- 1Institute for Land and Water Management Research, Federal Agency for Water Management, Petzenkirchen, Austria
- 2WasserCluster Lunz—Biologische Station GmbH, Lunz am See, Austria
- 3The James Hutton Institute, Aberdeen, United Kingdom
- 4Lancaster Environment Centre, Lancaster University, Lancaster, United Kingdom
- 5Institute of Hydrobiology and Aquatic Ecosystem Management, University of Natural Resources and Life Sciences Vienna, Vienna, Austria
- 6Institute of Soil Research, University of Natural Resources and Life Sciences Vienna, Vienna, Austria
Vegetative filter strips (VFS) are best management practices with the primary aim of protecting surface waters from eutrophication resulting from excess nutrient inputs from agricultural sources. However, we argue that there is a substantial time and knowledge lag from the science underpinning VFS to policy and implementation. Focussing on phosphorus (P), we strive to introduce a holistic view on VFS that accounts for the whole functional soil volume, temporal and seasonal effects, the geospatial context, the climatic and physico-chemical basic conditions, and the intricate bio-geochemical processes that govern nutrient retention, transformation, and transport. Specifically, we suggest a step-wise approach to custom VFS designs that links and matches the incoming P from event to multi-annual timescales from the short- and mid-term processes of P retention in the effective soil volume and to the longer-term P retention and offtake coupled to the soil-vegetation system. An a priori assessment of the P export potential should be followed by bespoke VFS designs, in line with local conditions and socio-economic and ecological constraints. To cope with increasingly nutrient saturated or functionally insufficient VFS installed over the last decades, concepts and management strategies need to encompass the transition in understanding of VFS as simple nutrient containers to multifunctional buffer zones that have a complex inner life. We need to address these associated emerging challenges and integrate their implications more thoroughly into VFS research, monitoring, policy, and implementation than ever before. Only then we may get VFS that are effective, sustainable, and persistent.
Introduction
Vegetative filter strips (VFS) are a commonly prioritised best management practice for protecting surface waters from eutrophication resulting from excess nutrient inputs from agricultural sources (Schindler et al., 2016; Lind et al., 2019). Their implementation began in the early 20th century, initially as a means against sediment loss (e.g., Allison, 1935), later on as a barrier to edge-of-field nutrient export (e.g., Willrich and Boda, 1976; Vanderholm et al., 1979). Since their conception, the role of VFS has evolved: from a simple solution mitigating the inflow of various forms of agricultural pollutants to multifunctional buffer zones in which nutrients are not only retained but also altered through biological activity and geochemical processes (Roberts et al., 2012; Weihrauch and Opp, 2018). Coupled with this was the recognition that VFS are finite nutrient sinks that require management to remain effective (Cooper and Gilliam, 1987; Schauder and Auerswald, 1992) and an awareness that VFS can change from nutrient sinks to sources under certain circumstances—especially for phosphorus (P), e.g., due to a saturation of sorption sites (Stutter et al., 2009).
With this Perspective article, we call for an integration of recent developments in soil P research into VFS management science, notably that P pools are a continuum of solubility/bioavailability rather than discrete P fractions and the acknowledgement of high spatio-temporal dynamics and complexity in P biogeochemistry (Kruse et al., 2015; Weihrauch and Opp, 2018; Weihrauch, 2019). While some of the advances in soil science have been incorporated theoretically into VFS science and included in review papers (e.g., Carstensen et al., 2020; Prosser et al., 2020; Stutter et al., 2021), we argue that a substantial part of VFS research is based on outdated concepts, especially regarding studies that deal with retention effectivity or monitoring. Often such studies still focus on short term nutrient retention rather than a permanent continuous removal. Furthermore, many studies apply one-dimensional designs based only on VFS width rather than considering all three dimensions of the soil-vegetation space, ignoring important aspects related to nutrient dynamics and retention efficiency. Thus, a substantial share of the mainstream VFS research is lagging behind recent scientific developments. However, it is much more likely that monitoring or effectivity studies—rather than more difficult to comprehend theoretical research or complex modelling approaches—are used as the basis for the recommendations provided by agricultural authorities or agri-environmental funding programmes. Guidelines on VFS designs are often further simplified and only updated between funding periods. Thus, those responsible for the implementation of VFS receive little guidance on designing up-to-date VFS, which also means that many VFS are far less efficient than they could be.
Here, we describe these issues in detail and discuss why they need to be more deeply embedded into VFS management science (Figure 1). Our focus is on phosphorus; nevertheless, the presented core ideas also apply to other agricultural pollutants.
