T. (2010). Rat molar teeth as a study model for direct pulp capping research in dentistry. Lab. Anim. 44, 1–6. doi: 10.1258/la.2009.008120
DeSantis, L. R., Schubert, B. W., Scott, J. R., and Ungar, P. S. (2012). Implications of diet for the extinction of saber-toothed cats and American lions. PLoS One 7:e52453. doi: 10.1371/journal.pone.0052453
Dumont, E. R. (1995). Enamel thickness and dietary adaptation among extant primates and chiropterans. J. Mammal. 76, 1127–1136. doi: 10.2307/1382604
Elzinga, R. J. (1996). A comparative study of microspines in the alimentary canal of five families of Orthoptera (Saltatoria). Int. J. Insect Morphol. Embryol. 25, 249–260. doi: 10.1016/0020-7322(96)00007-4
Evans, A. R., and Sanson, G. D. (2005). Biomechanical properties of insects in relation to insectivory: Cuticle thickness as an indicator of insect ‘hardness’ and ‘intractability’. Aust. J. Zool. 53, 9–19. doi: 10.1071/ZO04018
Frei, J., Clauss, M., Winkler, D. E., Tütken, T., and Martin, L. F. (2021). Use of running plates by floor housed rats: A pilot study. Lab. Anim. 55, 521–530. doi: 10.1177/00236772211036572
Grine, F. E., and Kay, R. F. (1988). Early hominid diets from quantitative image analysis of dental microwear. Nature 333, 765–768. doi: 10.1038/333765a0
Kay, R. F. (1981). The nut-crackers—a new theory of the adaptations of the Ramapithecinae. Am. J. Phys. Anthropol. 55, 141–151. doi: 10.1002/ajpa.1330550202
Kirby, P., Beck, R., and Clarke, K. U. (1982). Undescribed anatomical features of the gut of the house cricket, Acheta domesticus L. (Insecta, Orthoptera). J. Nat. Hist. 16, 707–714. doi: 10.1080/00222938200770541
Lambert, J. E., Chapman, C. A., Wrangham, R. W., and Conklin-Brittain, N. L. (2004). Hardness of cercopithecine foods: Implications for the critical function of enamel thickness in exploiting fallback foods. Am. J. Phys. Anthropol. 125, 363–368. doi: 10.1002/ajpa.10403
Lee-Thorp, J. (2011). The demise of “Nutcracker Man”. Proc. Natl. Acad. Sci. U.S.A. 108, 9319–9320. doi: 10.1073/pnas.1105808108
Li, C., Gorb, S. N., and Rajabi, H. (2020). Cuticle sclerotization determines the difference between the elastic moduli of locust tibiae. Acta Biomater. 103, 189–195. doi: 10.1016/j.actbio.2019.12.013
Louail, M., Ferchaud, S., Souron, A., Walker, A. E., and Merceron, G. (2021). Dental microwear textures differ in pigs with overall similar diets but fed with different seeds. Palaeogeogr. Palaeoclimatol. Palaeoecol. 572:110415. doi: 10.1016/j.palaeo.2021.110415
Lucas, P. W., Constantino, P. J., Chalk, J., Ziscovici, C., Wright, B. W., Fragaszy, D. M., et al. (2009). Indentation as a technique to assess the mechanical properties of fallback foods. Am. J. Phys. Anthropol. 140, 643–652. doi: 10.1002/ajpa.21026
Martin, L. B., Olejniczak, A. J., and Maas, M. C. (2003). Enamel thickness and microstructure in pitheciin primates, with comments on dietary adaptations of the middle Miocene hominoid Kenyapithecus. J. Hum. Evol. 45, 351–367. doi: 10.1016/j.jhevol.2003.08.005
Merceron, G., Ramdarshan, A., Blondel, C., Boisserie, J. R., Brunetiere, N., Francisco, A., et al. (2016). Untangling the environmental from the dietary: Dust does not matter. Proc. R. Soc. B Biol. Sci. 283:20161032. doi: 10.1098/rspb.2016.1032
Merceron, G., Blondel, C., Viriot, L., Koufos, G. D., and de Bonis, L. (2007a). Dental microwear analysis of bovids from the Vallesian (late Miocene) of Axios Valley in Greece: Reconstruction of the habitat of Ouranopithecus macedoniensis (Primates, Hominoidea). Geodiversitas 29, 421–433.
