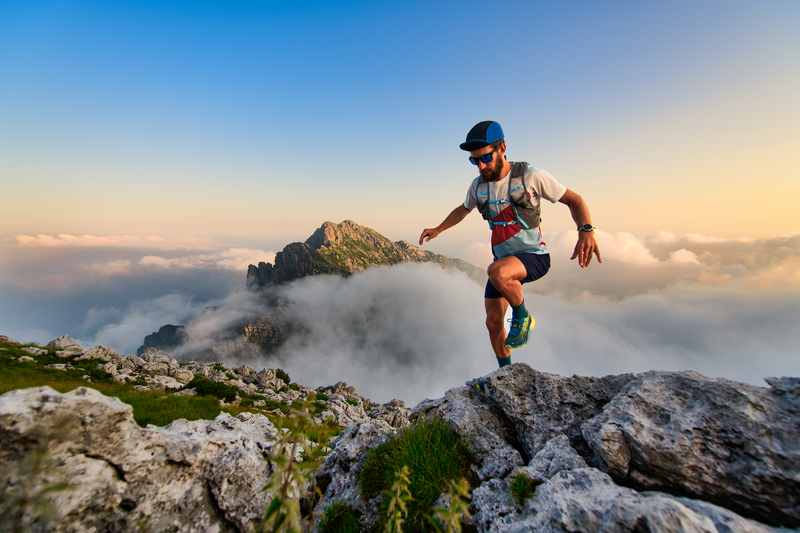
94% of researchers rate our articles as excellent or good
Learn more about the work of our research integrity team to safeguard the quality of each article we publish.
Find out more
ORIGINAL RESEARCH article
Front. Sustain. Food Syst. , 31 July 2020
Sec. Water-Smart Food Production
Volume 4 - 2020 | https://doi.org/10.3389/fsufs.2020.00112
This article is part of the Research Topic Water-Smart Agricultural Production Technologies Under a Changing Climate View all 4 articles
Surface water is used for irrigation by farmers. However, surface waters may be a source of bacterial foodborne pathogens which contaminate fresh produce intended for human consumption. Proposed but not finalized standards for microbial quality of irrigation water through the Produce Safety Rule (PSR) of the Food Safety Modernization Act (FSMA) in the US emphasize the need for effective reduction of levels of pathogens in surface water intended to be used on fruit and vegetable crops. This study evaluated a zero-valent iron (ZVI)-sand filtration system to reduce E. coli populations in pond water and those transferred to growing spinach plants in a field trial. Six filtration events were conducted with the same ZVI-sand or sand (S) laboratory filtration systems. Filtration systems were constructed by connecting 4 PVC pipes (1.25 L) together. ZVI-sand filters contained 50% ZVI/50% sand (0.43–0.60 mm particle size), while sand filters contained 100% sand (0.45–55 mm particle size). In each event, autoclaved pond water (PW) inoculated with E. coli (ca. 4 log CFU/ml)−8 L—was pumped (1 L/min) through each filter followed by uninoculated autoclaved PW (15 L) with samples taken throughout the filtering process for enumeration of E. coli. Data were fit to a linear model to determine reductions of E. coli levels. ZVI-sand filtration removed significantly (p < 0.05) more E. coli (1.1 log CFU) compared to sand filtration. For ZVI-sand-filtered water, there was a statistically significant (p < 0.05) difference of E. coli removal from early trials (trials 1–3, average removal 96%) than in later trials (trial 4–6, average removal 44%), suggesting that age of the ZVI-sand filters influences E. coli inactivation. Overall, ZVI-sand and sand filtration reduced E. coli populations by 70 and −10%, respectively, indicating that ZVI filtration lowered or inactivated E. coli populations while sand filters accumulated E. coli. Field trials showed that soil and spinach plants irrigated with ZVI-sand-filtered water had significantly lower E. coli levels than soils/plants irrigated with sand-filtered water or unfiltered control water. Overall, ZVI-sand filtration significantly reduced E. coli populations in water compared to sand filtration.
Population growth, climate change, and urbanization have focused more attention on the availability and microbial quality of water used for irrigation for fresh fruits and vegetables (USEPA, 2012). Proposed but not finalized microbial irrigation water standards in the Produced Safety Rule (PSR) developed by the U.S. Food and Drug Administration in the Food Safety Modernization Act (FSMA) state that generic E. coli levels cannot exceed 410 CFU/100 mL for any water sample or a geometric mean of more than 126 CFU/100 mL in irrigation water intended for the edible portion of crop (USFDA, 2018). Surface waters in the U.S. can contain bacterial foodborne pathogens like shiga-toxigenic E. coli (STEC), Salmonella spp., and Listeria monocytogenes (Cooley et al., 2014; Weller et al., 2015; Cho et al., 2018; Callahan et al., 2019; Haymaker et al., 2019; Sharma et al., 2020). Several outbreaks related to the consumption of contaminated Romaine lettuce have been traced back to irrigation water containing the pathogen E. coli O157:H7 (CDC, 2018, 2019), indicating that surface irrigation water can serve as a vehicle for contamination of leafy greens. Improving irrigation water quality through targeted interventions may reduce the burden of foodborne illness related to contaminated produce in the U.S. Previous studies have shown that contaminated produce accounts for one-fourth of the total medical costs associated with foodborne illness in the US (USDA, 2019). Reducing levels of bacterial foodborne pathogens in surface water may allow more growers to use it for irrigation.
Almost 30% of farms in the United States have sales of less $100,000/year. Cost-effective mitigation strategies improving the microbial quality of irrigation water may be of interest to fruit and vegetable farming operations with limited capital resources (Scharff, 2010). Survey results have shown that across the U.S., many farmers already use surface water as a primary irrigation water source and are interested in using additional sources of non-traditional irrigation water, provided that the microbial quality is acceptable at the point of use (Suri et al., 2019). Irrigation water withdrawals accounted for 42% of total freshwater withdrawals in the U.S., while 52% of irrigation water was obtained from surface water sources (Dieter et al., 2018).
