- Soil and Water Sciences Department, Institute of Food and Agricultural Sciences, University of Florida, Gainesville, FL, United States
Agricultural wastes from plant and animal operations are often land applied to recycle and manage residues. Compositional variability among these wastes is vast. Some waste components can potentially represent a threat to the environment and humans depending on their nature, application loads, and soil type. Biochar, the product obtained by biomass heating under oxygen-limited conditions, has the potential to minimize risks associated with waste characteristics while promoting soil health. However, variation in the residue wastes (feedstocks) used to produce biochar carries over to the resultant biochar. In this study, we compare the chemistry and composition of biochars representing two broadly defined sources—animal (poultry litter, biosolids) and plant (mixed hardwoods, pure maple, pine)—and highlight phosphorus (P) management implications and opportunities presented by the variability among them. We also evaluate P leaching patterns of four selected biochars (poultry litter, biosolids, hardwoods, and maple) as applied at an identical rate (1% w/w) to two soils differing in P retention capacities. Cumulative P release following poultry litter biochar application and 20 leaching events was much lower for the more P retentive soil. Total P release from biochar-amended soils did not differ between soils when biochar from biosolids or from plant sources were used as the soil amendment. However, the biosolids-biochar released higher levels of P initially from the Candler compared with the Apopka (more P retentive than the Candler) soils, similar to that of poultry litter biochar. Compositional variations in feedstocks and resultant biochars must be understood in order to judiciously use them as crop nutrient amendments. There is potential to minimize nutrient deficiencies and environmental liabilities of biochars by matching feedstocks, or mixtures of feedstocks, to the needs of specific crops, and by considering the P retentive capacity of the soil where the biochar is applied.
Introduction
According to the International Biochar Initiative (IBI, 2003), biochar is the term used to describe the material obtained by the thermochemical conversion of organic matter in the complete absence or low oxygen environment through a process called pyrolysis. Biochar can be used for improving soil fertility when used alone or blended with a range of other materials, environmental/soil remediation, sequestering carbon (C), and so mitigating climate change (IBI, 2003; Lehmann et al., 2006). Biochar origin relates to the discovery of Amazonian Dark Earths, or Terra Preta de Índio, soils that are higher in soil organic matter (reason for its dark color), pH and base saturation compared to the surrounding soils (Cunha et al., 2009). They are believed to be the result of anthropogenic activities such as slash/burn and bury, dating back from 500 to 2500 years B.P. (Smith, 1980; Woods et al., 2000). Amazonian Dark Earths are so highly fertile that it enables continuous cultivation without fertilization, differing from the surrounding soils that are generally infertile, typical of the tropical regions. The discovery of these fertile soils led to research on the use of biochar as a nutrient source (Ippolito et al., 2015).
In agricultural farms, particularly under tropical conditions, there are several wood and straw-based “waste products” such as pruned branches and tree limbs, maize, rice, and wheat straw (Nair et al., 2017). Further, in animal-production areas, organic wastes such as manure from poultry, dairy and beef cattle, and swine need to be removed off-site or land-applied. Manure sources tend to lose nutrients from soil, particularly phosphorus (P), and could result in polluting the environment when land applied (Nair et al., 2003). If these farm wastes can be recycled as biochar and managed as a soil amendment, it would be a win-win situation decreasing the need for commercial fertilizer application while utilizing the waste products (Nair et al., 2017).
Agronomic benefits of biochar application are highly variable; some studies show positive, neutral, and even negative effects of biochar application in crop productivity (Sohi et al., 2009; Jeffery et al., 2011) that could be explained by different chemical and physical characteristics of the biochar. The effects of biochar as an amendment is highly dependent on the elemental content of the raw material from which the biochar was obtained (Chan and Xu, 2009; Hossain et al., 2011; Wang et al., 2012). Biochar properties also vary both as a consequence of conditions under which they are produced (e.g., pyrolysis temperature, residence time) (Sohi et al., 2009) but also due to the variability among distinct feedstocks from which they are produced (Mašek et al., 2018). The soil to which the biochar is added also influences the P availability as it has its own P retention capacity (Dari et al., 2016). The biochar can compete with the soil for P binding sites or can be the P source that could be bound to the soil sorption sites. Therefore, P release and availability is a function of the feedstock and the soil as shown by Dari et al. (2016) in their isotherm studies. There is a need to address the P availability from biochar-amended soils with differing P retention capacities. Determining the environmentally safe quantity of a biochar that can be added to a given soil for agronomic purposes requires an understanding of the P release behavior of the biochar-soil system.
Our overarching objective was to compare some of the basic properties of biochars from animal- and plant-based feedstocks and examine implications for their judicious use as a soil amendment to explain positive, neutral, and negative effects of biochar application in crop productivity reported in literature. Specific objectives were to: (i) evaluate the chemical properties of plant-based (mixed hardwoods, pure maple, pine) and animal-based (poultry litter and biosolids) feedstocks using identical methodology and, (ii) determine the mineralogy of the biochars via x-ray diffraction (XRD) and scanning electron microscopy with energy dispersive spectroscopy (SEM-EDS) to obtain evidence of P associations with other elements in the biochar from different feedstocks, and thereby provide an insight into the P release potential of biochars from these feedstocks, (iii) evaluate phosphorus release patterns when biochars from different feedstocks applied at same rate are mixed with distinct P retentive soils.
Materials and Methods
Biochar Characterization
Various animal- and plant-based biochar feedstocks were used in this study. For animal-based biochars, biosolids, and poultry litter (PLB1 and PLB2; commercially available products from different batches) were selected. The plant-based feedstocks included mixed hardwoods (manufacturer's product labeled as HWB), pure maple (Acer spp.), and Douglas-fir (Psuedotsuga menziesii), referred to as pine in this manuscript. The HWB feedstock consisted wood from the following trees: maple (Acer spp.), oak (Quercus spp.), and pine (Pinus spp.) slowly pyrolyzed at 650–700°C (Bakshi et al., 2014; Dari et al., 2016). The animal sources PLB1 and PLB2 are two different batches of poultry litter biochar obtained from a commercial source (Frye Poultry). They consist of granular forms of activated biochar obtained from broiler manure and bedding material used in poultry operations that include saw dust, straw, and other materials as described by Lima et al. (2014). The feedstock was also characterized by Lima and Marshall (2005). PLB1 and PLB2 were produced through pyrolysis at 700°C under nitrogen gas flow for 1 h followed by 45-min steam activation at 800°C at water flow rates ranging from 1 to 5 mL min−1 (Lima et al., 2014; Dari et al., 2016). Phosphorus release at environmentally-relevant P concentration is independent of the temperature of biochar preparation as shown by Nair et al. (2016) justifying the use of materials produced under different temperatures in this study. Further, farmers and others use biochar as a nutrient source in their fields without an understanding of differences in properties of the biochar which would affect its value as a nutrient source.