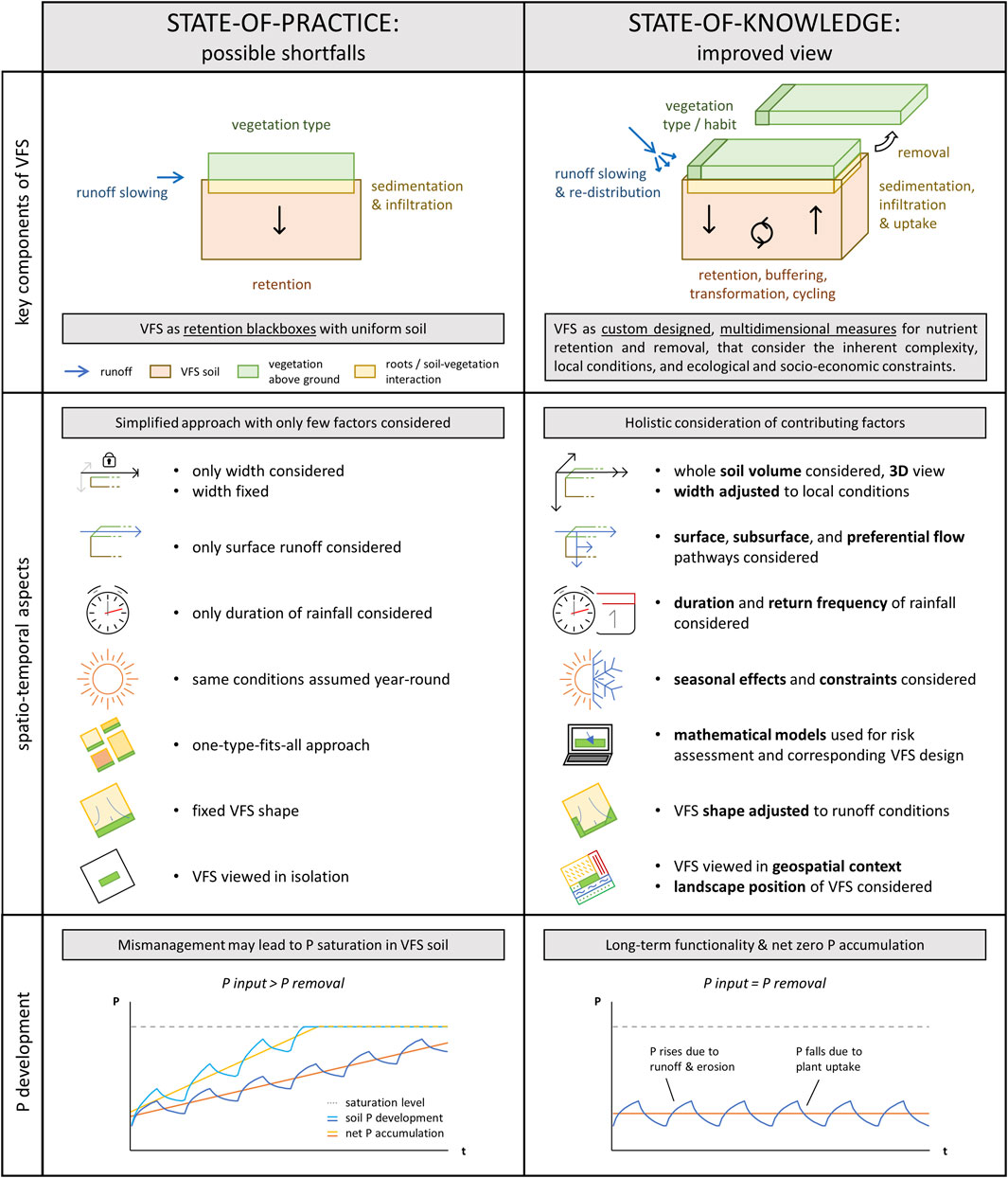
FIGURE 1. Schematic overview of possible shortfalls during VFS design and research (left column) and suggested improvements (right column), regarding the conceptualization (top panel), considered aspects (mid panel), and P development in the VFS soil (bottom panel; lighter colours depict P development without harvesting). Legends apply for both columns.
Principles of VFS Design
A Holistic Approach on the Functional Three-Dimensional VFS Space
Recent research has revealed a multitude of physical and bio-geochemical processes that modify and transform biotic and abiotic P forms between inputs and outputs (Stutter et al., 2009; Weihrauch and Opp, 2018). Depending on the soil properties and chemical gradients, P sorbs on and desorbs from soil particles, precipitates and dissolves, shifting between various forms and states along a solubility and bioavailability continuum. Furthermore, plants and microbes release enzymes that can degrade organic P into dissolved P fractions for uptake into both the vegetative pool or soil microbial biomass (Roberts et al., 2012; Weihrauch and Opp, 2018). In VFS, this cycling affects incoming P sources as well as native soil P. Consequently, P that leaves a VFS may differ substantially from the original P inputs in terms of chemical speciation, timing, or concentration (Figure 2).
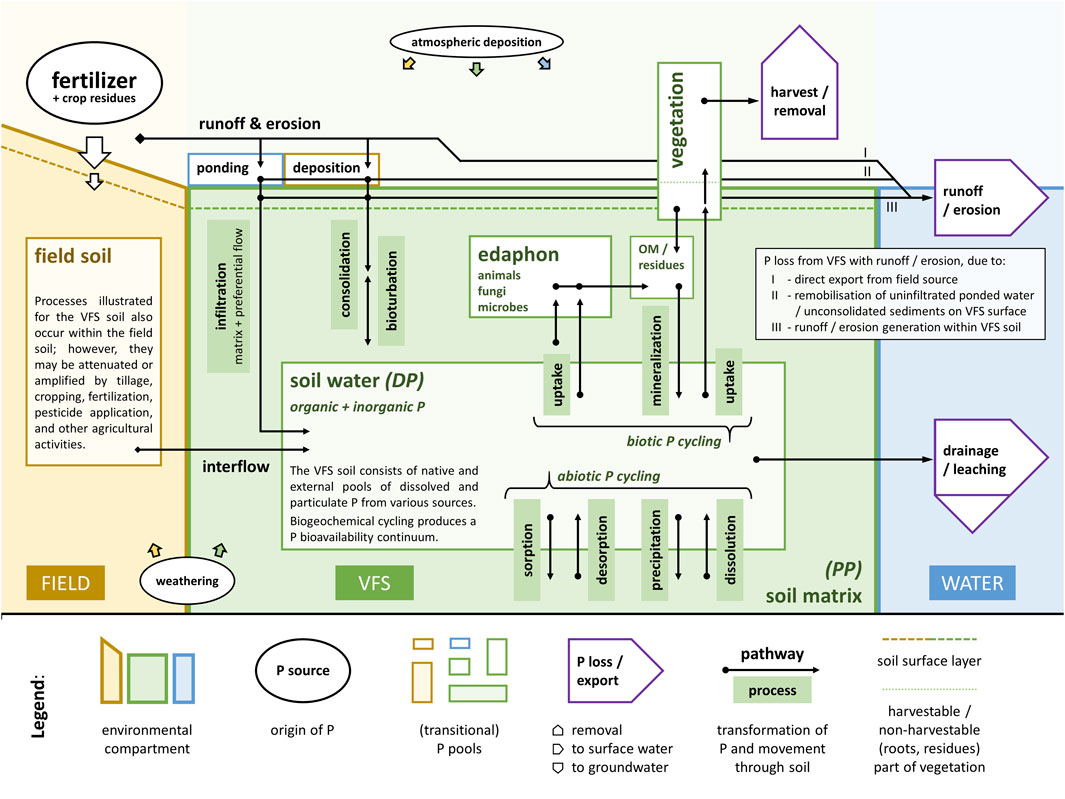
FIGURE 2. Diagram of the most important pathways and processes of phosphorus (P) dynamics that have to be considered in VFS design and research. DP–dissolved P; PP–particulate P; OM–organic matter.