Various studies have been conducted to develop and improve the methods to remove and inactivate microbial contamination from water. Membrane filtration technology has been considered a useful method for wastewater treatment but can be prone to biofouling, reducing effectiveness of filtration (Chang et al., 2002; Herzberg and Elimelech, 2007). Ultraviolet (UV) light is effective in inactivating microorganisms but requires an electrical power source (Gayan et al., 2012) and may have limited penetration in turbid water.
Zero-valent ion (ZVI)/sand filtration of agricultural irrigation water can improve its microbial quality without the addition of disinfectant chemicals. Permeable reactive barriers (PRBs) incorporated with bio-sand and ZVI filters have been previously utilized to remove chemical contaminants in groundwater (Henderson and Demond, 2007), based on its adsorption and potential inactivation of contaminants of concern in drinking water, including bromate (Xie and Shang, 2005), chloropicrin (Pearson et al., 2005), haloacetic acids (Hozalski et al., 2001; Zhang et al., 2004), and N-nitrosodimethylamine (Odziemkowski et al., 2000) ZVI-based filter systems have reported to have a >5 log reduction of viruses in synthetic groundwater (You et al., 2005). The removal rate of viruses or bacteria through ZVI filters varies with contact time, particle size, solution pH (Zhang, 2003), dissolved oxygen (Lee et al., 2008), concentration of ions (Diao and Yao, 2009), and redox potential (Auffan et al., 2008; Bradley et al., 2011). Other workers have shown that modified zero-valent iron sand filters can reduce fecal coliform levels in contaminated river water by 1 log CFU (Bradley et al., 2011; George and Ahammed, 2019) and E. coli in drinking water by between 90.9 and 99.9% (Sizirici et al., 2019). In reclaimed water, ZVI filtration reduced concentrations of antibiotics (Kulkarni et al., 2019) and virus like particles (Chopyk et al., 2019). Several studies examining the inactivation of bacterial species have shown that Listeria innocua and E. coli in ZVI-filtered water applied to foliar died off more quickly than E. coli or Listeria spp. in sand-filtered water (Ingram et al., 2012; Marik et al., 2019).
The evaluation of ZVI filtration and implementation for agricultural irrigation water remains limited. Contaminated irrigation water containing E. coli may introduce bacterial foodborne pathogens to soil, where they may persist and transfer to crops which grow close to the soil surface (Shah et al., 2019). Extended persistence of bacterial pathogens in soil may increase the likelihood of transfer of pathogens to produce crops. However, there is a lack of understanding on the persistence of E. coli present in sand or ZVI-effluent applied to agricultural soils. Sand filters are commonly used in the irrigation of many fruit and vegetable crops, especially for drip irrigation purposes. Our goal was to use existing agricultural practices and modify it in order to improve agricultural water quality used in production of fruits and vegetables.
Our work presented here is an evaluation of the use of ZVI-based filtration in laboratory and field trials. The objectives of this study are (1) to evaluate and compare the removal of inoculated E. coli from pond water via ZVI-sand and sand filtration; (2) to determine if E. coli removal is consistent using the same ZVI-sand and sand filters multiple times over the duration (50–60 days) of an agricultural growing season for leafy greens; (3) determine presence of E. coli TVS 353 on spinach plants in fields irrigated with ZVI and sand filtered water.
Each filter system was constructed using 4 pieces of 5 cm (diameter) by 60 cm (length) of Charlotte PVC linked together with U shaped connectors (Figure 1). For construction of ZVI-sand filters, PVC pipes were filled with equal volumes (625 mL, measured in a 1 L graduated cylinder) of 0.43–0.60 mm ZVI particles (Peerless Metals, Detroit MI) and sand particles (0.45–0.55 mm) (Northern Filter Media, Muscatine, IA). Sand and ZVI particles were mixed well by adding material to a disinfected commercial bucket, and rotating the bucket on it's side for 5 min. Sand and ZVI particle were further mixed for 1 min to obtain a distributed mixture. This mixture (1.18 L) was transferred to a graduated cylinder. Columns (PVC pipes) were packed one at a time with efforts made to mix particles uniformly, and packed columns were not disturbed after packing to minimize particle resettling. PVC pipes were sealed at the end with landscape fabric to prevent leakage of sand/ZVI particles. Once PVC pipes were filled, pipes were attached to U-shaped connectors (which did not contain sand or ZVI/sand) so that 4 PVC pipes containing 1.18 L of sand/ZVI were connected. Sand filters were constructed identically and filled with 100% sand particles (0.45–0.55 mm). The approximate porosity of both ZVI and sand filters was 0.6, and the volumetric flow rate was set at 1 L/min for both filters using a metering pump (Flex pro A4 ProSeries, Standard Peristaltic Metering Pump, Cole-Parmer, Vernon Hills, IL) with a residence time of 3 min. Prior to testing, 2 L of autoclaved pond water were added to saturate both ZVI and sand filters to minimize air gaps in the filtration systems and held for 7 days prior to filtration.
Figure 1. ZVI/sand and sand filtration for laboratory and field trials of pond water. Each white PVC tube (60 cm length ×5 cm diameter) is filled with either (1) a mixture of 50% ZVI/50% sand (0.43–0.55 mm—particle size) for ZVI/sand filtration or (2) 100% sand (0.45–0.55 mm particle size) for sand filtration. Autoclaved pond water inoculated with E. coli TVS 353 was pumped through either ZVI/sand or sand filters at 1 L/min.
Pond water samples were collected from the a pond located at the University of Maryland Wye Research and Education Center (Wye REC, Queenstown, MD). Prior to each sampling event, ~80 L of pond water was collected in sterile 20-L carboys (VWR, Philadelphia, NJ) using a Honda WX10TA pump (Swepsonville NC). Pond water samples were stored at 4°C after collection and filtered within 1 week. Pond water characteristics were measured using a ProDSS multi-parameter water quality sonde/meter (YSI, Yellow Springs, OH) and are summarized in Table 1 for the duration between trials 1–8. Prior to filtration, pond water was sterilized by autoclaving. Autoclaved pond water contained no rifampicin-resistant E. coli TVS 353 when tested.
Table 1. Pond water quality characterization for dates during laboratory ZVI-sand or sand filtration trials to reduce inoculated E. coli levels.
