- 1Ecodynamics Group, Department of Earth, Environmental and Physical Sciences, University of Siena, Siena, Italy
- 2R2ES Lab, Department of Biotechnology, Chemistry and Pharmacy, University of Siena, Siena, Italy
- 3Center for Colloid and Surface Science (CSGI), Florence, Italy
- 4Santa Chiara Lab, University of Siena, Siena, Italy
- 5Section of Biomass Technology, Center of Bioresource and Biorefinery, Danish Technological Institute, Taastrup, Denmark
The overexploitation of fossil fuels as main energy source to support the global economy is identified as the most responsible of the current critical situation from an environmental viewpoint. The need to replace fossil fuels has posed the attention on alternative energy sources such as biofuels, in both developed and developing countries. Africa, for example, has enormous natural resources in the form of biomass from agriculture and other related processes (i.e., food residues). An action that can help fight climate change is the implementation of biofuel refineries to maximize the value of biomass by converting it into a range of products, like energy vectors, biomaterials, feed and fertilizers. By using emergy evaluation and Life Cycle Assessment (LCA) this study focused on the potential development of sustainable biotechnological processes fed by biowaste and bioresidues in two African countries (Egypt and Ghana). We assessed the sustainability level of two biofuel productions based on starch and lignocellulosic feedstocks (i.e., cassava peel and corn stover, respectively). A first understanding of the sustainability of the case studies was obtained and the results showed that the biorefinery based on cassava peel was more sustainable from both the user and donor perspectives. Indeed, the LCA results showed that impact categories Global Warming Potential (GWP) and Acidification Potential (AP) had lower values for cassava compared to corn stover biorefinery and emergy outcomes highlighted that the starch-rich feedstock had lower Unit Emergy Value (UEV) and higher renewability percentage (94%). These results suggest that biorefineries are an option for world bioeconomy strategy as they enable optimization of agricultural and food residues and their environmental performance in producing a renewable substitute for fossil fuels and other non-renewable materials is promising.
Introduction
Since non-renewable resources, such as crude oil, are limited and its overexploitation has negative consequence for the planet and humans, the need for human society to move away from dependence on fossil fuels is now widely recognized [United Nations (UN), 2015a]. To this aim, two major actions were the adoption of the Sustainable Development Goals in September 2015 [United Nations (UN), 2015b], particularly Goal 7 (Affordable and clean energy) and Goal 13 (Climate action), and the Paris agreement on reduction of global emissions [United Nations (UN), 2015a].
Alternative energy sources like biofuels have been considered to replace fossil fuels both in developed and developing countries (Lamers et al., 2011). According to the FAO definition [Food Agriculture Organization of the United Nations (FAO), 2006], biofuels are “fuels produced directly or indirectly from biomass, such as fuelwood, charcoal, bioethanol, biodiesel, biogas (methane), and biohydrogen.” Biofuels can be classified as first, second and third generation [International Energy Agency (IEA), 2008], depending on the feedstocks and conversion technology used to produce them.
To quote the EU bioeconomy policy, “the scopes of the Circular Economy and the Bioeconomy intersect in their common aim to add value to biological waste and residues” (European Commission (EC), 2018). The policy also suits developing countries, where bio-based waste is abundant. Africa, for example, has enormous natural resources in the form of biomass. However, innovative ways to exploit biomass from cultivation and other related processes are needed to enable African countries to benefit from the emerging bioeconomy sector (Fernando et al., 2006; Nzila et al., 2010; Okello et al., 2013; Thomsen et al., 2014; Nizami et al., 2017). Agricultural residues are a major source of lignocellulose for biofuel production, besides being waste materials that do not compete for land against food crops.
An action that can help fight climate change is the development of biofuel refineries, considered as sustainable alternative to oil refineries (Ghatak, 2011). The biorefinery concept relies on maximizing the value of biomass by converting it into a range of products, such as energy vectors, biomaterials, feed and fertilizers (Kamm and Kamm, 2004). While traditional oil-based refineries run on non-renewable resource, biorefineries are fed by renewable biomass feedstocks and can be classified as whole-crop, green or lignocellulose biorefineries (Kamm and Kamm, 2004). The first type uses raw materials such as cereals including maize; green biorefineries are based on biomass like grass, clover and lucerne, and lignocellulose biorefineries exploit biomass rich in cellulose. Almeida et al. (2013) pointed out that appropriate methods are essential to assess the actual environmental costs of any cleaner production and to support informed decision-making. Chemical analysis, biofuel yield assessment and systemic accounting are useful to understand the overall sustainability level of a bio-based production. Rosen (2018) highlighted the necessity of taking into account the environmental sustainability of biofuel production processes. This concept has been underpinned by Gnansounou and Alves (2019) recalling the importance of integrated sustainability assessments to depict and assess the biofuel complex production chain. Most of the methods currently used to investigate the sustainability of biofuels are based on energy analysis, Emergy evaluation, Life Cycle Assessment (LCA), Carbon Footprint and Water Footprint (Gopalakrishnan et al., 2009; Markeviius et al., 2010; Solomon, 2010; Kumar and Singh, 2019; Bezergianni and Chrysikou, 2020; Ubando et al., 2020). Most of the emergy-based studies regard first-generation biofuels and they all stress the criticality of these productions from a sustainability perspective. For example, Pereira and Ortega (2010) observed the low renewability of a large-scale system for ethanol production from sugarcane in Brazil, due to major environmental impacts and consumption of natural resources. Agostinho and Ortega (2013) analyzed the energy-environmental performance of a large-scale biorefinery in Brazil that produces ethanol from cellulose, comparing it with another large-scale system that produces bioethanol from sugarcane, and a small-scale Integrated system of Food, Energy and Environmental Services (IFEES). Of the two large-scale systems, the ethanol from cellulose option had better emergy efficiency, whereas the IFEES had the best energy-environmental performance. For second generation biofuels, Patrizi et al. (2015) did an emergy study of a designed biorefinery in the Province of Siena (Italy), fueled by locally produced residual heat and straw. The study introduced the concept of Unit Emergy Investment (UEI) as a basis for evaluation of outputs. Bioethanol production by a biorefinery coupled with local resource optimization was found to be feasible for that specific site.
LCA is another method widely used to investigate the sustainability of biofuel production systems (Cherubini and Strømman, 2011). Several recent LCA-based studies of bioethanol production focused on ethanol from lignocellulose systems, suggested to have the potential for mitigating climate change. In a review performed by Borrion et al. (2012), many studies were found to investigate issues related to climate change and energy consumption, with results that varied significantly from study to study. With regard to corn-based bioethanol, Pieragostini et al. (2014) performed an LCA on a production chain in Argentina, finding that the cultivation phase is the main contributor to the overall environmental impacts. With an LCA approach, Zhao et al. (2016) reviewed the technological advancement of bioethanol conversion based on corn stover in the Chinese context, while Muñoz et al. (2014) evaluated that bioethanol produced with corn stover was the best option regarding greenhouse gases (GHG) emissions, compared with other feedstocks.
