- Food Quality & Design, Wageningen University & Research, Wageningen, Netherlands
This study aimed at effective fractionation of Hermetia illucens into lipid and protein, considering the insects' anatomy. A hybrid approach was developed, separating the exoskeleton by mechanical pressing and fractionating the slurry by sonication and enzymatic treatment. Microscopy showed that the peripheral fat bodies were tightly attached to muscle fibers. Blanching caused protein aggregation and entrapment of fat in the protein matrix, resulting in 25.0% loss in fat extraction, compared to only 3.3% loss for unblanched larvae. After centrifugation of the juice obtained from unblanched larvae a cream layer rich of lipids and proteins, a supernatant with soluble protein, and a pellet including mainly insoluble proteins were obtained. Blanched juice centrifugation formed a lipid fraction, a reduced cream layer, and more pellet. Whereas, sonication hardly affected the fractionation, enzymatic treatment resulted in solubilisation of pellet protein and an increased lipid layer. In conclusion, processing without blanching is very effective to extract more lipids (>80%) from whole larvae in the form of a cream layer, while blanched-enzymatic treatment allows the extraction of lipids in a clear lipid layer (>40%). In both cases, around 50% of protein was recovered in the residue and the other 50% mainly in the pellet after centrifugation.
Introduction
Insects are recognized as bio-convertors of organic side streams into sustainable biomass (Cičková et al., 2015). As such, insects show great promise in the management of waste, producing marketable food and feed products, fuels, and chemicals that favor a more circular economy.
The black soldier fly (BSF; Hermetia illucens) is one of the most studied insect species in this regard. Factors determining its success in the biodegradation process include its ability to be reared and harvested in a controllable manner within a short life cycle (22–24 days), and its capability to grow on a wide range of substrates, e.g., by-products from food processing, animal waste and organic waste (Tomberlin et al., 2006). The BSF can efficiently convert these substrates in high quality nutrients like proteins, lipid, and minerals. As a result, BSF larvae have been used as feed for a variety of animals, including swine, poultry, and fish (Henry et al., 2015; Barragan-Fonseca et al., 2017; Belghit et al., 2018), and are being explored as an important ingredient for pet food (Bosch et al., 2014). Larvae of BSF also offer a green approach for the production of biodiesel (Li et al., 2011; Wang et al., 2017).
In order to convert BSF larvae biomass into biomolecules, suitable fractionation processes are needed to obtain high value marketable products, including soluble and insoluble protein, lipid and chitin fractions. Studies on the fractionation of BSF include solvent extraction (Tzompa-Sosa et al., 2014; Purschke et al., 2016; Surendra et al., 2016) mechanical separation (Kroeckel et al., 2012; Tschirner and Simon, 2015) and also aqueous extraction as more sustainable alternative (Yi et al., 2013; Tzompa-Sosa et al., 2014). Unfortunately aqueous extraction is characterized by a low oil yield even after high centrifugation forces, likely because of oil-in-water emulsions and entrapment of lipids in insoluble solids (Tzompa-Sosa et al., 2019).
To optimize the fractionation process, an in-depth investigation of the material structure is needed. However, no studies have been conducted to understand the role of the insect anatomy in relation with the release of lipids from the biomass. Insects have been considered as a source of lipid with little or no attention to the compartmentalization of tissues. In insects, lipids are stored in trophocytes (adipocytes), which are the major cells of a storage organ, the fat body (Šobotník et al., 2006). The insect fat body plays an essential role in energy storage and utilization, and it is the central storage depot for excess nutrients. As in mammals, the fat body is an organ controlling the synthesis and utilization of energy reserves—fat and glycogen—but also synthesizing most of the hemolymph proteins and circulating metabolites. The anatomy of the two different types of fat body, perivisceral (surrounding the alimentary tract; located in the abdominal cavity) and peripheral (subcuticular; located in between the skin and musculature), should be understood and considered during the fractionation process. The same approach should be followed for insect proteins which can be classified as hemolymph, muscle and cuticular proteins (Chapman, 2013) with different distribution and performance in the fractionation.
In this study, we explored the anatomy of BSF larvae with regard to lipid distribution and its effect on the fractionation process and studied the role of the protein matrix in fractionation of lipids from protein. A hybrid fractionation approach was taken, where the exoskeleton was separated by mechanical pressing and fractionation of nutrients was based on aqueous (in)solubility aided with sonication and enzymatic proteolysis.
Materials and Methods
Larval Anatomy
Larvae were kindly provided by the lab. colony of the Laboratory of Entomology (Wageningen University and Research, Wageningen, The Netherlands) and ranged approximately from 14 days old to pre-pupal age. For the anatomical study, multiple larvae were anesthetized with CO2, refrigerated at 4°C and re-anesthetized with CO2. Then the specimen was placed on the preparation plate under a preparation stereomicroscope (Olympus SZX12 10383, city, country). Larvae were submerged in a Phosphate buffered saline solution (Sigma Aldrich, St. Luis, USA), prepared by dissolving 1 tablet in 100 ml distilled water, to prevent desiccation and maintain the larval osmotic pressure. The specimen was dissected along the abdomen and the gut was removed to provide maximum visibility of the fat body. Images of the larvae body were taken with a stereoscope camera (Euromex Holland, CMEX DC.5000, Color USB2 Camera). Subsequently, the perivisceral and peripheral fat body was harvested with tweezers, respectively, from the abdomen and from the exoskeleton, collected in PBS solution and analyzed under a light microscope (Axioskop 2 Plus with Axiocam ERc 5s, Carl Zeiss microscopy, Thornwood, USA).