E. coli strain TVS 353, originally recovered from an agricultural water source (Tomás-Callejas et al., 2011), was isolated from frozen stock onto MacConkey agar (Neogen, Lansing, MI) supplemented with 80 μg/mL rifampicin (MACR) (Sigma-Aldrich, St. Louis, MO). Plates were incubated at 37 for 18–24 h and a single colony was transferred to 100 mL of tryptic soy broth containing 80 μg/mL rifampicin (TSBR) and incubated for 18–24 h at 37. The culture was then diluted 1:100, and 23 mL of this dilution was added to 8 L of autoclaved pond water.
Autoclaved pond water (8 L) was inoculated with E. coli TVS 353 (4 log CFU/ml) mixed vigorously and then pumped through the ZVI-sand and sand filters, followed by 15 L of uninoculated pond water. This level was chosen to be 1-log greater than the maximum level of E. coli observed in pond water from Wye REC over a 12-month period (3 log CFU/mL) (Solaiman et al., submitted). For each trial, the first 2 L of the effluent from both ZVI-sand and sand filters were discarded because this volume was assumed to be contained in the filter before pumping. The remaining 23 L effluent was collected to determine E. coli TVS 353 populations in each liter of the effluent. Six separate filtration trials were conducted sequentially for the ZVI-sand and sand filters in laboratory experiments.
For the field trial, separate ZVI-sand and sand filters were constructed specifically for field trials as described above. Two filtration events were performed 7 days apart in the laboratory, and filtered water was transported to the field for irrigation.
Butlertown silt loam soil was leveled in each field plot at the University of Maryland Wye REC. Approximately 22 kg of nitrogen (N) was applied to the entire plot area before making the planting beds, incorporated using a rototiller. Each plot was subdivided into three 3 × 2.1 m subplots to apply irrigation water through either ZVI or sand filtration, each of which were divided by 2.1 × 0.6 m buffer strip (Figure 2). Savoy type spinach was planted in each plot. Seeds were started in 50-cell tray flats in a greenhouse and then transplanted into plots. Plants were started 35 days before irrigation events occurred. Spinach plants were close to harvest when the two irrigation events occurred.
Figure 2. Field design of Savoy spinach plots irrigated with either Zero-valent iron sand-filtered water, sand-filtered water, and control (not filtered water) containing E. coli.
Autoclaved pond water was inoculated and filtered in the laboratory using the same ZVI/sand and sand filtration setup described previously. Filtered water or control (not filtered) was then transported to WyeREC. Carboys of ZVI-sand–and sand-filtered water were transported to the field and fed through drip tape to irrigate spinach plants.
Three replicate plots of spinach plants were irrigated with either ZVI/sand, sand, or control (not-filtered) water using drip tape (Rivulis Irrigation, Gvat, Israel). with 24 L of ZVI-sand, sand-filtered or control for each irrigation event. Each replicate plot was irrigated twice, 7 days apart. Drip tape for spinach plots was placed 1.5 cm below soil surface and irrigation emitters were 30 cm apart. The drip tape flow rate was 0.75 L/min per 30.48 m of tape at 12 PSI. The planting bed was 75 cm on top and 10 cm high spinach plants were spaced 30 cm between the double rows and 15–30 cm between plants down each row with ~28 plants in each plot. Up to 2 L of water was collected at the end of the drip tape in sterile glass bottles, which were transported back to the laboratory in refrigerated coolers. Two irrigation events were performed 7 days apart. Pre- and post-filtered water samples were analyzed for E. coli as described below, as well as samples collected from the end of driplines.
Three replicate plots of spinach for each irrigation event (sand, ZVI, and control) were evaluated. No rainfall was recorded in the 7 days between the first and second irrigation events during this field trial. Control (non-filtered water) was collected and stored in the same manner as ZVI-sand- and sand-filtered water.
For laboratory experiments, each liter (n = 24) of filtered water was collected in sterile glass or plastic bottles and shaken for 20 s before 1 mL of from each liter was used to make serial dilutions in 0.1% peptone water. Volumes of serial dilutions (50 μL) were spiral plated (WASP2 spiral plater (Microbiology International, Frederick, MD), in duplicate, onto MACR. Plates were incubated at 37°C for 18–24 h before enumeration. For field experiments, the 24 L effluent from both ZVI-sand and sand filtration events were collected and analyzed for E. coli TVS 353 as previously described. Water samples in bottles collected from ends of driplines in the field study were shaken for 20 s by hand and serial dilutions were plated on MACR as described previously.
Three soil samples from each replicate plot were collected from the soil surface (0–3 cm) after irrigation events, and transported back to the laboratory in refrigerated coolers. Samples were homogenized by hand for 30 s and 20 g was measured into sterile 24 oz. filtered Whirlpak bags (Nasco, Ft Atkinson, WI). For each soil sample, 80 mL of Buffered Peptone Water (BPW, Neogen, Lansing, MI) were added and soil was homogenized by hand. The homogenate (50 μL, in duplicate) was spiral plated onto MACR and incubated for 18–24 h at 37. To determine moisture levels in soil samples, ~5 g of the original homogenized sample was aliquoted to 2 oz. Whirl-pak bags and analyzed using a TrueDry CV9 (Decagon Devices, In., Pullman, WA). An additional soil sampling was conducted 7 days after the second irrigation event to determine if E. coli TVS 353 populations changed after that time period.
Each replicate field plot was divided into four zones, and one spinach plant from each zone was collected using sterile scissors immediately after irrigation. Spinach samples were collected in 92 oz. Whirl-pack bags and transported back to the laboratory in coolers. For each plant, 500 mL TSBR was added and then hand-massaged for 1 min before being incubated for 18–24 h at 37°C. Following incubation, enrichments were streaked for isolation on MACR using a 10 μL loops and plates which were incubated at 37 for 18–24 h. Presumptive violet-colored colonies from MACR plates were isolated on tryptone bile x-glucuronide (TBX, Neogen, Lansing, MI) and incubated at 37 for 18–24 h. Teal-colored colonies on TBX indicated the confirmed presence of E. coli TVS 353.
For statistical analysis, E. coli TVS 353 counts were log-transformed (log CFU/mL), and populations in each liter of filtered effluents were plotted and fit to a linear regression model to compare the overall reductions achieved by ZVI-sand and sand filtration using R version 3.4.3 (R Core Team, 2017).