Agricultural systems stand at the interface between natural and human systems and the emergy evaluation is an appropriate tool for its assessment (Bastianoni et al., 2007; Niccolucci et al., 2010; Ghisellini et al., 2014). Emergy highlights and accounts for the fundamental contribution of renewable resources in agricultural productions with respect to the human made resources. Nevertheless, emergy includes the non-renewable resources feeding a system as well. Hence, emergy is a holistic tool and best expresses its potentials in assessing agricultural systems (Bastianoni et al., 2007). Whereas, LCA is an environmental accounting tool able to depict and assess potential impacts occurring in the technosphere (i.e., human-dominated process) neglecting the environmental effort required for the resources production. Translating in emergy domain means that LCA accounts for the consequences of using the internal and imported non-renewable resources sustaining a product or production process.
According to Nizami et al. (2017), in developing countries, biorefineries fed by wastes represent a way forward to achieve both sustainable waste management and environmental benefits. In view of the above-mentioned literature, we focused here on integrated sustainability assessment of the potential development of sustainable biorefinery systems which run on biowaste and bioresidues produced by agriculture and industrial food processing in two African countries (i.e., Egypt and Ghana). Firstly, the exploitation of natural resources for the provision of biowaste and bioresidues has been considered through the emergy assessment. Then the environmental implications of the hypothesized biorefinery implementation has been investigated by means of LCA. Finally, by jointly reading outcomes of the two environmental sustainability assessments, a first insight on the feasibility of the two proposed biofuel solution for Egypt and Ghana has been drawn and discussed.
Materials and Methods
Case Studies
African food waste is associated with fruit and crop productions like bananas, cassava and sweet potatoes that are starch-rich products (Gustavsson et al., 2011), while agriculture produces a considerable amount of biowaste such as straw, leaves, roots and stover.
In this work we identified and compared a lignocellulosic and starch feedstock, namely corn stover and cassava peel, for their biofuel potential. Corn stover consists of leaves, stalks and husks left in the field after the corn harvest. Cassava peel is a starch-rich residue of cassava-processing.
The case study on sugar-rich (cellulose) feedstock was based on corn residues left in a field in Egypt. The stover to grain mass ratio was estimated at about 0.9:1 fresh weight (Pordesimo et al., 2004) and the system under study was a commercial cultivation of 142 ha. The case study on sugar-rich (starch) feedstock was based on cassava peel left over from cassava-processing in Ghana. The cassava reference system was a commercial farm of 10 ha producing 250 t of cassava per year. Since the study regarded hypothetical biorefineries, plant data (inputs and outputs) was drawn from the literature.
Methods
In order to better explore the environmental consequences due to the hypothesized biorefinery, two complementary methodologies have been selected. The environmental implications of the hypothesized productions have been considered firstly from a donor side perspective, with a focus on the exploitation of natural resources, by means of the emergy assessment (Odum, 1996). Then, the potential environmental impacts have been assessed through the LCA framework, thus considering the user side perspective. These two environmental accounting methodologies share many analogies in the way they are implemented (Raugei et al., 2014) up to the point that emergy has been proposed as an additional impact category within the LCA framework (Raugei et al., 2006; Rugani and Benetto, 2012). Raugei et al. (2014), in evaluating the possible integration of emergy into the LCA, concluded that “emergy may be a most valuable addition to LCA, specifically by providing a complementary donor-side perspective, a unified measure of the provision of environmental support, and an indication of the work of the environment that would be needed to replace what is consumed.” The approach implemented here adopts the complementarity of emergy evaluation and LCA and results have been drawn accordingly. Figure 1 shows the boundaries here adopted in carrying out the two methodologies.
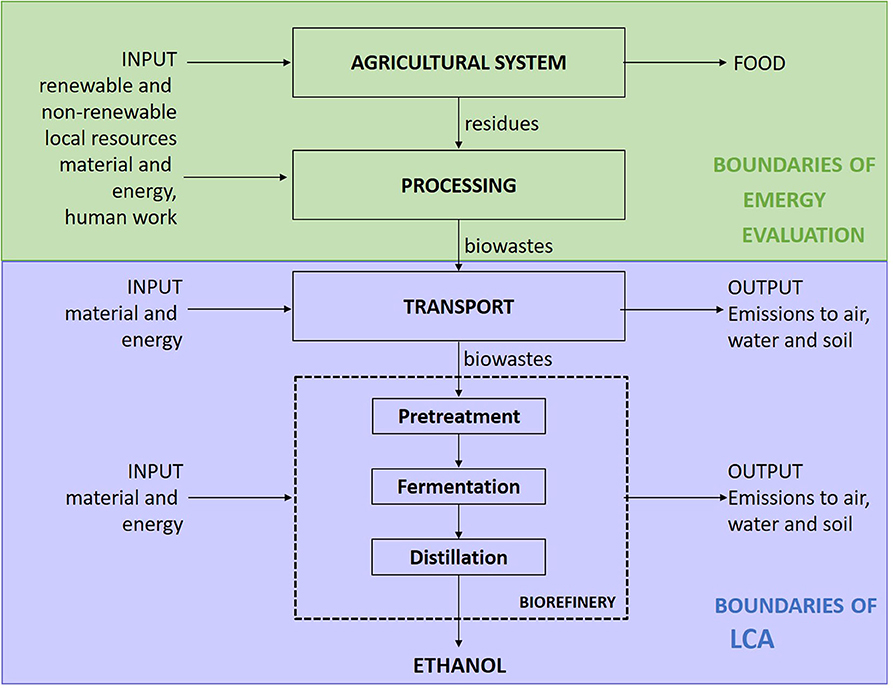
Figure 1. Boundaries of the Emergy evaluation (upper part) and LCA (bottom). Boundaries of the Emergy evaluation are related to the first part of the whole bioethanol production system that produces the main goods (food) and related residues (i.e., biosphere). Boundaries for the LCA are associate to the processes occurring in the second phase of the bioethanol production chain (i.e., from the field to the gate of the bioerefinery).
Emergy Evaluation
Emergy evaluation assigns a value to products and services by converting them into solar energy equivalents. This common denominator enables different resources, whether energy or matter, to be measured and compared. The unit of solar emergy is the solar emergy Joule (sej) (Odum and Odum, 2006). The emergy of different products was assessed by multiplying mass or energy of inputs by a transformation coefficient, called Unit Emergy Value (UEV). UEV is the solar emergy required, directly or indirectly, to make 1 unit (e.g., J or g) of a product. By definition, the solar emergy Em of a product or process is:
where Ei is the energy content of the ith independent input flow to the process and UEVi is the unit emergy value of the ith input flow.
According to Odum (1996) and based on their provenance and regeneration times, flows of resources can be: (a) emergy flows related to local renewable resources (R); (b) emergy flows related to local non-renewable resources (N); (c) emergy flows related to goods and services purchased outside the study system (F). Besides UEV, other emergy-related indicators may be used to characterize emergy results based on these different emergy flows. To this aim common indicators considered in the assessment of agricultural and agricultural residues productions are: percentage of renewable inputs (%R),) and Emergy Yield Ratio (EYR) (Ulgiati et al., 1993; Ghisellini et al., 2014). The first indicator highlights the contribution of renewable resources in producing the output considered with respect to the whole emergy supporting flow and is calculated as %R = R/Em. EYR is calculated as (R+N+F)/F and it expresses the ability of a process to using local renewable and non-renewable resource by investing in outside resources. These indicators will complete results obtained by the emergy evaluation providing an overview on the use of the different type of resources required by the agricultural systems.
