- 1Guelph Research and Development Center, Agriculture and Agri-Food Canada, Guelph, ON, Canada
- 2Lethbridge Research and Development Centre, Agriculture and Agri-Food Canada, Lethbridge, AB, Canada
- 3Canadian Food Inspection Agency (CFIA) National Centres for Animal Disease, Lethbridge Laboratory, Lethbridge, AB, Canada
- 4British Columbia Ministry of Agriculture, Abbotsford, BC, Canada
- 5London Research and Development Centre, Agriculture and Agri-Food Canada, London, ON, Canada
The objective of this study was to investigate the distribution and persistence of antimicrobial resistance genotypes of enterococci from broilers fed bambermycin (BAM), penicillin (PEN), salinomycin (SAL), bacitracin (BAC), or a salinomycin/bacitracin combination (SALBAC) for 35 days. A total of 95 enterococci from cloacal (n = 40), cecal (n = 38), and litter samples collected on day 36 (n = 17) were isolated weekly from day 7 to 36. All isolates were identified by API-20 Strep and their antimicrobial susceptibilities were evaluated using the Sensititre system with the plates for Gram positive bacteria. Whole genome sequencing (WGS) was used to assess their intra- and inter-genetic variability, with a focus on virulence and antibiotic resistance characteristics. All isolates were further characterized for hemolysin production (HEM), bile salt hydrolysis (BSH), and gelatinase (GEL) activities. Of the 95 isolates, Enterococcus faecium (n = 58) and Enterococcus faecalis (n = 24) were the most common Enterococcus species identified. Significant differences in the level of resistance for the E. faecium isolates to ciprofloxacin, macrolide, penicillin and tetracycline were observed among treatments. The bcrR, mefA, and aac(6) genes were higher in BAM treatment than the other groups whereas bcrR, ermA, ermB, aphA(3), and tetL were more prevalent in PEN and BAC treatments. Overall, E. faecium isolates showed a higher prevalence of antimicrobial resistance, but E. faecalis from litter also exhibited a significant level of resistance. A range of 4–15 different virulence genes was detected in E. faecalis. All isolates from litter but one (94.1%) showed BSH activities while 52.9% of them produced GEL. HEM activity was observed only in isolates collected on Day 7 (n = 9) and Day 14 (n = 1). This study confirmed that genetically diverse AMR enterococci harboring virulence factors can be promoted by the use of certain antimicrobials in feed. Such enterococci could persist in broiler chickens and their litter, which can potentially contaminate the soil upon land application.
Introduction
Enterococci are Gram-positive facultative anaerobic bacteria that are part of the normal intestinal microbiota, with densities ranging from 105 to 108 CFU/g of intestinal content (Yost et al., 2011; Dubin and Pamer, 2017). Members of the genus Enterococcus, which includes presently about 40 recognized species, were initially classified as group D streptococci sharing several phenotypic and biochemical similarities, making their identification difficult (Yost et al., 2011). Enterococci have been proposed as fecal indicator bacteria for microbial source tracking (Byappanahalli et al., 2012; Boehm and Sassoubre, 2014) and are often used in tracking trends in resistance to antimicrobials for various resistance surveillance systems (Tyson et al., 2018a).
Enterococcus species have emerged as the cause of ~12% of nosocomial infections, with only two species, Enterococcus faecalis and Enterococcus faecium, causing about 90% of clinical infections (Billington et al., 2014; Torres et al., 2018). Moreover, these two species are considered the third and fourth most prevalent human pathogens worldwide (ECDC, 2011) and ranked third in causing bacteremia in Europe and North America, responsible for ~11–13% of all bacteremia cases (Ammerlaan et al., 2013; De Kraker et al., 2013). Hospital associated outbreaks linked to vancomycin-positive E. faecium, which belongs to MLST clonal complex 17 (CC17), have been reported in several countries (Werner et al., 2008; Pinholt et al., 2015). Genetic relatedness was found between E. faecalis isolates from urinary tract infection cases and those from poultry, reinforcing the zoonotic potential of this species and suggesting a possible role of poultry in its spread to humans (Poulsen et al., 2012; Bortolaia and Guardabassi, 2015; Abat et al., 2016).
In poultry, enterococci have been associated with septicemia, endocarditis, and other diseases (Gilmore, 2002). The safety issue regarding enterococci has not been recognized in poultry meat, however concerns about transmission of antimicrobial resistant enterococci to humans have been reported (Simonsen et al., 1998; Marshall and Levy, 2011). Recently, the isolation of AMR E. faecalis strains from broilers with vertebral osteomyelitis disease has been reported (Braga et al., 2018). Antibiotic resistant enterococci have been reported in poultry retail meats (Aslam et al., 2012). The ability of enterococci to acquire AMR through the transfer of plasmids and transposons, chromosomal exchange, or mutation presents a significant challenge to infection control (Hollenbeck and Rice, 2012). Mobile genetic elements, including transposons, play an important role in the dissemination of AMR though horizontal gene transfer in bacteria including enterococci. Transposons such as Tn916/Tn1545, Tn917/Tn551, and Tn5397 have been reported to disseminate resistant genes, including tetM, ermB, and aphA-III, by enterococci (Hegstad et al., 2010). Furthermore, in the absence of antimicrobials, pheromone production was reported to induce a high-frequency plasmid transfer in E. faecalis (Hirt et al., 2018).
Due to substantial scientific evidence on the selection, distribution, and dissemination of AMR genes in broiler chicken production systems, in relation to the use of specific therapeutic agents or antimicrobial growth promoters (AGPs) (Aarestrup, 2000; Diarra et al., 2007; Nhung et al., 2016), and due to public and possible food safety and environmental health concerns, the monitoring of AMR in chicken production is imperative.
In our previous study, we described the effects of the in-feed inclusion of bambermycin (BAM), penicillin (PEN), salinomycin (SAL), bacitracin (BAC), or a salinomycin/bacitracin combination (SALBAC) on bacterial population, including enterococci, as well as the incidence and distribution of antibiotic-resistant Escherichia coli in broiler chickens (Diarra et al., 2007). The present study describes details of resistance and virulence genotypes of enterococci isolates in relation to their phenotypes using the whole genome sequencing (WGS) approach. WGS is being rapidly adapted in many laboratories for strain typing, outbreak investigations, and comparative genome analysis (Salipante et al., 2015; Sekse et al., 2017; He et al., 2018; Pightling et al., 2018). Several studies have highlighted the need of WGS for antimicrobial resistance (AMR) characterization (Pightling et al., 2018; Tyson et al., 2018b) and accurate prediction of AMR phenotypes from the genotype data. This has been realized to be a critical step in the event that WGS becomes the benchmark to predict the MICs in order to guide clinical decision making (Macesic et al., 2017). Currently, the majority of WGS-based studies focused on Gram-negative bacteria to study AMR phenotype-to-genotype correlations, for example Salmonella, Campylobacter, E. coli, and Pseudomonas (Tyson et al., 2015; Davis et al., 2016; McDermott et al., 2016; Zhao et al., 2016; Jeukens et al., 2017; Macesic et al., 2017; Rehman et al., 2017; Neuert et al., 2018). In contrast, relatively limited data is available on Gram-positive bacteria such as enterococci (Macesic et al., 2017; Mason et al., 2018).
The objective of the present study was to examine the complete genome sequences of enterococci isolated from broiler chickens that were fed various antimicrobial agents in order to provide a detailed genome content and to perform comparative genomic analysis of major species (E. faecalis and E. faecium). These genome sequences were used to determine phylogenetic relationships among isolates, including contemporary human isolates from various sources and days, as well as to show how genomic variations between isolates may influence phenotypic traits such as antibiotic resistance and virulence phenotypes. The sequence data were further used to determine the link between specific antimicrobials administered in the feed and the presence of specific genotypes presenting health risks to both animals and humans.
