- 1School of Nutrition Sciences, Faculty of Health Sciences, University of Ottawa, Ottawa, ON, Canada
- 2Department of Chemistry and Biomolecular Sciences, Faculty of Science, University of Ottawa, Ottawa, ON, Canada
- 3Institute for Science, Society and Policy, University of Ottawa, Ottawa, ON, Canada
Various kinds of vesicles have been produced from plant, animal and inorganic materials for use as delivery vehicles especially in functional food formulation. However, major drawbacks associated with most of them include issues with sustainability, safety, biocompatibility, biorecognition, stability, bioavailability, bioadhesion, generation of reactive species, inefficient encapsulation and protection, and inability to release the bioactive compounds at target regions of the gastrointestinal tract. The use of vesicles innately formed in plant and animal cells as delivery agents would potentially solve most problems associated with the existing nanodelivery systems. Underutilized vesicles, known as exosomes, exist in plant and animal cells, where they play roles in cell communication and nutrient delivery. To date, exosomes have proven to be stable, biocompatible and able to withstand the activity of digestive enzymes until they reach their target locations. However, there is a need to explore better ways of inducing exosome production, to elucidate their physiological roles, and understand their biogenesis in plants, to discover sustainable methods of isolation of high yields of the vesicles. There is also a need to clarify the digestibility and interaction of the exosomes with blood and gastrointestinal fluids. This review highlights the isolation techniques and delivery potential of exosomes, and equally presents research gaps for enhancing the use of the natural vesicles for delivery purposes.
Introduction
During the past decade, there has been a pressing need for developing green, sustainable and biocompatible materials for the delivery of bioactive compounds within the food, pharmaceutical and medical industry. Extracellular vesicles, derived from plants and animals are considerably important in this area. Among the various extracellular vesicles, exosome, an intraluminal vesicle released when multivesicular bodies undergo exocytosis, have gained attention as a potential nanodelivery system for nutraceutical compounds. This is because of their various desirable properties such as small size, safety, biocompatibility, biorecognition, high stability and target specificity. Other extracellular vesicles such as microvesicles differ from exosomes in their biogenesis and size. Exosomes have a size range of 30–100 nm (Raposo and Stoorvogel, 2013) compared to sizes of 100–1,000 nm reported for most microvesicles that are derived mainly from outward budding of the plasma membrane (Meldolesi, 2016). For several years, the role of exosomes in normal physiological function was unknown. They were assumed to be waste products obtained from the shedding of plasma membranes. Further research has now proposed the participation of exosomes in various human physiological functions. As shown in Figure 1, exosome vesicles are composed of proteins, lipids and nuclear components that accumulate randomly from the cell in which they originate. Because of the way they are formed, their phospholipid bilayer exterior is made up of cell surface proteins, which allow them to take part in processes such as intercellular communication, exchange of materials with other cells, elimination of unwanted products from cells, and immune surveillance (Andaloussi et al., 2013; Beach et al., 2014). Whether it is by phagocytosis, endocytosis or activating another cell through the surface proteins, exosomes have been proven to be functional in communication and delivery in biological systems.
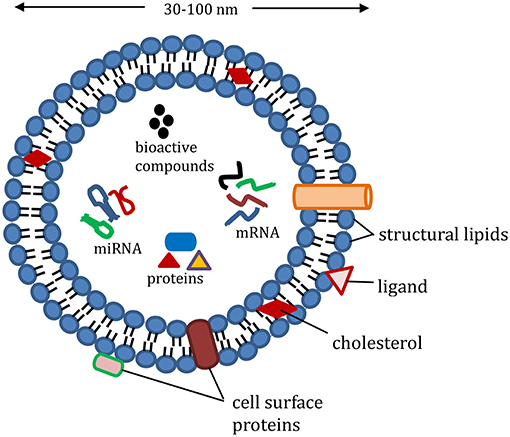
Figure 1. Representative structure of exosomes loaded with cargo (proteins, e.g., heat shock proteins, Ras-related protein; mRNA, miRNA, and non-coding RNA; nutrients, and bioactive compounds); structural lipids in exosomes include ceramide, sphingolipids, and phospholipids; exosome membrane proteins include major histocompatibility complex-II, cluster of differentiation, and integrin.
In animals, exosomes are secreted mainly by immune cells such as tumor cells, red blood cells, platelets, lymphocytes, and dendritic cells (Mandal, 2016). They are present in biological fluids such as milk, urine and blood plasma (Keller et al., 2007). More recently, there are reports of the presence of plant vesicles that resemble exosomes in structure and function, but their biogenesis is still not clear. Several methods have been established for isolating exosomes and exosome-like vesicles from animal and plant sources, with differential ultracentrifugation as the major technique. Once isolated, exosomes can further be loaded with the desired bioactive compounds.