The science behind VFS stems from various disciplines and is the basis of VFS design recommendations. Ideally, this is an iterative process in which studying and monitoring existing VFS increases our understanding of VFS functioning, which leads to improved designs, and in turn, more effective VFS, and so forth (McGonigle et al., 2012). From a practitioner’s view, a VFS may be regarded as a black box or as two separate compartments: one below (the soil) and one aboveground (the vegetation). In fact, the two compartments are closely interlinked and need to be considered and managed together. Belowground, the storage capacity of the soil for P can be substantial (Roy et al., 2017); however, it is finite. Consequently, relying on retention and storage to mitigate P pollution is unsustainable for long-term functioning. Furthermore, the risk of (dissolved) P loss increases with the degree of P saturation (DPS), which means that considerable amounts may leach from VFS soils as they become saturated (Pautler and Sims, 2000; Kleinman, 2017). Therefore, the DPS should be kept low and P that has already accumulated in the soil should be removed. By far the most practical option is via removal of the aboveground vegetative compartment, and with it the soil P that has been taken up by the plants. To prevent a built-up of soil P and to ensure a sufficient P removal by plant harvesting, we suggest a step-wise approach to customised VFS designs. These should consider the amount of incoming P from the agricultural system, the amount that can be temporarily retained in the soil, and the amount that can be removed by harvesting.
Estimating P Export Potential
Firstly, the source area for P that is supposed to be intercepted by a VFS has to be identified; in most cases, this would be the field(s) of the (sub-)watershed that drains into a VFS. This includes collection and collation of general field and soil characteristics (e.g., soil type, slope, area, topography), physico-chemical soil parameters (e.g., texture, density, carbon content, nutrient concentrations), and agronomic factors (e.g., cropping, tillage, fertilisation). These parameters determine erosion and runoff generation and are, thus, directly or indirectly linked to the potential nutrient export (Wang et al., 2014; Roberts and Israel, 2017).
Secondly, climate and weather conditions have to be assessed. As infiltration and runoff are related to soil water content, the prevailing rainfall characteristics (i.e., intensity, duration, return frequency) substantially impact runoff generation and the occurrence of erosive events (Ries et al., 2020; Tiefenbacher et al., 2021). Furthermore, seasonal aspects may lead to a mismatch of runoff occurrence and P retention/uptake potential of a VFS. In cold climates, much of the annual runoff occurs during snowmelt, at a time where soils are still frozen, severely limiting interaction and exchange processes necessary for P retention (Kieta et al., 2018; Vanrobaeys et al., 2019). Consequently, climate and seasonality have direct implications for VFS design. If most runoff is triggered by extreme events or occurs outside of the growing season, VFS may even be largely ineffective, requiring the implementation of additional countermeasures (e.g., retention ponds).
Taken together, the first two steps provide the data necessary to estimate how often and to what extent a field is expected to export sediment and water along with the amount of particulate (PP) and dissolved P (DP) that is transported with it. This should be further improved by using process-orientated models to calculate runoff and erosion characteristics (e.g., WEPP, Laflen et al., 1997; EUROSEM, Morgan et al., 1998) or nutrient pathways (e.g., PhosFate, Kovacs et al., 2008). The more reliably we can predict P exports in space and time, the better we may be able to design effective VFS.
Designing Custom VFS
In combination with the aforementioned examples, mathematical models can also aid in VFS design and the evaluation of VFS performance (e.g., VFSMOD, Muñoz-Carpena et al., 1999; REMM, Lowrance et al., 2000; SWAT, Arnold et al., 2012). Models are purpose-designed tools to get reasonable estimates of how much runoff, erosion, or nutrient loadings a VFS would have to handle, as well as where and when they most probably occur—making it possible to estimate the required VFS dimensions and to design bespoke countermeasures. The models may be constantly adapted to include important biogeochemical and mechanistic factors concerning flow, sedimentation, and nutrient pathways. However, despite their importance and long-time availability, they are rarely used outside academia. More user-friendly interfaces, together with easily comprehensible manuals/tutorials could help to improve their adoption. A drawback of current models is that they do not account for P accumulation in the VFS soil.
To prevent a build-up of nutrients, their input and offset must be balanced. Therefore, the nutrient removal potential of the vegetation needs to be an integral part of VFS design. The amount of P that can be removed by harvesting is commonly around 10–20 kg P ha−1 a−1 (Hoffmann et al., 2009; Hille et al., 2019), but up to >100 kg P ha−1 a−1 if highly productive plants are used. This ‘phytoremediation’ may also be used to deplete sites with high P saturation (Silveira et al., 2013). Moreover, vegetation type (grasses, herbs, trees) impacts the nutrient uptake rate and filter properties (Prosser et al., 2020). Functional VFS are able to stop runoff and erosion before they reach a water body, thus keeping PP and DP within VFS boundaries where they can be incorporated in the soil matrix or solution, transformed and cycled, and taken up by the vegetation. Infiltration capacity and contact time can be optimised by selectively choosing plants with desirable characteristics, such as deep roots, stiff-stems, or tall vegetation forms—traits that have been shown to increase infiltration, slow down runoff, or support sediment deposition (Blanco-Canqui et al., 2004, Blanco-Canqui et al., 2006; Wu et al., 2016). Improved infiltration increases the volume of soil that comes into contact with runoff water and takes part in P retention and cycling. Expanding the functional soil volume could therefore relieve the P burden of the surface layers, especially as subsoils commonly have lower P concentrations and DPS, and thus high retention potential (Pizzeghello et al., 2016; Habibiandehkordi et al., 2019). Infiltration could be further enhanced by promoting a diverse invertebrate community (Colloff et al., 2010). However, the prevalence of an extensive macropore network and preferential flow could potentially limit soil-runoff interaction time and VFS nutrient mitigation efficacy (Orozco-López et al., 2018). Other limiting factors that need to be considered are overbank flooding and the presence of a seasonal water table (Sheppard et al., 2006; Fox et al., 2018).