To evaluate and compare feedstocks on the common basis of emergy, the glucan content was assumed to reflect the sugar content of the feedstocks, since glucans are the sum of starch and cellulose (Gustavsson et al., 2014). Given these values for both types of residue, the UEVs were calculated as:
To compare the potential of the residues for transformation into value-added products, their UEVs based on glucan content were calculated starting from their specific emergies, as shown by equation 2.
Emergy evaluation considered an emergy baseline of 12.00E+24 sej y−1 (Brown and Ulgiati, 2016). As shown in Figure 1 (upper section), emergy was used to evaluate the first part of the biofuel production chain (i.e., production of biowaste). Emergy evaluation has been found to have small sensitivity to the transformation phase (see e.g., Bastianoni and Marchettini, 1996; Seghetta et al., 2014) and therefore has been neglected.
LCA
LCA is a tool that enable the evaluation of the environmental burdens of products through their life cycle, from extraction of the resources, production of material, product parts and the product itself, to use of the product and end-of-life management (from cradle to grave) (Guinée et al., 2001; ISO 14040 14044, 2006).
According to Ahlgren et al. (2015), zero environmental burdens should be allocated to feedstock biomass, as it is recycled. Consequently, its potential impacts should not be included in the life cycle inventory of biorefinery systems. We therefore set the system boundaries of the present LCA of biofuel production from cradle to gate, i.e., from biowaste (to include the post cultivation phase), to the biorefinery plant producing bioethanol, considering also the transport of the feedstock (see Figure 1 bottom part). If we consider the system boundaries of the whole study (Figure 1 in total), we can maintain that boundaries are from grave (agricultural residues) to the gate (bioethanol production). LCA results enabled to assessing the environmental investment (in terms of potential environmental impacts) required to upgrade residues (i.e., cassava peels and corn stover) into added value products (the bioethanol) by exploiting their unexploited energy (glucan content).
Since the biorefineries have been based on information collected from the scientific literature, no primary data were available. However, data from reference studies on biorefinery systems based on corn stover (Jensen and Thyø, 2007) and cassava peel (Le et al., 2013) have been considered to draw up the related inventories (Tables 1, 2). We have hypothesized that the each of the two reference plants [namely the one from Jensen and Thyø (2007) and the other from Le et al. (2013)] and the correspondent hypothesized biorefinery will be equal per unit of output. It should be highlighted that the reference plant for the bioethanol production from corn stover represents one of the plants that actually reached the demonstration scale in Europe (Balan et al., 2013). The reference study for the cassava bioethanol production has been chosen because it has been carried out in a developing country, and it can be considered as the best solutions for the case study carried out in this work. A common functional unit (1 ton of bioethanol) was used for the LCA and to enable an appropriate comparison of the results.
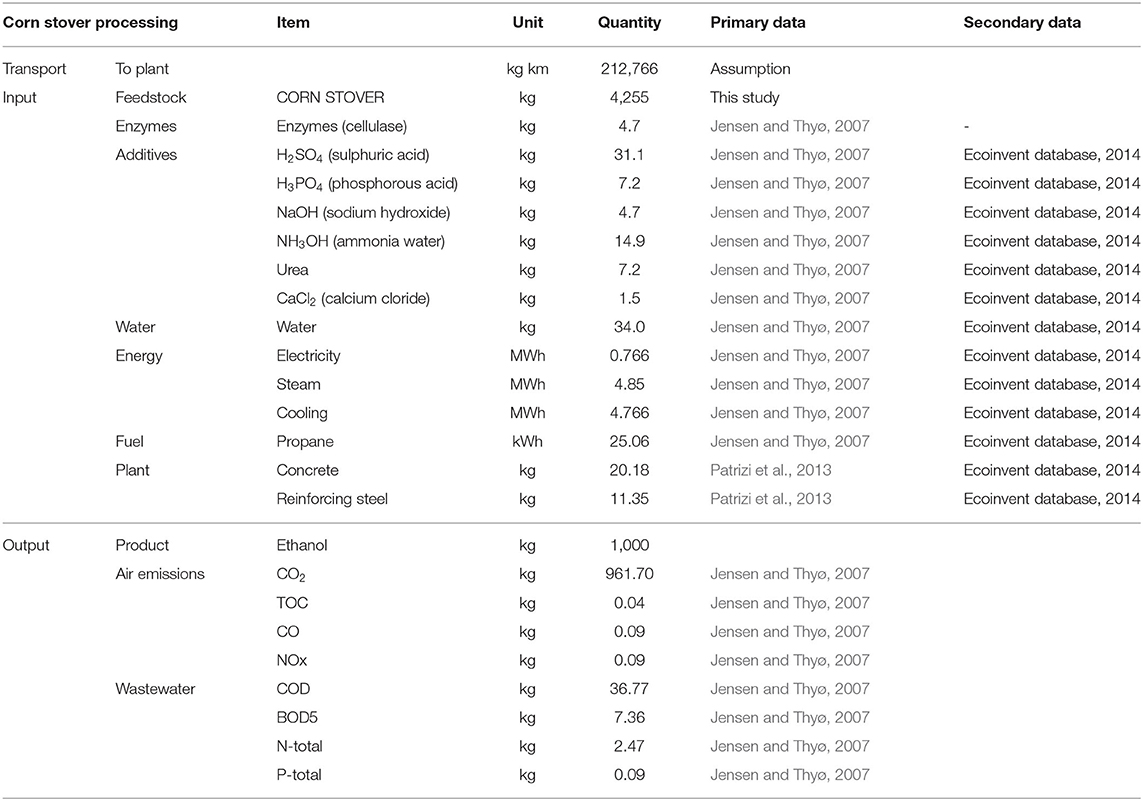
Table 1. Data Life cycle inventory for the production of 1 t of bioethanol based on corn stover feedstock.
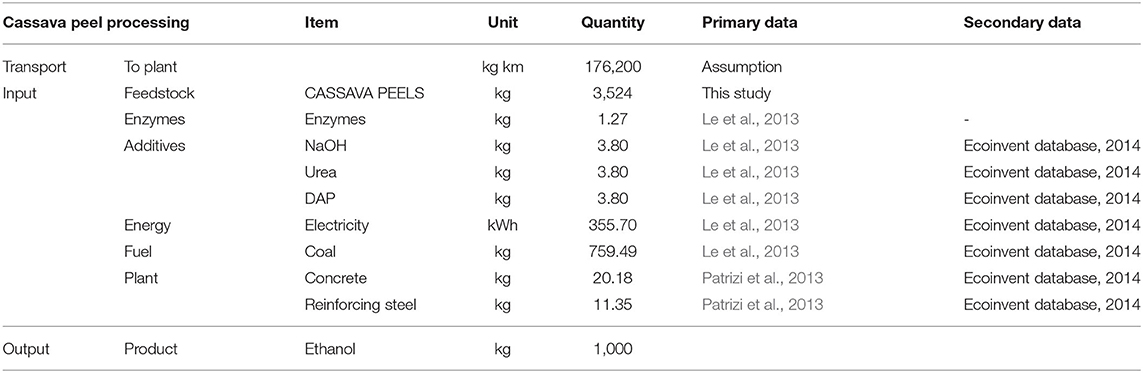
Table 2. Data Life cycle inventory for the production of 1 t of bioethanol based on cassava peel feedstock.