Even though exosomes have prospects for use in drug delivery applications, there are many knowledge gaps to fully utilize their competitive properties. For instance, what happens to the exosomes in the body during and after delivery of bioactive compounds? Are human cells and organs comfortable interacting with foreign exosomes from plants the way they do with human exosomes? Moreover, the process of offloading the content of exosome to achieve high loading efficiency is not clear, and the distinctive behavior and contents of exosomes derived from plant and animal sources need to be established. There is also a need to sustainably produce and isolate high yields of exosome vesicles from plants for practical use in the delivery of bioactive compounds.
Isolation of Exosomes
Isolation by Differential Ultracentrifugation
This method has become the gold standard procedure for isolation and purification of exosomes and exosome-like particles mainly because of its simplicity and cost-effectiveness (Li et al., 2017). The method relies on differences in density and size of the separated particles. The exosome source, biological fluid or plant juice, is subjected to series of centrifugation processes with increasing speeds and duration to eliminate the larger and higher-density components; the pellets derived from this step are discarded. The final round of centrifugation of the supernatant is done at a higher speed of about 100,000 × g to recover the pellet containing the exosomes, which is then resuspended and washed in a buffer. This method is often modified for the different sources of exosomes. For instance, for animal fluids (e.g., plasma), because of their viscosity, dilution of the fluid and centrifugation at increased speed and duration are necessary for obtaining high exosome yields (Théry et al., 2006). Differential ultracentrifugation is the basic procedure for exosome isolation and the resulting exosomes often contain contaminants, mainly nucleic acids and protein aggregates (Chiou and Ansel, 2016). For further purification, an extra step could be added involving 30% sucrose gradient. Exosomes float on a sucrose gradient (Figure 2); hence, this step is invaluable for obtaining purer exosomes. This process ensures that majority of the contaminants isolated with the exosomes do not float with the exosomes and, thus, would be separated. Munagala et al. (2016) demonstrated that discarding the pellets derived from centrifugation at 100,000 × g and adding an extra centrifugation at 135,000 × g successfully eliminated other contaminating extracellular vesicles (Arntz et al., 2015). Although this process increases purity, it however reduces the yield of the isolated exosomes.
Isolation by Ultrafiltration
Due to the low purity of exosomes isolated by differential ultracentrifugation, efforts have been made to devise methods for large-scale isolation of exosomes with both higher purity and yield. Ultrafiltration is a technique that separates biomolecules based on size difference. Lobb et al. (2015) suggested this method as a faster alternative to ultracentrifugation when they observed that it led to a higher recovery of exosome particles. Ultrafiltration involves the use of membrane filters and pressure to eliminate large molecular size contaminants with subsequent isolation of exosomes. Despite the promise, the applied pressure in this process may lead to contamination, as larger vesicles may disintegrate into vesicles that resemble exosomes and be co-isolated with the exosomes (Li et al., 2017). An emerging modification to this approach involves separation of the ultrafiltration product by liquid chromatography for further purification (Andaloussi et al., 2013). This extra step is expected to increase the production cost, which is an important consideration when using exosomes in food applications.
Isolation by Immunoisolation
In this method, magnetic beads are coated with antibodies that target the proteins present on the exosome surface (Théry et al., 2006). This method relies on surface biomarkers that are uniquely expressed on exosomes (Li et al., 2017). Although it ensures isolation with high purity, the major drawback of this approach is the limited information available on exosomal structures and surface compositions, especially for new plant sources. Besides, the process would not be efficient for large-scale isolation of exosomes due to the dissociation and purification steps required after their binding to the antibodies.
Stability of Exosomes
Exosomes have proven to increase the stability of its content and, hence, can play a role in enhancing bioavailability of bioactive compounds. Some studies have shown that exosomes can resist the enzymes in digestive and other biological fluids, so their contents are protected from degradation until they reach their target. For instance, curcumin encapsulated in exosomes derived from EL-4 cells was found to be about four times more stable, with higher water solubility, when compared to free curcumin (Mandal, 2016). Among the extracellular vesicles, namely ectosomes, exosomes and apoptotic bodies, exosomes have proven to be most stable. Osteikoetxea et al. (2015) found that exosomes possess a liquid-ordered phase membrane and demonstrate the least sensitivity to detergent lysis of the three vesicle types.