For statistical analysis, Equation 1 was used:
Where α1 is the y-intercept value, α2 is the slope value, and (Y) is the log CFU/ml of sand-filtered water in the specific liter [filtered water (l)] of effluent of sand-filtered water. A variable T can be applied, which is set to 0 if evaluating sand filtration, and T = 1 if ZVI/sand filtration was evaluated, leading to Equation 2:
By combining Equations (1) and (2), the following equation is derived:
Where α3 is the y-intercept and α4 is slope value for ZVI-sand filtered water. Two different Equations were obtained:
Where YA is the log CFU/ mL of E. coli TVS 353 for sand-filtered water and YB is the log CFU/ml for E. coli TVS 353 for ZVI-sand filtered water for specific liter [filtered water (l)] of the effluent. Equations used for these regression models are based on those described for biophase dose-response time models (Gabrielsson et al., 2019).
The null hypothesis for the linear regression modeling is that all coefficients αi are equal to zero; the alternative hypothesis is that αi values are not zero. Percentage reductions of E. coli populations in inoculated water by ZVI-sand- and sand-filtration were determined by calculating the area under the curve (AUC) for each ZVI-sand or sand filtration trial using the linear regression equation for each trial and comparing to the population of the initial E. coli inoculum introduced to filters.
The Kruskal-Wallis and pairwise Wilcoxon rank sum tests was used to determine differences in E. coli populations introduced to soils through control (unfiltered), ZVI- or sand-filtered water after the first and second irrigation events, and 7 days after the second irrigation event. These non-parametric tests were used because there were <20 observations and a normal distribution of these values during these experiments could not be assumed. In all cases, p-values of 0.05 were defined as statistically significant.
Average populations of E. coli TVS 353 recovered from each liter of 24 L of ZVI-sand or sand-filtered effluent over 6 trials are shown in Figure 3. These data show that ZVI-sand filtration was more effective at removing E. coli than sand filtration. ZVI-sand filtered water contained significantly (p < 0.001) lower populations of E. coli than sand filtered water. For both ZVI-sand and sand filtration, the first 2 L of effluent contained relatively low E. coli TVS 353 levels for both filters. Populations of E. coli were relatively constant between liters 3–11 for both ZVI-sand and sand-filtered effluents (Figure 3). The concomitance and similar duration (~8 L) of the high E. coli population plateaus suggests both ZVI-sand and sand filters performed like plug-flow reactors. Therefore, the ~1-log lower E. coli population in ZVI-sand filter effluent was most likely due to removal of E. coli by ZVI rather than retarded transport, which would have shifted the maximum E. coli population to a later time. Table 2 shows the reduction of E. coli TVS 353 by ZVI-sand and sand filtration in each of the six individual trials. The reduction of E. coli by ZVI-sand and sand filtration were varied over 6 trials. Mean reductions of E. coli TVS 353 were 70 ± 35% by ZVI-sand and −10 ± 87% by sand filtration. ZVI-sand filtration reduced E. coli populations more effectively in trials 1–3 (mean reduction: 96%) compared to trials 4–6 (mean reduction: 44%) (Table 2), indicating that the age of the ZVI-sand filtration media may influence its efficacy in reducing E. coli populations in surface waters.
Figure 3. Mean E. coli populations (log CFU/mL) in each liter (n = 24) of ZVI-sand or sand-filtered effluent from six laboratory trials. E. coli TVS 353 was inoculated at 4 log CFU/mL before filtration events.
Table 2. The reduction of E. coli TVS 353 by ZVI-sand and sand filtration in each of the six individual trials as shown by percentage or log CFU/ml reduction.
The highest populations of E. coli were detected after 8 L (3.47 log CFU/ml) and 10 L (4.26 log CFU/ml) in ZVI-sand- and sand-filtered effluents, respectively. Trials 2–4 of sand filtration had an average E. coli reduction of 57% (Table 2). However, sand filtration also showed a negative reduction of E. coli in trials 5–6, indicating that E. coli cells may be accumulating in the sand filters from trial to trial, leading to diminished or no reduction of E. coli populations. The gradual diminished efficacy of sand filtration on reducing E. coli levels may be due to the adsorption and mechanical trapping of the E. coli cells in the sand filter in early trials, and those cells being released from the filter during later trials, leading to a higher number of cells in the effluent compared to the initial inoculum level (4 log CFU/ml). It is possible the conditions inside the sand filter supported E. coli growth because of sufficient moisture and organic material introduced through pond water. During sand filtration, E. coli populations in effluent were generally higher than the initial inoculum between liters 3–10. E. coli populations in ZVI-sand filters did not exceed the inoculum level in effluent collected in liters 3–10, potentially indicating that ZVI-sand filters may suppress the growth or physiologically stress E. coli populations. Neither ZVI-sand or sand filters were back-flushed during these experiments.
Examining the y-intercept values α1 and α3 indicates a significant (p < 0.05) difference between sand-filtration and ZVI-sand filtration (Table 3), which shows approximately a 1-log CFU/ml difference between E. coli levels in ZVI-sand- and sand-filtered water. The lack of statistical differences between the coefficient α2 and α4 (slope values) of the survival curve of E. coli in ZVI-sand- and sand-filtered effluents indicates that the majority of the reduction in E. coli populations occurs at the outset of ZVI-filtration.
Table 3. Statistical and estimated coefficients for model to evaluate the use of ZVI-sand and sand for average inactivation of E. coli from Equations (7) and (8).
Table 4 shows the fitting coefficients determined by applying the linear model to each of the six individual trials. The differences (log CFU/mL E. coli) between sand and ZVI-sand y-intercept (α3) values for trials 1, 2, 3, and 5 ranged between −2.5 and −0.8 (p < 0.05), indicating that ZVI-sand filtration resulted in significantly lower E. coli populations in effluents from these trials compared to sand filtration. Coefficients of α4–the differences between the slope values for ZVI-sand and sand effluents—for trial 1, 2, 3, and 5—were not statistically significant, indicating that effluent release patterns through both ZVI and sand filters are similar.
Table 4. Statistical and estimated coefficients for model to evaluate the longevity and comparisons of E. coli survival in effluent by use of ZVI-sand and sand for trials 1-6.