Sample Preparation and Pre-treatment for Fractionation
Fresh larvae of BSF were sourced from BESTICO B.V. (Berkel & Rodenrijs, The Netherlands) and kept alive in plastic containers at 4°C for maximum 2 days before the experiments. Processing was carried out as shown in Figure 1. To test the effect of blanching on the mechanical separation, 100 g of larvae were immersed in 10 L water at 90°C for 5 min, subsequently kept in cold water for 1 min and sieved to remove excess water. This process is being referred to as blanching throughout this paper. The non-blanched larvae were washed for 5 min in cold water and sieved to remove the excess water.
Hybrid Fractionation
The fractionation scheme is summarized in Figure 1. Both blanched and non-blanched larvae were mechanically processed with a slow juicer (mod. Angelia 8500 S, Angel Juicer Co., LTD, Busan, South Korea) to separate the fibrous residue (washed residue –WR and blanched residues—BR) from the liquid extract, which is referred to as juice. Fractionation was aided with sonication and/or proteolytic hydrolysis. About 40 mL of juice was poured in 50 mL Greiner tubes and further processed with a sonication step for 30 min at 60°C (sonicator with Ultrasonic Bath Box by Sonication GmbH, Biberach, Germany). Sonication was for part of the samples combined with enzyme treatment at 60°C for 1 h (Alcalase 2.4L, P4860 Sigma-Aldrich, Saint Louis, USA), with enzymatic activity of 2.4 AU-A/g. Also, part of the samples underwent enzyme treatment without the preceding sonication step. Preliminary experiments showed a limited protein denaturation at 60°C measured as percentage of pellet fraction weight. After Alcalase addition (5% w/w of enzyme on juice dry matter), samples were mixed for 30 s on a vortex (VV3, VWR International B.V., Amsterdam, The Netherlands) to distribute the enzyme through the juice. Samples of blanched-enzyme treated (BE) and blanched-sonicated-enzyme treated (BSE) were submitted to a heating treatment at 90°C for 10 min to deactivate enzymes, while washed samples were not heated to 90°C. Before centrifugation, the pH was adjusted to 7.0 and all samples were heated to 40°C to stabilize their viscosity and centrifuged at 4000 g for 10 min. After centrifugation, the slurry distributed in four different layers, which from the bottom were identified as pellet, supernatant, cream, and a lipid layer at the top Sample preparation and fractionation was carried out in triplicate.
Moisture, Crude Protein and Lipid Content
Each of the four layers obtained after centrifugation, including the fibrous residue, was weighed. The moisture content of each fraction was analyzed by weighing before and after drying at 100°C for 24 h. The nitrogen content was determined by the Dumas method (Flash EA 1112 Protein analyzer with Eager 200 software, ThermoFisher Scientific Inc., Waltham, USA) and the crude protein content was determined using the insect specific conversion factor of 5.6 (Janssen et al., 2017). Crude lipid content was determined gravimetrically by petroleum ether extraction using Soxhlet extraction. Lipid and protein extraction yield was expressed as relative to the initial lipid and protein content in the whole larvae and in the juice, based on dry matter.
Degree of Protein Hydrolysis
The degree of protein hydrolysis of the supernatant was assessed by determining the content of free α-amino groups (NH2) in supernatant from W (washed), B (blanched) and BE (blanched and enzymatically treated). Free α-amino groups were determined after reaction with ortho-phthalaldehyde (OPA) according to Nielsen et al. (2001) with minor modifications. For quantification of NH2 groups, a calibration curve of L-serine (0.1 to 1.6 mM) was made and the degree of hydrolysis was computed according to Equation 1,
In Equation 1 NH2 (final) corresponds to the concentration of free amino groups in the processed sample after hydrolysis, NH2 (initial) is the concentration of free amino groups in the sample before hydrolysis, and NH2 (acid) reflects the total content of free amino groups in the sample completely hydrolyzed in 6 N HCl at 110°C for 24 h.
Microstructural Characterization
The distribution of protein and lipid in the fibrous residue, cream and pellet was analyzed by confocal laser scanning microscopy based on fluorochrome binding affinity. The fluorescent dyes rhodamine B (CAS 509-34-2) and BODIPY 505/515 (CAS 21658-70-8) were used to stain, respectively, proteins and lipids. The cream layer and pellet were diluted with demineralized water in a ratio 1:1 to reach a final concentration of 0.001% for rhodamine B and BODIPY 505/515. Approximately 50 mg of the stained mixture was uniformly distributed on glass slides and samples were visualized through a confocal scanning laser microscope (CLSM) type 510 (Zeiss, Oberkochen, Germany) using a 543 nm HeNe laser for rhodamine B and a 488 nm argon laser for BODIPY. Images were acquired using a 10/20 EC Plan-Neofluar/0.5 A lens and analyzed with ZEN blue edition (Carl Zeiss Microscopy).
Statistics
All experiments were performed at least in duplicate. Data were analyzed by ANOVA with StatSoft, and significant differences (p < 0.05) among means were identified using post hoc analysis by LSD testing.