Stability of Animal-Derived Exosomes
Exosomes are in their optimal state when freshly isolated but can be stored for up to 1 year at −80°C with no coagulation (Théry et al., 2006; Munagala et al., 2016). However, repeated freeze-thaw cycles affect the structural integrity and shelf life of exosomes. Biological fluids (e.g., plasma) containing exosomes can be stored for up to 5 days at 4°C in a glass bottle (Théry et al., 2006). Stability for up to 3 months at −20°C has also been reported (Kalra et al., 2013). A study by Kumeda et al. (2017) revealed that the integrity of exosomes isolated from human saliva was retained for up to 20 months when stored at 4°C, and 28 days when stored at 4°C in whole saliva containing various enzymes. Although animal cell-derived exosomes are stable at low temperatures, their structural and physicochemical properties could be affected by pressure, repeated freeze-thaw cycles, nature of solvent, and storage duration. Understanding the relationship between these factors and the integrity of exosomes is essential for efficient application of the vesicles in enhancing the bioavailability of bioactive compounds.
Stability of Plant-Derived Exosome-Like Vesicles
Plant-derived edible vesicles respond differently in biological (e.g., stomach-like and intestinal) conditions. Changes in the size and surface charge of exosomes depend on the plant source and environment (Mu et al., 2014). For instance, exosome properties may be pH-dependent. Grape-derived exosome-like vesicles were reduced in size in stomach and intestinal conditions, compared to vesicles suspended in water, while a portion of the vesicles derived from ginger were enlarged in stomach-like and intestine-like environments (Mu et al., 2014). Since dynamic light scattering was used in the analysis, it is difficult to conclude if the size differences were due to aggregation of the vesicles, increase in the size of individual particles, or fluid absorption under these biological conditions. This finding is contrary to the work of Mandal (2016), where exosomes isolated from EL-4 (murine lymphoblast) cells were stable when treated with digestive enzymes. In our opinion, the surface and compositional differences underscores the need to further study the stability of exosomes from different sources in food and biological matrices.
Loading Efficiency of Exosomes
Exosomes already contain some compounds encapsulated naturally in the vesicles. To maximize the loading efficiency, there may be a need to offload the content of the exosomes and exosome-like particles. To date, no information is available in the literature on the offloading of exosomes. This step could enhance the use of exosomes in delivery if the membrane integrity is not lost during the offloading process. Plant exosome-like particles possess more bioactive compounds than those derived from animals, but in scenarios where the bioactive compound is not the one of interest, an offloading step may be added. Regarding the loading process, two major methods have been applied: passive and active cargo loading.
Passive Cargo Loading
Passive cargo loading involves an incubation process that recruit bioactive compounds into the exosomes. It consists of two major methods. The first method involves incubation of compounds with the exosomes (Luan et al., 2017). This method of encapsulation and its loading efficiency rely on the process of diffusion and hydrophobic interaction between the lipid layer of the exosomes and the molecule to be loaded (Luan et al., 2017). Vashisht et al. (2017) reported the loading efficiency of curcumin in exosomes using this loading method to be 70.46%. Curcumin was also loaded into exosomes derived from EL-4 cells with a loading capacity of 2.9 g curcumin per 1 g exosomes. However, a major drawback of this method is that it depends on diffusion through a concentration gradient for loading. The second method is less common and involves incubation with the donor cells. The purpose of this approach is for the host cells to accumulate the bioactive compound and secrete exosomes that possess these compounds (Luan et al., 2017). Due to the untargeted nature, this method may lead to low yield of exosomes containing the compounds of interest.
Active Cargo Loading
Active cargo loading involves methods that disrupt the membrane of the exosomes temporarily so that the desired compounds could easily diffuse into the vesicles. Membrane integrity is then restored after the bioactive compounds are loaded into the exosomes. The membrane could be disrupted by sonication, extrusion, and freeze-thaw cycles. This method of cargo loading was reported to increase the loading capacity of extracellular vesicles by up to 11 times when compared to passive cargo loading (Fuhrmann et al., 2015). The problem associated with this method is the potential of damaging the native structures and targeting features of exosomes during the membrane disruption process. We suggest that a combination of passive and active cargo loading may solve the problems associated with each method.