Ingram et al. (2012) reported that ZVI filtration was more effective than sand filtration at inactivating E. coli O157:H12 in inoculated water. Several studies have shown that inactivation of bacterial cells has resulted from contact with ZVI particles (You et al., 2005; Lee et al., 2008; Ingram et al., 2012; Marik et al., 2019). The transition of iron from a zero-valent state (Fe0) to ferric iron (Fe3+) in the ZVI system leads to the formation of reactive oxygen species, causing the degradation of bacterial cells under oxidative stress conditions (Auffan et al., 2008; Lee et al., 2008). Other workers have suggested that increasing the influent volume may reduce E. coli reductions over time in ZVI-sand filtration systems (Ahammed and Davra, 2011). In our study, volume did not exceed 23 L during any trial, which may have extended the efficacy of the ZVI-sand filter and increased its longevity as compared to sand filtration.
Other research evaluating the longevity of ZVI-sand and sand filters, showed that both types of filters were effective over 13 trials (390 L of water), a 22-week period (Marik et al., 2019). In those experiments, a 35% ZVI-sand filter was effective consistently reducing E. coli, by 2.27 ± 0/27 log CFU/ml) and L. monocytogenes by 2.53 ± 0.31 log CFU/ml when inoculated into pond water at ca. 7 log CFU/ml. In that work, ZVI-sand-filtration was significantly more effective in reducing L. monocytogenes compared to E. coli. The differences in longevity between the Marik et al. study and the current one presented here may be due to different water preparation methods, inoculum levels, and ZVI to sand ratios used in the composition of the filters. The same source of ZVI was used in the Marik study and our current study; however their filtration system had a total volume of 1.25 L compared to 5 L in our current study. In our current study these ZVI-sand and sand filters were evaluated over 56 days, which estimates a growing season for leafy green crops; this is shorter than previous studies evaluating sand/iron filters for decontaminating drinking water (Bradley et al., 2011), which evaluated virus removal by sand/iron filters over a 12-month time period. Work examining the removal of MS-2 bacterial, an enteric viral surrogate, showed that iron-oxide containing biosand filters were able to effectively remove 6.5 log PFU after over 370 days (Bradley et al., 2011). The addition of iron-coated sand to biosand filters resulted in a 1-log increase in removal of E. coli compared to biosand filters without iron, improving the quality of drinking water (Ahammed and Davra, 2011). Previous work has shown that an increase in the concentration of humic acid, a component of natural organic material (NOM), measured as dissolved organic carbon (DOC), decreased the ZVI effectiveness in removing nitrobenzene by fouling ZVI binding sites (Yin et al., 2012); fulvic acid, another component of NOM, may also have the same affect (Qin et al., 2020). Neither the ZVI-sand or sand filtration systems were back-flushed during these experiments.
Previous studies have shown that internal oxidation reduction potential, influent pH, and alkalinity are major predictors of the performance of zero valent iron permeable reactive barriers (PRB) (Henderson and Demond, 2007). The relatively high pH of influent pond water (8.18) for this study (Table 1) may have been a factor in the reduced performance of ZVI after the 4th trial as well. The increased pH of pond water over trials, potentially due to the changes in trophic activity in ponds, may affect the bactericidal mechanism of ZVI. At pH values >4, ZVI particles become coated in various iron oxides (Noubactep, 2009). The combination of increased pH and accumulation of NOM in ZVI/sand filters may have decreased the ability of ZVI to strongly adsorb to E. coli cells by making the charge of the surface negative, which may have decreased the removal efficacy of the ZVI/sand filters. The accumulation of NOM and available soluble carbon in sand filters may have allowed a higher level of E. coli population to remain in the filter, which may partially explain the higher levels of E. coli in the effluent of sand filters for trials 5 and 6 compared to introduced amount. The use of autoclaved pond water may have reduced the number and diversity of microorganisms in forming the biological sand layer (schmutzdecke), which may have affected E. coli levels in the effluent and the filter. Marik et al. (2019) did not autoclave pond water from the same source and observed more consistent reductions of E. coli over a longer duration of time in ZVI/sand and sand filters. Since our study was intended to evaluate agricultural water used for irrigation, these 6 trials occurred over 56 days, which represented the growing season for various leafy green commodities (Table 1). This is considerably shorter than the evaluation period for biosand filters for drinking water disinfection, which can last for more than 1 year. Agricultural operations may present more opportunities for changing or replacing filters between growing seasons than drinking water filters, which may be kept in use for longer periods of time.
Table 5 describes the reduction of E. coli TVS 353 populations by ZVI-sand, sand, or no (control) filtration for field trials. New ZVI and sand filters were constructed for field trials after laboratory trials. Initial populations of inoculated E. coli TVS 353 in pond water were 4.51 log CFU/ml. As in laboratory trials, ZVI-sand-filtration significantly (p < 0.05) reduced E. coli TVS 353 levels by 2.01 log CFU/ml compared to 0.68 log CFU/ml by sand filtration. There were no significant differences between E. coli levels in sand-filtered and control (non-filtered) effluents (Table 5). E. coli populations were significantly lower in effluents of all water types when collected from the ends of driplines when compared to pre-filtration levels and levels before irrigation pumping commenced.
Table 5. Summary of E. coli TVS 353 levels inoculated into autoclaved pond water (pre-filter), recovered after ZVI-sand and sand filtration (post-filter), or control (not filtered).
The pond water quality characteristics both before and after filtration for the field trials are summarized in Table 6. As expected, ZVI-sand filtration increased the pH of filtered water (9.3–9.4) compared to pre-filtered water (8.5) but sand filtration did not (8.5–8.5), (Zhang, 2003), compared to pre-filtered water in field irrigation trials. Other characteristics of the pond water (dissolved oxygen, oxidation reduction potential) might have influenced E. coli reductions. Previous filtration trials using nanoscale ZVI (nZVI) elevated pH values by 2–3 units (Zhang, 2003). In our study, the pH of ZVI-sand filtered water was greater than the acceptable range for agricultural irrigation (6.5–8.4), but may have been influenced by the relatively high pH of pond water before filtration (USEPA, 2012). ZVI-sand filtration significantly reduced dissolved oxygen percentage (DO%) and oxidation reduction potential (ORP) values in field trials. Turbidity values of pond water were lowered after ZVI-sand filtration compared to sand filtration in trial 1 could not be measured after ZVI-filtration in trial 2. Several previous studies confirmed that the reaction between ZVI and DO is one of the most rapid among the chemical reactions involving ZVI, especially when DO% values are relatively low (He et al., 2010; Johnson et al., 2013; Kocur et al., 2014). These conditions may not be applicable to the pond water since the DO% is relatively high (84%), and therefore more investigation is needed. Rapidly decreasing DO levels in ZVI-sand-filtered water might be an indicator of ZVI activity and may be used as an indicator of the longevity of the ZVI filter.