Results and Discussions
Anatomy of the Fat Body
Two different fat body (FB) types can be distinguished in the insect larvae: the ones that surround the organs in the body cavity (perivisceral FB) and the one directly under the skin (peripheral FB; Buys, 1924; Coupland, 1957). As shown in Figure 2, this was also found in BSF larvae. The perivisceral FB (encircled in Figure 2A) was white to pale yellow in color, showing a ribbon like structure, with a flat, windy structure. It interlinked at times, but could also have longer, straight parts. Contrary to the perivisceral FB, the peripheral FB (encircled in Figure 2B) had a more amorphous organ structure, visible on the inside of the exoskeleton.
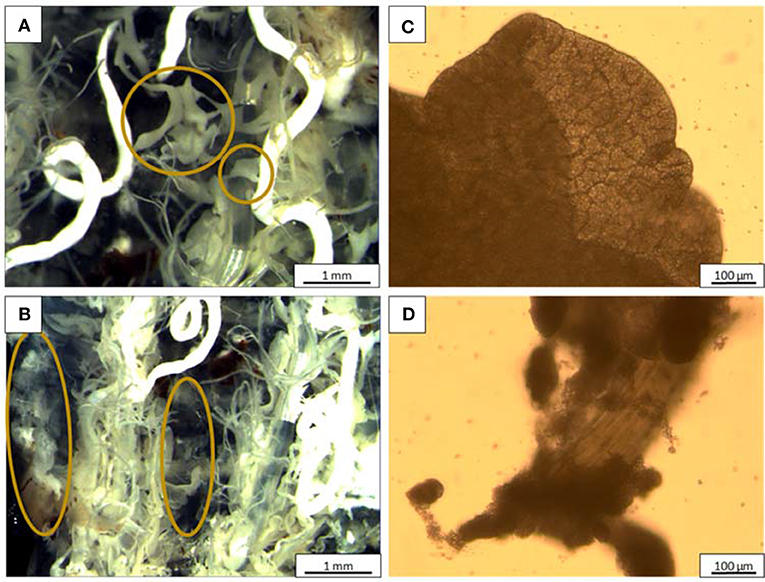
Figure 2. Stereomicroscopy and light microscopy images, respectively, of (A,C) perivisceral fat body; (B,D) peripheral fat body. The organs encircled in (A,B) are, respectively, the perivisceral and peripheral FBs.
The positioning of the FB in BSF corresponded with the anatomy reported for Lutzomyia longipalpis, Drosophilia larvae and Migdolus fryanus larvae (Marchetti et al., 2003; Assis et al., 2014; Nakayama et al., 2017) and the generally described FB placement and structure for insects (Buys, 1924; Dean et al., 1985; Keeley, 1985). The structure of the fat body also corresponds with that found in other insects, like termites (Costa-Leonardo et al., 2013) and locusts (Coupland, 1957). It is reasonable to assume that the FB structure at cell level will be similar to other insect species as well, with metabolic cells in the outer layer of the FB to ensure maximum access to the nutrients in the hemolymph, and storage-excretion cells in the center of the FB lobe (Keeley, 1985).
A closer investigation with light microscopy allowed to observe the perivisceral FB anatomy on a cellular level (Figure 2C), with a typical cell shaped structure filled with oil droplets. A denser structure could be seen in the investigated peripheral FB (Figure 2D), so that no individual cells were observed. Interestingly, the peripheral FBs were tightly attached to a muscle fiber and this feature has significant implications during fractionation.
Effect of Blanching on Mechanical Separation
The process of mechanical separation to obtain washed and blanched is summarized in Figure 1, while the mass balance is shown in Figure 3. In the washed samples, 92% of the total fat was extracted into the juice by the mechanical separation, whereas for the blanched sample only 72% of the fat was found into the juice, while the remaining fat was entrapped in the fibrous residue. Chemical data of the juice composition showed the fat content of juice from washed larvae (WJ) was significantly higher than juice from blanched (BJ) (P < 0.014). However, the amount of protein in the WJ and BJ juice is the same i.e., 6.8 kg protein from 100 kg fresh weight larvae. The higher relative percentage of protein in BJ (32.3%) compared to 28.8% in WJ is explained by the lower fat content, making the share of protein larger in the total juice weight. The mass labeled as “Other” in Figure 3 refers to any dry matter that was not measured in the fat or protein analyses, for example carbohydrates. Some mass was also lost in the juicer, as it was not possible to completely empty the final residue during a production run. Therefore, some material seems to disappear from the mass balance. In our experiments, 421 g of larvae were needed to obtain 100 g of extracted dry material from washed larvae, and 470 g of larvae when blanching was applied. Azzollini et al. (2016) reported a leakage of protein into the surrounding medium during blanching of Yellow mealworms (Tenebrio molitor), explaining the loss in the total protein. For industrial application, this loss could be prevented by heating larvae directly into an extruder, which can also exert a higher pressure, favoring a better extraction. Further, the WJ was highly prone to uncontrolled blackening caused by polyphenol oxidation and iron complex formation (Janssen et al., 2019).