Delivery Application of Exosomes
Exosomes gained a lot of interest over the past decade because of their potential as a nanodelivery system (Table 1), and advantages over other delivery systems. For instance, exosome vesicles form innately and naturally participate as nanocarriers. Thus, unlike some synthetic liposomes used in delivery, exosomes would be expected to be more biocompatible and safer. Exosomes are found in various biological fluids such as milk (Figure 2) and are generally considered stable in digestive and other biological fluids. Thus, exosomes can be efficient in long distance biological communication (Luan et al., 2017), as they would not be destroyed by the digestive condition and would likely remain unchanged until they reach their destination. Exosomes also have a targetability advantage as they bind specific targets due to their unique surface compositions. On reaching their destination, exosomes can offload their contents into the target cells through either phagocytosis or membrane fusion (Figure 3) (Aryani and Denecke, 2016). Although exosomes generally have a natural targeting capability, which differs depending on their source, their preferred target can be customized by inserting molecules in donor cells (sources) that would be recognized by the recipient cells in the body (Luan et al., 2017). This process includes inserting genes that code for proteins that target specific cells, so that exosomes secreted from those cells possess the desired targeting capability.
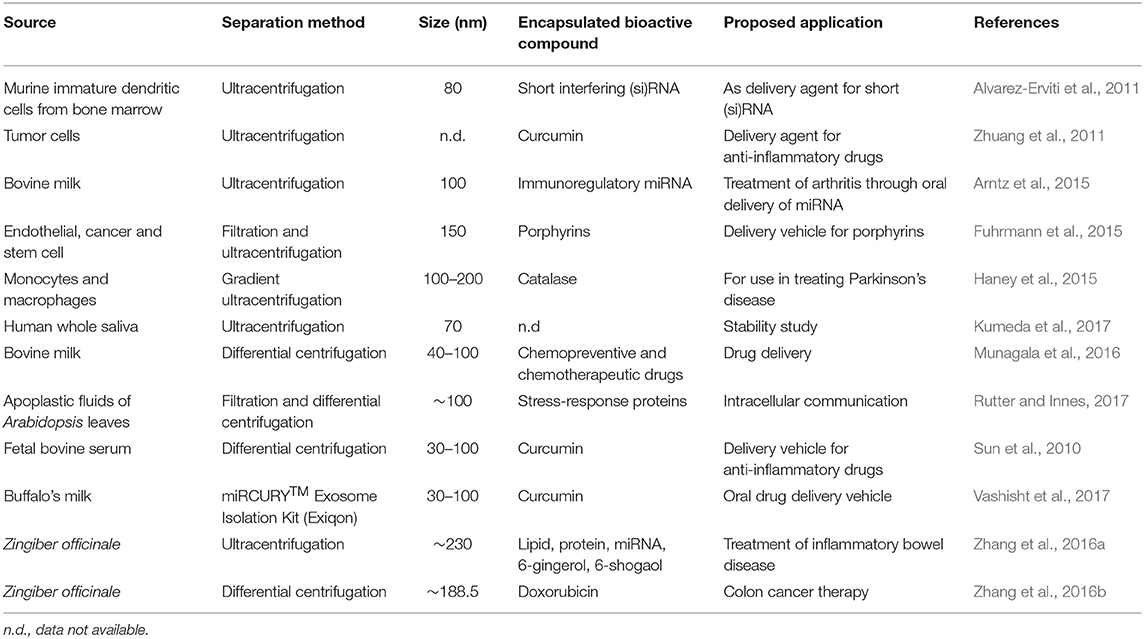
Table 1. Sources, extraction, and proposed application of exosome vesicles in the delivery of functional and bioactive molecules.
Mammalian Exosomes as a Delivery Vehicle for Nutraceutical Compounds
In designing a drug delivery system for Parkinson's disease, Haney et al. (2015) reported the efficient encapsulation and stabilization of catalase with exosomes isolated from murine monocytes and macrophages. A size range of 100–200 nm was obtained for the catalase-loaded exosomes, which had a high loading efficiency, sustained release, its content preserved from degradation by proteases, and significant in vitro and in vivo neuroprotective properties. Moreover, fetal calf serum exosome has proven to be a good vehicle for delivering short interfering (si)RNA into the mouse brain (Alvarez-Erviti et al., 2011). Exosomes isolated from murine lymphoma and macrophage cell lines have also been employed in curcumin encapsulation and delivery (Sun et al., 2010; Zhuang et al., 2011). In one study, exosome encapsulation enhanced the bioavailability and anti-inflammatory activity of curcumin and ensured target specificity toward inflammatory cells (Sun et al., 2010). In the other, curcumin or a signal transducer and activator of transcription 3 (stat3) inhibitor encapsulated in exosomes and delivered intranasally protected mice from LPS-induced inflammation, myelin oligodendrocyte glycoprotein peptide-induced autoimmune encephalomyelitis progression, and inhibited murine brain tumor (GL26) cell growth (Zhuang et al., 2011). The attention given to animal-derived exosomes as delivery vehicles for bioactive compounds is because of their biocompatibility, biorecognition, target specificity, and stability (Keller et al., 2006).