Biosolids material was obtained from a municipal wastewater treatment facility in Jacksonville, Florida, U.S.A. (30.3322° N, 81.6557° W). This material originated from an aerobic digestion process and was subsequently classified as A (classification of virtually pathogen free as regulated by the Environmental Protection Agency, EPA). Biochar from the biosolids was prepared in a muffle furnace. The feedstock (biosolids) was crushed with a mortar and pestle, sieved through a 2 mm screen and heated for 24 h in a muffle furnace at 105°C to determined moisture content. After cooling off, the material was placed in a ceramic crucible and the weight was recorded. The crucible containing the feedstock was covered with a loose-fitting ceramic lid and subjected to pyrolysis for 2.5 h at 400°C (Gonzaga et al., 2017).
Solid State Characterization
All biochar samples were subjected to XRD analysis to identify the minerals present in the biochar from varying feedstocks. Samples were ground using an agate mortar and pestle, passed through a 500 μm sieve, and subsequently placed and leveled in a cavity mount designed for this specific analysis. Samples were scanned from 2 to 60° 2θ at rate of 2° per min using Cu Kα radiation. The scans were performed on a computer-controlled x-ray diffractometer (Ultima IV X-Ray Diffractometer, Rigaku Corporation, Japan) equipped with stepping motor and graphite crystal monochromator. Scanning electron microscopy (SEM) analyses, in conjunction with energy-dispersive x-ray fluorescence elemental spectroscopy (EDS), were also conducted on samples. Samples for SEM were prepared on a carbon stub by applying small portions of the biochar and then coating with an ultra-thin C layer to conduct electrons from the sample surface.
Elemental Composition Characterization
Biochars were homogenized and sieved to pass through a 2 mm sieve before analysis. Mehlich 3 (M3-) extractable P, K, Ca, Mg, Zn, Mn, Cu, Fe, Al, Ni, and Na were analyzed using ICP (Inductively Coupled Plasma Spectrometry). Water soluble P (WSP) was determined in double deionized water using 1:10 soil:solution ratio extraction and analyzed by colorimetric method (Murphy and Riley, 1962) using a Technicon™ Autoanalyzer (EPA 365.1). Electrical conductivity (EC) and pH were determined in deionized water at 1:2 biochar:water ratio.
Total P (TP) was determined by ashing 1 g of soil for 2 h at 550°C and then solubilizing with 6 M HCl (Andersen, 1975). Total Kjeldahl nitrogen was analyzed according to EPA 351.2 and total organic carbon (TOC) by dry combustion at 900°C with Shimadzu total organic carbon and nitrogen analyzer (TOC-VCPH and TNM-1 connected to SSM5000) (Shimadzu Corp. Kyoto, Japan).
Soil Characterization
Two minimally P-impacted soils from Florida were used in the P release from biochar-amended soil studies. The more P retentive one is from a Bt horizon of the Apopka soil series (Loamy, siliceous, subactive, hyperthermic grossarenic Paleudults). The other one with lower P retention capacity is from an E horizon of the Candler soil series (Hyperthermic, uncoated Typic Quartzipsamments). Soil samples were randomly collected from the sites, mixed, and homogenized to get a composite sample (Dari et al., 2016). Soil samples were then air-dried and passed through a 2 mm sieve. Samples were subjected to various physical-chemical analyses and used for the P release experiments (Table 3).
P Release From Soil-Biochar Mixtures
The P release measurements in soils amended with biochars (PLB, Biosolids, HWB, and Maple) were performed after mixing the biochars with the minimally-impacted soils specified above. Selected biochars were added to the soils at 1% w/w (1.98 g of soil + 0.02 g of biochar). All experiments were conducted in triplicate. The soil-biochar mixture was added to a glass vial with 10 mL of 0.01 M KCl, which was then sealed and left for incubation for 20 days at room temperature (25C ± 1°C) to attain chemical equilibrium. The number of days for incubation was chosen after a series of pH measurements indicating that equilibrium had been reached for all biochar-soil mixtures well within 20 days. After the incubation period, mixtures were transferred to polystyrene filter units, fitted with inert filter membranes of 0.45 μm pore size (Supplementary Figure 1). Samples were then washed 20 times with 20 mL of 0.01 M KCl each time. Extractants was analyzed for soluble P (leached P) by an autoanalyzer (Kopp and McKee, 1979) following the Murphy and Riley (1962) procedure. All extractions and determinations were at room temperature (25C ± 1°C).
Statistical Analyses
Each sample was considered a replicate within a group according to its source (animal or plant-based biochars) for statistical purposes. ANOVA was performed to test differences in P parameters between those groups. Experimental treatments involving P release from soil-biochar mixtures were done in triplicates; means and standard deviations were calculated for each mixture. All statistical calculations were performed using RStudio 1.3.1056.
Results and Discussion
Phosphorus in Biochars
Selected chemical characteristics for biochar feedstocks are presented in Tables 1, 2. Values of P parameters of animal-based were statistically higher than those of plant based-biochars (by ANOVA test), with p = 0.013, 0,029, 0.035 for M3-P, WSP, and total P respectively, and are generally similar to results of other studies (Lehmann et al., 2006; Singh et al., 2010). Singh et al. (2010), for instance, reported 1,555–2,446 mg kg−1 of Olsen P in the poultry litter biochar in their studies, whereas our study indicates 13,000–17,000 mg kg−1 of M3-P. Bedding materials and their proportion (e.g., wood shaving, straw), concentration of other chemical elements, and type of chemical extraction applied during P analysis could be responsible for such discrepancy. Therefore, biochar from the same nominal feedstock but from different sources or batches could have different P release properties resulting in different yields when applied at identical rates of biochar to the same soil. A recent meta-analysis by Glaser and Lehr (2019) could not find information regarding relations between biochar TP content and plant-available P in their analysis of biochar experiments from across the world. In our current study, available P measured as M3-P, accounted for 45% of the TP from PLB1 and 60% from PLB2 (Tables 1, 2) compared to 10% of the TP from biosolids-biochar.
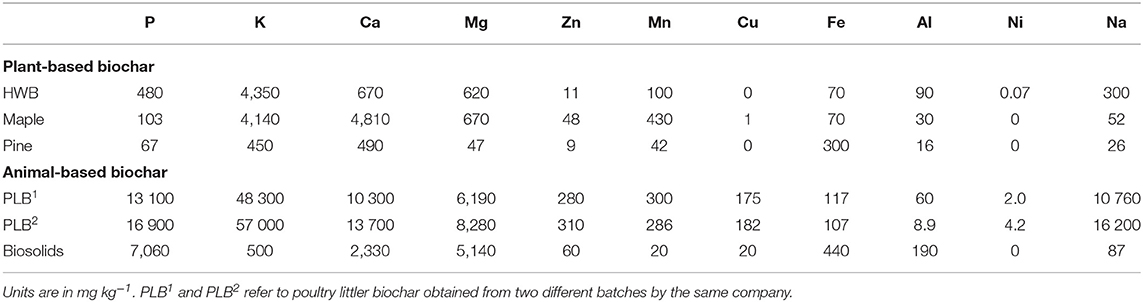
Table 1. Composition of biochar from plant- and animal-based feedstocks based on Mehlich-3 extraction.