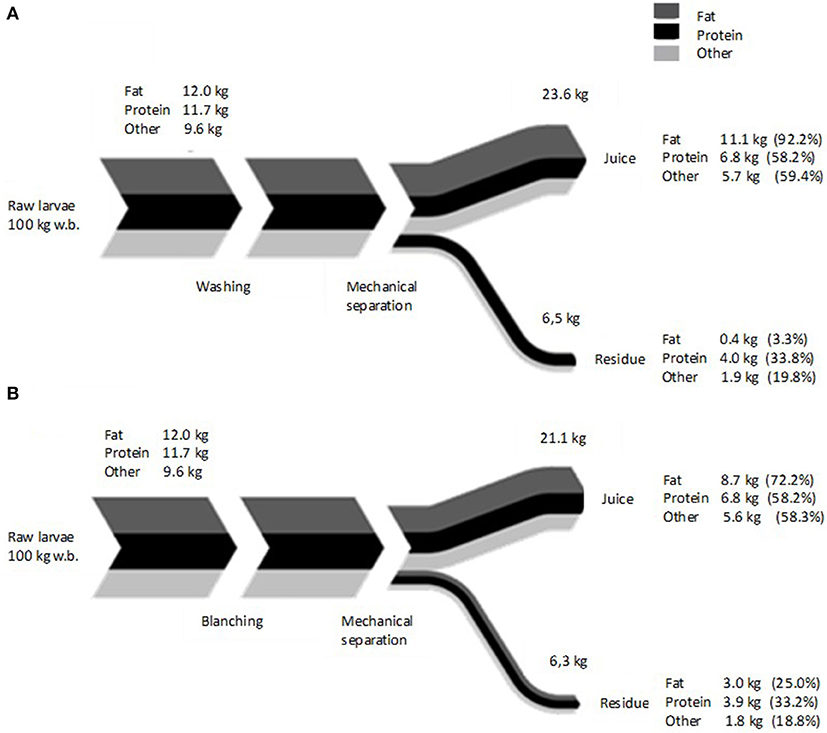
Figure 3. Average mass balance of (A) washed, and (B) blanched larvae submitted to mechanical separation. Data are based on 100 kg larval fresh basis, n = 3. Values in brackets represent the percentage of recovered component relative to the initial quantity in fresh insects. Line thickness represents the value of the fractions relative to each other.
Results indicated that blanching caused a loss in lipid extraction from the juice to the residual fraction. About 25% of fat was found in the residue when blanching was applied, compared to only 3.3%, when larvae were only washed. Looking at the anatomy of the larvae, it is possible to hypothesize that protein denaturation, due to blanching, created some physical constrains that limited the extractability of lipids. Due to the entangled position of the peripheral fat body, it is likely that cuticular and muscle protein formed aggregates that include fat (bodies) which limits the extraction. The analysis of the residues by confocal microscopy, showed in Figure 4 supported this hypothesis. Much smaller amounts of lipids are found in the residue from washed larvae compared to blanched larvae. Lipids in blanched larvae are surrounded by protein and connected to the chitin exoskeleton as clearly visible by the areolate surface.
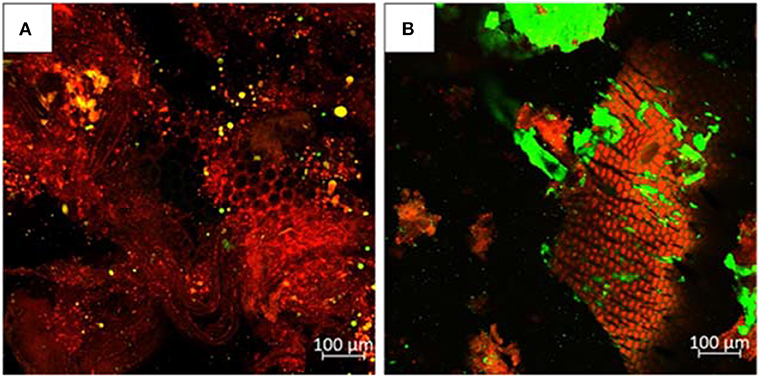
Figure 4. Confocal microscopy of the BSF residues obtained by (A) WR; (B) BR. Red color indicates protein, green color indicates lipid.
Fractionation by Assisted Aqueous Extraction
The centrifugation of insect juice obtained after the various treatment fractionated it in multiple fractions. Namely, a clear lipid layer, a cream layer containing lipids and proteins, a supernatant of water and soluble proteins and a pellet of insoluble protein and most likely part of chitin that remained after mechanical separation. Depending on the processing conditions the yield of the various fractions are significantly different as summarized in Figure 5. Visible differences were observed between the differently pre-treated samples. The overall fraction distribution was not very different comparing W with WS, B with BS and BE with BSE, respectively, indicating little effect of sonication. Samples obtained from washed larvae revealed the largest amount cream layer, where sonication further increased the amount of cream. All blanched samples showed a substantial reduction in the mass of cream and the formation of an additional pure lipid layer. A general increase of its amount with the proteolytic treatment was observed. These results suggested that heating during blanching caused a damage of the emulsion, possibly created during mechanical separation, that was further disrupted by the enzymatic hydrolysis.
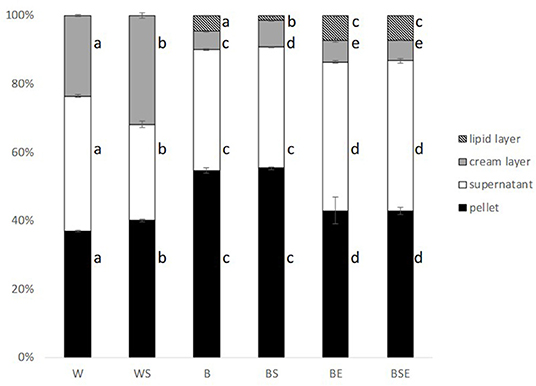
Figure 5. Weight based fraction distribution of centrifuged samples after different treatments (fresh basis). W, washed; WS, washed and sonicated; B, blanched; BS, blanched and sonicated; BE, blanched and enzyme; BSE, blanched; sonicated and enzyme treated. (n = 3; error bars represent standard deviation) Different letters indicate statistical difference (p < 0.05).