The bioethanol production data inventories were built up with info concerning materials and energy inputs flows needed to produce 1 ton of biofuel. The Life Cycle Inventory was developed with secondary data from the Ecoinvent v3 database (Ecoinvent, 2014). For both case studies we assumed that feedstock was transported for 50 km by a 7.5 ton truck from the biomass production site to the refinery plant; such assumption allowed to accounting for indirect emissions due to this phase of the bioethanol production (Gidamis et al., 2015). It should be highlighted that direct airborne and waterborne emissions due to the production phase of bioethanol have been assessed only for the biorefinery fueled by corn stover. Direct emissions associated to the bioethanol production from cassava peels conversion were not reported in the reference study and therefore have not been accounted. Therefore, direct emissions have been discussed only concerning the results of the bioethanol production process. Whereas, the environmental investment comparison between the two production processes has been carried out without considering direct emission.
We implemented the Life Cycle Impact Assessment method CML-IA, the updated version of CML 2 Baseline Method 2000, to characterize the LCA results (Guinée et al., 2001). In particular, Acidification Potential (AP), Eutrophication Potential (EP) and Global Warming Potential (GWP100) impact categories were selected, among the eleven available (PRé Consultants, 2016), as they have been widely applied in LCA studies of biorefineries projects (Bezergianni and Chrysikou, 2020). The LCA was run using the software SimaPro v8.
Results
Emergy of Biomass Feedstocks
Table 3 shows emergy evaluation details for traditional corn cultivation. The UEV of corn stover was 1.06E+09 sej g−1 and the final emergy per unit area was 6.37E+15 sej ha−1 y−1. Almost half the total emergy came from natural inputs (42%). The other contributors were fuel for field operations (20%), N fertilizers (22%) and human work (13%). %R is 48%; Emergy Yield Ratio (EYR) is 1.92.
Table 4 shows emergy evaluation details for cassava cultivation and processing. The UEV of cassava was 1.69E+08 sej g−1 and the emergy per unit area 2.21E+15 sej ha−1 y−1. The cassava production was mainly based on natural resources (67% of total emergy) and human work (28%), while the local non-renewable resources account for 2% of the total emergy flow supporting the system. The UEV of cassava peel was 8.57E+08 sej g−1 and the final emergy was 2.67E+15 sej ha−1 y−1. 83% of the emergy flow is mainly due to the cassava production, 16% to the human work required in the production plant and only 1% to the transport from the field to the plant. Cassava peels emergy indicators demonstrated a low impact on natural ecosystems and relied mainly on local resources indices: %R = 94%; EYR = 21.19.
The calculation of renewability of both the productions was carried out in line with Saladini et al. (2016), and do not include human work since it is itself supported by field production.
Life Cycle Impact Assessment
The AP, EP, and GWP 100 indicator values of bioethanol production from corn stover are reported in Table 5. For the GWP100 and AP impact categories the largest environmental burdens were due to energy consumed as electricity and steam (65% and 90% of the total impacts, respectively). Direct airborne and waterborne emissions were the second contributor to GWP100 (30%), and they generated the highest impacts for EP (43%), followed by potential burdens for plant construction: materials (29%) and energy (23%).
Table 6 shows the results concerning the biofuel production from cassava peels. For all categories, a large part of potential impacts was attributed to the consumption of coal to make the plant run. It contributed to 72% of GWP100, 54% of AP, and 33% of total EP impacts. Another significant contributor to EP was the plant construction materials (40%). Electricity process consumption contributes for 19% of GWP100 and 28% of AP impacts, respectively.
Figures 2–4 show the comparisons of the two biorefinery systems for the different impact categories. Regarding GWP100 (Figure 2), the corn stover system had showed a higher potential impact than the cassava biorefinery. The largest contributor was the energy input, responsible for 2063.43 kg CO2 eq (Figure 2).
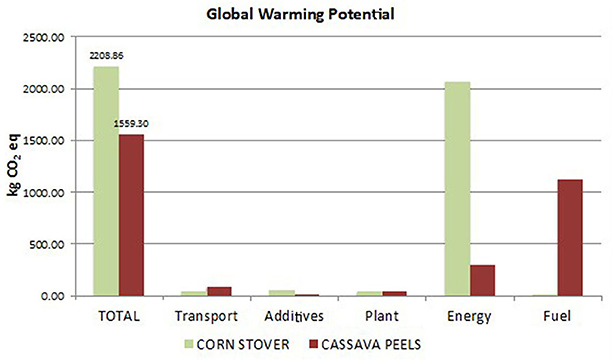
Figure 2. Results for the indicator GWP100 (i.e., Carbon Footprint), comparing bioethanol production from corn stover (green) and cassava peel (red).
A similar trend can be observed for AP (Figure 3), with higher impacts generated by the lignocellulosic-based biorefinery compared to the starch-based one. As already pointed out for GWP100, energy input also contributed heavily to AP.
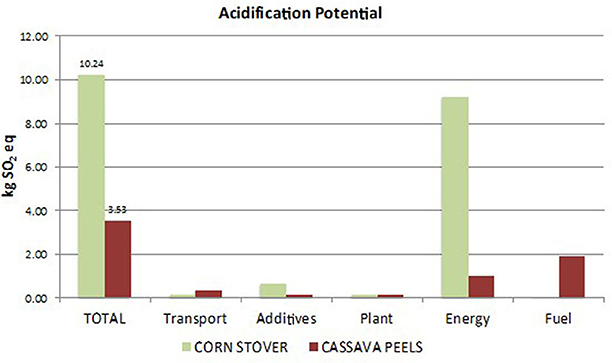
Figure 3. Results for the indicator AP, comparing bioethanol production from corn stover (green) and cassava peel (red).
Regarding EP (Figure 4), the impact assessments gave different results. The cassava biorefinery produced slightly higher impacts than the corn stover biorefinery (3.57 kg and 2.86 kg , respectively). In this case the highest impacts for both productions were attributed to the construction materials of biorefinery plant.
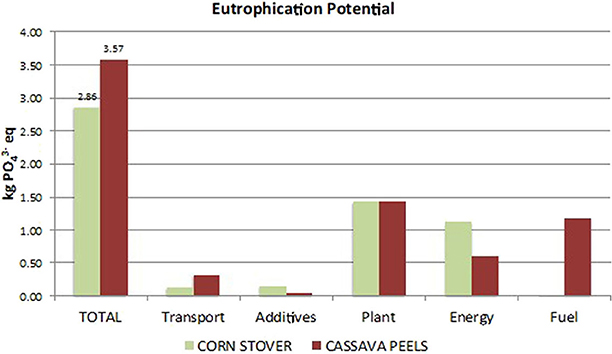
Figure 4. Results for the indicator EP, comparing bioethanol production from corn stover (green) and cassava peel (red).
Discussion
Cassava peels proved to be a promising organic residue from an emergy viewpoint: the UEVs based on glucan content confirmed that cassava peels was the more efficient in transforming the past and present solar energy needed for the production system into glucans. The high sugar content and low production intensity were responsible for this good result. The better results of this organic residue are also shown by the other emergy-related indices as the Emergy Yield Ratio value (EYR = 21.19) remarked the higher return obtained per unit of emergy invested, while the higher %R highlights the inferior pressure of the production system on the environment. These results confirm the outcome of Yang et al. (2011), for which cassava is a suitable alternative feedstock to produce ethanol, because comparing its emergy indices with those of ethanol based on wheat and corn residues, it was demonstrated the most sustainable one.