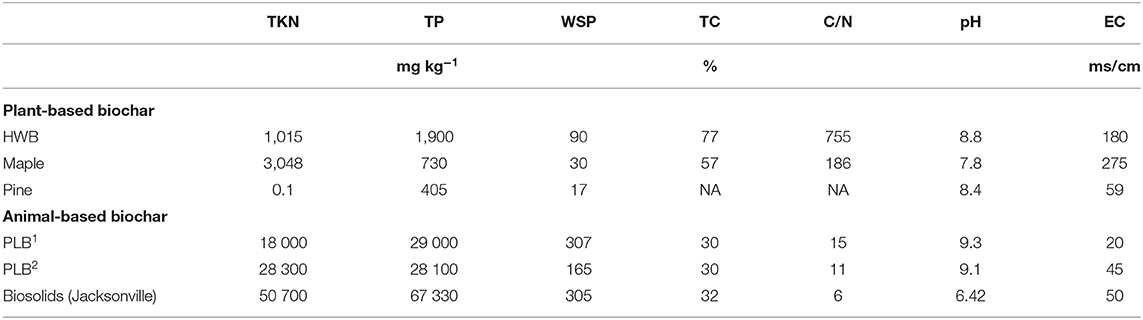
Table 2. Total Kjeldahl nitrogen (TKN), total P (TP), water soluble P (WSP), total carbon (TC), pH and electrical conductivity (EC) for plant- and animal-based biochars.
Plant available P in the biochar feedstocks, measured as M3-P, in ascending order is as follows: pine < maple < HWB < biosolids < PLB1,2; the relationship for TP concentration was pine < maple < HWB < PLB1,2 < biosolids (Table 2). Although biosolids-biochar had double the amount of TP compared with PLB1,2, it had only about half the available P of PLB1,2. The greater recalcitrance of M3-P in the biosolids biochar than in the PLB1,2 could be related to the higher stability of its mineral composition. In some other cases, however, low solubility of P in the biosolids-biochar could be due to the concentration of other elements that have the potential to interfere in its availability, i.e., Al and Fe, purposely used in the process of biosolids stabilization to decrease P solubility. Doing so, the land application of this kind of biosolids reduces environmental risk without compromising crop production (Huang and Shenker, 2004; Huang et al., 2007). The total concentration of a certain element, therefore, is not always a measure of nutrient availability for plants and should not be taken as the only criterion used for nutrition management (Ippolito et al., 2015).
Biosolids can be obtained by various processes. The labile P fraction of biosolids varies greatly among the different stabilization processes (Brandt et al., 2004). In a study that evaluated the conversion of different biosolids into biochar, Freitas et al. (2017) revealed the elemental and mineral content distinction varied among biosolids and their corresponding biochars. Although the biosolids biochar in this current study had a TP content of 67,300 mg kg−1, no crystalline phase of a phosphate mineral was identified through XRD (Figure 1). Qian and Jiang (2014), also using XRD techniques to assess the influence of temperature in the P transformation during thermal treatment of biosolids, detected in the biochar only the phosphate mineral stanfieldite, Ca4(Mg, Fe)5(PO4)6. However, compounds of orthophosphate and pyrophosphate made up a big portion of TP in the feedstock. They speculated the P could be adsorbed on Al, Fe, or Ca, just like in soils (McDowell and Condron, 2000; Sharpley et al., 2004; Qian and Jiang, 2014). Since biosolids differ in their characterization based on their treatment processes (Freitas et al., 2017; Silveira et al., 2019), associations with P could also differ depending on the biosolids source.
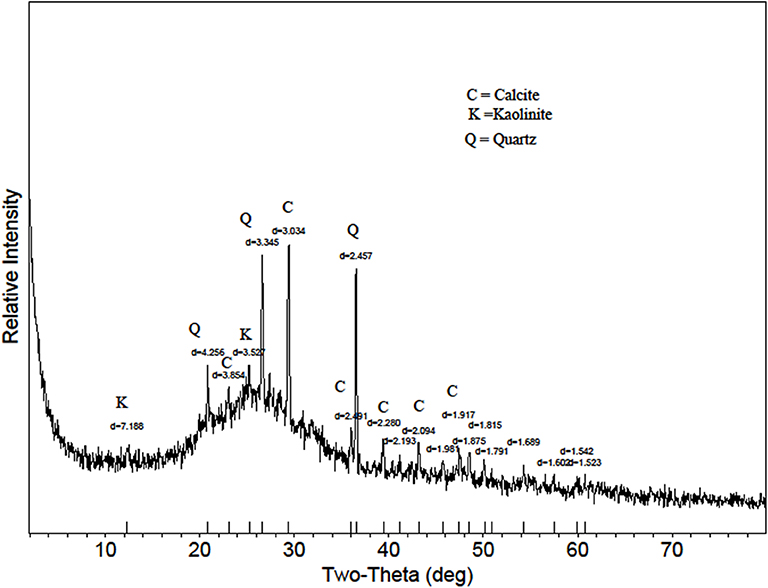
Figure 1. X-ray diffraction pattern of a biosolids biochar from Jacksonville, FL. Calcite = CaCO3; Kaolinite = Al2Si2O5(OH)4; Quartz = SiO2.
The biosolids-biochar in this study showed Mg-P associations (Figure 2) in SEM-EDS assessment, consistent with the presence of struvite (MgNH4PO4·H2O) that was identified in the biosolids feedstock (Freitas et al., 2017). Minerals identified in the biosolids biochar included calcite, kaolinite, and quartz; struvite was not present, despite being in the feedstock, likely due to its thermal destabilization during the pyrolysis process (Bhuiyan et al., 2008). The phosphate mineral whitlockite, β-Ca3(PO4)2, was identified in the PLB1 (Dari et al., 2016) and PLB2 (Figure 3). These results were later corroborated by SEM-EDS that showed Ca-P associations (Figure 4). This secondary phosphate mineral has also been identified in dairy manure biochar produced at temperatures ≥ 500°C (Cao and Harris, 2010). It has the potential to be a slow P-release fertilizer due the steady dissolution of the mineral. Wang et al. (2015) studying P dissolution from poultry litter biochar, speculated that the slow-release pattern of P, along with 1:1 Mg:P ratio from leachate, were due to struvite (Manning, 2008; Wang et al., 2015), although the XRD assessment in our studies did not show the presence of this mineral. The thermal instability of struvite should preclude its presence as a crystalline phase in biochar during the heat treatment. Solid state characterization enables mineral identification and consequently provides insight into the release behavior of several elements with important agronomic and environmental implications. For instance, the solubility of whitlockite (Li et al., 2009; Magalhães and Costa, 2018) is lower than that of the struvite (Bhuiyan et al., 2007), suggesting that a biochar containing the former mineral would potentially release P less freely than the latter. However, dissolution rates would need to be determined to directly confirm this difference.