Looking at the protein distribution among the fractions, samples of B and BS showed the highest amount of pellet as opposed to washed samples, clearly due to the protein denaturation and precipitation caused by blanching. Moreover, a decrease in the mass of pellet was visible after hydrolysis, suggesting that the enzymatic treatments were efficient in the re-solubilization of denatured protein.
Effect of Pre-treatments on Distribution of Lipid and Proteins
The distribution of lipid and protein after centrifugation of samples is displayed in Figure 6. All blanched samples contained a lipid layer, cream, supernatant and a pellet fraction. As reported, in washed sample (W), lipids were mostly found in the cream layer, and a small amount was present in the pellet. For the blanched sample (B), more lipids were situated in the pellet. Significantly less lipid was found in the cream and pellet of the enzymatically treated samples, as of BE and BSE.
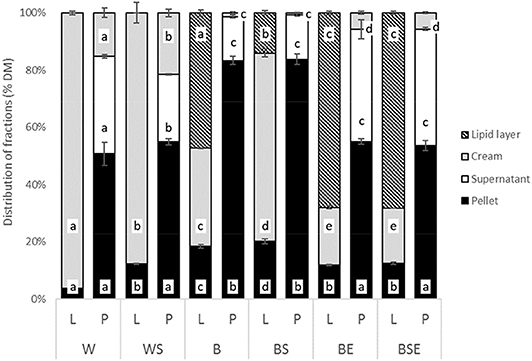
Figure 6. Lipid (L) and protein (P) distribution (% w/w on dry matter). (W, washed; WS, washed and sonicated; B, blanched; BS, blanched and sonicated; BE, blanched and enzyme treated; BSE, blanched; sonicated and enzyme treated. n = 3, error bars represent standard deviations. Different letters in different fractions among L bars and P bars indicate statistical difference (p < 0.05).
Figure 7 shows the microstructure of the cream and pellet fraction analyzed by confocal microscopy. Contrary to the pellet from washed larvae, blanched larvae showed protein aggregation that led to lipid entrapment, and consequently limited lipid extraction. It is also visible how protein hydrolysis reduced the sizes of aggregates, favoring lipid fractionation. This indicated that the protein matrix plays an important role in the lipid extraction, since no cell-like organized structure was visible in W pellet or cream. The mechanical separation effectively disrupted the fat body tissues, favoring lipid separation. Interestingly, sonication had limited effect on lipid extractability of blanched larvae, but a negative effect on washed larvae, suggesting the tendency to emulsify lipid to protein. Apart from mechanical constrains, the chemical structure and affinity of fat with different fractions may have hampered its extraction.
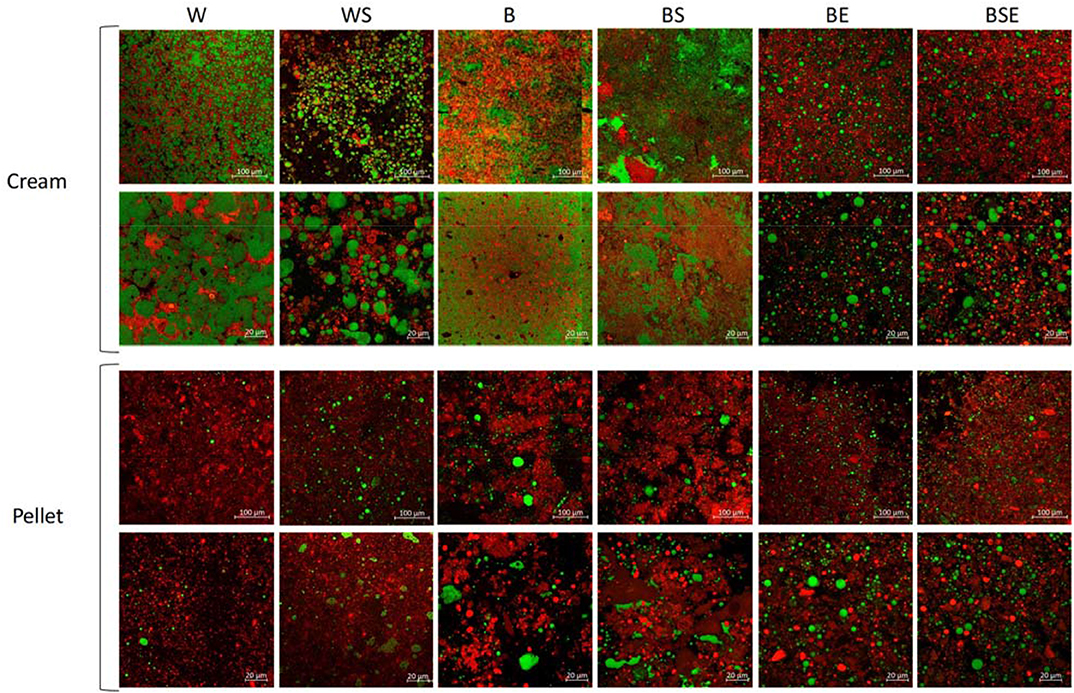
Figure 7. Confocal microscopy of cream and pellet 20x and 63x magnification from W, washed larvae; WS, washed sonicated; B, blanched; BS, blanched sonicated; BE, blanched enzymatically treated; BSE, blanched sonicated enzymatically treated. Red color indicates protein, green color indicates lipid.