In addition to vegetation, location and shape are essential elements in VFS design. Generally, width is considered to be the most critical variable in VFS performance. Consequently, many studies dealing with VFS efficacy ignore other crucial dimensions (see Prosser et al., 2020). Width is taken as a proxy for area, assuming that length (i.e., along the field/VFS border) is fixed and that depth is negligible. This ignores the role of subsurface layers and functional soil volume, and implies that runoff is exported uniformly across the entire field edge. It is, however, probable that runoff is instead bundled at distinct locations, due to flow convergence (Dosskey et al., 2002; Verstraeten et al., 2006). If these concentrations always occur at similar positions (e.g., thalweg), the VFS could be planned accordingly, e.g., wider at areas with concentrated flow and smaller at areas that receive little runoff. Additionally, the vegetation could be adjusted; stiff grasses can slow down runoff velocities and promote a more evenly distributed flow through the VFS (grass barriers; Blanco-Canqui et al., 2004). Also, improper maintenance and vegetation aging have an effect on VFS efficacy under concentrated flow (Lambrechts et al., 2014).
Slope and tillage direction may divert runoff to field corners, in which case L- or triangle-shaped VFS are advisable. Furthermore, anthropogenic flow paths (e.g., off-field ditches or channels) may bundle flowing off waters, or, due to local topography, runoff may be exported at an edge that is not closest to the receiving water body (Hösl et al., 2012). Both scenarios cause the runoff to completely bypass a shore-parallel VFS, mandating a more flexible placement of VFS where runoff and erosion actually occur. Customised VFS entail extra efforts for the farmers; it may thus be necessary to increase the financial incentives or find design compromises to ensure a high acceptance of non-standard VFS amongst practitioners. Finally, VFS design and shape could also be linked to cropping. A farmer could adopt supplemental measures when erosion-prone crops are cultivated, such as a greater VFS extent, grass barriers, or contour cropping.
All these considerations are crucial for an effective P management. Custom VFS designs as a function of local conditions are highly advisable on both the field and watershed scale (Jiang et al., 2020). However, many agri-environmental programmes do not demand such adaptive designs and recommend VFS of a fixed shape, width, and location instead (JRC, 2020). A more flexible approach with more possibilities and options would certainly not only increase the efficacy of VFS in general but also the allocation of appropriate funding schemes.
Conclusion and Outlook
There is a substantial delay of knowledge transfer from the research front to VFS design, implementation, monitoring and, ultimately, policy. In the United Kingdom, for instance, there is no targeting of funding of VFS to water quality areas, no bespoke variation of VFS widths, and there are no resources for monitoring. In Austria, grasslands may be eligible to be funded as VFS by the agri-environmental programme ÖPUL if their nearest part is within 50 m distance of surface water, have an average width of 12 m, and are at the field edge that is closest to the water (BMNT, 2015); no modelling, a-priori measurements, or monitoring is required. Similar static, unsatisfactory conditions are found across most European countries (JRC, 2020). Although there is some promising progress in VFS research, such as the development of tools for mapping flow paths or the experimental implementation of variable width buffers, these are often incomplete or restricted to pilot projects (e.g., Shrivastav et al., 2020).
VFS designs are therefore often still unspecific and outdated, especially regarding sustainability and long-term efficiency. We suggest a step-wise approach to custom VFS designs, leading to VFS that are effective and persistent. Based on local conditions rather than a one-type-fits-all scheme, this procedure is inevitably more labour- and cost-intensive. Nonetheless, the substantial variability in VFS performance—from 100% retention to net nutrient release or completely ineffective VFS due to flow bypass—is clearly not satisfactory and the ecological and socio-economic cost/benefit ratio is far from what could actually be achievable (Hoffmann et al., 2009; Stutter et al., 2021). The presented steps are a best-case scenario; however, even following only a few of the recommendations would be an improvement compared to standard VFS designs (Figure 1).
VFS need to be appropriately designed to counter high nutrient dynamics, i.e., their P retention and removal potential has to meet actual P inputs in space and time. Accordingly, the scientific evaluations of VFS have to account for the resulting heterogeneity in VFS and the vertical (e.g., infiltration, preferential flow, root zone) and lateral aspects (e.g., flow concentration, subsurface interflow) that affect VFS performance, e.g., by sampling along transects and depth profiles, or the inclusion of subsurface flow in runoff/erosion experiments (Weihrauch, 2019; Prosser et al., 2020). Different P forms along the solubility/bioavailability continuum are of different (ecological) concern and require the use of appropriate measurement techniques to adequately assess the most relevant P species. The complex bio-geochemistry of VFS and internal P cycling and transformation processes complicate an a priori estimation of P export risks. To be able to counter unforeseen developments and ensure long-term efficacy and protection of surface waters, VFS should also be monitored, as is common practice for other environmental mitigation measures (Wortley et al., 2013). Furthermore, VFS models should be improved by incorporating factors such as flow concentrations and diversions (Fox et al., 2010; Hénault-Ethier et al., 2017), preferential flow pathways (Guertault et al., 2021), or nutrient accumulation in the soil.