Delivery of Bioactive Plants Components
Exosome-like particles derived from plants have recently gained a lot of interest. The vesicles, located in the paramural space of plants, are similar in structure and function to their mammalian counterparts (An et al., 2007) and are known as plant-derived edible nanoparticles (PDENs). There is still an uncertainty regarding the biogenesis of the exosome-like particles. The vesicles participate not only in plant cell communication but also in interspecies communication. Zhang et al. (2016a) reported a high yield of exosome-like vesicles of about 50 mg per 1 kg of ginger. The vesicles also contain proteins, lipids and nuclear components of their sources. Their compositions vary since different plants would possess different type or proportions of the contents. The exosome vesicles also have factors that determine their delivery efficiency, such as long-distance communication factors. Majority of the plant sources have inherent and unique compounds with physiologically relevant bioactivities. It is likely that the bioactive compounds are also delivered to their targets via exosome-like vesicles. Zhang et al. (2016a) reported that ginger-derived exosomes possess high levels of phosphatidic acid, which promotes intervesicular fusion. Furthermore, Rutter and Innes (2017) reported the presence of exosomes in the apoplasts of leaves, and its role in plant immunity was confirmed based on their protein profile and high expression during stress. For target delivery, there would be a need to offload the inherent bioactive compounds in exosomes in order to achieve a high loading efficiency with the bioactive compounds of interest.
Plant Lipid-Derived Vesicles as Delivery Vehicles
Nanovectors can also be made from lipids extracted from plant sources and used as nanocarriers of bioactive compounds other than their inherent compounds. Using sonication, lipids extracted from plants can form nanostructures onto which small compounds can be embedded. For instance, doxorubicin was successfully inserted into nanovesicles derived from ginger lipids through electrostatic interaction and this encapsulation prompted the drug release in a pH-dependent manner (Zhang et al., 2016b). This stands as a good large-scale method for nanodelivery, and overcomes the low yield issue associated with exosomes. However, the lipid nanocarriers are not exosomes, in structure and function, but they have the benefits of a natural, food-derived delivery system. A limitation of this system is the exclusive dependence on electrostatic interaction for the loading and offloading of the vesicles.
Exosomes and Liposomes
Unlike exosomes, which are produced naturally, liposomes are made synthetically and have a similar lipid bilayer structure. Liposomes are designed to enable both encapsulation of hydrophilic molecules such as siRNA, DNA, and RNA, into the aqueous core of the vesicle, and hydrophobic bioactive compounds such as proteins, peptides, phenolics and antibodies, into the lipid bilayer (Çağdaş et al., 2014). Liposomes are prepared by membrane extrusion, sonication, microemulsification, and freeze-thawing, and have been utilized in many drug delivery applications, e.g., in the formulation of amphotericin B, doxorubicin, verteporfin, cytarabine, morphine sulfate, and daunorubicin (Fan and Zhang, 2013). Preparation of liposomes, however, can be problematic as it requires numerous chemical treatments and steps to modify the lipid bilayer structure using protein, ligands, and antibodies (Ishida et al., 1999; Theek et al., 2016). The difficulty in functionalizing the lipid bilayer and rigorous synthetic protocol can be circumvented by the use of exosome for delivery purposes. Exosomes and liposomes have similar physicochemical properties but unlike liposomes, exosomes cannot be currently used to effectively deliver exogenous hydrophilic macromolecules (Stremersch et al., 2016). The inherent biochemical similarities between the exosome surface and that of the originating cells makes it more feasible, when compared to liposomes, to target cells from which the exosomes are derived (Keller et al., 2006).
Challenges With the Use of Exosomes for Delivery Purposes
The major drawback with the use of exosomes as a delivery agent is centered on inefficient extraction and isolation process, low extraction yield, low encapsulation and loading efficiencies, and issues with delivery of exogenous hydrophilic macromolecules, and potential delivery of unwanted cargo materials naturally present in the exosomes. Most exosome extraction methods are complex, labor intensive, and give low yield. However, recent extraction and detection techniques have shown promise, such as magnetic adhesion (Qi et al., 2016) and flow cytometry (Morales-Kastresana et al., 2017) in addition to improved loading technique such as sonication (Kim et al., 2016), electroporation (Kamerkar et al., 2017), and incubation (Qi et al., 2016). However, offloading the content of exosome without distorting the structural integrity is very important in ensuring high loading and encapsulation efficiencies. Lastly, there is a need for exosome functionalization to accommodate the encapsulation of exogenous hydrophilic macromolecules either by pre-formation (i.e., during exosome biogenesis) or post-formation loading (i.e., after exosome isolation) (Stremersch et al., 2016).