Merceron, G., Schulz, E., Kordos, L., and Kaiser, T. M. (2007b). Paleoenvironment of Dryopithecus brancoi at Rudabánya, Hungary: Evidence from dental meso- and micro-wear analyses of large vegetarian mammals. J. Hum. Evol. 53, 331–349. doi: 10.1016/j.jhevol.2007.04.008
Nishijima, K., Kuwahara, S., Ohno, T., Miyaishi, O., Ito, Y., Sumi, Y., et al. (2007). Occlusal tooth wear in female F344/N rats with aging. Arch. Oral Biol. 52, 844–849. doi: 10.1016/j.archoralbio.2007.03.001
Nishijima, K., Kuwahara, S., Ohno, T., Miyaishi, O., Ito, Y., Sumi, Y., et al. (2009). Occlusal tooth wear in male F344/N rats with aging. Arch. Gerontol. Geriatr. 48, 178–181. doi: 10.1016/j.archger.2008.01.006
Nishinari, K., Fang, Y., and Rosenthal, A. (2019). Human oral processing and texture profile analysis parameters: Bridging the gap between the sensory evaluation and the instrumental measurements. J. Texture Stud. 50, 369–380. doi: 10.1111/jtxs.12404
Ohba, Y. (1974). Form of the molar crown with especial reference to tooth wear, and occlusion in rats. Aichi Gakuin Daigaku Shigakkai Shi 12, 171–192.
Purnell, M. A., Crumpton, N., Gill, P. G., Jones, G., and Rayfield, E. J. (2013). Within-guild dietary discrimination from 3-D textural analysis of tooth microwear in insectivorous mammals. J. Zool. 291, 249–257. doi: 10.1111/jzo.12068
Purnell, M. A., and Darras, L. P. (2015). 3D tooth microwear texture analysis in fishes as a test of dietary hypotheses of durophagy. Surf. Topogr. 4:014006. doi: 10.1088/2051-672X/4/1/014006
Rivals, F., Schulz, E., and Kaiser, T. M. (2008). Climate-related dietary diversity of the ungulate faunas from the middle Pleistocene succession (OIS 14–12) at the Caune de l’Arago (France). Paleobiology 34, 117–127. doi: 10.1666/07023.1
Rivals, F., Solounias, N., and Mihlbachler, M. C. (2007). Evidence for geographic variation in the diets of late Pleistocene and early Holocene Bison in North America, and differences from the diets of recent Bison. Quat. Res. 68, 338–346. doi: 10.1016/j.yqres.2007.07.012
Schulz, E., Calandra, I., and Kaiser, T. M. (2010). Applying tribology to teeth of hoofed mammals. Scanning 32, 162–182. doi: 10.1002/sca.20181
Schulz, E., Calandra, I., and Kaiser, T. M. (2013). Feeding ecology and chewing mechanics in hoofed mammals: 3D tribology of enamel wear. Wear 300, 169–179. doi: 10.1016/j.wear.2013.01.115
Schulz-Kornas, E., Winkler, D. E., Clauss, M., Carlsson, J., Ackermans, N. L., Martin, L. F., et al. (2020a). Everything matters: Molar microwear texture in goats (Capra aegagrus hircus) fed diets of different abrasiveness. Palaeogeogr. Palaeoclimatol. Palaeoecol. 552:109783. doi: 10.1016/j.palaeo.2020.109783
Schulz-Kornas, E., Kaiser, T. M., Calandra, I., and Winkler, D. E. (2020b). “A brief history of quantitative wear analyses with an appeal for a holistic view on dental wear processes,” in Mammalian teeth – form and function, eds T. Martin and W. von Koenigswald (Munich: Verlag Dr. Friedrich Pfeil), 44–53.