The success of VFS relies heavily on the willingness of farmers to implement them—and their acceptance will rise and fall with the costs and benefits that come with VFS (Chapman et al., 2019). Trade-offs between environmental interests and agricultural production and a potential mismatch of the goals and motivations of the stakeholders involved (i.e., scientists, policy-makers, farmers) may hinder the implementation of VFS. A fair and balanced mix of regulatory (e.g., legislation, directives) and economic levers (e.g., subsidies, incentive payments) is probably the most promising approach (McGonigle et al., 2012). Within such a framework, national and regional agricultural authorities should provide the resources and expertise to put forth site-specific solutions and corresponding VFS specifications that can be used as a sound basis for consultation with farmers. Furthermore, agricultural authorities could select representative sites for a given region that are monitored in detail and can be used to develop improved VFS designs in line with local characteristics. An intensified pro-active informing of authorities on new approaches and technologies and a closer collaboration of scientists and consultants could further bridge the gap between the state-of-knowledge and the state-of-implementation (Fox et al., 2021).
We want to stress that the most appropriate approach to address the root problem—excess P in agricultural systems—is via an improved P management and the prevention of a build-up of nutrients in the field in the first place. If this is not possible or insufficient, implementing VFS is an efficient, eco-friendly, and cost-effective method for nutrient retention and removal (Lovell and Sullivan, 2006; Jabłońska et al., 2020) that should be considered over more technical solutions (e.g., filters, chemical amendments, P-sorbing materials; Habibiandehkordi et al., 2015; Cooke et al., 2021). In many cases, a VFS will make up one part of a larger conservation scheme, or one link in a chain of measures (e.g., “designed multicomponent buffer zones”, “3D buffer zones”, Stutter et al. (2019), Stutter et al. (2020); “integrated buffer zones”, Zak et al. (2019)). Such measures can also tackle other environmental issues such as biodiversity or carbon sequestration, enrich the landscape, or provide additional income for the farmer (Stutter et al., 2012; Uggeldahl and Olsen, 2019; Cole et al., 2020). This multifunctionality and the ability to address several problems at once should be seen as a strong asset of VFS—creating ample reasons for agricultural authorities to promote their use and increase compensation schemes.
Since their introduction, VFS have evolved from simple nutrient containers to multi-dimensional entities with an increasingly complex inner life (Weihrauch, 2019). Our obligation is to face these new arising challenges and communicate their implications to those responsible for the implementation.
Data Availability Statement
The original contributions presented in the study are included in the article/Supplementary Material, further inquiries can be directed to the corresponding author.
Author Contributions
All authors participated in the discussion leading to the paper and contributed to the conception and writing of the manuscript. DR took the lead in writing and overall organization of the manuscript. All authors contributed to manuscript revision and read and approved the submitted version.
Funding
This paper was partly funded by the Province of Lower Austria under the project RIBUST (K3-F-130/005-2019) and the SHui project of the EU (RIA project number 773903-1)
Conflict of Interest
The authors declare that the research was conducted in the absence of any commercial or financial relationships that could be construed as a potential conflict of interest.pt
Publisher’s Note
All claims expressed in this article are solely those of the authors and do not necessarily represent those of their affiliated organizations, or those of the publisher, the editors, and the reviewers. Any product that may be evaluated in this article, or claim that may be made by its manufacturer, is not guaranteed or endorsed by the publisher.
References
Allison, R. V. (1935). The Importance of the Vegetation Factor in Water Conservation and Erosion Control. Soil Sci. Soc. Am. J. B16, 119–122. doi:10.2136/sssaj1935.036159950B1620010033x
Arnold, J. G., Moriasi, D. N., Gassman, P. W., Abbaspour, K. C., White, M. J., Srinivasan, R., et al. (2012). SWAT: Model Use, Calibration, and Validation. Trans. ASABE 55, 1491–1508. doi:10.13031/2013.42256
Blanco-Canqui, H., Gantzer, C. J., Anderson, S. H., and Alberts, E. E. (2004). Grass Barriers for Reduced Concentrated Flow Induced Soil and Nutrient Loss. Soil Sci. Soc. Am. J. 68, 1963–1972. doi:10.2136/sssaj2004.1963
Blanco-Canqui, H., Gantzer, C. J., and Anderson, S. H. (2006). Performance of Grass Barriers and Filter Strips under Interrill and Concentrated Flow. J. Environ. Qual. 35, 1969–1974. doi:10.2134/jeq2006.0073
BMNT (2015). Sonderrichtlinie ÖPUL 2015. Available at: https://info.bmlrt.gv.at/themen/landwirtschaft/eu-agrarpolitik-foerderungen/laendl_entwicklung/foerderinfo/sonderrichtlinien_auswahlkriterien/srl_oepul.html (Accessed 02 15, 2022).
Carstensen, M. V., Hashemi, F., Hoffmann, C. C., Zak, D., Audet, J., and Kronvang, B. (2020). Efficiency of Mitigation Measures Targeting Nutrient Losses from Agricultural Drainage Systems: A Review. Ambio 49, 1820–1837. doi:10.1007/s13280-020-01345-5
Chapman, M., Satterfield, T., and Chan, K. M. A. (2019). When Value Conflicts Are Barriers: Can Relational Values Help Explain Farmer Participation in Conservation Incentive Programs? Land use policy 82, 464–475. doi:10.1016/j.landusepol.2018.11.017
Cole, L. J., Stockan, J., and Helliwell, R. (2020). Managing Riparian Buffer Strips to Optimise Ecosystem Services: A Review. Agric. Ecosyst. Environ. 296, 106891. doi:10.1016/j.agee.2020.106891
Colloff, M. J., Pullen, K. R., and Cunningham, S. A. (2010). Restoration of an Ecosystem Function to Revegetation Communities: The Role of Invertebrate Macropores in Enhancing Soil Water Infiltration. Restor. Ecol. 18, 65–72. doi:10.1111/j.1526-100X.2010.00667.x
Cooke, A. L., Simmons, R. W., Rickson, R. J., and Sakrabani, R. (2021). Efficacy of Selected Phosphorus Sorbing Materials (PSMs) to Enhance the Orthophosphate Sorption Capacity of Filter Socks. Water Environ. J. 35, 807–818. doi:10.1111/wej.12671
Cooper, J. R., and Gilliam, J. W. (1987). Phosphorus Redistribution from Cultivated Fields into Riparian Areas. Soil Sci. Soc. Am. J. 51, 1600–1604. doi:10.2136/sssaj1987.03615995005100060035x
Dosskey, M. G., Helmers, M. J., Eisenhauer, D. E., Franti, T. G., and Hoagland, K. D. (2002). Assessment of Concentrated Flow through Riparian Buffers. J. Soil Water Conserv. 57, 336. Available at: http://www.jswconline.org/content/57/6/336.abstract.