Materials and Methods
Enterococcal Strain Selection
The bacterial isolates used in this study were from a previous study conducted on broiler chicken fed with or without [per kg of feed] the following: basal level (non-antimicrobial diet) as control, 2 mg bambermycin, 2.2 mg procaine penicillin, 60 mg salinomycin, 55 mg bacitracin, and a combination of 55 mg bacitracin plus 60 mg salinomycin with each treatment being applied to three pens. Ceca, cloacae (day 7–35) and litter (day 36) samples were collected to isolate enterococci as described previously (Diarra et al., 2007). Bacteriological analyses were performed with a total of 90 fecal, 90 cecal, and 48 litter samples. Presumptive colonies were identified previously by API and a DNA microarray carrying 70 taxonomic, 17 virulence, and 174 antibiotic resistance gene probes (Champagne et al., 2011). A total of 184 enterococci isolates were identified, however non-redundant isolates were used in this study. All experimental procedures performed in this study were approved by the Animal Care Committee of the Agassiz Research and Development Center and followed principles described by the Canadian Council on Animal Care.
Antimicrobial Susceptibility Testing
The Sensititre automated system (Trek Diagnostic Systems, Cleveland, OH, USA) using the Gram-positive antimicrobial panel CMV3AGPF plates, was used to determine the minimal inhibitory concentrations (MICs) of all isolates. The MIC data was interpreted according to the Clinical and Laboratory Standards Institutes (CLSI) breakpoints (CLSI, 2015) and the Canadian Integrated Program for Antimicrobial Resistance Surveillance guidelines (CIPARS, 2008). All enterococci isolates were tested against four major categories of antimicrobials based on their importance in human medicine (https://www.canada.ca/en/health-canada/services/drugs-health-products/veterinary-drugs/antimicrobial-resistance/categorization-antimicrobial-drugs-based-importance-human-medicine.html). The category I antimicrobials (representing very high importance in human medicine) were ciprofloxacin, daptomycin, linezolid, quinupristin/dalfopristin, and vancomycin; category II antimicrobials (high importance) included erythromycin, gentamicin, kanamycin, lincomycin, penicillin, streptomycin, tylosin; category III (medium importance) included chloramphenicol, nitrofurantoin, tetracycline, tigecycline, and bacitracin; while category IV (low importance) included flavomycin. Multidrug resistance was defined as resistance to at least three different classes of antimicrobials. E. faecalis ATCC 51299 and E. faecalis ATCC 29212 were used as quality control strains in this study.
Gelatinase (GEL), Hemolytic (HEM), and Bile Salt Hydrolyze (BSH) Activities
All enterococcal isolates were screened for GEL, HEM, and BSH activities in order to assess phenotype and genotype correlations for these characters as well as their ability to survive in the gut (BSH activity), as has previously been described (Diarra et al., 2010). Briefly, the production of gelatinase was determined using Todd-Hewitt agar (Becton Dickinson) containing 30 g of gelatin per liter and incubated overnight at 37°C. The HEM production was performed by cultivating colonies onto layered fresh horse blood agar plates for 1–2 days at 37°C. Clearing zones around colonies indicated hemolysin production. The BSH activity was determined using MRS agar plates supplemented with 0.5% (wt/vol) sodium salt of taurodeoxycholic acid (Sigma-Aldrich, Oakville, Ontario, Canada) and 0.37 g/liter CaCl2. Plates were then incubated anaerobically (atmosphere of 80% N2, 10% CO2, and 10% H2) at 37°C for 48 h (Diarra et al., 2010).
Pulsed Field Gel Electrophoresis (PFGE) Analysis
Selected enterococci isolates, based on resistance phenotype, were typed using the PFGE technique according to the Centers for Disease Control and Prevention (CDC) PulseNet standardized protocol using SmaI (Roche, Laval, QC, Canada). The PFGE cluster analysis was performed in BioNumerics software version 7.5 (Applied Maths, Austin, TX) using Dice's coefficient and the Unweighted Pair Group Method (UPGMA). Isolates with similar banding patterns were considered as closely related.
Genome Sequencing, Assembly, and Comparative Genome Analysis
DNA Extraction
To perform whole genome sequencing (WGS), genomic DNA was extracted from overnight cultures in 5 mL of Brain Heart Infusion (BHI) broth (BD, New Jersey, USA) using the DNeasy Blood & Tissue Kits (QIAGEN) following the protocol as described (Beukers et al., 2017). The extracted DNA was stored in 10 mM Tris-HCl buffer (pH 8.0) and quantified by Invitrogen Qubit® 2.0 Fluorometer (Life Technologies). The quality of DNA was visualized by electrophoresis on a 1% agarose gel and stored at −20°C until genomic library construction.
Sequencing and Assembly Statistics
Sequencing libraries were prepared from 1 ng of genomic DNA with an Illumina Nextera XT DNA sample preparation kit (Illumina, Inc., CA, USA), and paired-end sequencing was performed on an Illumina MiSeq platform (Illumina Inc.) using a 600 cycle MiSeq reagent kit (v3), generating 2 × 300 bps paired-end reads. Sequences were analyzed and quality checked using FastQC (http://www.bioinformatics.babraham.ac.uk/projects/fastqc/). The reads were combined using [FLASH] Fast Length Adjustment of SHort reads v1.2.9 (Magoc and Salzberg, 2011). High-quality reads were then assembled de novo using SPAdes genome assembler version 3.9.0 software (Bankevich et al., 2012). On average the genome coverage, genome size, number of contigs and G+C contents were 88.6-fold ± 32.3-fold, 22.95 ± 0.089 Mbp, 70 ± 13 (N50 37.9 ± 5.3 kbp) and 37.0 ± 1.5%, respectively. Additionally, two representative isolates, one each of E. faecalis and E. faecium, were selected for PacBio long read sequencing, and their genomes were assembled in the Hierarchical Genome Assembly Process (HGAP) (Chin et al., 2013), producing an estimated depth of coverage 317-fold, generating two contigs and a N50 of 31.9 kbp. The assembly statistics of all sequenced genomes in this study were comparable to those previously published using similar sequencing technologies (Bertels et al., 2014; Beukers et al., 2017). The assembled genomes were annotated using Prokka version 1.11 (Seemann, 2014), which on average identified a total of ~2,900 coding sequences, five to seven rRNA, 53–56 tRNA, and 67 miscellaneous RNA.
Genomes From Public Repositories
The genomes of 81 E. faecalis and 73 E. faecium isolates arising from bloodstream and urinary infections, animals (chicken and cow), and environmental sources (aquatic, metal, wood, plastic) collected from 1960 to 2015 were obtained from NCBI database GenBank (Benson et al., 2013) (accessed on February 06, 2018) and used as references in phylogenetic and comparison studies. The list of selected reference genomes, with their accession numbers, source and host of isolation and geographical location, is presented in Tables S1, S2.
WGS-Based Species Identification
An in silico ribosomal multi-locus sequence typing (rMLST) approach that indexes the variation of the 53 genes that encode the bacterial ribosome protein subunits (rps genes) was used as a means of integrating microbial taxonomy and typing of Enterococcus-assembled genomes using the Bacterial Isolate Genome Sequence Database (BIGSdb) (Jolley et al., 2012). MLST from the assembled genomes was determined based on in silico analysis of seven housekeeping genes, including gdh, gyd, pstS, gki, aroE, xpt, yqil for E. faecalis and adk, atpa, ddl, gdh, gyd, psts, purk for E. faecium (Larsen et al., 2012).
Bioinformatics Analysis
The detection of a comprehensive set of full length ARGs in the assembled genomes was performed using a combination of ResFinder v3.0 (Zankari et al., 2012) and the comprehensive Antimicrobial Resistance Database (CARD), the Resistance Gene Identifier (RGI) version 4.0.3 (McArthur et al., 2013). Additionally, two resistance genes (streptothricin N-acetyltransferase (Sat4) and bacitracin (bcr) were manually screened among all genomes using the National Center for Biotechnology Information (NCBI), Basic Local Alignment Search Tool (BLAST), BLASTn and BLASTp (http://blast.ncbi.nlm.nih.gov/Blast.cgi). Virulence factors were identified using the VirulenceFinder v1.5 (Joensen et al., 2014). The plasmids were identified using PlasmidFinder version 1.5 (Carattoli et al., 2014).