Conclusion and Future Directions
Since exosomes have piqued curiosity, there has been an increasing focus on their potential application in the delivery of bioactive food compounds. As some nutrients and phytochemicals can pass through the digestive tract without being absorbed efficiently, exosomes can be explored as a stable and natural nanodelivery system for increasing bioavailability of these compounds. Moreover, it is likely that these vesicles already function as nanocarriers within the plant and animal tissues. For instance, when fruits are consumed, there is the possibility that exosome-like particles are used as nanovectors in the body, thereby functioning in delivering of their inherent nutrients and bioactive compounds. Some studies have focused on the loading of exosomes with bioactive compounds to achieve a high loading efficiency. We recommend the addition of an extra step to the standard procedure where the vesicles are stripped of their initial contents prior to the loading step. The offloading process should be such that the content is removed without significantly altering the native structure (e.g., size, surface composition) and biological function (e.g., long-distance communication) of exosomes. More research is needed on the biogenesis of plant-derived exosome-like vesicles and how they respond to various environmental conditions, to enhance their production and extraction yields for large-scale food applications. Furthermore, the role of exosomes as delivery vesicles within the plant and animal systems needs clarification. Lastly, as the topic develops within the food science discipline, it is crucial to verify the stability and safety of the natural vesicles when used as delivery vehicles in different food product matrices.
Author Contributions
CU conceived the project. PA and OO conducted the literature search and wrote the manuscript draft. CU revised the manuscript. PA, OO, and CU reviewed the final version of the manuscript.
Conflict of Interest Statement
The authors declare that the research was conducted in the absence of any commercial or financial relationships that could be construed as a potential conflict of interest.
References
Alvarez-Erviti, L., Seow, Y., Yin, H. F., Betts, C., Lakhal, S., and Wood, M. J. A. (2011). Delivery of siRNA to the mouse brain by systemic injection of targeted exosomes. Nat. Biotechnol. 29:341. doi: 10.1038/nbt.1807
An, Q., Van Bel, A. J. E., and Hückelhoven, R. (2007). Do plant cells secrete exosomes derived from multivesicular bodies? Plant Signal. Behav. 2:1. doi: 10.4161/psb.2.1.3596
Andaloussi, S. E. L., Mäger, I., Breakefield, X. O., and Wood, M. J. A. (2013). Extracellular vesicles: biology and emerging therapeutic opportunities. Nat. Rev. Drug Delivery 12:5. doi: 10.1038/nrd3978
Arntz, O. J., Pieters, B. C. H., Oliveira, M. C., Broeren, M. G. A., Bennink, M. B., de Vries, M., et al. (2015). Oral administration of bovine milk derived extracellular vesicles attenuates arthritis in two mouse models. Mol. Nutr. Food Res. 59:9. doi: 10.1002/mnfr.201500222
Aryani, A., and Denecke, B. (2016). Exosomes as a nanodelivery system: a key to the future of neuromedicine? Mol. Neurobiol. 53:2. doi: 10.1007/s12035-014-9054-5
Beach, A., Zhang, H., Ratajczak, M. Z., and Kakar, S. S. (2014). Exosomes: an overview of biogenesis, composition and role in ovarian cancer. J. Ovar. Res. 7:14. doi: 10.1186/1757-2215-7-14
Çağdaş, M., Sezer, A. D., and Bucak, S. (2014). “Liposomes as Potential Drug Carrier Systems for Drug Delivery,” in Application of Nanotechnology in Drug Delivery, ed A. D. Sezer (London: IntechOpen Limited). doi: 10.5772/58459
Chiou, N., and Ansel, M. (2016). Improved Exosome Isolation by Sucrose Gradient Fractionation of Ultracentrifuged Crude Exosome Pellets. Protocol Exchange. doi: 10.1038/protex.2016.057