Fox, G. A., Muñoz-Carpena, R., and Sabbagh, G. J. (2010). Influence of Flow Concentration on Parameter Importance and Prediction Uncertainty of Pesticide Trapping by Vegetative Filter Strips. J. Hydrol. 384, 164–173. doi:10.1016/j.jhydrol.2010.01.020
Fox, G. A., Muñoz-Carpena, R., and Purvis, R. A. (2018). Controlled Laboratory Experiments and Modeling of Vegetative Filter Strips with Shallow Water Tables. J. Hydrol. 556, 1–9. doi:10.1016/j.jhydrol.2017.10.069
Fox, G. A., Muñoz-Carpena, R., Brooks, B., and Hall, T. (2021). Advancing Surface Water Pesticide Exposure Assessments for Ecosystem Protection. Trans. ASABE 64, 377–387. doi:10.13031/trans.14225
Guertault, L., Fox, G. A., Halihan, T., and Muñoz-Carpena, R. (2021). Quantifying the Importance of Preferential Flow in a Riparian Buffer. Trans. ASABE 64, 937–947. doi:10.13031/trans.14286
Habibiandehkordi, R., Quinton, J. N., and Surridge, B. W. J. (2015). Long-term Effects of Drinking-Water Treatment Residuals on Dissolved Phosphorus export from Vegetated Buffer Strips. Environ. Sci. Pollut. Res. 22, 6068–6076. doi:10.1007/s11356-014-3802-y
Habibiandehkordi, R., Lobb, D. A., Owens, P. N., and Flaten, D. N. (2019). Effectiveness of Vegetated Buffer Strips in Controlling Legacy Phosphorus Exports from Agricultural Land. J. Environ. Qual. 48, 314–321. doi:10.2134/jeq2018.04.0129
Hénault-Ethier, L., Larocque, M., Perron, R., Wiseman, N., and Labrecque, M. (2017). Hydrological Heterogeneity in Agricultural Riparian Buffer Strips. J. Hydrol. 546, 276–288. doi:10.1016/j.jhydrol.2017.01.001
Hille, S., Graeber, D., Kronvang, B., Rubaek, G. H., Onnen, N., Molina-Navarro, E., et al. (2019). Management Options to Reduce Phosphorus Leaching from Vegetated Buffer Strips. J. Environ. Qual. 48, 322–329. doi:10.2134/jeq2018.01.0042
Hösl, R., Strauss, P., and Glade, T. (2012). Man-made Linear Flow Paths at Catchment Scale: Identification, Factors and Consequences for the Efficiency of Vegetated Filter Strips. Landscape Urban Plann. 104, 245–252. doi:10.1016/j.landurbplan.2011.10.017
Hoffmann, C. C., Kjaergaard, C., Uusi-Kämppä, J., Hansen, H. C. B., and Kronvang, B. (2009). Phosphorus Retention in Riparian Buffers: Review of Their Efficiency. J. Environ. Qual. 38, 1942–1955. doi:10.2134/jeq2008.0087
Jabłońska, E., Wiśniewska, M., Marcinkowski, P., Grygoruk, M., Walton, C. R., Zak, D., et al. (2020). Catchment-Scale Analysis Reveals High Cost-Effectiveness of Wetland Buffer Zones as a Remedy to Non-Point Nutrient Pollution in North-Eastern Poland. Water 12, 629. doi:10.3390/w12030629
Jiang, F., Preisendanz, H. E., Veith, T. L., Cibin, R., and Drohan, P. J. (2020). Riparian Buffer Effectiveness as a Function of Buffer Design and Input Loads. J. Environ. Qual. 49, 1599–1611. doi:10.1002/jeq2.20149
JRC (2020). Good Agricultural and Environmental Condition. Available at: https://marswiki.jrc.ec.europa.eu/gaec/index.php (Accessed 02 15, 2022).
Kieta, K. A., Owens, P. N., Lobb, D. A., Vanrobaeys, J. A., and Flaten, D. N. (2018). Phosphorus Dynamics in Vegetated Buffer Strips in Cold Climates: A Review. Environ. Rev. 26, 255–272. doi:10.1139/er-2017-0077
Kleinman, P. J. A. (2017). The Persistent Environmental Relevance of Soil Phosphorus Sorption Saturation. Curr. Pollut. Rep 3, 141–150. doi:10.1007/s40726-017-0058-4
Kovacs, A. S., Honti, M., and Clement, A. (2008). Design of Best Management Practice Applications for Diffuse Phosphorus Pollution Using Interactive GIS. Water Sci. Technol. 57, 1727–1733. doi:10.2166/wst.2008.264
Kruse, J., Abraham, M., Amelung, W., Baum, C., Bol, R., Kühn, O., et al. (2015). Innovative Methods in Soil Phosphorus Research: A Review. J. Plant Nutr. Soil Sci. 178, 43–88. doi:10.1002/jpln.201400327
Laflen, J. M., Elliot, W. J., Flanagan, D. C., Meyer, C. R., and Nearing, M. A. (1997). WEPP-predicting Water Erosion Using a Process-Based Model. J. Soil Water Conserv. 52, 96. Available at: http://www.jswconline.org/content/52/2/96.abstract.