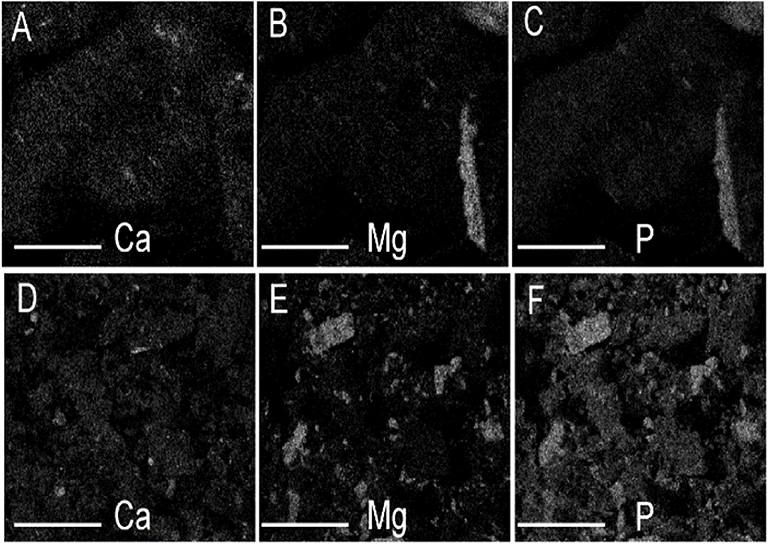
Figure 2. Elemental dot map from SEM-EDS analysis of Jacksonville biosolids (A–C) and its corresponding biochar (D–F), showing Mg-P associations (scale bar = 200 μm).
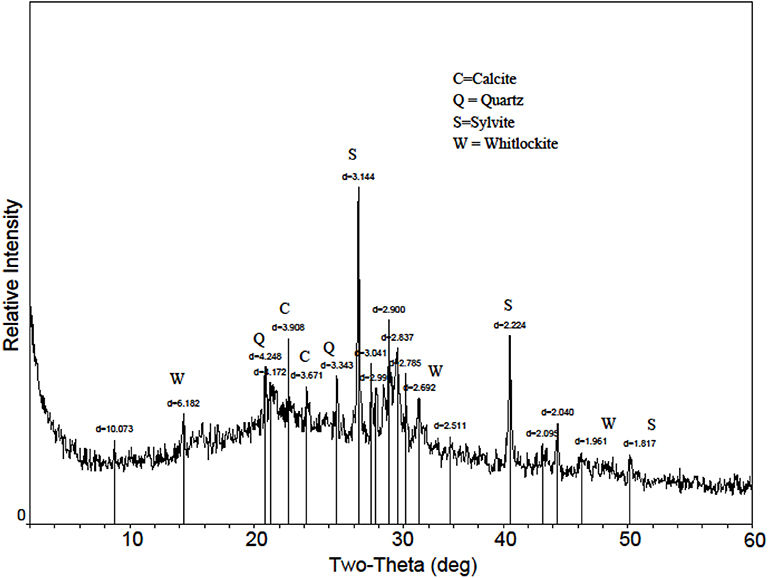
Figure 3. X-ray diffraction pattern of Poultry Litter Biochar2 (PLB2). Calcite = CaCO3; Quartz = SiO2; Sylvite = KCl; Whitlockite = (β-Ca3(PO4)2).
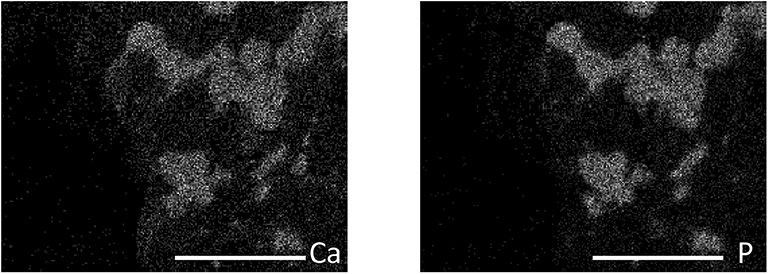
Figure 4. Elemental dot map from SEM-EDS analysis of PLB2 showing Ca-P associations (scale bar = 20 μm).
Other Physical and Chemical Properties of Biochars
The high pH values determined for PLB1 and PLB2 (Table 2) are similar to those reported by Chan et al. (2008) and Gaskin et al. (2008). The high pH is due to elevated concentration of bases in manure compared to plant residues (Table 1). This finding suggests that PLB1 and PLB2 could have a liming effect when applied to the soil, which is confirmed by the presence of calcite in both PLB1 (Dari et al., 2016) and PLB2 (Figure 3). Domingues et al. (2017) showed high liming values for chicken manure biochar and coffee husk when comparing these materials with other biochars: eucalyptus sawdust, sugarcane bagasse, and pine bark. They credit these high liming values to the presence of calcium- and potassium carbonates found in the XRD spectra of those biochars. Maple biochar also seems to have a liming effect if land applied due to the presence of calcite (Supplementary Figure 2), consistent with its alkaline pH of 7.8 (Table 2). X-ray diffraction patterns of the HWB in our study showed the presence of sylvite (Supplementary Figure 3) consistent with high amount of K in this biochar feedstock (Table 1).
High base concentrations, especially potassium (K) and sodium (Na), in PLB1 and PLB2 (Table 1) are reported to have a negative effect on plant growth when applied to a poorly buffered soil, due to increase in EC (Macdonald et al., 2014). Studies have reported increases in soil salinization and consequently negative effects on plant growth by the application of animal manure and/or corresponding biochar to the soils (Yao et al., 2007; Macdonald et al., 2014; Rombola et al., 2015). However, if poultry litter biochar is field applied on a P basis, just a small amount of the material would be necessary, reducing the salinization risk associated with its application. Moreover, the apparently steady P release from this material also suggests that the risk of P leaching and runoff from the field application could be minimized. According to Dari et al. (2016), PLB could still adsorb P from the system, despite the high content of this nutrient in the material, and thus could also be used for soil remediation.
Higher concentrations of M3-extractable P, K, and Mg for HWB and maple compared to pine were noted (Table 1). Maple biochar, HWB, PLB1, and PLB2 had higher concentrations of K (>10-fold in magnitude) compared to pine- and biosolids biochar, indicating not only significant variability among plant- but also animal-based biochars. High solubility of K in PLB1 and PLB2 could be attributed to the presence of sylvite (KCl) (Figure 3). Neither maple biochar (Supplementary Figure 2) nor pine biochar (Supplementary Figure 4), indicated the presence of sylvite, a mineral noted to be especially common in plant-based biochars (Zhao et al., 2013). This mineral was only detected in the HWB (Supplementary Figure 4) among the plant-based biochars in our study. The high concentration of Ca in maple biochar is due to the presence of calcite (CaCO3) (Supplementary Figure 2) in the material. Some studies (Singh et al., 2010; Zhao et al., 2013) suggest that more calcite is formed when pyrolysis temperature is increased to 500°C and believed to be the result of decomposition of calcium oxalate into calcite. No crystalline phase was detected in the pine biochar (Supplementary Figure 4), nor were elemental associations evident through SEM-EDS (figure not shown). Although pine biochar did not present appreciable nutritional value, it has been reported to increase water holding capacity (Yu et al., 2013). Our results bring to light the high elemental variability and the need to evaluate biochar from different feedstocks prior to land application. Additionally, they corroborate other studies showing that if the selected materials were to be used as a P fertilizer, much more of plant-based would be necessary to meet the crop requirements compared to the animal-based biochars.