Schwartz, G. T., McGrosky, A., and Strait, D. S. (2020). Fracture mechanics, enamel thickness and the evolution of molar form in hominins. Biol. Lett. 16:20190671. doi: 10.1098/rsbl.2019.0671
Scott, R., Ungar, P., Bergstrom, T. S., Brown, C. A., Grine, F. E., Teaford, M. F., et al. (2005). Dental microwear texture analysis shows within-species diet variability in fossil hominins. Nature 436, 693–695. doi: 10.1038/nature03822
Scott, R. S., Teaford, M. F., and Ungar, P. S. (2012). Dental microwear texture and anthropoid diets. Am. J. Phys. Anthropol. 147, 551–579. doi: 10.1002/ajpa.22007
Semprebon, G. M., and Rivals, F. (2007). Was grass more prevalent in the pronghorn past? An assessment of the dietary adaptations of Miocene to recent Antilocapridae (Mammalia: Artiodactyla). Palaeogeogr. Palaeoclimatol. Palaeoecol. 253, 332–347. doi: 10.1016/j.palaeo.2007.06.006
Shellis, R. P., Beynon, A. D., Reid, D. J., and Hiiemae, K. M. (1998). Variations in molar enamel thickness among primates. J. Hum. Evol. 35, 507–522. doi: 10.1006/jhev.1998.0238
Sponheimer, M., Daegling, D. J., Ungar, P. S., Bobe, R., and Paine, O. C. (2022). Problems with Paranthropus. Quat. Int. doi: 10.1016/j.quaint.2022.03.024 [Epub ahead of print].
Strait, S. G., and Vincent, J. F. V. (1998). Primate faunivores: Physical properties of prey items. Int. J. Primatol. 19, 867–878. doi: 10.1023/A:1020397430482
Teaford, M. F., and Ungar, P. S. (2000). Diet and the evolution of the earliest human ancestors. Proc. Natl. Acad. Sci. U.S.A. 97, 13506–13511. doi: 10.1073/pnas.260368897
Teaford, M. F., Ungar, P. S., Taylor, A. B., Ross, C. F., and Vinyard, C. J. (2020). The dental microwear of hard–object feeding in laboratory Sapajus apella and its implications for dental microwear formation. Am. J. Phys. Anthropol. 171, 439–455. doi: 10.1002/ajpa.24000
Thompson, C. L., Valença-Montenegro, M. M., Melo, L. D. O., Valle, Y. M., Oliveira, M. D., Lucas, P. W., et al. (2014). Accessing foods can exert multiple distinct, and potentially competing, selective pressures on feeding in common marmoset monkeys. J. Zool. 294, 161–169. doi: 10.1111/jzo.12164
Ungar, P. S. (2011). Dental evidence for the diets of Plio-Pleistocene hominins. Am. J. Phys. Anthropol. 146, 47–62. doi: 10.1002/ajpa.21610
Ungar, P. S. (2012). Dental evidence for the reconstruction of diet in African early Homo. Curr. Anthropol. 53, 318–329. doi: 10.1086/666700
Ungar, P. S., and Berger, L. R. (2018). Brief communication: Dental microwear and diet of Homo naledi. Am. J. Phys. Anthropol. 166, 228–235. doi: 10.1002/ajpa.23418
Ungar, P. S., Grine, F. E., and Teaford, M. F. (2008). Dental microwear and diet of the Plio-Pleistocene hominin Paranthropus boisei. PLoS One 3:e2044. doi: 10.1371/journal.pone.0002044
Ungar, P. S., Merceron, G., and Scott, R. S. (2007). Dental microwear texture analysis of Varswater bovids and early Pliocene paleoenvironments of Langebaanweg, Western Cape Province, South Africa. J. Mamm. Evol. 14, 163–181. doi: 10.1007/s10914-007-9050-x
Ungar, P. S., Scott, R. S., Grine, F. E., and Teaford, M. F. (2010). Molar microwear textures and the diets of Australopithecus anamensis and Australopithecus afarensis. Philos. Trans. R. Soc. B Biol. Sci. 365, 3345–3354. doi: 10.1098/rstb.2010.0033
Ungar, P. S., and Sponheimer, M. (2011). The diets of early hominins. Science 334, 190–193. doi: 10.1126/science.1207701
van Casteren, A., Strait, D. S., Swain, M. V., Michael, S., Thai, L. A., Philip, S. M., et al. (2020). Hard plant tissues do not contribute meaningfully to dental microwear: Evolutionary implications. Sci. Rep. 10:582. doi: 10.1038/s41598-019-57403-w
van der Merwe, N. J., Masao, F. T., and Bamford, M. K. (2008). Isotopic evidence for contrasting diets of early hominins Homo habilis and Australopithecus boisei of Tanzania. S. Afr. J. Sci. 104, 153–155.