Table 6. Water quality parameters measured before water samples were obtained (24 h before filtration) and immediately after sand and ZVI-sand filtration before irrigation of spinach plots.
Populations of E. coli TVS 353 in soils irrigated by control, ZVI-sand- and sand-filtered water are shown in Table 7. Soils were analyzed after each of two irrigation events (7 days apart), and then 7 days following the 2nd irrigation event. ZVI-sand-irrigated soils contained significantly (p < 0.001) lower levels of E. coli TVS 353 than control (p < 0.001) or sand-filtered irrigated soils (p < 0.001). There were no statistical differences (p = 0.31) between E. coli levels in soils irrigated with sand-filtered or control water, indicating that E. coli persisted at lower levels in ZVI-sand-irrigated soils compared to sand-filtered or control irrigated soils.
Table 7. E. coli TVS 353 population (log CFU/g) in soil samples after irrigation by ZVI-sand, sand filtration and control (no filtration) water.
In both irrigation trials, 8% (1/12) of spinach plants irrigated with ZVI-sand-filtered water contained E. coli TVS 353, while 25% (3/12) of plants irrigated with sand-filtered water contained E. coli TVS 353. In control-irrigated plots, 0% (0/12) and 33% (4/12) of the spinach plants in the first and second irrigation trials contained E. coli TVS 353, respectively. Previous studies have shown that ZVI-sand-irrigated leaves of plants showed lower survival or faster die-off of bacterial pathogens on foliar surfaces. Ingram et al. (2012) showed that leaves irrigated with ZVI-filtered water had E. coli O157:H12 populations ca. 3–4 log CFU/g lower than leaves irrigated with sand-filtered water over 4 days in a field study. Marik et al. (2019) showed that die-off rates of Listeria innocua introduced to cucumber leaves through ZVI-sand filtration were significantly lower on days 3 and 4 compared to those introduced through sand filtration. However, die-off rates of E. coli TVS 353 on cucumber leaves irrigated with ZVI-sand- or sand-filtered water were similar over 4 days.
ZVI-sand filtration was more effective than sand filtration in reducing E. coli inoculated in pond water. Results show that reductions of E. coli populations occurred at the outset of filtration events, and ZVI-sand-mediated reductions were greater in early trials (1–3) than later trials (4–6). In field trials, ZVI-sand-filtered water contained less E. coli than sand-filtered waters, and also introduced lower levels of E. coli to spinach leaves and soils than sand-filtered waters. ZVI-sand-filtration represents a potential practical solution for small-scale growers to expand their use of different sources of irrigation water beyond groundwater while attempting to help them comply with microbial water quality standards.
The datasets generated for this study are available on request to the corresponding author.
SK, PK, RB, EH, CE, SA, and MN contributed to experimental design, planning, and execution. KK, PC, and MS contributed to all aspects of the research project. AS responsible for experimental planning. SK responsible for statistical analysis/manuscript writing. MS responsible for experimental coordination and manuscript writing. All authors contributed to the article and approved the submitted version.
This work was funded by the USDA NIFA Grant 20166800725064 entitled CONSERVE (Coordinating Nontraditional Sustainable watER Use in Variable climatEs): A Center of Excellence at the Nexus of Sustainable Water Reuse, Food and Health (www.conservewaterforfood.org), and by USDA ARS project plan 8042-32420-006-00-D: Characterization and Mitigation of Bacterial Pathogens in the Fresh Produce Production and Processing Continuum.
The authors declare that the research was conducted in the absence of any commercial or financial relationships that could be construed as a potential conflict of interest.
We would like to thank Sarah Behal and Donghyun Kim for their laboratory support.
Ahammed, M., and Davra, K. (2011). Performance evaluation of biosand filter modified with iron oxide-coated sand for household treatment of drinking water. Desalination 276, 287–293. doi: 10.1016/j.desal.2011.03.065
Auffan, M., Achouak, W., Rose, J., Roncato, M. A., ChanÃac, C., Waite, D. T., et al. (2008). Relation between the redox state of iron-based nanoparticles and their cytotoxicity toward Escherichia coli. Environ. Sci. Tech. 42, 6730–6735. doi: 10.1021/es800086f
Bradley, L., Straub, A., Maraccini, P., Markazi, S., and Nguyen, T. H. (2011). Iron oxide amended biosand filters for virus removal. Water Res. 45, 4501–4510. doi: 10.1016/j.watres.2011.05.045
Callahan, M. T., Van Kessel, J. A., and Micallef, S. A. (2019). Salmonella enterica recovery from river waters of the Maryland Eastern Shore reveals high serotype diversity and some multidrug resistance. Environ. Res. 168, 7–13. doi: 10.1016/j.envres.2018.09.012
Centers for Disease Control Prevention (CDC) (2018). Multistate outbreak of E. coli O157:H7 infections linked to Romaine lettuce (Final update). Available online at: https://www.cdc.gov/ecoli/2018/o157h7-04-18/index.html (accessed December 9, 2019).
Centers for Disease Control Prevention (CDC) (2019). Outbreak of E. coli infections linked to Romaine lettuce. Available online at: https://www.cdc.gov/ecoli/2018/o157h7-11-18/index.html (accessed December 9, 2019).