Lambrechts, T., François, S., Lutts, S., Muñoz-Carpena, R., and Bielders, C. L. (2014). Impact of Plant Growth and Morphology and of Sediment Concentration on Sediment Retention Efficiency of Vegetative Filter Strips: Flume Experiments and VFSMOD Modeling. J. Hydrol. 511, 800–810. doi:10.1016/j.jhydrol.2014.02.030
Lind, L., Hasselquist, E. M., and Laudon, H. (2019). Towards Ecologically Functional Riparian Zones: A Meta-Analysis to Develop Guidelines for Protecting Ecosystem Functions and Biodiversity in Agricultural Landscapes. J. Environ. Manage. 249, 109391. doi:10.1016/j.jenvman.2019.109391
Lovell, S. T., and Sullivan, W. C. (2006). Environmental Benefits of Conservation Buffers in the United States: Evidence, Promise, and Open Questions. Agric. Ecosyst. Environ. 112, 249–260. doi:10.1016/j.agee.2005.08.002
Lowrance, R., Altier, L. S., Williams, R. G., Inamdar, S. P., Sheridan, J. M., Bosch, D. D., et al. (2000). REMM: The Riparian Ecosystem Management Model. J. Soil Water Conserv. 55, 27. Available at: http://www.jswconline.org/content/55/1/27.abstract.
McGonigle, D. F., Harris, R. C., McCamphill, C., Kirk, S., Dils, R., Macdonald, J., et al. (2012). Towards a More Strategic Approach to Research to Support Catchment-Based Policy Approaches to Mitigate Agricultural Water Pollution: A UK Case-Study. Environ. Sci. Pol. 24, 4–14. doi:10.1016/j.envsci.2012.07.016
Morgan, R. P. C., Quinton, J. N., Smith, R. E., Govers, G., Poesen, J. W. A., Auerswald, K., et al. (1998). The European Soil Erosion Model (EUROSEM): a Dynamic Approach for Predicting Sediment Transport from fields and Small Catchments. Earth Surf. Process. Landforms 23, 527–544. doi:10.1002/(sici)1096-9837(199806)23:6<527::aid-esp868>3.0.co;2-5
Muñoz-Carpena, R., Parsons, J. E., and Gilliam, J. W. (1999). Modeling Hydrology and Sediment Transport in Vegetative Filter Strips. J. Hydrol. 214, 111–129. doi:10.1016/S0022-1694(98)00272-8
Orozco-López, E., Muñoz-Carpena, R., Gao, B., and Fox, G. A. (2018). Riparian Vadose Zone Preferential Flow: Review of Concepts, Limitations, and Perspectives. Vadose Zone J. 17, 1–20. doi:10.2136/vzj2018.02.0031
Pautler, M. C., and Sims, J. T. (2000). Relationships between Soil Test Phosphorus, Soluble Phosphorus, and Phosphorus Saturation in Delaware Soils. Soil Sci. Soc. Am. J. 64, 765–773. doi:10.2136/sssaj2000.642765x
Pizzeghello, D., Berti, A., Nardi, S., and Morari, F. (2016). Relationship between Soil Test Phosphorus and Phosphorus Release to Solution in Three Soils after Long-Term mineral and Manure Application. Agric. Ecosyst. Environ. 233, 214–223. doi:10.1016/j.agee.2016.09.015
Prosser, R. S., Hoekstra, P. F., Gene, S., Truman, C., White, M., and Hanson, M. L. (2020). A Review of the Effectiveness of Vegetated Buffers to Mitigate Pesticide and Nutrient Transport into Surface Waters from Agricultural Areas. J. Environ. Manage. 261, 110210. doi:10.1016/j.jenvman.2020.110210
Ries, F., Kirn, L., and Weiler, M. (2020). Experimental Investigation of Runoff Generation Following Extreme Precipitation. Hydrol. Water Resour. Manag. 5, 221–236. doi:10.5675/HyWa_2020.5_1
Roberts, J., and Israel, D. W. (2017). Influence of Soil Properties and Manure Sources on Phosphorus in Runoff during Simulated Rainfall. Commun. Soil Sci. Plant Anal. 48, 222–235. doi:10.1080/00103624.2016.1254236
Roberts, W. M., Stutter, M. I., and Haygarth, P. M. (2012). Phosphorus Retention and Remobilization in Vegetated Buffer Strips: A Review. J. Environ. Qual. 41, 389–399. doi:10.2134/jeq2010.0543
Roy, E. D., Willig, E., Richards, P. D., Martinelli, L. A., Vazquez, F. F., Pegorini, L., et al. (2017). Soil Phosphorus Sorption Capacity after Three Decades of Intensive Fertilization in Mato Grosso, Brazil. Agric. Ecosyst. Environ. 249, 206–214. doi:10.1016/j.agee.2017.08.004
Schauder, H., and Auerswald, K. (1992). Long‐term Trapping Efficiency of a Vegetated Filter Strip under Agricultural Use. Z. Pflanzenernaehr. Bodenk. 155, 489–492. doi:10.1002/jpln.19921550523
Schindler, D. W., Carpenter, S. R., Chapra, S. C., Hecky, R. E., and Orihel, D. M. (2016). Reducing Phosphorus to Curb Lake Eutrophication Is a Success. Environ. Sci. Technol. 50, 8923–8929. doi:10.1021/acs.est.6b02204
Sheppard, S. C., Sheppard, M. I., Long, J., Sanipelli, B., and Tait, J. (2006). Runoff Phosphorus Retention in Vegetated Field Margins on Flat Landscapes. Can. J. Soil Sci. 86, 871–884. doi:10.4141/S05-072
Shrivastav, M., Mickelson, S. K., and Webber, D. (2020). Using ArcGIS Hydrologic Modeling and LiDAR Digital Elevation Data to Evaluate Surface Runoff Interception Performance of Riparian Vegetative Filter Strip Buffers in central Iowa. J. Soil Water Conserv. 75, 123–129. doi:10.2489/jswc.75.1.123
Silveira, M. L., Vendramini, J. M. B., Sui, X., Sollenberger, L., and O’Connor, G. A. (2013). Screening Perennial Warm-Season Bioenergy Crops as an Alternative for Phytoremediation of Excess Soil P. Bioenerg. Res. 6, 469–475. doi:10.1007/s12155-012-9267-2
Stutter, M. I., Langan, S. J., and Lumsdon, D. G. (2009). Vegetated Buffer Strips Can Lead to Increased Release of Phosphorus to Waters: A Biogeochemical Assessment of the Mechanisms. Environ. Sci. Technol. 43, 1858–1863. doi:10.1021/es8030193
Stutter, M. I., Chardon, W. J., and Kronvang, B. (2012). Riparian Buffer Strips as a Multifunctional Management Tool in Agricultural Landscapes: Introduction. J. Environ. Qual. 41, 297–303. doi:10.2134/jeq2011.0439
Stutter, M., Kronvang, B., Ó hUallacháin, D., and Rozemeijer, J. (2019). Current Insights into the Effectiveness of Riparian Management, Attainment of Multiple Benefits, and Potential Technical Enhancements. J. Environ. Qual. 48, 236–247. doi:10.2134/jeq2019.01.0020
Stutter, M., Wilkinson, M., and Nisbet, T. (2020). 3D Buffer Strips: Designed to Deliver More for the Environment. Bristol, UK. Available at: https://www.gov.uk/government/publications/3d-buffer-strips-designed-to-deliver-more-for-the-environment (Accessed 02 15, 2022).