The sequenced genomes were investigated for the presence of transposons or integrative conjugative elements (ICEs) by homology search using BLAST against 460 ICEs in the ICEberg database version 1.0 (Bi et al., 2012). In combination with their respective reference genome sequences downloaded from the NCBI, GenBank database (Benson et al., 2013), the whole genomes of E. faecium and E. faecalis sequenced in this study were comparatively analyzed with the CGView comparison tool (CCT) (Grant et al., 2012). All phylogenetic analyses were conducted using the single nucleotide variant phylogenomics (SNVphyl) (Petkau et al., 2017) and/or Reference sequence Alignment-based Phylogeny builder (RealPhy) version 1.12 pipelines (Bertels et al., 2014) with default parameters. The resulting tree was visualized in FigTree software version 1.4.3 (http://tree.bio.ed.ac.uk/software/figtree).
Statistical Analysis
Data were analyzed using a completely randomized design with the GLM procedure of SAS (SAS Institute, 2016). This generated the total number of antibiotics against which isolates were resistant and the number of resistance genes found in isolates. The association test of Cochran-Mantel-Haenszel and Fisher's exact test were used to determine the relationship between the presence of resistance phenotypes and genotypes using the FREQ procedure. Logistic analysis (proportional odds model) was used to determine the relationship between resistance phenotypes and genotypes (Diarra et al., 2007). The P-value of 0.05 was used to declare significance.
Genome Sequence Accession Numbers
The draft whole genome sequences of the 95 enterococci have been deposited in GenBank under Bio Project no. PRJNA273513 with the submission ID SUB4666681.
Results
Antimicrobial Susceptibility of Enterococcus spp.
A total of 95 identified enterococci isolates were screened for antimicrobial susceptibility testing (AST) and WGS (Table 1). These isolates derived from diverse sources, including ceca, cloaca and litter samples of broiler chickens fed with and without antibiotics. The percentages of resistant isolates to each class of antimicrobial, and their associated MIC values for each species, are presented in Table 2a.
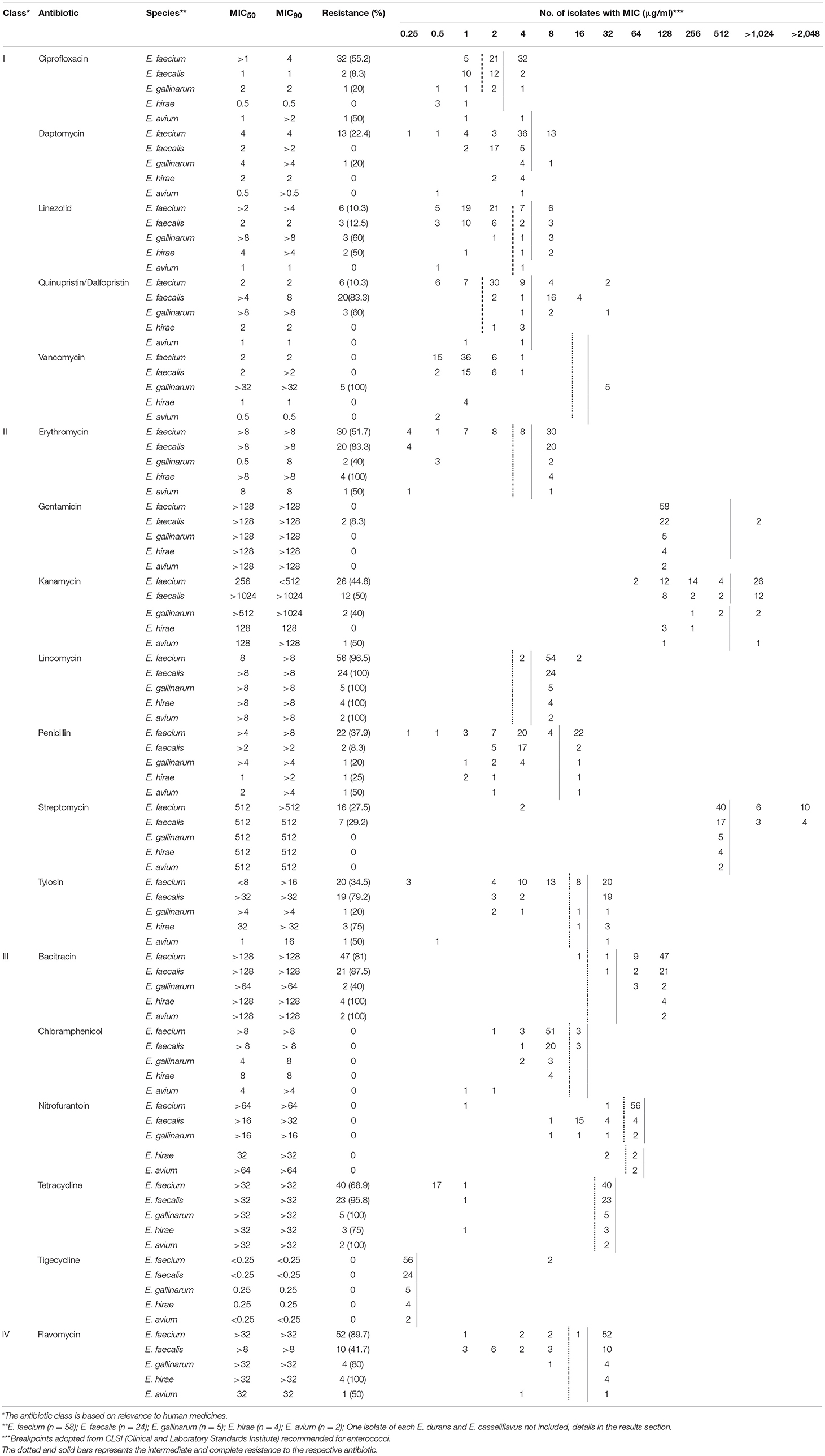
Table 2a. Distribution of MICs and resistance among Enterococcus spp. isolated from broiler chickens.
All E. faecium (n = 58) isolates were susceptible to vancomycin, gentamicin, and tigecycline, while some isolates showed intermediate resistance to chloramphenicol (5.1%) and nitrofurantoin (96.5%). The majority of E. faecium showed a high prevalence of resistance to lincomycin (96.5%), flavomycin (89.7%), bacitracin (81%), tetracycline (68.9%), ciprofloxacin (55.2%), erythromycin (51.7%), kanamycin (44.8%), penicillin (37.9%), tylosin (34.5%), and streptomycin (27.5%). Intermediate resistance to daptomycin (22.4%) as well as a low frequency of resistance to linezolid and quinupristin/dalfopristin (10.3%) were observed among the E. faecium isolates. The most common resistance phenotypes in E. faecium were ciprofloxacin-lincomycin-bacitracin-tetracycline-flavomycin in combination with resistance to other antimicrobials at varying frequencies. Significant effects of antimicrobial feeding were observed for levels of resistance to ciprofloxacin, macrolide, penicillin, and tetracycline. For example, the lowest resistance to ciprofloxacin was observed in birds that received diets with SAL and BAC, while the highest resistance to penicillin was observed in isolates from birds fed diets containing BAC and SAL + BAC (P < 0.05) (Table 2b).