Among the biochars in this study, PLB1 and PLB2 presented the highest content of the main micronutrients (Zn, Mn, Cu) (Table 1), in accordance with other studies (Macdonald et al., 2014). The low C/N ratio from this biosolids-biochar (Table 2) suggests that it could be a promising material to serve as an N fertilizer (Hossain et al., 2011); among our studied materials, biosolids-biochar had the highest total Kjeldahl nitrogen (TKN) content. However, with the high variability in properties of biosolids-biochar from different processing techniques, we cannot consider all biosolids-biochar to have similar effects on land-application. Freitas et al. (2017) indicated TKN content ranging from 13,000 to 50,000 in different biosolids-biochar tested in their study.
Various studies from 1850 to 2011 reported variable effects of biochar on plant yield: 50% reported positive, 20% no effect, and 30% reported negative effects (Maddox, 2013). These findings are not surprising given the huge variation in biochar properties noted above. Differences in soil P storage capacity (Nair et al., 2010) is another important factor to be taken into consideration regarding the application rate of the biochar. A system with high soil P retention capacity would require more of a given biochar to offset the soil P fixation. Dari et al. (2016) showed that nutrient retention and release during biochar application depends not only on the nutrient retention/release potential of the biochars, but also the nutrient retention properties of the soil. A meta-analysis of biochar effects on P availability when applied to soils, reported no observed effect on P bioavailability from wood-based biochar feedstocks, suggesting their ineffectiveness as a P fertilizer (Glaser and Lehr, 2019). However, several studies indicated that biochar from wood-based feedstocks can serve as a soil amendment by increasing cation exchange capacity and pH (Yamato et al., 2006; Van Zwieten et al., 2010; Nelson et al., 2011), which in turn could indirectly increase P availability and improve crop yields (Yamato et al., 2006; Asai et al., 2009; Nelson et al., 2011).
Micronutrient content is another possible factor influencing the disparities in reported yield. It is clear, then, that the amount of biochar required to meet a given crop requirement would vary substantially according to each nutrient demand, and biochar application rates will be site-specific (Nair et al., 2017). Discrepancies in nutrient content between biochar feedstocks call for the mixture of different available feedstocks as a possible alternative (Nair et al., 2017). Biochars with high C/N ratio, such as those from plant-based feedstocks (Table 2), still have the potential to act as a soil conditioner and to enhance N use efficiency when mixed with animal manure (Steiner et al., 2008, 2015; Wang et al., 2019), and should still be considered despite low nutritional value. Nair et al. (2017) suggest a mixture of different available feedstocks could be very important under smallholder farming systems, that can utilize agricultural residues to create a valuable product to achieve sustainable crop production. It seems reasonable, therefore, to critically select biochar feedstocks that provide nutrient balanced content for a field application. Those considerations should be properly addressed along with possible environment constraints. A knowledge of elemental composition enables the identification of biochars, including mixtures from different feedstocks, that would be most effective for a specific purpose. The focus of this study was on characteristics of biochar from different feedstocks. Findings will have implications for biochar applications in different parts of the world.
Phosphorus Release From Soil-Biochar Mixtures
The physico-chemical properties of the two soils—Candler and Apopka—are given in Table 3. Apopka had much higher P retentive capacity compared to Candler (Dari et al., 2016). The difference in P retention capacity affected the release pattern in some cases. For instance, cumulative P release, as %WSP, from PLB1 + Apopka mixture was a little over half that of the PLB1 + Candler mixture (Table 4; Figure 5). In this particular case, the P retentive potential of the soil played a role on the P release and demonstrated the importance of accounting for the soil P retention capacity for a safe amendment application to the soil. Furthermore, the proper application rate of the amendment will also provide adequate P for plant uptake while minimizing a risk to the environment. Not all animal-based biochar would yield similar results, however. In the case of biosolids-biochar, no difference was seen in the cumulative P leached after 20 extractions of this biochar amended with Apopka and Candler that were 214 and 215 mg kg−1, respectively (Figure 6). However, the first biosolids-biochar leaching event resulted in the maximum leached P similar to P leaching from the PLB and showed greatest P retention for the Apopka soils (Figure 5). After 20 days' incubation, the biochar-soil P had likely reached a quasi-equilibrium to enable maximum expression of a soil effect. The P released from subsequent leaching events is likely lower because of less equilibration time and possible depletion of the most soluble P phases during the first event. Release of P from Apopka and Candler soils amended with HWB and maple biochar did not differ despite differences in P retention capacities of these soils (Table 3). Based on chemical and solid-state characteristics, plant-based biochars are not susceptible to high P release due to the low concentration of this nutrient; pine had the lowest P content and was left out of the leaching studies.
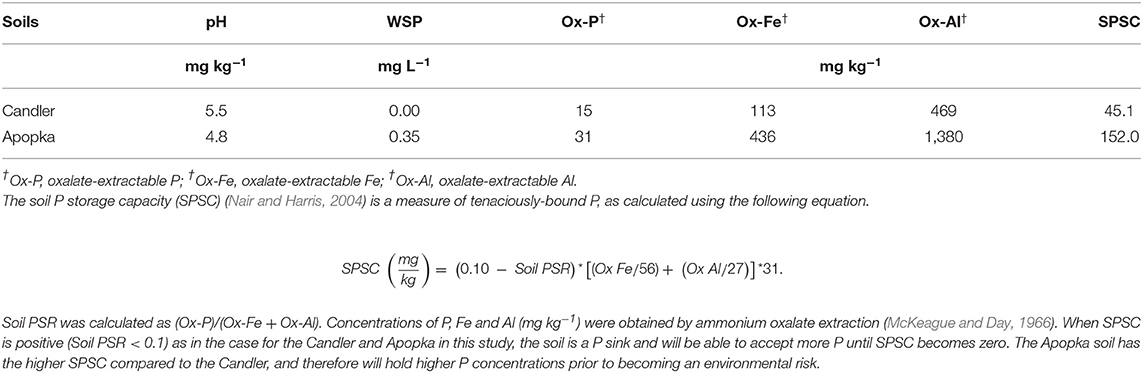
Table 3. Physico-chemical properties of soils used for the desorption experiment adapted from Dari et al. (2016).
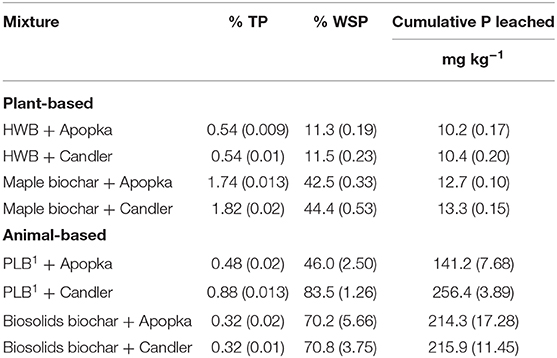
Table 4. Mean percentage (with standard deviation) of leached P as percentage of TP and WSP, and total P leached in 20 extractions.