Stutter, M., Costa, F. B., and Ó hUallacháin, D. (2021). The Interactions of Site-specific Factors on Riparian Buffer Effectiveness across Multiple Pollutants: A Review. Sci. Total Environ. 798, 149238. doi:10.1016/j.scitotenv.2021.149238
Tiefenbacher, A., Weigelhofer, G., Klik, A., Mabit, L., Santner, J., Wenzel, W., et al. (2021). Antecedent Soil Moisture and Rain Intensity Control Pathways and Quality of Organic Carbon Exports from Arable Land. CATENA 202, 105297. doi:10.1016/j.catena.2021.105297
Uggeldahl, K. C., and Olsen, S. B. (2019). Public Preferences for Co-benefits of Riparian Buffer Strips in Denmark: An Economic Valuation Study. J. Environ. Manage. 239, 342–351. doi:10.1016/j.jenvman.2019.03.078
Vanderholm, D. H., Dickey, E. C., Jackobs, J. A., Elmore, R. W., and Spahr, S. L. (1979). Liestock Feedlot Runoff Control by Vegetative Filters. EPA Rep. 600, 1–66.
Vanrobaeys, J. A., Owens, P. N., Lobb, D. A., Kieta, K. A., and Campbell, J. M. (2019). Seasonal Efficacy of Vegetated Filter Strips for Phosphorus Reduction in Surface Runoff. J. Env Qual. 48, 880–888. doi:10.2134/jeq2018.12.0452
Verstraeten, G., Poesen, J., Gillijns, K., and Govers, G. (2006). The Use of Riparian Vegetated Filter Strips to Reduce River Sediment Loads: an Overestimated Control Measure? Hydrol. Process. 20, 4259–4267. doi:10.1002/hyp.6155
Wang, G., Wu, B., Zhang, L., Jiang, H., and Xu, Z. (2014). Role of Soil Erodibility in Affecting Available Nitrogen and Phosphorus Losses under Simulated Rainfall. J. Hydrol. 514, 180–191. doi:10.1016/j.jhydrol.2014.04.028
Weihrauch, C., and Opp, C. (2018). Ecologically Relevant Phosphorus Pools in Soils and Their Dynamics: The story So Far. Geoderma 325, 183–194. doi:10.1016/j.geoderma.2018.02.047
Weihrauch, C. (2019). Dynamics Need Space - A Geospatial Approach to Soil Phosphorus' Reactions and Migration. Geoderma 354, 113775. doi:10.1016/j.geoderma.2019.05.025
Willrich, T. L., and Boda, J. O. (1976). Overland Flow Treatment of Swine Lagoon Effluent. ASAE Pap. No. 764515.
Wortley, L., Hero, J.-M., and Howes, M. (2013). Evaluating Ecological Restoration Success: A Review of the Literature. Restor. Ecol. 21, 537–543. doi:10.1111/rec.12028
Wu, G.-L., Yang, Z., Cui, Z., Liu, Y., Fang, N.-F., and Shi, Z.-H. (2016). Mixed Artificial Grasslands with More Roots Improved Mine Soil Infiltration Capacity. J. Hydrol. 535, 54–60. doi:10.1016/j.jhydrol.2016.01.059
Keywords: riparian buffer strips (RBS), nutrient management, adaptive design, functional soil volume, erosion, runoff, concentrated flow, vegetated filter strips
Citation: Ramler D, Stutter M, Weigelhofer G, Quinton JN, Hood-Nowotny R and Strauss P (2022) Keeping Up with Phosphorus Dynamics: Overdue Conceptual Changes in Vegetative Filter Strip Research and Management. Front. Environ. Sci. 10:764333. doi: 10.3389/fenvs.2022.764333
Received: 25 August 2021; Accepted: 25 February 2022;
Published: 01 April 2022.
Edited by:
Andrew Hursthouse, University of the West of Scotland, United KingdomReviewed by:
Alper Elçi, Dokuz Eylül University, TurkeyMosammat Mustari Khanaum, North Dakota State University, United States
Rafael Muñoz-Carpena, University of Florida, United States
Copyright © 2022 Ramler, Stutter, Weigelhofer, Quinton, Hood-Nowotny and Strauss. This is an open-access article distributed under the terms of the Creative Commons Attribution License (CC BY). The use, distribution or reproduction in other forums is permitted, provided the original author(s) and the copyright owner(s) are credited and that the original publication in this journal is cited, in accordance with accepted academic practice. No use, distribution or reproduction is permitted which does not comply with these terms.
*Correspondence: David Ramler, ZGF2aWQucmFtbGVyQGJhdy5hdA==