Fan, Y., and Zhang, Q. (2013). Development of liposomal formulations: from concept to clinical investigations. Asian J. Pharmaceut. Sci. 8, 81–87. doi: 10.1016/j.ajps.2013.07.010
Fuhrmann, G., Serio, A., Mazo, M., Nair, R., and Stevens, M. M. (2015). Active loading into extracellular vesicles significantly improves the cellular uptake and photodynamic effect of porphyrins. J. Control. Release 205, 35–44. doi: 10.1016/j.jconrel.2014.11.029
Haney, M. J., Klyachko, N. L., Zhao, Y., Gupta, R., Plotnikova, E. G., He, Z., et al. (2015). Exosomes as drug delivery vehicles for Parkinson's disease therapy. J. Control. Release 207, 18–30. doi: 10.1016/j.jconrel.2015.03.033
Ishida, T., Iden, D. L., and Allen, T. M. (1999). A combinatorial approach to producing sterically stabilized (Stealth) immunoliposomal drugs. FEBS Lett. 460, 129–133. doi: 10.1016/S0014-5793(99)01320-4
Kalra, H., Adda, C. G., Liem, M., Ang, C.-S., Mechler, A., Simpson, R. J., et al. (2013). Comparative proteomics evaluation of plasma exosome isolation techniques and assessment of the stability of exosomes in normal human blood plasma. Proteomics 13:22. doi: 10.1002/pmic.201300282
Kamerkar, S., LeBleu, V. S., Sugimoto, H., Yang, S., Ruivo, C. F., Melo, S. A., et al. (2017). Exosomes facilitate therapeutic targeting of oncogenic KRAS in pancreatic cancer. Nature 546, 498. doi: 10.1038/nature22341
Keller, S., Rupp, C., Stoeck, A., Runz, S., Fogel, M., Lugert, S., et al. (2007). CD24 is a marker of exosomes secreted into urine and amniotic fluid. Kidney Int. 72:9. doi: 10.1038/sj.ki.5002486
Keller, S., Sanderson, M. P., Stoeck, A., and Altevogt, P. (2006). Exosomes: from biogenesis and secretion to biological function. Immunol. Lett. 107, 102–108. doi: 10.1016/j.imlet.2006.09.005
Kim, M. S., Haney, M. J., Zhao, Y., Mahajan, V., Deygen, I., Klyachko, N. L., et al. (2016). Development of exosome-encapsulated paclitaxel to overcome MDR in cancer cells. Nanomedicine 12, 655–664. doi: 10.1016/j.nano.2015.10.012
Kumeda, N., Ogawa, Y., Akimoto, Y., Kawakami, H., Tsujimoto, M., and Yanoshita, R. (2017). Characterization of membrane integrity and morphological stability of human salivary exosomes. Biol. Pharmaceut. Bull. 40:8. doi: 10.1248/bpb.b16-00891
Li, P., Kaslan, M., Lee, S. H., Yao, J., and Gao, Z. (2017). Progress in exosome isolation techniques. Theranostics 7:3. doi: 10.7150/thno.18133
Lobb, R. J., Becker, M., Wen, S. W., Wong, C. S. F., Weigmans, A. P., Leimgruber, A., et al. (2015). Optimized exosome isolation protocol for cell culture supernatant and human plasma. J. Extracel. Vesicles 4:1. doi: 10.3402/jev.v4.27031
Luan, X., Sansanaphongpricha, K., Myers, I., Chen, H., Yuan, H., and Sun, D. (2017). Engineering exosomes as refined biological nanoplatforms for drug delivery. Acta Pharmacol. Sinica 38, 754–763. doi: 10.1038/aps.2017.12
Mandal, S. (2016). Curcumin, a promising anticancer therapeutic: its bioactivity and development of the drug delivery vehicles. Int. J. Drug Res. Technol. 6:2. Available online at: http://ijdrt.com/drug-research-and-technology/article/view/curcumin-a-promising-anticancer-therapeutic-its-bioactivity-and-development-of-drug-delivery-vehicles
Meldolesi, J. (2016). Ectosomes and exosomes - two extracellular vesicles that differ only in some details. Biochem. Mol. Biol. J. 2:1. doi: 10.21767/2471-8084.100012
Morales-Kastresana, A., Telford, B., Musich, T. A., McKinnon, K., Clayborne, C., Braig, Z., et al. (2017). Labeling extracellular vesicles for nanoscale flow cytometry. Sci. Rep. 7:1878. doi: 10.1038/s41598-017-01731-2
Mu, J., Zhuang, X., Wang, Q., Jiang, H., Deng, Z., Wang, B., et al. (2014). Interspecies communication between plant and mouse gut host cells through edible plant derived exosome-like nanoparticles. Mol. Nutr. Food Res. 58:7. doi: 10.1002/mnfr.201300729
Munagala, R., Aqil, F., Jeyaprakash, J., and Gupta, R. C. (2016). Bovine milk-derived exosomes for drug delivery. Cancer Lett. 371:1. doi: 10.1016/j.canlet.2015.10.020