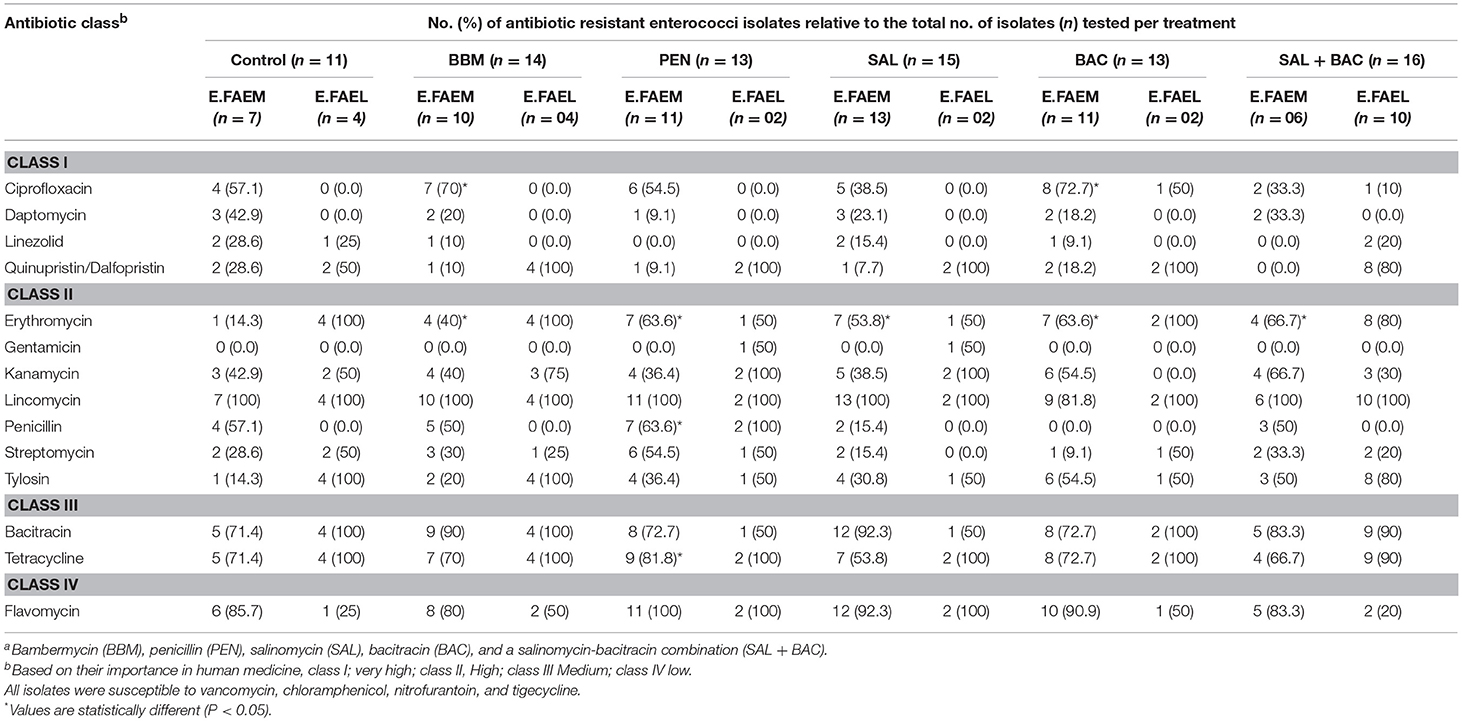
Table 2b. Distribution of resistance phenotypes among enterococci isolated from chickens fed antimicrobialsa.
The E. faecalis (n = 24) isolates were susceptible to vancomycin, tigecycline and daptomycin, while some isolates showed decreased susceptibility to chloramphenicol (12.5%) and nitrofurantoin (25%). As expected, intrinsic resistance to lincomycin (100%) and quinupristin/dalfopristin (83.3%) in all E. faecalis isolates was observed. Of these isolates, a high level of resistance to tetracycline (95.8%), bacitracin (87.5%), erythromycin (83.3%), and tylosin (79.2%) was observed, while resistance to other antimicrobials such as ciprofloxacin (8.3%), linezolid (12.5%), gentamicin and penicillin (8.3%) was less frequent. The most frequently observed profile among E. faecalis was resistance to quinupristin/dalfopristin-erythromycin-lincomycin-tylosin-bacitracin-tetracycline in combination with varying degrees of resistance to other antimicrobials. Among E. faecalis, the highest resistance to macrolides (P < 0.05) was observed in isolates from birds treated with BAM, BAC, and SAL + BAC, while levels of kanamycin and streptomycin resistance were higher in isolates from birds receiving BAC than the control or those receiving other treatments (Table 2b).
Other enterococci isolates, including E. gallinarum (n = 5), E. hirae (n = 4), E. avium (n = 2), E. casseliflavus (n = 1), and E. durans (n = 1), were susceptible to gentamicin and streptomycin (Table 2a). Among these isolates, a high frequency of resistance to lincomycin (100%), tetracycline (92.3%), flavomycin (84.6), bacitracin (76.9%), and erythromycin (53.8%) was observed, while resistance to other antimicrobials such as ciprofloxacin and daptomycin (15.4%), linezolid and tylosin (38.5%), quinupristin/dalfopristin and kanamycin (30.8%), and penicillin (23.1%) was less frequent. Only E. gallinarum and E. casseliflavus isolates were resistant to vancomycin. Differences between antimicrobial feeding were observed in the frequencies of resistance to erythromycin, kanamycin, and quinupristin/dalfopristin. For example, the highest resistance level to erythromycin was observed in isolates receiving BAC and SAL, while levels of kanamycin and quinupristin/dalfopristin resistance were higher in isolates from broilers receiving PEN as compared to the control or other treatments.
Antimicrobial Resistance Genes (ARGs)
The PacBio sequencing data from two isolates in this study provided high quality long reads, allowing accurate detection of plasmids or transposons associated with specific ARGs. A total of 40 ARGs were detected in the genome of all 95 sequenced Enterococcus spp. (Table 3). Among these isolates, the most frequently detected resistance genes included ermA/B (52.6%), tetL (54.7%), msrC (60%), efmA (62.1%), bcr (62.1%), lsaA/E (66.3%), tetM (68.4%), and aac(6′)-Ii (69.5%).
E. faecium
Overall the number of resistance genes was higher in E. faecium than in other Enterococcus species (P < 0.05). Among the 58 isolates, the number of detected genes in individual isolates ranged from 1 to 23, with 63.7% of isolates carrying at least 10 resistant genes. Nine aminoglycoside resistant genes were detected among E. faecium, with aac(6′)-Ii (n = 58), ant(6)-Ia (n = 19); aph(3')-III (n = 19), aadE (n = 18), and ant(9)-Ia (n = 14) being the most common genes detected. The aac(6')-Ii gene was the most prevalent in isolates from BAC-fed birds. Four macrolide resistance genes, lsaA/E (n = 35 isolates), ermA/B (n = 24 isolates), mefA (n = 5 isolates), and mprF (n = 21 isolates) were detected, with ermA/B being predominant in isolates from PEN-fed birds (P < 0.05). Thirty-one and 37 isolates carried the tetracycline resistance genes tetL and tetM, respectively, which were more prevalent in isolates from birds treated with PEN, BAC and SAL + BAC. The 35 isolates carrying the bacitracin resistance gene bcrA were prevalent in BAC and SAL + BAC treated birds compared to the control birds (P < 0.05). The macrolide-lincosamide-streptogramin B (MLSB) genes such as msrC and lnuB/, as well as the gene cluster PBP5 (~36% amino acid similarity to the mecA specific to staphylococci; alignment shown in Figure S1), trimethoprim-sulfamethoxazole dfrE gene homolog and fluoroquinolone mfd resistance genes, were detected in 48, 28, 24, 24, and 24 isolates, respectively. Additionally, a multitude of antimicrobial efflux pump genes such as efmA were found in 52 isolates, along with arlR, efrA/B, lmrB/C/D, pmrA/E, and taeA in 24 additional isolates. In addition, the fabI gene, encoding enoyl reductase, which prevents the inhibition of fatty acid synthesis by triclosan, was found in 24 E. faecium isolates. Several E. faecium isolates were found to carry multiple resistance genes, with the most prevalent resistance combination being bcrR-ermA/B-msrC-mefA-aac-aphA-tetL-tetM. Moreover, 24 E. faecium genomes contained several mutations in the quinolone resistance-determining region (QRDR) of parC, parE, and gyrA genes, and six carried nucleotide substitutions (G2576T) in 23S rRNA (E. coli-K12 strain GenBank accession #HG738867 was used as reference) (data not shown). However, no vancomycin resistance gene was detected in any E. faecium isolate.
E. faecalis
Among all 24 studied isolates, 0 to 23 different resistant genes were detected from individual isolates, with 41.6% harboring at least 10 resistance genes. Consistent with their resistant phenotypes, eight aminoglycoside resistance genes, including sat4 (9 isolates), aph(3′)-III (8 isolates), aad(6) (7 isolates), ant(6)-Ia (7 isolates), aac(6′)-Ii (2 isolates), aac(6′)-aph(2″) (2 isolates), spc/str (2 isolate), and ant(9)-Ia (1 isolate), were found among the 24 E. faecalis isolates. No significant effects of antimicrobial feeding on the distribution of these genes were observed. For macrolide resistance, all E. faecalis isolates (100%) carried the lsaA/E gene, while the ermA/B and mprF genes were detected in 20 and 7 of them, respectively. The trimethoprim-sulfamethoxazole resistance dfrE gene homolog was detected in 22 isolates. The tetracycline resistance tetM and tetL genes and the bacitracin resistance bcr gene were detected in 21, 19, and 17 bacitracin-resistant E. faecalis isolates across the birds with antimicrobials in their diet. The efflux pump genes arlR, cdeA efmA, efrA/B, emeA, lmrB/C/D, pmrA/E, and taeA, as well as the triclosan resistance fabI gene were found in seven E. faecalis isolates. Like E. faecium, multiple substitutions in the quinolone resistance-determining region (QRDR) of parC, parE, and gyrA were detected in two E. faecalis and three contained nucleotide G2576T substitutions in 23S rRNA. No vancomycin-resistant gene was detected in any of E. faecalis isolates.