Very limited amount of lipid is visible in the CSLM images of the W pellet (Figure 7). In fact, in terms of yield with respect to the amount of lipids in the juice, the lipid extraction ranged from 81% (W) to 52% (BS) (Figure S1 Supplement). Part of this finding could be explained by the different yield occurring after mechanical extraction. In fact, as also shown in Figure 3, 92% of fat was extracted in the juice in the washed samples, compared to 72% of fat when heating was applied. Of the total lipids present in the larvae prior to aqueous extraction, between 9% (BS) and 48% (BSE) were recovered via the lipid layer. In the washed samples, when sonication was applied, the extraction yield decreases from 81% (W) to 67% (WS). This was the opposite of what it was intended with the use of sonication.
In literature, lipid extraction yields were found of 87 and 90% for solvent extraction (Surendra et al., 2016; Caligiani et al., 2018), 40% for mechanical extraction (Surendra et al., 2016) and 60-18% for aqueous extraction (Tzompa-Sosa et al., 2019). In the latter paper, five different insect species, but not BSF, were investigated. Furthermore, the extraction method used was different: insects were blended with an immersion blender in water (1:4 ratio), and centrifuged at 4°C with 15000 g, compared to 4000 g employed in this paper. Also, no extra water was added in our research to aid the separation of the fractions in the juice, adding to the efficiency of further processing of the fractions. Comparing the two methods, two large differences can be highlighted. Firstly, the mechanical separation in the slow juicer adopted in this paper, compared to the blender, allowed a better breakage of the fat cells and consequent leakage of lipids in the liquid matrix. Secondly, in our work, the whole matrix was heated to 40°C before centrifugation, allowing the melting of the more-saturated fatty acids, which may consequently have been released from the protein matrix. Caligiani et al. (2018) assisted the fractionation of BSF larvae by means of proteolytic enzymes, obtaining only 10% lipid extraction yield. Possibly, the low yield was due to the mincing technique used to grind the insects, as opposed to the mechanical separation used in this research, which improved the disruption of tissues. It can be concluded that the BE and BSE extraction methods constitute an advancement in the aqueous extraction protocols found in literature (Tzompa-Sosa et al., 2014, 2019; Caligiani et al., 2018), and performed better than the mechanical extraction based on compression of previously dried larvae. Furthermore, the unassisted extraction of fresh larvae (sample W) was as successful as the solvent extraction in extracting lipid from BSF larvae, but produced an emulsified cream that would require further refinery. At a microstructural level, the cream in native larvae (W and WS) was an oil in water emulsion stabilized by protein, with lipid droplets of ~20 μm. Blanching intensely modified the nature of the emulsion, forming a very fine and compact structure similar to a coagulum, ultimately loosen by proteolysis allowing lipid separation.
Regarding protein distribution with respect to the juice in the samples without any treatment (W) about 50% of the protein was situated in the pellet, 35% in the supernatant and 15% in the cream. When larvae are blanched (B) more than 80% of protein was located in the pellet due to protein aggregation caused by temperature denaturation. After enzyme hydrolysis, about 30% of protein was solubilized. The degree of protein hydrolysis in supernatant protein was measured by OPA. The enzymatic treatment showed the highest hydrolysis of protein (41% ± 2% in BE), followed by blanched samples (35 ± 2% in B) and washed samples (15 ± 3% in W). Furthermore, results of DH show that protein in the supernatant of blanched samples are made of smaller peptides compared to native protein from washed larvae. As a result, protein extracted in the supernatant by enzymatic treatment could be easily hydrolyzed to peptides and free amino acids during digestion.
In all samples, the total protein yield calculated based on whole larvae was ~54–58% (Figure S2, supplemental material). Our data can be compared with those obtained by Caligiani et al. (2018) who also performed an enzyme-assisted extraction of BSF obtaining a yield from 47 to 67% for the proteins in the supernatant, whereas in our research the highest protein yield in the supernatant was only 23% (BSE). This discrepancy could be due to (i) the different duration of the enzyme treatment, (overnight in Caligiani et al. (2018) and 60 min in this research); (ii) the use of buffer that enhances protein solubility while in our research no extra liquid was added; (iii) the loss of protein due to blanching in our research, compared to the enzymatic hydrolysis on the entire larvae.
Conclusion
This study stemmed from the anatomy of fat body distribution in the BSF larvae, to provide insights for the design of a fractionation system that takes into account the biological nature of the insect.
The selection of the appropriate processing for BSF depends on several consideration. Larvae washing is needed to remove the debris and other residues while blanching is important to ensure microbiological and biochemical stability. Our data indicate that washing did not influence the extractability of lipids, however the W-juice was highly prone to uncontrolled blackening caused by polyphenol oxidation and iron complex formation (Janssen et al., 2019).
The hybrid fractionation approach designed, based on the mechanical and enzymatic assisted separation of the larvae tissue from the exoskeleton, was very effective in terms of protein and lipid fractionation. Compared to aqueous extraction protocols found in literature, the methods described in this paper do not foresee the addition of extra water, exploiting the water present in fresh larvae, thereby limiting the energetically intense evaporation treatment. Another advantage of this method was to separate in the first step chitin from lipid and protein, also without protein denaturation. Using this separation principle different fractions of soluble protein, insoluble protein, lipid and cream could be obtained, of which the yield and composition can be modulated by choosing combinations between blanching and enzymatic proteolysis. With respect to the insects' anatomy, protein denaturation lead to entrapment of fat thereby hampering the fractionation between fat and protein.