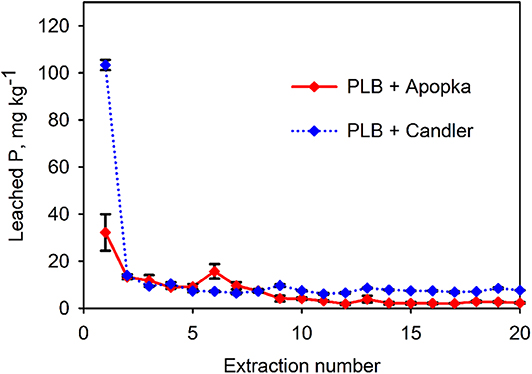
Figure 5. Apopka and Candler soils amended with 1% of PLB1. Bars represent standard deviation of the mean (n = 3). Over 20 sequential extractions PLB1+ Apopka released a total of 141 mg kg−1 and PLB1+ Candler 256 mg kg−1.
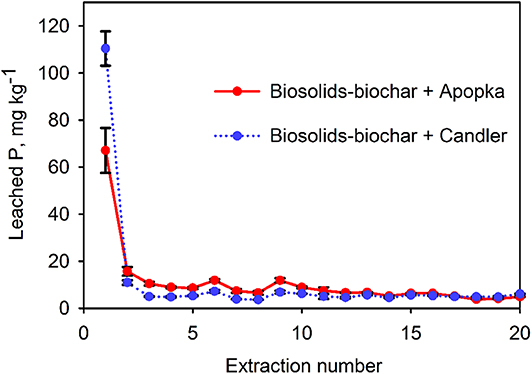
Figure 6. Apopka and Candler soils amended with 1% biosolids-biochar from Jacksonville. Bars represent standard deviation of the mean (n = 3). Over 20 sequential extractions Jacksonville biosolids-biochar + Apopka released a total of 214 mg kg−1 and Jacksonville biosolids-biochar + Candler 215 mg kg−1.
Both Apopka and Candler are sandy soils and only a small amount of the material (<1% w/w) from an animal-based biochar such as PLB would be needed for sufficient P release from the soil for plant uptake while at the same time minimize P loss via runoff or leaching. Further, PLB has the additional advantage of acting as a slow P-release fertilizer due to the presence of whitlockite, a sparingly soluble salt. It is anticipated that a higher rate of biochar additions would be needed if applied as a nutrient source for more P retentive soils in general. In addition to the soil type, the P history of the soil needs to be taken into consideration while deciding on land-application of biochar. When biochar is added to a soil below its threshold PSR (Nair, 2014), the soil will continue to retain P released by the biochar until the threshold is reached, at which point the soil itself becomes a P source (Dari et al., 2016). The addition of any biochar to a soil can reduce P loss from the soil if its equilibrium phosphorus concentration (EPC0) is lower than that of the soil. Plant-based biochars would likely be useful as a soil amendment to reduce P loss from a soil.
Summary and Conclusions
There are substantial differences in nutrient content between biochars from animal and plant-based feedstocks. Animal-based biochars analyzed in this study had the highest concentration of macro and micronutrients, although in the case of K, HWB and maple had higher content of this element compared to biosolids-biochar. The highest content of K and available P was found in the PLB1 and PLB2, despite TP being highest in the biosolids-biochar. The reported imbalance between available nutrients in these materials suggests the possibility of mixing biochar from different feedstocks to attain optimal crop yields. Moreover, it possibly helps explain the yield discrepancies reported in the literature. Biochar application as a nutrient source for crop production should likely take a site-specific approach that is based on availability and composition of feedstocks, nature of soil and climatic conditions. Despite large variations in the characteristics of biochar from different sources, biochars have potential to be a beneficial tool for sustainable soil management and conservation. Moreover, P release depends not only on biochar feedstock (biochar source), but also on the P retention capacity of the soil, as reflected in results from this study. An optimum biochar application rate must account for soil-crop competition for P while avoiding negative environmental consequences of P loss from the field.
Data Availability Statement
All datasets generated for this study are included in the article/Supplementary Material.
Author Contributions
AF was a graduate student and contributed to the main contents of the manuscript. VN is the graduate supervisor of AF and responsible for the overall contribution to the manuscript. WH was responsible for the solid-state characteristics of the biochars in this study. All authors contributed to writing and proof-reading of the manuscript.
Funding
This work was supported by the USDA National Institute of Food and Agriculture, Hatch project, Accession # 1018576.
Conflict of Interest
The authors declare that the research was conducted in the absence of any commercial or financial relationships that could be construed as a potential conflict of interest.
Supplementary Material
The Supplementary Material for this article can be found online at: https://www.frontiersin.org/articles/10.3389/fsufs.2020.510982/full#supplementary-material
Abbreviations
XRD, X-ray diffraction; SEM-EDS, Scanning electron microscope with energy dispersive spectroscopy.
References
Andersen, J. M. (1975). Influence of pH on release of phosphorus from lake sediments. Arch. Hydrobiol. 76, 411–419.
Asai, H., Samson, B. K., Stephan, H. M., Songyikhangsuthor, K., Homma, K., Kiyono, Y., et al. (2009). Biochar amendment techniques for upland rice production in northern laos. 1. soil physical properties, leaf SPAD and grain yield. Field Crops Res. 111, 81–84. doi: 10.1016/j.fcr.2008.10.008
Bakshi, S., He, Z. L., and Harris, W. G. (2014). Biochar amendment affects leaching potential of copper and nutrient release behavior in contaminated sandy soils. J. Environ. Qual. 43, 1894–1902. doi: 10.2134/jeq2014.05.0213
Bhuiyan, M. I. H., Mavinic, D. S., and Beckie, R. D. (2007). A solubility and thermodynamic study of struvite. Environ. Technol. 28, 1015–1026. doi: 10.1080/09593332808618857
Bhuiyan, M. I. H., Mavinic, D. S., and Koch, F. A. (2008). Thermal decomposition of struvite and its phase transition. Chemosphere 70, 1347–1356. doi: 10.1016/j.chemosphere.2007.09.056
Brandt, R. C., Elliott, H. A., and O'Connor, G. A. (2004). Water-extractable phosphorus in biosolids: implications for land-based recycling. Water Environ. Res. 76, 121–129. doi: 10.2175/106143004X141645
Cao, X., and Harris, W. (2010). Properties of dairy-manure-derived biochar pertinent to its potential use in remediation. Bioresour. Technol. 101, 5222–5228. doi: 10.1016/j.biortech.2010.02.052
Chan, K., and Xu, Z. (2009). “Biochar: nutrient properties and their enhancement,” in Biochar for Environmental Management: Science and Technology, eds. J. Lehmann, and S. Joseph (Earthscan: London), 67–84.