The other Enterococcus species harbored a limited number of resistance genes. Among those, aac(6')-Ii, bcrA, ermA/B, tetL, and tetM were identified in some of the Enterococcus species included in this study. The vanC gene was exclusively detected in E. gallinarum and E. casseliflavus genomes.
Correlation Between AMR Phenotype and Genotype
For the majority of antimicrobials, there was a strong positive correlation between resistance phenotypes and genotypes (Table 4).
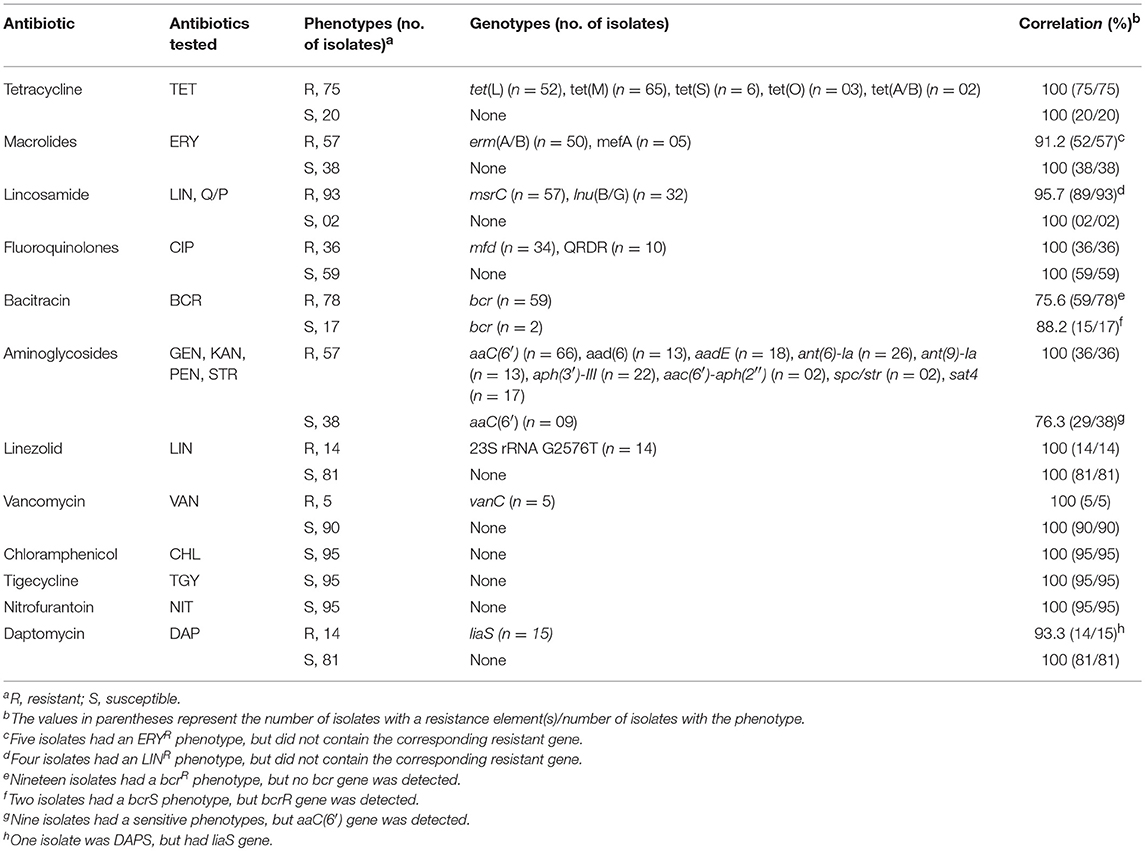
Table 4. Resistance phenotype and genotype correlations among 95 enterococci isolated from broiler chickens.
Tetracycline
At least one tetracycline resistance gene was detected in all 75 isolates with phenotypic resistance to tetracycline. Of these, 65 contained the tetM gene while 52 carried both the tetM and tetL genes. The tetS, tetO, and tetA/B genes were found in six, three, and two tetracycline-resistant isolates, respectively. No tetracycline resistance gene was detected in any of the 20 tetracycline susceptible isolates.
Macrolide, Lincosamide Streptogramin B, and Quinupristin-Dalfopristin (MLSB)
Fifty of the 57 isolates resistant to erythromycin (macrolide) harbored ermB or ermA alone, while three contained both genes. Five isolates harbored the mefA gene, of which three contained both ermB and mefA genes, while two had a combination of ermA, ermB, and mefA. No corresponding macrolide resistance genes were detected in five resistant isolates and none were detected in the 38 macrolide-susceptible isolates. E. faecalis is intrinsically resistant to lincomycin and streptogramin B and all 24 (100%) and 20 (83.3%) isolates of this species carried lsaA/E and erm(A/B) gene, respectively. The msrC gene, which is known to be intrinsic to E. faecium (Champagne et al., 2011) and confers resistance to macrolides, was found in 48 (82.6%) and 6 (25%) of the E. faecium and E. faecalis isolates, respectively.
Fluoroquinolone
Either the mfd gene or mutations in parC, parE, or gyrA were detected in all 36 resistant isolates associated with ciprofloxacin resistance. None of the susceptible isolates had the mfd gene or parC, parE, or gyrA mutations detected in their genomes.
Bacitracin
Among 78 bacitracin resistant isolates, 59 (75.6%) harbored the bcr gene, while this gene containing no mutation was also detected in 2 of the 17 phenotypically susceptible isolates.
Aminoglycoside
Various levels of resistance to aminoglycosides were observed, and their corresponding genes aac(6′)li, aac(6′)li, and aac(6′)lih were detected within the genomes. The two E. faecalis isolates with high levels of gentamicin resistance carried a corresponding bifunctional aminoglycoside-modifying enzyme, aac(6′)-aph(2″) [one isolate contained both aac(6′)-Ie-aph(2″)-Ia and aph(2″)-Ic], that conferred resistance to high concentrations of gentamicin.
Linezolid
All 14 enterococci that showed resistance to linezolid carried corresponding nucleotide substitutions in 23S rRNA.
Vancomycin
The resistance to vancomycin in E. gallinarum and E. casseliflavus was associated with the presence of vanC operon, comprising up to seven genes as detected in their respective genomes. Moreover, all vancomycin susceptible isolates lacked the corresponding van related genes in their genomes.
Chloramphenicol, Tigecycline, and Daptomycin
The isolates that were susceptible to chloramphenicol, tigecycline, and daptomycin consistently had no corresponding genetic determinant detected in their genomes.
Virulence Genes of Enterococcus spp.
Genes encoding virulence factors from the 95 assembled genomes of the Enterococcus isolates were identified using VirulenceFinder (Table 5). Overall, no significant differences in the distribution of virulence factors or their associated virulence phenotypes were observed among isolates of the same species collected from different antimicrobial feeding groups, source, or day of isolation (data not shown).
E. faecium
The majority of E. faecium genomes were positive for two well conserved virulence genes: efaAfm (57/58, 98.3%) and acm (51/58, 87.9%). Additionally, a putative virulence gene, bsh (978 bp), encoding a 326-amino acid bile hydrolysis enzyme (EC 3.5.1.24) was identified in the majority of E. faecium (54/58, 93.1%) genomes, and such isolates exhibited a positive bile salt hydrolyze (BSH) phenotype. Among the BSH-positive isolates, 20 (34.5%) had low, 25 (43.1%) medium, and 13 (22.4%) exhibited high BSH activity based on the size of precipitation zones (Table 1). Moreover, a 663-bp gene coding for a putative membrane protein hemolysin III homolog was detected in four hemolysin-positive E. faecium (6.8%). Interestingly, in contrast to human clinical cases, all E. faecium isolates of poultry origin tested in this study lacked the virulence gene espfm, encoding a putative surface protein precursor that plays a major role in biofilm formation and is associated with urinary tract infections (Semedo et al., 2003).