Chang, I. S., Clech, P. L., Jefferson, B., and Judd, S. (2002). Membrane fouling in membrane bioreactors for wastewater treatment. J. Environ. Eng. 128, 1018–1029. doi: 10.1061/(ASCE)0733-9372(2002)128:11(1018)
Cho, S., Hiott, L. M., Barrett, J. B., McMillan, E. A., House, S. L., Humayoun, S. B., et al. (2018). Prevalence and characterization of Escherichia coli isolated from the upper oconee watershed in Northeast Georgia. PLoS ONE 13:e0197005. doi: 10.1371/journal.pone.0197005
Chopyk, J., Kulkarni, P., Nasko, D., Bradshaw, R., Kniel, K., Chiu, P., et al. (2019). Zero-valent iron sand filtration reduces concentrations of virus-like particles and modifies virome community composition in reclaimed water used for agricultural irrigation. BMC Res. Notes 12:223. doi: 10.1186/s13104-019-4251-y
Cooley, M., Quiñones, B., Oryang, D., Mandrell, R., and Gorski, L. (2014). Prevalence of shiga toxin producing Escherichia coli, Salmonella enterica, and Listeria monocytogenes at public access watershed sites in a California central coast agricultural region. Front. Cell. Infect. Microbiol. 4:30. doi: 10.3389/fcimb.2014.00030
Diao, M., and Yao, M. (2009). Use of zero-valent iron nanoparticles in inactivating microbes. Water Res. 43,5243–5251. doi: 10.1016/j.watres.2009.08.051
Dieter, C. A., Mapin, M. A., Caldwell, R. R., Harris, M. A., Ivahnenko, T. I., Lovelace, J. K., et al. (2018). Estimated use of water in the United States in 2015: U.S. Geol. Surv. Circ. 1441:65. doi: 10.3133/cir1441
Gabrielsson, J., Andersson, R., Jirstraand, R., and Hjorth, S. (2019). Dose-response-time data analysis: an underexploited trinity. Pharmacol. Rev. 71, 89–122. doi: 10.1124/pr.118.015750
Gayan, E., Serrano, M. J., Raso, J., Alvarez, I., and Condon, S. (2012). Inactivation of Salmonella enterica by UV-C light alone and in combination with mild temperatures. Appl. Environ. Microbiol. 78, 8353–8361. doi: 10.1128/AEM.02010-12
George, D., and Ahammed, M. M. (2019). Effect of zero-valent iron amendment on the performance of biosand filters. Water Supply 19, 1612–1618. doi: 10.2166/ws.2019.032
Haymaker, J. R., Sharma, M., Parveen, S., Hashem, F., May, E. B., Handy, E. T., et al. (2019). Prevalence of shiga-toxigenic and atypical enteropathogenic Escherichia coli in untreated surface water and reclaimed water in the Mid-Atlantic U.S. Environ. Res. 172, 630–636. doi: 10.1016/j.envres.2019.02.019
He, F., Zhao, D., and Paul, C. (2010). Field assessment of carboxymethyl cellulose stabilized iron nanoparticles for in situ destruction of chlorinated solvents in source zones. Water Res. 44, 2360–2370. doi: 10.1016/j.watres.2009.12.041
Henderson, A. D., and Demond, A. H. (2007). Long-term performance of zero-valent iron permeable reactive barriers: a critical review. Environ. Eng. Sci. 24, 401–423. doi: 10.1089/ees.2006.0071
Herzberg, M., and Elimelech, M. (2007). Biofouling of reverse osmosis membranes: role of biofilm-enhanced osmotic pressure. J. Memb. Sci. 295, 11–20. doi: 10.1016/j.memsci.2007.02.024
Hozalski, R. M., Zhang, L., and Arnold, W. A. (2001). Reduction of haloacetic acids by Fe0: implications for treatment and fate. Environ. Sci. Tech. 35, 2258–2263. doi: 10.1021/es001785b
Ingram, D. T., Callahan, M. T., Ferguson, S., Hoover, D. G., Shelton, D. R., Millner, P. D., et al. (2012). Use of zero-valent iron biosand filters to reduce Escherichia coli O157: H12 in irrigation water applied to spinach plants in a field setting. J. Appl. Microbiol. 112, 551–560. doi: 10.1111/j.1365-2672.2011.05217.x
Johnson, R. L., Nurmi, J. T., Johnson, G., Fan, D., Johnson, R., Shi, Z., et al. (2013). Field-scale transport and transformation of carboxymethylcellulose-stabilized nano zero-valent iron. Environ. Sci. Technol. 47, 1573–1580. doi: 10.1021/es304564q
Kocur, C. M., Chowdhury, A. I., Sakulchaicharoen, N., Boparai, H. K., Weber, K. P., Sharma, P., et al. (2014). Characterization of nZVI mobility in a field scale test. Environ. Sci. Techn. 48, 2862–2869. doi: 10.1021/es4044209
Kulkarni, P., Raspanti, G. A., Bui, A. Q., Bradshaw, R. N., Kniel, K. E., Chiu, P. C., et al. (2019). Zerovalent iron-sand filtration can reduce the concentration of multiple antimicrobials in conventionally treated reclaimed water. Environ. Res. 172, 301–309. doi: 10.1016/j.envres.2019.02.012
Lee, C., Kim, J. Y., Lee, W. I., Nelson, K. L., Yoon, J., and Sedlak, D. L. (2008). Bactericidal effect of zero-valent iron nanoparticles on Escherichia coli. Environ. Sci. Technol. 42, 4927–4933. doi: 10.1021/es800408u
Marik, C., Anderson, B., Gartley, S., Craighead, S., Bradshaw, R., Kulkarni, P., et al. (2019). ZVI filtration reduced Listeria innocua in pond water compared to sand filtration. Environ. Res. 173, 33–39. doi: 10.1016/j.envres.2019.02.028
Noubactep, C. (2009). An analysis of the evolution of reactive species in Fe0/H2O systems. J. Hazard. Mater. 168, 1626–1631. doi: 10.1016/j.jhazmat.2009.02.143
Odziemkowski, M., Gui, L., and Gillham, R. (2000). Reduction of N-nitrosodimethylamine with granular iron and nickel-enhanced iron. 2. mechanistic studies. Environ. Sci. Tech. 34, 3495–3500. doi: 10.1021/es9909780
Pearson, C., Hozalski, R., and Arnold, W. (2005). Degradation of chloropicrin in the presence of zero-valent iron. Environ. Toxicol. Chem. 24, 3037–3042. doi: 10.1897/04-614Ra.1
Qin, H., Yin, D., Bandstra, J. Z., Sun, Y., Cao, G., and Guan, X. (2020). Ferrous ion mitigates the negative effects of humic acid on removal of 4-nitrophenol by zerovalent iron. J. Hazard. Mater. 383:121218. doi: 10.1016/j.jhazmat.2019.121218
R Core Team (2017). R: The R Project for Statistical Computing. Avaliable online at: http://www.R-project.org/ (accessed September 15, 2019).