Chan, K. Y., Van Zwieten, L., Meszaros, I., Downie, A., and Joseph, S. (2008). Using poultry litter biochars as soil amendments. Aust. J. Soil Res. 46, 437–444. doi: 10.1071/SR08036
Cunha, T. J. F., Madari, B. E., Canellas, L. P., Ribeiro, L. P., Melo, B. V., and Araujo, S. G. (2009). Soil organic matter fertility of the anthropogenic dark earths (Terra Preta de Indio) in the Brazilian amazon basin. Revis. Brasil. Ciencia Solo. 33, 85–93. doi: 10.1590/S0100-06832009000100009
Dari, B., Nair, V. D., Harris, W. G., Nair, P. K. R., Sollenberger, L., and Mylavarapu, R. (2016). Relative influence of soil-vs. biochar properties on soil phosphorus retention. Geoderma 280, 82–87. doi: 10.1016/j.geoderma.2016.06.018
Domingues, R. R., Trugilho, P. F., Silva, C. A., Melo, I. C. N., de Melo, L. C., Magriotis, Z. M., et al. (2017). Properties of biochar derived from wood and high-nutrient biomasses with the aim of agronomic and environmental benefits. PLoS ONE 12:e0176884. doi: 10.1371/journal.pone.0176884
Freitas, A. M., Nair, V. D., Harris, W. G., Mosquera-Losada, M. R., and Nair, P. K. R. (2017). Phosphorus release behavior of biosolids and corresponding biochars in Abstract Retrieved From ASA, CSSA and SSSA International Annual Meetings. Available online at: https://scisoc.confex.com/crops/2017am/webprogram/Paper106996.html (accessed September 19, 2019).
Gaskin, J. W., Steiner, C., Harris, K., Das, K. C., and Bibens, B. (2008). Effect of low temperature pyrolysis conditions on biochar for agricultural use. Trans. ASABE 51, 2061–2069. doi: 10.13031/2013.25409
Glaser, B., and Lehr, V. I. (2019). Biochar effects on phosphorus availability in agricultural soils: a meta-analysis. Sci. Rep. 9:9338. doi: 10.1038/s41598-019-45693-z
Gonzaga, M. I. S., Mackowiak, C. L., Comerford, N. B., Moline, E. F. D., Shirley, J. P., and Guimaraes, D. V. (2017). Pyrolysis methods impact biosolids-derived biochar composition, maize growth and nutrition. Soil Tillage Res. 165, 59–65. doi: 10.1016/j.still.2016.07.009
Hossain, M. K., Strezov, V., Chan, K. Y., Ziolkowski, A., and Nelson, P. F. (2011). Influence of pyrolysis temperature on production and nutrient properties of wastewater sludge biochar. J. Environ. Manag. 92, 223–228. doi: 10.1016/j.jenvman.2010.09.008
Huang, X. L., Chen, Y., and Shenker, M. (2007). Solid phosphorus phase in aluminum-and iron-treated biosolids. J. Environ. Qual. 36, 549–556. doi: 10.2134/jeq2006.0155
Huang, X. L., and Shenker, M. (2004). Water-soluble and solid-state speciation of phosphorus in stabilized sewage sludge. J. Environ. Qual. 33, 1895–1903. doi: 10.2134/jeq2004.1895
IBI (2003). Standardized Product Definition and Product Testing Guidelines for Biochar That is Used in Soil. IBI-STD-01.1, International Biochar Initiative. Available online at: www.biochar-international.org/characterizationstandard (accessed November 10, 2019).
Ippolito, J. A., Spokas, K. A., Novak, J. M., Lentz, R. D., and Cantrell, K. B. (2015). “Biochar elemental composition and factors influencing nutrient retention,” in Biochar for Envrionmental Management: Science, Technolody and Implementation, 2nd edn. eds. L. Johannes and J. Stephen (Routledge), 137–161.
Jeffery, S., Verheijen, F. G., van der Velde, M., and Bastos, A. C. (2011). A quantitative review of the effects of biochar application to soils on crop productivity using meta-analysis. Agric. Ecosyst. Environ. 144, 175–187. doi: 10.1016/j.agee.2011.08.015
Kopp, J. F., and McKee, G. D. (1979). Methods for Chemical Analysis of Water and Waste. U.S. Environmental Protection Agency.
Lehmann, J., Gaunt, J., and Rondon, M. (2006). Bio-char sequestration in terrestrial ecosystems – a review. Mitig. Adapt. Strateg. Glob. Change 11, 403–427. doi: 10.1007/s11027-005-9006-5
Li, X., Ito, A., Sogo, Y., and Wang, X. (2009). LeGeros, R.Z. solubility of mg-containing β-tricalcium phosphate at 25 °C. Acta Biomater. 5, 508–517. doi: 10.1016/j.actbio.2008.06.010
Lima, I. M., Debbie, L. B., Klasson, K. T., and Uchimiya, M. (2014). Influence of post treatment strategies on the properties of activated chars from broiler manure. Chemosphere 95, 96–104. doi: 10.1016/j.chemosphere.2013.08.027
Lima, I. M., and Marshall, W. E. (2005). Adsorption of selected environmentally important metals by poultry manure-based granular activated carbon. J. Chem. Tech. Biotech. 80, 1054–1061. doi: 10.1002/jctb.1283
Macdonald, L., Farrell, M., van Zwieten, L., and Krull, E. (2014). Plant growth responses to biochar addition: an Australian soils perspective. Biol. Fertil. Soil 50, 1035–1045. doi: 10.1007/s00374-014-0921-z
Maddox, N. (2013). The promise (and uncertainties) of biochar. CSA News Mag. 58, 4–9. doi: 10.2134/csa2013-58-9-1
Magalhães, M. M., and Costa, M. O. G. (2018). On the solubility of whitlockite, Ca9Mg (HPO4)(PO4)6, in aqueous solution at 298.15 K. Monatsh. Chem. 149, 1–8. doi: 10.1007/s00706-017-2129-z
Manning, D. A. C. (2008). Biological enhancement of soil carbonate precipitation: passive removal of atmospheric CO2. Mineral. Mag. 72, 639–649. doi: 10.1180/minmag.2008.072.2.639
Mašek, O., Buss, W., Roy-Poirier, A., Lowe, W., Peters, C., Brownsort, P., et al. (2018). Consistency of biochar properties over time and production scales: a characterization of standard materials. J. Anal. Appl. Pyrol. 132, 200–210. doi: 10.1016/j.jaap.2018.02.020
McDowell, R. W., and Condron, L. M. (2000). Chemical nature and potential mobility of phosphorus in fertilized grassland soils. Nutr. Cycling Agroecosyst. 57, 225–233. doi: 10.1023/A:1009838424935
McKeague, J. A., and Day, J. H. (1966). Dithionate-and oxalate-extractable Fe and Al as aids in differentiating various classes of soils. Can. J. Soil Sci. 46, 13–22. doi: 10.4141/cjss66-003
Murphy, J., and Riley, J. P. (1962). A modified single solution method for the determination of phosphate in natural waters. Anal. Chim. Acta 27, 31–36. doi: 10.1016/S0003-2670(00)88444-5
Nair, V. D. (2014). Soil phosphorus saturation ratio for risk assessment in land use systems. Front. Environ. Sci. 2:6. doi: 10.3389/fenvs.2014.00006
Nair, V. D., Freitas, A. M., and Harris, W. G. (2016). Land Application of Biochar and Phosphorus Retention: An Inconvenient Truth. Abstract/Presentation Retrieved from ASA, CSSA and SSSA International Meetings. Available online at: https://scisoc.confex.com/crops/2016am/webprogram/Paper99414.html (accessed October 2, 2019).
Nair, V. D., Graetz, D. A., and Dooley, D. O. (2003). Phosphorus release characteristics of manure and manure-impacted soils. J. Food Agric. Environ. 1, 217–223.