Walker, A. (1981). Diet and teeth. Dietary hypotheses and human evolution. Philos. Trans. R. Soc. Lond. B Biol. Sci. 292, 57–64. doi: 10.1098/rstb.1981.0013
Winkler, D. E., Andrianasolo, T. H., Andriamandimbiarisoa, L., Ganzhorn, J. U., Rakotondranary, S. J., Kaiser, T. M., et al. (2016). Tooth wear patterns in black rats (Rattus rattus) of Madagascar differ more in relation to human impact than to differences in natural habitats. Ecol. Evol. 6, 2205–2215. doi: 10.1002/ece3.2048
Winkler, D. E., Clauss, M., Rölle, M., Schulz-Kornas, E., Codron, D., Kaiser, T. M., et al. (2021). Dental microwear texture gradients in guinea pigs reveal that material properties of the diet affect chewing behaviour. J. Exp. Biol. 224:jeb242446. doi: 10.1242/jeb.242446
Winkler, D. E., Schulz-Kornas, E., Kaiser, T. M., De Cuyper, A., Clauss, M., and Tütken, T. (2019a). Forage silica and water content control dental surface texture in guinea pigs and provide implications for dietary reconstruction. Proc. Natl. Acad. Sci. U.S.A. 116, 1325–1330. doi: 10.1073/pnas.1814081116
Winkler, D. E., Schulz-Kornas, E., Kaiser, T. M., and Tütken, T. (2019b). Dental microwear texture reflects dietary tendencies in extant Lepidosauria despite their limited use of oral food processing. Proc. R. Soc. B 286:20190544. doi: 10.1098/rspb.2019.0544
Winkler, D. E., Tütken, T., Schulz-Kornas, E., Kaiser, T. M., Müller, J., Leichliter, J., et al. (2020a). Shape, size, and quantity of ingested external abrasives influence dental microwear texture formation in guinea pigs. Proc. Natl. Acad. Sci. U.S.A. 117, 22264–22273. doi: 10.1073/pnas.2008149117
Winkler, D. E., Schulz-Kornas, E., Kaiser, T. M., Codron, D., Leichliter, J., Hummel, J., et al. (2020b). The turnover of dental microwear texture: Testing the” last supper” effect in small mammals in a controlled feeding experiment. Palaeogeogr. Palaeoclimatol. Palaeoecol. 557:109930. doi: 10.1016/j.palaeo.2020.109930
Keywords: hard-object feeding, mechanical properties, dental wear, material properties, microwear
Citation: Winkler DE, Clauss M, Kubo MO, Schulz-Kornas E, Kaiser TM, Tschudin A, De Cuyper A, Kubo T and Tütken T (2022) Microwear textures associated with experimental near-natural diets suggest that seeds and hard insect body parts cause high enamel surface complexity in small mammals. Front. Ecol. Evol. 10:957427. doi: 10.3389/fevo.2022.957427
Received: 31 May 2022; Accepted: 01 September 2022;
Published: 27 September 2022.
Edited by:
Ferran Estebaranz-Sánchez, Milá y Fontanals Institution for Research in Humanities (CSIC), SpainReviewed by:
Mark Purnell, University of Leicester, United KingdomGina Marie Semprebon, Bay Path University, United States
Mark Teaford, Touro University California, United States
Copyright © 2022 Winkler, Clauss, Kubo, Schulz-Kornas, Kaiser, Tschudin, De Cuyper, Kubo and Tütken. This is an open-access article distributed under the terms of the Creative Commons Attribution License (CC BY). The use, distribution or reproduction in other forums is permitted, provided the original author(s) and the copyright owner(s) are credited and that the original publication in this journal is cited, in accordance with accepted academic practice. No use, distribution or reproduction is permitted which does not comply with these terms.
*Correspondence: Thomas Tütken, dHVldGtlbkB1bmktbWFpbnouZGU=
†ORCID: Daniela E. Winkler, orcid.org/0000-0001-7501-2506; Marcus Clauss, orcid.org/0000-0003-3841-6207; Mugino O. Kubo, orcid.org/0000-0002-7748-7377; Ellen Schulz-Kornas, orcid.org/0000-0003-1657-8256; Thomas M. Kaiser, orcid.org/0000-0002-8154-1751; Anja Tschudin, orcid.org/0000-0002-0495-3197; Annelies De Cuyper, orcid.org/0000-0003-2057-8283; Tai Kubo, orcid.org/0000-0003-3207-0399; Thomas Tütken, orcid.org/0000-0002-2590-8600