Scharff, R. L. (2010). Health-related costs from foodborne illness in the United States. Available online at: http://www.producesaf-etyproject.org/admin/assets/files/Health-Related-Food-borne-Illness-Costs-Report.pdf-1.pdf (accessed September 29, 2011).
Shah, M. K., Bradshaw, R., Nyarko, E., Handy, E. T., East, C., Millner, P. D., et al. (2019). Salmonella Newport in soils amended with heat-treated poultry pellets survived longer and more readily transferred to and persisted on spinach. Appl. Environ. Microbiol. 85, 00334–00319. doi: 10.1128/AEM.00334-19
Sharma, M., Handy, E. T., East, C. L., Kim, S., Jiang, C., Callahan, M. T., et al. (2020). Prevalence of Salmonella and Listeria monocytogenes in non-traditional irrigation waters in the Mid-Atlantic United States is affected by water type, season, and recovery method. PLoS ONE 5:e0229365. doi: 10.1371/journal.pone.0229365
Sizirici, B., Yildiz, I., Al Ali, A., Alkhemeiri, A., and Alawadi, R. (2019). Modified biosand filters enriched with iron oxide coated gravel to remove chemical, organic and bacteriological contaminants. J. Water Process Eng. 27, 110–119. doi: 10.1016/j.jwpe.2018.11.015
Suri, M., Dery, J., Pérodin, J., Brassill, N., He, X., Ammons, S., et al. (2019). US farmers' opinions on the use of nontraditional water sources for agricultural activities. Environ. Res. 172, 345–357. doi: 10.1016/j.envres.2019.02.035
Tomás-Callejas, A., López-Velasco, G., Camacho, A. B., Artés, F., Artés-Hernández, F., and Suslow, T. V. (2011). Survival and distribution of Escherichia coli on diverse fresh-cut baby leafy greens under preharvest through postharvest conditions. Int. J. Food Microbiol. 151:216–222. doi: 10.1016/j.ijfoodmicro.2011.08.027
United States Department of Agriculture (USDA) (2019). 2017 Census of Agriculture – United States. Available online at: https://www.nass.usda.gov/Publications/AgCensus/2017/Full_Report/Volume_1,_Chapter_1_US/usv1.pdf (accessed September 28, 2019).
United States Environmental Protection Agency (EPA) (2012). U S Environmental Protection Agency. 2012 Guidelines for Water Reuse, Development. Washington, DC: USEPA.
United States Food and Drug Administration (USFDA) (2018). Food Safety Modernization Act Final Rule on Produce Safety: Standards for the Growing, Harvesting, Packing, and Holding of Produce for Human Consumption. Silver Spring, MD: USFDA.
Weller, D., Wiedmann, M., and Strawn, L. K. (2015). Irrigation is significantly associated with an increased prevalence of Listeria monocytogenes in produce production environments in New York state. J. Food Prot. 78, 1132–1141. doi: 10.4315/0362-028X.JFP-14-584
Xie, L. I., and Shang, C. (2005). Role of humic acid and quinone model compounds in bromate reduction by zerovalent iron. Environ. Sci. Technol. 39, 1092–1100. doi: 10.1021/es049027z
Yin, W., Wu, J., Ping, L., Wang, X., Zhu, X., Wu, P., and Yang, B. (2012). Experimental study of zero-valent iron induced nitrobenzene reduction in groundwater: The effects of pH, iron dosage, oxygen and common dissolved anions. Chem. Eng. J. 184, 198–204. doi: 10.1016/j.cej.2012.01.030
You, Y., Han, J., Chiu, P., and Jin, Y. (2005). Removal and inactivation of waterborne viruses using zerovalent iron. Environ. Sci. Tech. 39, 9263–9269. doi: 10.1021/es050829j
Zhang, L., Arnold, W. A., and Hozalski, R. M. (2004). Kinetics of haloacetic acid reactions with Fe (0). Environ. Sci. Technol. 38, 6881–6889. doi: 10.1021/es049267e
Keywords: Escherichia coli, irrigation, agricultural water, sand filtration, zero-valent iron
Citation: Kim S, Bradshaw R, Kulkarni P, Allard S, Chiu PC, Sapkota AR, Newell MJ, Handy ET, East CL, Kniel KE and Sharma M (2020) Zero-Valent Iron-Sand Filtration Reduces Escherichia coli in Surface Water and Leafy Green Growing Environments. Front. Sustain. Food Syst. 4:112. doi: 10.3389/fsufs.2020.00112
Received: 29 September 2019; Accepted: 22 June 2020;
Published: 31 July 2020.
Edited by:
Mathias Neumann Andersen, Aarhus University, DenmarkReviewed by:
Yaosheng Wang, Chinese Academy of Agricultural Sciences, ChinaCopyright © 2020 Kim, Bradshaw, Kulkarni, Allard, Chiu, Sapkota, Newell, Handy, East, Kniel and Sharma. This is an open-access article distributed under the terms of the Creative Commons Attribution License (CC BY). The use, distribution or reproduction in other forums is permitted, provided the original author(s) and the copyright owner(s) are credited and that the original publication in this journal is cited, in accordance with accepted academic practice. No use, distribution or reproduction is permitted which does not comply with these terms.
*Correspondence: Manan Sharma, bWFuYW4uc2hhcm1hQHVzZGEuZ292
†Present address: Sarah Allard, Department of Pediatrics, University of California San Diego School of Medicine, San Diego, CA, United States
Disclaimer: All claims expressed in this article are solely those of the authors and do not necessarily represent those of their affiliated organizations, or those of the publisher, the editors and the reviewers. Any product that may be evaluated in this article or claim that may be made by its manufacturer is not guaranteed or endorsed by the publisher.
Research integrity at Frontiers
Learn more about the work of our research integrity team to safeguard the quality of each article we publish.