E. faecalis
A total of 15 well-defined virulence genes were detected among E. faecalis genomes. Nine of the 15 genes were identified in all isolates, while four E. faecalis genomes (IDs: 2807, 2810, 2891, and 2879) were positive for the agg gene, encoding an aggregation protein involved in adherence to host cells. The genes associated with activation, transportation and modifications of the cytolysin (cylA, cylB, cylL, and cylM) (Hallgren et al., 2009) were identified in a single E. faecalis genome (day 36, litter sample ID 2968) that clustered closely with E. faecalis of human origin. All E. faecalis were positive for a chromosomal-mediated gene gelE, encoding gelatinase, an extracellular zinc metallo-endopeptidase secreted by E. faecalis species that hydrolyze gelatin, collagen, hemoglobin and other bioactive peptides. Furthermore, the gelE-bearing isolates were found positive for the locus fsrB, encoding a processing enzyme that liberates gelatinase biosynthesis activating pheromone (GBAP) peptide, indicating the importance of fsr in virulence and disease (Hancock and Perego, 2004). Consistent with their gelE and fsrB genotypes, all E. faecalis isolates showed in vitro gelatinase phenotypes (a turbid halo zone around the colonies) (Table 1). The bsh gene was identified in all E. faecalis isolates, and these isolates also showed BSH activity in vitro. Our study also highlighted that all E. faecalis isolates carried a chromosomal hylA/B gene, which encodes the hydrolytic enzyme hyaluronidases (Golinska et al., 2013), and accordingly these isolates exhibited BSH phenotypes in vitro (Table 1). The hemolysin III homolog was detected in three E. faecalis (12.5%) which aligned with their hemolytic activity. Other virulence factors identified in E. faecalis in this study included sex pheromone-associated genes camE, cad, cCF10, and cOB1 in 91.7–100% of isolates, respectively, and the cell wall adhesion expressed in the serum gene efaAfs (95.8%). Additionally, a novel enterococcal leucine-rich protein A gene, elr (91.7%), which facilitates bacterial escape from host immune defenses, and the thiol peroxidase gene tpx (100%), which protects pathogenic bacteria against oxidative stress (Fisher and Phillips, 2009), were detected. The endocarditis and biofilm-associated pili gene locus ebpABC, an associated sortase gene srtA, and a cell wall-anchored adhesion gene ace were also detected in all E. faecalis isolates. The espfs surface protein found in human clinical isolates (Diarra et al., 2010) was not detected in any of the E. faecalis isolates originating from poultry.
Among other enterococci, including E. gallinarum, E. casseliflavus, E. avium, E. hirae, and E. durans, only three isolates contained the hemolysin III homolog and exhibited their corresponding hemolytic phenotypes while all carried the bsh gene, which was consistent with their BSH phenotype (Table 1). No other virulence genes were detected among these isolates. Additionally, all enterococci isolates were negative for asa1 (aggregation substance), a virulence gene commonly found in human pathogenic isolates (Hallgren et al., 2009).
Mobile Genetic Elements
Plasmids
All three classes of plasmids, including the rolling circle replication (RCR), Inc18 and the pheromone-responsive plasmids, were detected among the sequenced genomes using the plasmid finder database. There was at least a minimum of one to several plasmids detected in a majority of the genomes sequenced in this study. No significant effect of antimicrobial feeding on the distribution of plasmids was observed. The plasmids identified among the sequenced genomes are listed in Table 1.
The pAMβ-1 family of non-conjugative broad host range plasmids was common among E. faecium genomes (Grohmann et al., 2003). Several ARGs, including ermB, aad6, Aph(3′)-IIIa, tetM/L, Ant(9)-Ia/Ant(9)-Ia, lnuB, Sat4, were present on the plasmid-bearing contigs in 6 of 58 (10.3%) E. faecium genomes sequenced in this study (Table 6). Interestingly, an aminoglycoside-streptothricin resistance gene cluster aadE-sat4-aphA-3 of ~3–3.5 kb in size was identified on plasmid-associated contigs in three E. faecium genomes (two from BAC treatment cloacal samples collected on day 14 and one from a BAM treatment litter sample collected on day 36). This gene cluster was located on a DNA fragment containing a Tn5405-like (putative) transposable element containing site-specific recombinase and other phage-related regions. Furthermore, all genomes that were positive for the aadE-sat4-aphA-3 gene cluster were also positive for the ermB gene (Figure 1). No plasmid sequence was detected in four (6.9%) E. faecium genomes. The isolates from BAC and BAM treatment contained a relatively higher number of plasmids carrying ARGs as compared to the control or other treatment groups.
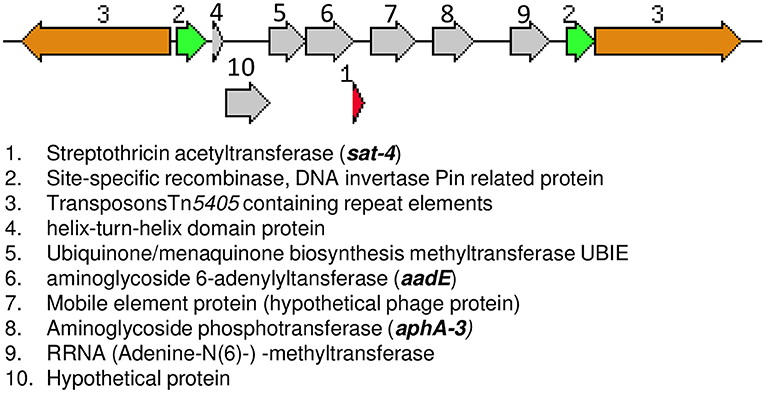
Figure 1. Schematic of gene cluster (not scaled) containing aadE-sat4-aphA-3 on a Tn5405-like (putative) transposable element linked with ermB gene found in three studied enterococci (not shown). The encoded proteins are labeled 1–10 on the gene cluster and their descriptions are provided at the bottom.
The pheromone-inducible plasmid pTEF2, structurally similar to pCF10 and pPD1, was the most prevalent type of plasmids detected in E. faecalis. Additionally, pTEF3, a non-conjugative plasmid harboring a pTEF2-like prgZ pheromone receptor adjacent to multiple insertion sequence (IS) elements (IS1216 and IS256), was also identified in two E. faecalis genomes. Two other E. faecalis genomes contained a pUB110 plasmid. Several ARGs were detected on plasmid-containing contigs in 16 of 24 (66.7%) E. faecalis genomes. Like E. faecium, an aadE-sat4-aphA-3 cluster linked with the ermB gene was detected on a plasmid-associated contig in one of E. faecalis genomes (Table 6). No plasmid sequence was detected in five (20.8%) E. faecalis genomes.
No plasmid was detected in the other sequenced Enterococcus species, including E. gallinarum, E. casseliflavus, E. avium, E. hirae, and E. durans (Table 1).
Transposons
The Tn3-like transposon Tn917 (~18-kb in size) was detected in several of the sequenced genomes. The Tn917 transposon contained five open reading frames (ORFs); all oriented in the same direction on the transposon and were flanked by 38-bp inverted repeats. The presence of intact transposons and IS elements may lead to a variety of genetic rearrangements, including deletions, inversions, and translocations. All genomes that harbored the Tn917 also harbored the macrolide erythromycin resistance gene erm (B) in close proximity. The other four ORFs identified were rRNA methylase, resolvase, transposase, and an ORF of unknown function. In 24 (41.3%) E. faecium and 20 (83.3%) E. faecalis genomes, the Tn917 transposon was linked with the ermB gene. The Tn917 transposon was completely absent in other species except in one E. avium.
Several Enterococcus genomes also contained a Tn916 family of integrative conjugative elements (ICEs). Most studied enterococci also carried a tetracycline resistance gene, tetM, ~11.4 kb upstream of Tn916. The Tn916 elements were detected in 29 (50%) E. faecium, 21 E. faecalis (87.5%), 3 E. gallinarum (60%), and 2 E. hirae (50%). No sequence homology to Tn916 was detected in other species.