In order to compare the potentiality of cassava peels and corn stover as bioethanol feedstock from the donor side perspective, Figure 5 has been built starting from the approach proposed by Saladini et al. (2016). In order to compare the potential of different organic residues for transformation into value-added products, the UEVs based on glucan content were calculated by authors (cassava peels and corn stover), elaborated from other studies (wheat bran from Castellini et al., 2006; rice bran from Lu et al., 2010; sugar cane bagasse from Pereira and Ortega, 2010) and updated from Saladini et al. (2016) (olive pomace, pineapple peels, rejected bananas).
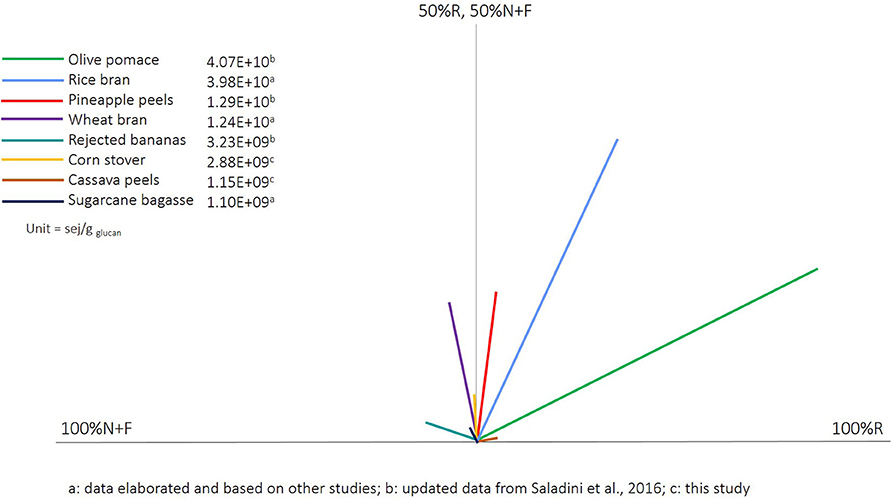
Figure 5. Summary of UEVs (expressed in sej/g of glucan) calculated for the sugar-rich feedstocks on the basis of glucan content, and related percentage renewability. The colored segments indicate the different feedstocks. Segment lengths are proportional to UEV (in sej g−1 of glucan) and slopes indicate the percentage of renewability level of the total emergy supporting the production system, from 100% (right end of horizontal axis) to 0% renewability (left end). A system with 50% renewability will fall on the vertical axis.
Figure 5 shows that cassava resulted with practically the same UEV as the sugarcane bagasse (that has the lowest UEV in absolute terms) however with a much higher renewability (35.4% for sugarcane). Considering both renewability and efficiency we can maintain that both cassava peels and corn stover can be considered good feedstock to be upgraded into bioethanol.
The LCA approach encloses the technosphere contribution and the environmental investment (accounted as potential impacts) required to upgrade residues into added value product (bioethanol). With regard to the GWP100 and AP impact categories, the results of this work showed that the production system fed by cassava peels generates lower potential environmental impacts against the biorefinery based on corn stover. For sake of clarity, even if we do not consider the direct emissions from bioethanol production from corn stover the same result is obtained (i.e., cassava has lower environmental impacts in GWP and AP indicators than corn stover). This outcome can be explained by the higher energy requirement of lignocellulosic-based biorefineries with respect to the starch-based ones, as the pretreatment phase is a crucial step in order to make cellulose and hemicellulose accessible for the subsequent enzymatic hydrolysis (Sims et al., 2008). Cassava peels have been recognized as very promising feedstock for bioethanol production by Rathnayake et al. (2018) compared to rice straw and cane molasses, based on LCA outcomes. Results from Rathnayake et al. (2018) are not comparable with those obtained in this paper since LCA boundaries are different. Nevertheless, we can maintain that the final judgment regarding cassava peels are consistent.
Even if corn stover showed lower performances, it should be noted that it has both economic and environmental potential for the production of bioethanol as an amendment to gasoline and thereby a substitute for fossil fuel (Morales et al., 2015; Zabed et al., 2016).
As highlighted by Leng et al. (2008), although cassava cultivation, treatment and denatured ethanol conversion are the most energy-intensive processes, the use of non-renewable resources (e.g., fossil fuel for energy) can improve our capacity to capture renewable energies in the future (e.g., biofuel). At the same time, the LCA methodology evaluates the environmental investment in terms of energy saving and carbon emission mitigation of biofuel and permits to appreciate the difference in sustainability between business-as-usual and bio-based fuel production, since the main goal of producing biofuels is to substitute fossil fuels. Comparing the GHG emissions of these two bioethanol productions with the fuel it should, at least partially, substitute (i.e., gasoline), we can see that the latter has 8.0 E-08 kg CO2eq/J while cassava peels has 5.8 E-08 kg CO2eq/J and corn stover has 1.1 E-07 kg CO2eq/J. This shows that, from a GHG emissions viewpoint, cassava peels based ethanol is surely an advantageous choice, while the one derived from corn stover needs further improvements to be competitive. One solution can be coupling the biorefinery with other production plants that require high temperature for their functioning, thus saving “fresh” energy to drive the transformation process (see for example Patrizi et al., 2013).
In a recent paper by Gnansounou et al. (2020), cassava peels and corn residues have been included in the portfolio of feedstock to satisfy energy demand in West Africa (i.e., 13 Countries) by 2050. Gnansounou et al. (2020) point out also that a policy shift is needed to implement their scenario: “If there is a political willingness to orient the energy system toward a bioeconomy, agricultural residues are potentially available in West Africa.” The very preliminary results presented in this paper complement outcomes of the Gnansounou et al. (2020), going a step further by assessing the environmental viability of two hypothesized biorefineries fueled by corn stover and cassava peels.
Conclusion
Several methods have been developed to evaluate the fundamental role of ecological systems in sustaining humanity and supporting bioeconomy. Bastianoni et al. (2008) highlighted the need for the joint use of many indicators to consider multiple sides of the state of a system. This approach has been followed in evaluating environmental feasibility of two hypothetical biorefineries in two African Countries. In this study, agricultural production is taken as an important source of biowaste and bioresidues to be exploited as potential feedstocks for biorefineries, with a focus on two African countries. We assessed the sustainability of biofuel production based on starch and lignocellulosic feedstocks (i.e., cassava peel and corn stover, respectively). To obtain a meaningful picture of the environmental sustainability of the case studies, we applied LCA and emergy evaluation jointly.
The adoption of emergy evaluation for assessing the ultimate energy required for the production of agricultural residues enabled a proper and consistent assessment of the biosphere's “work.” The assessment of the industrial phase through the LCA allowed the evaluation of drawback for the biosphere due to the bioethanol production process. A crucial point raised in this assessment is represented by the choice of the boundaries of the studies carried out. From a strict LCA viewpoint the boundaries are from cradle to gate, neglecting, in this way, the present and the past biosphere contribution to the production of agricultural residues. Indeed, no potential impacts have been generated by the production of these residues. Therefore, here we have considered expanded boundaries to appraise in a meaningful way the whole bioethanol production chain. The joint use of these two environmental methodologies enabled to depict consequences for both the resource side (biosphere) and human sphere (technosphere), in a coherent way.