Nair, V. D., and Harris, W. G. (2004). A capacity factor as an alternative to soil test phosphorus in phosphorus risk assessment. New Zeal. J. Agric. Res. 47, 491–497. doi: 10.1080/00288233.2004.9513616
Nair, V. D., Harris, W. G., Chakraborty, D., and Chrysostome, M. (2010). Understanding Soil Phosphorus Storage Capacity. SL 336. Available online at: http://edis.ifas.ufl.edu/pdffiles/SS/SS54100.pdf (accessed September 20, 2019).
Nair, V. D., Nair, P. K., Dari, B., Freitas, A. M., Chatterjee, N., and Pinheiro, F. M. (2017). Biochar in the agroecosystem–climate-change–sustainability nexus. Front. Plant Sci. 8:2051. doi: 10.3389/fpls.2017.02051
Nelson, N. O., Agudelo, S. C., Yuan, W., and Gan, J. (2011). Nitrogen and phosphorus availability in biochar-amended soils. Soil Sci. 176, 218–226. doi: 10.1097/SS.0b013e3182171eac
Qian, T.-T., and Jiang, H. (2014). Migration of phosphorus in sewage sludge during different thermal treatment processes. ACS Sust. Chem. Eng. 2, 1411–1419. doi: 10.1021/sc400476j
Rombola, A. G., Marisi, G., Torri, C., Fabbri, D., Buscaroli, A., Ghidotti, M., et al. (2015). Relationships between chemical characteristics and phytotoxicity of biochar from poultry litter pyrolysis. J. Agric. Food Chem. 63, 6660–6667. doi: 10.1021/acs.jafc.5b01540
Sharpley, A. N., McDowell, R. W., and Kleinman, P. J. A. (2004). Amounts, forms, and solubility o phosphorus in soils receiving manure. Soil Sci. Soc. Am. J. 68, 2048–2057. doi: 10.2136/sssaj2004.2048
Silveira, M. L., O'Connor, G. A., Lu, Y., Erickson, J. E., Brandani, C., and Kohmann, M. M. (2019). Runoff and leachate phosphorus and nitrogen losses from grass-vegetated soil boxes amended with biosolids and fertilizer. J. Environ. Qual. 48, 1498–1506. doi: 10.2134/jeq2019.03.0106
Singh, B., Singh, B. P., and Cowie, A. L. (2010). Characterisation and evaluation of biochars for their application as a soil amendment. Austr. J. Soil Res. 48, 516–525. doi: 10.1071/SR10058
Smith, N. J. H. (1980). Anthrosols and human carrying capacity in amazonia. Ann. Assoc. Am. Geogr. 70, 553–566. doi: 10.1111/j.1467-8306.1980.tb01332.x
Sohi, S. P., Lopez-Capel, E., Bol, R., and Krull, E. (2009). Biochar, Climate Change and Soil: A Review to Guide Future Research. CSIRO Land and Water Science Report 05/09. CSIRO Land and Water Science Report series ISSN: 1834–6618, 64
Steiner, C., Glaser, B., Teixeira, W. G., Lehmann, J., Blum, W. E. H., and Zech, W. (2008). Nitrogen retention and plant uptake on a highly weathered central amazonian ferralsol amended with compost and charcoal. J. Plant Nutr. Soil Sci. 171, 893–899. doi: 10.1002/jpln.200625199
Steiner, C., Sanchez, M. A., and Kammann, C. (2015). “Biochar as an additive to compost and growing media,” in Biochar for Environmental Management: Science Technology and Implementation, eds. J. Lehmann and S. Joseph (New York, NY: Routledge), 928.
Van Zwieten, L., Kimber, S., Morris, K. Y., Chan, A., Downie, J., Rust, S., et al. (2010). Effects of biochar from slow pyrolysis of papermill waste on agronomic performance and soil fertility. Plant Soil 327, 235–246. doi: 10.1007/s11104-009-0050-x
Wang, T., Camps-Arbestain, M., Hedley, M., and Bishop, P. (2012). Predicting phosphorous bioavailability from high-ash biochars. Plant Soil 357, 173–187. doi: 10.1007/s11104-012-1131-9
Wang, Y., Lin, Y. X., Chiu, P. C., Imhoff, P. T., and Guo, M. X. (2015). Phosphorus release behaviors of poultry litter biochar as a soil amendment. Sci. Total Environ. 512, 454–463. doi: 10.1016/j.scitotenv.2015.01.093
Wang, Y., Villamil, M. B., Davidson, P. C., and Akdeniz, N. (2019). A quantitative understanding of the role of co-composted biochar in plant growth using meta-analysis. Sci. Total Environ. 685, 741–752. doi: 10.1016/j.scitotenv.2019.06.244
Woods, W. I., McCann, J. M., and Meyer, D. W. (2000). “Amazonian dark earth analysis: state of knowledge and directions for future research,” in Papers and Proceedings of the Applied Geography Conferences, Vol. 23. ed. F. A. Schoolmaster (Denton, TX: Applied Geography Conferences), 114–121.
Yamato, M., Okimori, Y., Wibowo, I. F., Anshori, S., and Ogawa, M. (2006). Effects of the application of charred bark of ACACIA mangium on the yield of maize, cowpea and peanut, and soil chemical properties in South Sumatra, Indonesia. Soil Sci. Plant Nutr. 52, 489–495. doi: 10.1111/j.1747-0765.2006.00065.x
Yao, L. X., Li, G. L., Tu, S. H., Gavin, S., and Hem, Z. H. (2007). Salinity of animal manure and potential risk of secondary soil salinization through successive manure application. Sci. Total Environ. 383, 106–114. doi: 10.1016/j.scitotenv.2007.05.027
Yu, O.-Y., Raichle, B., and Sink, S. (2013). Impact of biochar on the water holding capacity of loamy sand soil. Int. J. Energ. Environ. Eng. 4:44. doi: 10.1186/2251-6832-4-44
Keywords: plant-based biochar, phosphorus, animal-based biochar, XRD, SEM-EDS
Citation: Freitas AM, Nair VD and Harris WG (2020) Biochar as Influenced by Feedstock Variability: Implications and Opportunities for Phosphorus Management. Front. Sustain. Food Syst. 4:510982. doi: 10.3389/fsufs.2020.510982
Received: 08 November 2019; Accepted: 17 August 2020;
Published: 18 September 2020.
Edited by:
José María De La Rosa, Institute of Natural Resources and Agrobiology of Seville (CSIC), SpainReviewed by:
Antonio F. Patti, Monash University, AustraliaBlaz Stres, University of Ljubljana, Slovenia
Copyright © 2020 Freitas, Nair and Harris. This is an open-access article distributed under the terms of the Creative Commons Attribution License (CC BY). The use, distribution or reproduction in other forums is permitted, provided the original author(s) and the copyright owner(s) are credited and that the original publication in this journal is cited, in accordance with accepted academic practice. No use, distribution or reproduction is permitted which does not comply with these terms.
*Correspondence: Vimala D. Nair, dmRuJiN4MDAwNDA7dWZsLmVkdQ==