The Tn6000 (formerly EfcTn1), a novel Tn916-like conjugative transposon linked with the tetracycline resistance tetS gene, was detected in only one isolate of each E. faecium and E. gallinarum.
Two to three copies of putative Tn552 transposons of the Tn3 family were detected in 12 (20.7%) E. faecium. The mobility module of Tn552 was comprised of genes such as tnpA (transposase), tnpB (ATP-binding protein), bin3 (resolvase-integrase) as well as arsR, tetR, and phoB (family of transcriptional regulators).
Like E. faecium, the genomes of E. hirae and E. durans also harbored two to three copies of Tn5252 conjugative transposons. No transposon was detected in the E. casseliflavus genomes.
Multi-Locus Sequence Typing (MLST) and Phylogenetic Analysis
Only the 58 E. faecium and the 24 E. faecalis genomes were included in MLST analysis due to the lack of an MLST scheme for other Enterococcus spp. (Table 1). The E. faecium isolates comprised 14 different MLST types, including 12 unknown sequence types (STs), including ST9 (9 isolates), ST26 (7 isolates), ST32 (9 isolates), ST-54 (5 isolates), and ST-329 (4 isolates). A total of 14 sequence types were found among E. faecalis isolates, with the most prevalent type being ST249 (7 isolates) and ST21 (3 isolates). No previously reported E. faecalis ST16 from poultry, swine and human urinary tract and endocarditis infections were detected among any of the studied isolates.
The initial phylogenetic tree was built using all 95 sequenced enterococci genomes based on the core genome single nucleotide polymorphism (SNPs). The E. faecalis V583 genome (GenBank accession #NC_004668) was used as reference. Each genome was placed into a correct species-specific cluster, further validating the accurate identity of each species by WGS based on the rMLST scheme used in this study (Figure S2). The overall topology of the tree showed substantial inter-species similarities, although some inter-species variations were also noticed with the PFGE profiles (Figure 2) of selected isolates from the control group as well as from BAM- and BAC-fed birds, which showed the overall highest AMR prevalence.
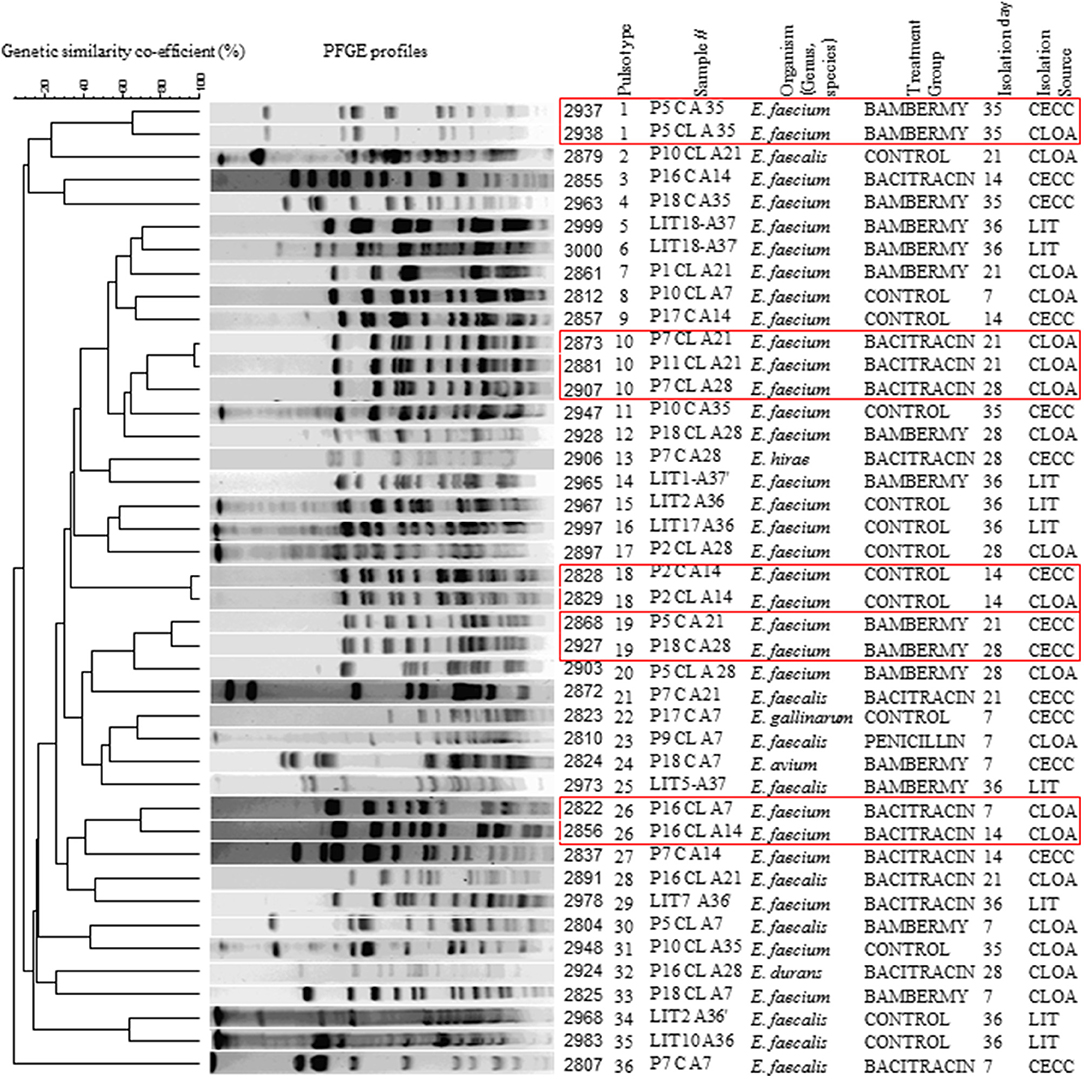
Figure 2. Dendrograms of pulsed-field gel electrophoresis (PFGE) profiles of smaI-digested genomic DNA showing relationship between 42 enterococci isolated at day 7 to 35–36 from cecal, cloacal, and litter samples of broiler fed control, bambermycin (BAM), and bacitracin (BAC). Due to their significant effects on the overall AMR, subsets of isolates were selected from BAM and BAC treatments to assess their diversity. The dendrogram on the left represents the genetic similarity co-efficient (%). The gel image in the middle separated by white space represents the banding pattern of individual isolate labeled on the right. Pulsotype refers to subtypes labeled as 1, 2, 3, and so forth. Day of isolation refers to broiler age. Two distinct clusters were observed, cluster I (pulsotype 1–4) and cluster II (pulsotype 5–36). The genetically homogeneous isolates presenting similar pulsotype are shown in red boxes.
Because of the limited numbers of E. hirae, E. gallinarum, and E. casseliflavus isolates in this study, only genomes of E. faecalis (n = 24), and E. faecium (n = 58) were comparatively analyzed, with their respective human clinical reference genomes obtained from the NCBI database.
E. faecium
The genetic relatedness between the 58 studied E. faecium genome sequences and the 73 E. faecium reference strains from human clinical cases was determined (Figure 3). Seven distinct clades were identified, with most genomes of strains from human clinical cases constituting a major (clade III, n = 48) and a minor clade (clade V, n = 23). All 58 E. faecium clustered into five distinct clades, containing six isolates in clade I, five in clade II, 11 in clade IV, 28 in clade VI, and eight in clade VII. Interestingly, eight isolates in clade VII clustered together with the human clinical reference E. faecium strain 1070_EFCM (tree id, JWEB01_1, GenBank accession # JWEB01000000), obtained from a tertiary care hospital's intensive care unit (ICUs). This strain exhibited the highest degree of genetic relatedness (>99%) with a minimum pairwise SNP difference in the range of seven to 15, with isolate ID 2997 from the cloacal samples of the 36 days BAM-fed broiler being the most closely related, with only seven SNP differences (Figures 3, 4).