The LCA results showed that the impact categories Global Warming and Acidification Potential had lower scores for cassava compared to corn stover biorefinery. This was mainly due to the higher energy requirements for pretreating lignocellulosic biomass, in order to make sugars available for the subsequent fermentation process.
Emergy outcomes highlighted that the starch-rich feedstock (i.e., cassava peel) was more promising than corn stover from an emergy viewpoint, as it had a lower UEV and a higher renewability percentage. These results showed that the cassava peel biorefinery system is more sustainable both from the user and donor perspectives.
Our results highlight that biorefineries are an option for global bioeconomy strategy as they enable optimization of agricultural and food residues and their environmental performance in producing a renewable substitute for fossil fuels and other non-renewable materials even though some improvements are still necessary for the optimization of the lignocellulosic one such as their coupling with other plants that has residual heat to partially decrease the request of energy for the pre-treatment phase. In this way, agro-industrial processes already demonstrated viable in Europe, (see for instance Jacob, 2006) can represents an opportunity for the progress of developing countries as well.
Data Availability Statement
The datasets generated for this study are available on request to the corresponding author.
Author Contributions
NP, SB, MB, and FS conceived the paper. NP, MB, and FS elaborated and discussed data. MLP, RMP, SB, and ABB supervised the paper. All authors discussed the feedback of the reviewers and contributed to the final manuscript.
Funding
This research was originated within the Biowaste4SP (Turning biowaste in to sustainable products: development of appropriate conversion technologies applicable in developing countries) project, funded by the European Commission's Seventh Framework Programme for Research and Technological Development (FP7/2007–2013); theme KBBE.2012.3.4-01, Conversion of biowaste in developing countries-SICA (African ACP, Mediterranean Partner Countries); grant agreement no: 312111.
Conflict of Interest
The authors declare that the research was conducted in the absence of any commercial or financial relationships that could be construed as a potential conflict of interest.
References
Agostinho, F., and Ortega, E. (2013). Energetic-environmental assessment of a scenario for Brazilian cellulosic ethanol. J. Clean. Prod. 47, 474–489. doi: 10.1016/j.jclepro.2012.05.025
Ahlgren, S., Bjorklund, A., Ekman, A., Karlsson, H., Berlin, J., Borjesson, P., et al. (2015). Review of methodological choices in LCA of biorefinery systems - key issues and recommendations. Biofuels Bioprod. Biorefining 9, 606–619. doi: 10.1002/bbb.1563
Almeida, C. M. V. B., Bonilla, S. H., Giannetti, B. F., and Huisingh, D. (2013). Cleaner production initiatives and challenges for a sustainable world: an introduction to this special volume. J. Clean. Prod. 47, 1–10. doi: 10.1016/j.jclepro.2013.03.010
Balan, V., Chiaramonti, D., and Kumar, S. (2013). Review of US and EU initiatives toward development, demonstration, and commercialization of lignocellulosic biofuels. Biofuels Bioprod. Biorefining 7, 732–759. doi: 10.1002/bbb.1436
Bastianoni, S., Campbell, D. E., Ridolfi, R., and Pulselli, F. M. (2009). The solar transformity of petroleum fuels. Ecol. Model 220, e40–e50. doi: 10.1016/j.ecolmodel.2008.09.003
Bastianoni, S., and Marchettini, N. (1996). Ethanol production from biomass: analysis of process efficiency and sustainability. Biomass Bioenergy 11, 411–418. doi: 10.1016/S0961-9534(96)00037-2
Bastianoni, S., Pulselli, F. M., Castellini, C., Granai, C., Dal Bosco, A., and Brunetti, M. (2007). Emergy evaluation and the management of systems towards sustainability: a response to Sholto Maud. Agric. Ecosyst. Environ. 120, 472–474. doi: 10.1016/j.agee.2006.08.010
Bastianoni, S., Pulselli, F. M., Focardi, S., Tiezzi, E. B., and Gramatica, P. (2008). Correlations and complementarities in data and methods through principal components analysis (PCA) applied to the results of the SPIn-Eco Project. J. Environ. Manage. 86, 419–426. doi: 10.1016/j.jenvman.2006.04.018
Bezergianni, S., and Chrysikou, L. P. (2020). Application of life-cycle assessment in biorefineries. Waste Biorefinery 17, 455–480. doi: 10.1016/B978-0-12-818228-4.00017-4
Borrion, A. L., McManus, M. C., and Hammond, G. P. (2012). Environmental life cycle assessment of lignocellulosic conversion to ethanol: a review. Renew. Sustain. Energy Rev. 16, 4638–4650. doi: 10.1016/j.rser.2012.04.016
Brandt-Williams, S. L. (2002). Handbook of Emergy Evaluation. A Compendium of Data for Emergy Computation Issued in a Series of Folios, Folio #4 (2nd Printing). Gainesville, FL: Emergy of Florida Agriculture, Center for Environmental Policy, University of Florida.
Brown, M. T., and Ulgiati, S. (2016). Assessing the global environmental sources driving the geobiosphere: a revised emergy baseline. Ecol. Modell. 339, 126–132. doi: 10.1016/j.ecolmodel.2016.03.017
Buranakarn, V. (1998). Evaluation of recycling and reuse of building materials using the emergy analysis method. (Ph.D. thesis). Gainesville, FL: University of Florida.
Cabezas, H., Campbell, D., Eason, T., Garmestani, A. S., Heberling, M. T., Hopton, M. E., et al. (2010). San Luis Basin Sustainability Metrics Project: A Methodology for Evaluating Regional Sustainability. Washington, DC: US Environmental Protection Agency.
Campbell, D. E., Brandt-Williams, S. L., and Meisch, M. (2005). Environmental Accounting Using Emergy: Evaluation of the State of West Virginia. Narragansett, RI: US Environmental Protection Agency.
Castellini, C., Bastianoni, S., Granai, C., Dal Bosco, A., and Brunetti, M. (2006). Sustainability of poultry production using the emergy approach: comparison of conventional and organic rearing systems. Agr. Ecosyst. Environ. 114, 343–350. doi: 10.1016/j.agee.2005.11.014
Chen, B., and Chen, G. Q. (2009). Emergy-based energy and material metabolism of the Yellow River basin. Commun. Nonlinear Sci. Numer. Simul. 14, 923–934. doi: 10.1016/j.cnsns.2007.05.034
Cherubini, F., and Strømman, A. H. (2011). Life cycle assessment of bioenergy systems: state of the art and future challenges. Bioresour. Technol. 102, 437–451. doi: 10.1016/j.biortech.2010.08.010
Cohen, M. J., Brown, M. T., and Shepherd, K. D. (2006). Estimating the environmental costs of soil erosion at multiple scales in Kenya using emergy synthesis. Agric. Ecosyst. Environ. 114, 249–269. doi: 10.1016/j.agee.2005.10.021
Ecoinvent (2014). Overview and methodology. Data Quality Guideline For The Ecoinvent Database Version 3. Ecoinvent Database v3. Swiss Centre for Life-cycle Inventories. Dübendorf. Available online at: https://www.ecoinvent.org/files/200712_frischknecht_jungbluth_overview_methodology_ecoinvent2.pdf
European Commission (EC) (2018). A Sustainable Bioeconomy for Europe: Strengthening the Connection Between Economy, Society and the Environment. Brussel: Updated Bioeconomy Strategy.