Looking at potential application for each fraction the soluble protein could replace the water–soluble protein used as a plasticizer in the fish feed extrusion process while the insoluble protein could be employed as fillers in fish feed. In addition, fat in the form of a heat stable emulsion may find novel uses in multiple food and feed applications. Furthermore, fat can be further fractionated emulsifiers, both of high economical relevance.
Data Availability Statement
The datasets generated for this study are available on request to the corresponding author.
Author Contributions
VF, DA, and CL designed the study. AI and DA performed the experiment and analyzed the data. AI, DA, VF, and CL wrote the paper.
Funding
This study was supported by the Norwegian Research Council, project ENTOFÔR: from waste to resource, Grant No. 268344.
Conflict of Interest
The authors declare that the research was conducted in the absence of any commercial or financial relationships that could be construed as a potential conflict of interest.
Supplementary Material
The Supplementary Material for this article can be found online at: https://www.frontiersin.org/articles/10.3389/fsufs.2020.00080/full#supplementary-material
References
Assis, W. A., de Malta, J., Pimenta, P. F. P., Ramalho-Ortigão, J. M., and Martins, G. F. (2014). The characterization of the fat bodies and oenocytes in the adult females of the sand fly vectors lutzomyia longipalpis and phlebotomus papatasi. Arthrop. Struct. Dev. 43, 501–509. doi: 10.1016/j.asd.2014.05.002
Azzollini, D., Derossi, A., and Severini, C. (2016). Understanding the drying kinetic and hygroscopic behaviour of larvae of yellow mealworm (Tenebrio molitor) and the effects on their quality. J. Insect. Food Feed 2, 233–243. doi: 10.3920/JIFF2016.0001
Barragan-Fonseca, K. B., Dicke, M., and van Loon, J. J. A. (2017). Nutritional value of the black soldier fly (Hermetia illucens L.) and its suitability as animal feed – a review. J. Insect. Food Feed 3, 105–120. doi: 10.3920/jiff2016.0055
Belghit, I., Liland, N. S., Waagbø, R., Biancarosa, I., Li, Y., Pelusio, N., et al. (2018). Potential of insect-based diets for atlantic salmon (Salmo salar). Aquaculture 491, 72–81. doi: 10.1016/j.aquaculture.2018.03.016
Bosch, G., Zhang, S., Oonincx, D. G. A. B., and Hendriks, W. H. (2014). Protein quality of insects as potential ingredients for dog and cat foods. J. Nutr. Sci. 3, 1–4. doi: 10.1017/jns.2014.23
Caligiani, A., Marseglia, A., Leni, G., Baldassarre, S., Maistrello, L., Dossena, A., et al. (2018). Composition of black soldier fly prepupae and systematic approaches for extraction and fractionation of proteins, lipids and chitin. Food Res. Int. 105, 812–820. doi: 10.1016/j.foodres.2017.12.012
Chapman, R. F. (2013). The Insects - Structure and Function, 5th Edn. eds. S. J. Simpson and A. E. Douglas (Cambridge: Cambridge University Press).
Cičková, H., Newton, G. L., Lacy, R. C., and Kozánek, M. (2015). The use of fly larvae for organic waste treatment. Waste Manag. 35, 68–80. doi: 10.1016/J.WASMAN.2014.09.026
Costa-Leonardo, A. M., Laranjo, L. T., Janei, V., and Haifig, I. (2013). The fat body of termites: functions and stored materials. J. Insect Physiol. 59, 577–587. doi: 10.1016/j.jinsphys.2013.03.009
Coupland, R. E. (1957). Observations on the normal histology and histochemistry of the fat body of the locust (Schistocerca gregaria). J. Exp. Biol. 34, 290–296.
Dean, R. L., Locke, M., and Collins, J. V. (1985). “Intugement, respiration and circulation,” in Comprehensive Insect Physiology Biochemistry and Pharmacology, Vol. 3. eds. G. A. Kerkut and L. I. Gilbert (Pergamon: Pergamon Press, 155–210.
Henry, M., Gasco, L., Piccolo, G., and Fountoulaki, E. (2015). Review on the use of insects in the diet of farmed fish: past and future. Anim. Feed Sci. Technol. 203, 1–22. doi: 10.1016/j.anifeedsci.2015.03.001
Janssen, R. H., Canelli, G., Sanders, M. G., Bakx, E. J., Lakemond, C. M. M., Fogliano, V., et al. (2019). Iron-polyphenol complexes cause blackening upon grinding Hermetia illucens (black soldier fly) larvae. Sci. Rep. 9:2967. doi: 10.1038/s41598-019-38923-x
Janssen, R. H., Vincken, J.-P., van den Broek, L. A. M., Fogliano, V., and Lakemond, C. M. M. (2017). Nitrogen-to-protein conversion factors for three edible insects: Tenebrio molitor, Alphitobius diaperinus and Hermetia illucens. J. Agric. Food Chem. 65, 2275–2278. doi: 10.1021/acs.jafc.7b00471
Keeley, L. (1985). “Integument, respiration and circulation,” in Comprehensive Insect Physiology, Biochemistry and Pharmacology, Vol. 3. eds. G. Kerkut and L. Gilbert (College Station, TX: Pergamon Press, 211–242.