Osteikoetxea, X., Sódar, B., Németh, A., Szabó-Taylor, K., Pálóczi, K., Vukman, K. V., et al. (2015). Differential detergent sensitivity of extracellular vesicle subpopulations. Org. Biomol. Chem. 13:38. doi: 10.1039/C5OB01451D
Qi, H., Liu, C., Long, L., Ren, Y., Zhang, S., Chang, X., et al. (2016). Blood exosomes endowed with magnetic and targeting properties for cancer therapy. ACS Nano 10, 3323–33. doi: 10.1021/acsnano.5b06939
Raposo, G., and Stoorvogel, W. (2013). Extracellular vesicles: exosomes, microvesicles, and friends. J. Cell Biol. 4:373. doi: 10.1083/jcb.201211138
Rutter, B. D., and Innes, R. (2017). Extracellular vesicles isolated from the leaf apoplast carry stress-response proteins. Plant Physiol. 173:1. doi: 10.1104/pp.16.01253
Stremersch, S., Vandenbroucke, R. E., Van Wonterghem, E., Hendrix, A., De Smedt, S. C., and Raemdonck, K. (2016). Comparing exosome-like vesicles with liposomes for the functional cellular delivery of small RNAs. J. Control. Release 232, 51–61. doi: 10.1016/j.jconrel.2016.04.005
Sun, D., Zhuang, X., Xiang, X., Liu, Y., Zhang, S., Liu, C., et al. (2010). A novel nanoparticle drug delivery system: the anti-inflammatory activity of curcumin is enhanced when encapsulated in exosomes. Mol. Ther. 18:1606. doi: 10.1038/mt.2010.105
Theek, B., Baues, M., Ojha, T., Möckel, D., Veettil, S. K., Steitz, J., et al. (2016). Sonoporation enhances liposome accumulation and penetration in tumors with low EPR. J. Control. Release 231, 77–85. doi: 10.1016/j.jconrel.2016.02.021
Théry, C., Amigorena, S., Raposo, G., and Clayton, A. (2006). Isolation and characterization of exosomes from cell culture supernatants and biological fluids. Curr. Protoc. Cell Biol. 30:1. doi: 10.1002/0471143030.cb0322s30
Vashisht, M., Rani, P., Onteru, S. K., and Singh, D. (2017). Curcumin encapsulated in milk exosomes resists human digestion and possesses enhanced intestinal permeability in vitro. Appl. Biochem. Biotechnol. 183:3. doi: 10.1007/s12010-017-2478-4
Zhang, M., Viennois, E., Prasad, M., Zhang, Y., Wang, L., Zhang, Z., et al. (2016a). Edible ginger-derived nanoparticles: a novel therapeutic approach for the prevention and treatment of inflammatory bowel disease and colitis-associated cancer. Biomaterials 101, 321–340. doi: 10.1016/j.biomaterials.2016.06.018
Zhang, M., Xiao, B., Wang, H., Han, M. K., Zhang, Z., Viennois, E., et al. (2016b). Edible ginger-derived nano-lipids loaded with doxorubicin as a novel drug-delivery approach for colon cancer therapy. Mol. Ther. 24:10. doi: 10.1038/mt.2016.159
Keywords: exosomes, extracellular vesicles, differential ultracentrifugation, immunoisolation, exosome-like vesicles, loading efficiency, biostability, nutraceutical compounds
Citation: Akuma P, Okagu OD and Udenigwe CC (2019) Naturally Occurring Exosome Vesicles as Potential Delivery Vehicle for Bioactive Compounds. Front. Sustain. Food Syst. 3:23. doi: 10.3389/fsufs.2019.00023
Received: 06 September 2018; Accepted: 26 March 2019;
Published: 16 April 2019.
Edited by:
Ashok R. Patel, Guangdong Technion-Israel Institute of Technology (GTIIT), ChinaReviewed by:
Miguel Cerqueira, Laboratório Ibérico Internacional de Nanotecnologia (INL), PortugalConstantinos V. Chrysikopoulos, Technical University of Crete, Greece
Copyright © 2019 Akuma, Okagu and Udenigwe. This is an open-access article distributed under the terms of the Creative Commons Attribution License (CC BY). The use, distribution or reproduction in other forums is permitted, provided the original author(s) and the copyright owner(s) are credited and that the original publication in this journal is cited, in accordance with accepted academic practice. No use, distribution or reproduction is permitted which does not comply with these terms.
*Correspondence: Chibuike C. Udenigwe, Y3VkZW5pZ3dAdW90dGF3YS5jYQ==
†These authors have contributed equally to this work