Fernando, S., Adhikari, S., Chandrapal, C., and Murali, N. (2006). Biorefineries: current status, challenges, and future direction. Energy Fuels 20, 1727–1737. doi: 10.1021/ef060097w
Food and Agriculture Organization of the United Nations (FAO) (2006) Introducing the International Bioenergy Platform (IBEP). Rome: FAO.
Ghatak, H. R. (2011). Biorefineries from the perspective of sustainability: feedstocks, products, and processes. Renew. Sustain. Energy Rev. 15, 4042–4052. doi: 10.1016/j.rser.2011.07.034
Ghisellini, P., Zucaro, A., Viglia, S., and Ulgiati, S. (2014). Monitoring and evaluating the sustainability of Italian agricultural system. An emergy decomposition analysis. Ecol. Model. 271, 132–148. doi: 10.1016/j.ecolmodel.2013.02.014
Gidamis, A., Bastianoni, S., Gustavsson, M., Langat, B., and Saladini, F. (2015). Guidelines for sustainable management of biowaste in Africa. Taastrup: Deliverable 7.3, Biowaste4SP project, 44.
Gnansounou, E., and Alves, C. M. (2019). “Integrated sustainability assessment of biofuels,” in Biofuels: Alternative Feedstocks and Conversion Processes for the Production of Liquid and Gaseous Biofuels, eds A. Pandey, C. Larroche, C.-G. Dussap, E. Gnansounou, S. K. Khanal, and S. Ricke (Amsterdam: Elsevier), 197–214. doi: 10.1016/B978-0-12-816856-1.00008-7
Gnansounou, E., Pachón, E. R., Sinsin, B., Teka, O., Togbé, E., and Mahamane, A. (2020). Using agricultural residues for sustainable transportation biofuels in 2050: case of West Africa. Bioresour. Technol. 305:123080. doi: 10.1016/j.biortech.2020.123080
Gopalakrishnan, G., Negri, M. C., Wang, M., Wu, M., Snyder, S. W., and Lafreniere, L. (2009). Biofuels, land, and water: a systems approach to sustainability. Environ. Sci. Technol. 43, 6094–6100. doi: 10.1021/es900801u
Guinée, J. B., Gorrée, M., Heijungs, R., Huppes, G., Kleijn, R., de Koning, A., et al. (2001). Life CYCLE ASSESSment. An operational Guide to the ISO Standards - Part 2b: Operational Annex, & Part 3: Scientific Background. Holland: Center of Environmental Science (CML), Leiden University.
Gustavsson, J., Cederberg, C., and Sonesson, U. (2011). Global Food Losses and Food Waste. Extent, Causes and Prevention. Study Conducted for the International Congress 'Save Food' at Interpack 2011. Düsseldorf.
Gustavsson, M., Bjerre, A. B., Bayitse, R., Gidamis, A. B., Houssine, B., El-tahlawy, Y., et al. (2014). Catalogue of Biowaste & Bioresidues in Africa. Taastrup: Deliverable 1.3, Biowaste4SP project, 238
International Energy Agency (IEA) (2008). Energy Technology Perspective. Scenario and Strategies to 2050. Paris: IEA.
ISO 14040 and 14044 (2006). Environmental Management - Life Cycle Assessment - Principles and Framework, Goal and Scope Definition and Life Cycle Inventory Analysis,Life Cycle Impact Assessment and Lyfe Cycle Interpretation. Geneva: The International Organization for Standardization.
Jacob, N. B. (2006). Industrial symbiosis in Kalunborg, Denmark: a quantitative assessment of economic and environmental aspects. J. Ind. Ecol. 10, 239–255. doi: 10.1162/108819806775545411
Jensen, K. H., and Thyø, K. A. (2007). 2nd Generation Bioethanol for Transport: the IBUS concept. Boundary conditions and environmental assessment (Master thesis). Department of Manufacturing Engineering and Management Technical University of Denmark, Lyngby, Denmark.
Kamm, B., and Kamm, M. (2004). Principles of biorefineries. Appl. Microbiol. Biotechnol. 64, 137–145. doi: 10.1007/s00253-003-1537-7
Kumar, D., and Singh, B. (2019). Algal biorefinery: an integrated approach for sustainable biodiesel production. Biomass Bioenergy 131:105398. doi: 10.1016/j.biombioe.2019.105398
Lamers, P., Hamelinck, C., Junginger, M., and Faaij, A. (2011). International bioenergy trade - a review of past developments in the liquid biofuel market. Renew. Sustain. Energy Rev. 15, 2655–2676. doi: 10.1016/j.rser.2011.01.022
Le, L. T., van Ierland, E. C., Zhu, X., and Wesseler, J. (2013). Energy and greenhouse gas balances of cassava-based ethanol. Biomass Bioenergy 51, 125–135. doi: 10.1016/j.biombioe.2013.01.011
Leng, R., Wang, C., Zhang, C., Dai, D., and Pu, G. (2008). Life cycle inventory and energy analysis of cassava-based Fuel ethanol in China. J. Clean. Prod. 16, 374–384. doi: 10.1016/j.jclepro.2006.12.003
Lu, H., Bai, Y., Ren, H., and Campbell, D. E. (2010). Integrated emergy, energy and economic evaluation of rice and vegetable production systems in alluvial paddy fields: implications for agricultural policy in China. J. Environ. Manage. 91, 2727–2735. doi: 10.1016/j.jenvman.2010.07.025
Markeviius, A., Katinas, V., Perednis, E., and Tamaauskien, M. (2010). Trends and sustainability criteria of the production and use of liquid biofuels. Renew. Sustain. Energy Rev. 14, 3226–3231. doi: 10.1016/j.rser.2010.07.015
Morales, M., Quintero, J., Conejeros, R., and Aroca, G. (2015). Life cycle assessment of lignocellulosic bioethanol: Environmental impacts and energy balance. Renew. Sustain. Energy Rev. 42, 1349–1361. doi: 10.1016/j.rser.2014.10.097
Muñoz, I., Flury, K., Jungbluth, N., Rigarlsford, G. I, Canals, L. M., et al. (2014). Life cycle assessment of bio-based ethanol produced from different agricultural feedstocks. Int. J. Life Cycle Assess. 19, 109–119. doi: 10.1007/s11367-013-0613-1
Niccolucci, V., Pulselli, R. M., Focardi, S., and Bastianoni, S. (2010). “Integrated indicators for evaluating ecosystem health: an application to agricultural systems,” in Handbook of Ecological Indicators for Assessment of Ecosystems Health, (Raton, FL: Taylor & Francis Boca), 421–441. doi: 10.1201/EBK1439809365-c17
Nizami, A. S., Rehan, M., Waqas, M., Naqvi, M., Ouda, O. K. M., Shahzad, K., et al. (2017). Waste biorefineries: enabling circular economies in developing countries. Bioresour. Technol. 241, 1101–1117. doi: 10.1016/j.biortech.2017.05.097
Nzila, C., Dewulf, J., Spanjers, H., Kiriamiti, H., and van Langenhove, H. (2010). Biowaste energy potential in Kenya. Renew. Energy 35, 2698–2704. doi: 10.1016/j.renene.2010.04.016
Odum, H. T. (1996). Environmental Accounting. Emergy and Environmental Decision Making. New York, NY: John Wiley & Sons.