Kroeckel, S., Harjes, A. G. E., Roth, I., Katz, H., Wuertz, S., Susenbeth, A., et al. (2012). When a turbot catches a fly: evaluation of a pre-pupae meal of the black soldier fly (Hermetia illucens) as fish meal substitute - growth performance and chitin degradation in juvenile turbot (Psetta maxima). Aquaculture 364–365, 345–352. doi: 10.1016/j.aquaculture.2012.08.041
Li, Q., Zheng, L., Tomberlin, J. K., Qiu, N., Yu, Z., and Cai, H. (2011). Bioconversion of dairy manure by black soldier fly (Diptera: Stratiomyidae) for biodiesel and sugar production. Waste Manag. 31, 1316–1320. doi: 10.1016/j.wasman.2011.01.005
Marchetti, M., Fanti, L., Berloco, M., and Pimpinelli, S. (2003). Differential expression of the drosophila BX-C in polytene chromosomes in cells of larval fat bodies: a cytological approach to identifying in vivo targets of the homeotic Ubx, Abd-A and Abd-B proteins. Development 130, 3683–3689. doi: 10.1242/dev.00587
Nakayama, D. G., Santos Júnior, C. D., Kishi, L. T., Pedezzi, R., Santiago, A. C., Soares-Costa, A., et al. (2017). A transcriptomic survey of Migdolus fryanus (sugarcane rhizome borer) larvae. PLoS ONE 12:e0173059. doi: 10.1371/journal.pone.0173059
Nielsen, P. M., Petersen, D., and Dambmann, C. (2001). Improved method for determining food protein degree of hydrolysis. J. Food Sci. 66, 642–646. doi: 10.1111/j.1365-2621.2001.tb04614.x
Purschke, B., Stegmann, T., Matthias, S., and Jager, H. (2016). Pilot-scale supercritical CO2 extraction of edible insect oil from Tenebrio molitor L. larvae - influence of extraction on kinetics, defatting performance and compositional properties. Eur. J. Lipid Sci. Technol. 119:1600134. doi: 10.1002/ejlt.201600134
Šobotník, J., Weyda, F., Hanus, R., Cvačka, J., and Nebesárová, J. (2006). Fat body of Prorhinotermes simplex (Isoptera: Rhinotermitidae): ultrastructure, inter-caste differences and lipid composition. Micron 37, 648–656. doi: 10.1016/J.MICRON.2006.01.012
Surendra, K. C., Olivier, R., Tomberlin, J. K., Jha, R., and Khanal, S. K. (2016). Bioconversion of organic wastes into biodiesel and animal feed via insect farming. Renew. Energy 98, 197–202. doi: 10.1016/j.renene.2016.03.022
Tomberlin, J. K., Sheppard, D. C., and Joyce, J. A. (2006). Selected life-history traits of black soldier flies (Diptera: Stratiomyidae) reared on three artificial diets. Ann. Entomol. Soc. Am. 95, 379–386. doi: 10.1603/0013-874620020950379:slhtob2.0.co2
Tschirner, M., and Simon, A. (2015). Influence of different growing substrates and processing on the nutrient composition of black soldier fly larvae destined for animal feed. J. Insect. Food Feed 1, 249–259. doi: 10.3920/JIFF2014.0008
Tzompa-Sosa, D. A., Yi, L., van Valenberg, H. J. F., and Lakemond, C. M. M. (2019). Four insect oils as food ingredient: physical and chemical characterisation of insect oils obtained by an aqueous oil extraction. J. Insect. Food Feed 5, 279–292. doi: 10.3920/jiff2018.0020
Tzompa-Sosa, D. A., Yi, L., van Valenberg, H. J. F., van Boekel, M. A. J. S., and Lakemond, C. M. M. (2014). Insect lipid profile: aqueous versus organic solvent-based extraction methods. Food Res. Int. 62, 1087–1094. doi: 10.1016/j.foodres.2014.05.052
Wang, H., Rehman, K. U., Liu, X., Yang, Q., Zheng, L., Li, W., et al. (2017). Insect biorefinery: a green approach for conversion of crop residues into biodiesel and protein. Biotechnol. Biofuels 10:304. doi: 10.1186/s13068-017-0986-7
Keywords: Edible insects, Bio-fractionation, Black soldier fly, mechanical pressing, alcalase
Citation: Azzollini D, van Iwaarden A, Lakemond CMM and Fogliano V (2020) Mechanical and Enzyme Assisted Fractionation Process for a Sustainable Production of Black Soldier Fly (Hermetia illucens) Ingredients. Front. Sustain. Food Syst. 4:80. doi: 10.3389/fsufs.2020.00080
Received: 27 November 2019; Accepted: 04 May 2020;
Published: 23 June 2020.
Edited by:
Paula Jauregi, University of Reading, United KingdomReviewed by:
Afroditi Chatzifragkou, University of Reading, United KingdomLorenzo Pastrana, International Iberian Nanotechnology Laboratory (INL), Portugal
Cid Gonzalez Gonzalez, Acayucan Higher Technological Institute, Mexico
Copyright © 2020 Azzollini, van Iwaarden, Lakemond and Fogliano. This is an open-access article distributed under the terms of the Creative Commons Attribution License (CC BY). The use, distribution or reproduction in other forums is permitted, provided the original author(s) and the copyright owner(s) are credited and that the original publication in this journal is cited, in accordance with accepted academic practice. No use, distribution or reproduction is permitted which does not comply with these terms.
*Correspondence: Vincenzo Fogliano, dmluY2Vuem8uZm9nbGlhbm9Ad3VyLm5s