Odum, H. T., and Odum, E. C. (2006). The prosperous way down. Energy 31, 21–32. doi: 10.1016/j.energy.2004.05.012
Okello, C., Pindozzi, S., Faugno, S., and Boccia, L. (2013). Bioenergy potential of agricultural and forest residues in Uganda. Biomass Bioenergy 56, 515–525. doi: 10.1016/j.biombioe.2013.06.003
Patrizi, N., Caro, D., Pulselli, F. M., Bjerre, A. B., and Bastianoni, S. (2013). Environmental feasibility of partial substitution of gasoline with ethanol in the Province of Siena (Italy). J. Cleaner Prod. 47, 388–395. doi: 10.1016/j.jclepro.2012.11.023
Patrizi, N., Pulselli, F. M., Morandi, F., and Bastianoni, S. (2015). Evaluation of the emergy investment needed for bioethanol production in a biorefinery using residual resources and energy. J. Clean. Prod. 96, 549–556. doi: 10.1016/j.jclepro.2014.03.079
Pereira, C. L. F., and Ortega, E. (2010). Sustainability assessment of large-scale ethanol production from sugarcane. J. Clean. Prod. 18, 77–82. doi: 10.1016/j.jclepro.2009.09.007
Pieragostini, C., Aguirre, P., and Mussati, M. C. (2014). Life cycle assessment of corn-based ethanol production in Argentina. Sci. Total Environ. 472, 212–225. doi: 10.1016/j.scitotenv.2013.11.012
Pordesimo, L. O., Edens, W. C., and Sokhansanj, S. (2004). Distribution of aboveground biomass in corn stover. Biomass Bioenergy 26, 337–343. doi: 10.1016/S0961-9534(03)00124-7
PRé Consultants (2016). Introduction to LCA with SimaPro. Available online at: www.pre-sustainability.com/download/SimaPro8IntroductionToLCA.pdf (accessed October, 2019).
Pulselli, R. M., Simoncini, E., Ridolfi, R., and Bastianoni, S. (2008). Specific emergy of cement and concrete: an energy-based appraisal of building materials and their transport. Ecol. Indic. 8, 647–656. doi: 10.1016/j.ecolind.2007.10.001
Rathnayake, M., Chaireongsirikul, T., Svangariyaskul, A., Lawtrakul, L., and Toochinda, P. (2018). Process simulation based life cycle assessment for bioethanol production from cassava, cane molasses, and rice straw. J. Cleaner Prod. 190, 24–35. doi: 10.1016/j.jclepro.2018.04.152
Raugei, M., Bargigli, S., and Ulgiati, S. (2006). “Nested emergy analyses: moving aheadfrom the spreadsheet platform,” in Presented at 4th Biennial Emergy Analysis and Research Conference, Gainesville, FL: University of Florida.
Raugei, M., Rugani, B., Benetto, E., and Ingwersen, W. W. (2014). Integrating emergy into LCA: potential added value and lingering obstacles. Ecol. Model. 271, 4–9. doi: 10.1016/j.ecolmodel.2012.11.025
Rosen, M. A. (2018). Environmental sustainability tools in the biofuel industry. Biofuel Res. J. 17 751–752. doi: 10.18331/BRJ2018.5.1.2
Rugani, B., and Benetto, E. (2012). Improvements to emergy evaluations byusing life cycle assessment. Environ. Sci. Technol. 46,4701–4712. doi: 10.1021/es203440n
Saladini, F., Vuai, S. A., Langat, B. K., Gustavsson, M., Bayitse, R., Gidamis, A. B., et al. (2016). Sustainability assessment of selected biowastes as feedstocks for biofuel and biomaterial production by emergy evaluation in five African countries. Biomass Bioenergy. 85, 100–108. doi: 10.1016/j.biombioe.2015.11.016
Seghetta, M., Østergård, H., and Bastianoni, S. (2014). Energy analysis of using macroalgae from eutrophic waters as a bioethanol feedstock. Ecol. Modell. 288, 25–37. doi: 10.1016/j.ecolmodel.2014.05.006
Sims, R., Taylor, M., Jack, S., and Mabee, W. (2008). From 1st to 2nd Generation Biofuel Technologies. An Overview of Current Industry and RD&D Activities. Paris: International Energy Agency (IEA).
Solomon, B. D. (2010). Biofuels and sustainability. Ann. N. Y. Acad. Sci. 1185, 119–134. doi: 10.1111/j.1749-6632.2009.05279.x
Thomsen, S. T., Kádár, Z., and Schmidt, J. E. (2014). Compositional analysis and projected biofuel potentials from common West African agricultural residues. Biomass Bioenergy 63, 210–217. doi: 10.1016/j.biombioe.2014.01.045
Ubando, A. T., Felix, C. B., and Chen, W. H. (2020). Biorefineries in circular bioeconomy: a comprehensive review. Bioresour. Technol. 299:122585. doi: 10.1016/j.biortech.2019.122585
Ulgiati, S., Odum, H. T., and Bastianoni, S. (1993). “Emergy analysis of Italian agricultural system. The role of energy quality and environmental inputs,” in Trends in Ecological Physical Chemistry (Elsevier Amsterdam), 17–215. doi: 10.1016/0304-3800(94)90064-7
United Nations (UN) (2015a). “Adoption of the paris agreement,” in Conference of the Parties (COP) (New York, NY), FCCC/CP/2015.
Yang, H., Chen, L., Yan, Z., and Wang, H. (2011). Emergy analysis of cassava-based fuel ethanol in China. Biomass Bioenergy 35, 581–589. doi: 10.1016/j.biombioe.2010.10.027
Zabed, H., Sahu, J. N., Boyce, A. N., and Faruq, G. (2016). Fuel ethanol production from lignocellulosic biomass: an overview on feedstocks and technological approaches. Renew. Sustain. Energy Rev. 751–774. doi: 10.1016/j.rser.2016.08.038
Keywords: biorefinery, food waste, organic residues, circular economy, biomass, bioethanol
Citation: Patrizi N, Bruno M, Saladini F, Parisi ML, Pulselli RM, Bjerre AB and Bastianoni S (2020) Sustainability Assessment of Biorefinery Systems Based on Two Food Residues in Africa. Front. Sustain. Food Syst. 4:522614. doi: 10.3389/fsufs.2020.522614
Received: 23 December 2019; Accepted: 26 August 2020;
Published: 06 October 2020.
Edited by:
José Martinez, National Research Institute of Science and Technology for Environment and Agriculture (IRSTEA), FranceReviewed by:
S. Venkata Mohan, Indian Institute of Chemical Technology (CSIR), IndiaSylvestre Njakou Djomo, Institut National de Recherche pour l'agriculture, l'alimentation et l'environnement (INRAE), France
Copyright © 2020 Patrizi, Bruno, Saladini, Parisi, Pulselli, Bjerre and Bastianoni. This is an open-access article distributed under the terms of the Creative Commons Attribution License (CC BY). The use, distribution or reproduction in other forums is permitted, provided the original author(s) and the copyright owner(s) are credited and that the original publication in this journal is cited, in accordance with accepted academic practice. No use, distribution or reproduction is permitted which does not comply with these terms.
*Correspondence: Riccardo M. Pulselli, cHVsc2VsbGlAdW5pc2kuaXQ=