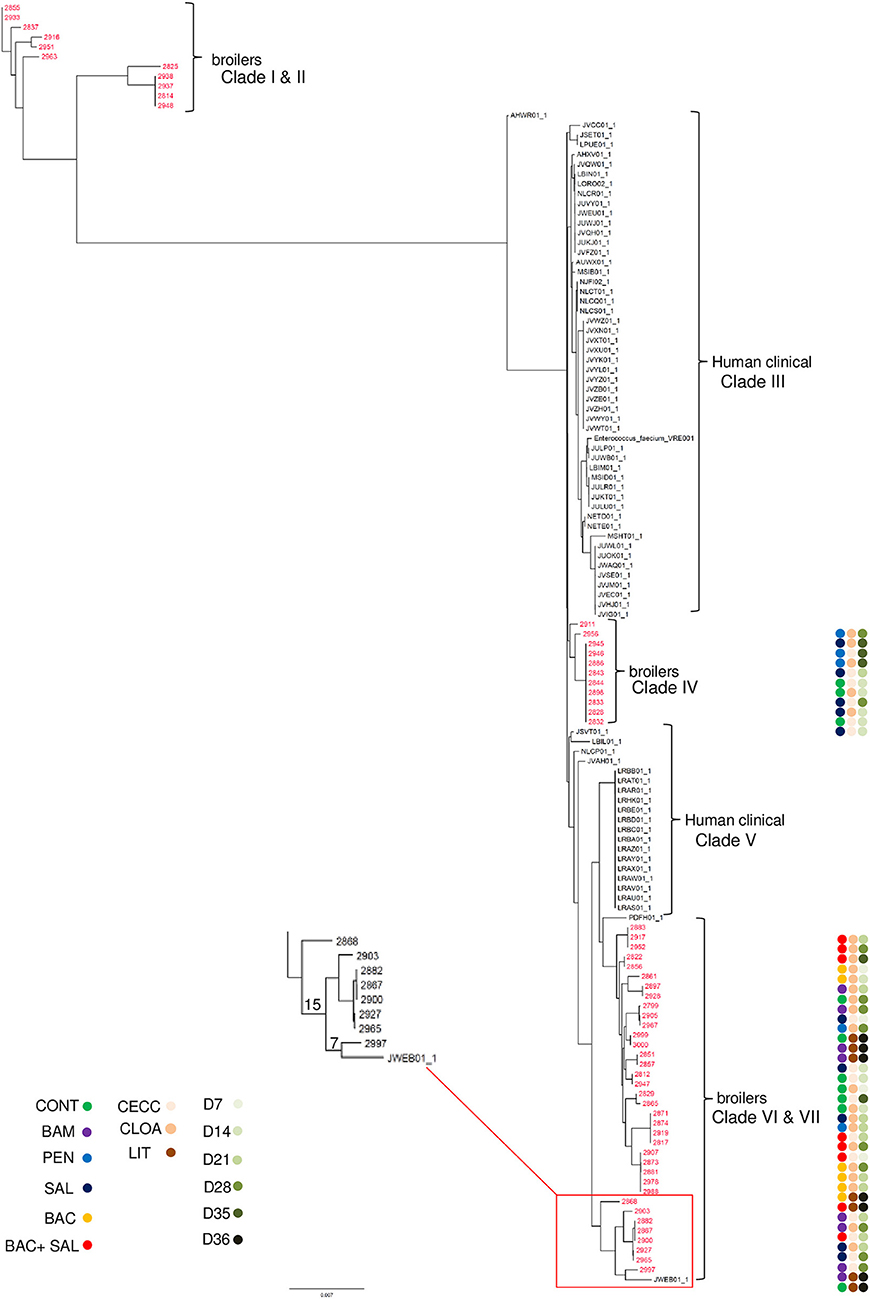
Figure 3. Phylogeny of E. faecium based on core genome (>99%) single-nucleotide polymorphisms (SNPs) analysis using SNVPhyl. E. faecium strain Aus0004 used as reference in this analysis. A maximum likelihood tree of 58 E. faecium multi-resistant isolates obtained from broiler chickens in the present study (labeled) and 73 reference isolates from human clinical sources (labeled). Isolates in each clade are labeled as I- VII. Studied isolates showing some close similarity with isolates of human clinical origin are presented in the red box. The enlarged view of closely related isolates from broiler chickens and an isolate associated with clinical infection in humans. The number on the tree nodes represents SNP differences between the genomes.
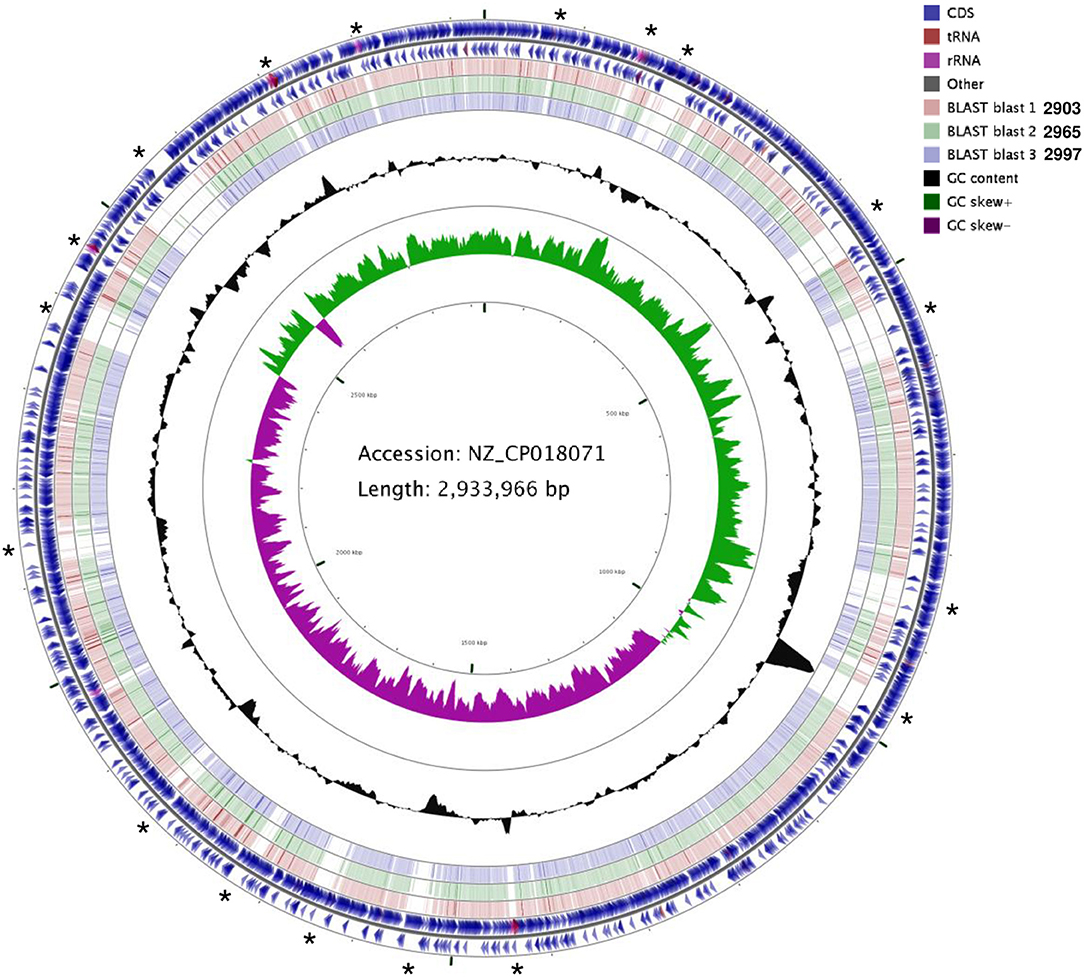
Figure 4. CGView: comparison of a bacterial genome from isolates of broiler origin with isolate of human clinical origin (accession # NZ_CP018071) using blastn. The contents of the feature rings (starting with the outermost ring) are as follows. Ring 1, 2: forward and reverse strand features read from the primary sequence GenBank file. Rings 3, 4, 5: Genomes 2903, 2965, 2997 in fasta format. Rings 7, 8: GC content and GC skew. The gaps in the alignment represent the regions missing in the query genomes in comparison to the reference genome.
E. faecalis
Phylogenetic inferences of 24 genomes from this study and 82 references revealed that the poultry isolates interspersed throughout the tree (Figure 5). Genetic relationships were found between several genomes of studied isolates and those from environmental sources (aquatic, isolated from agricultural feeding sites). For example, four sets of isolates from broilers administered different antimicrobials, and at different sampling days, clustered with aquatic isolates. Furthermore, isolates from six different treatment groups were evenly distributed across the branches of the phylogenetic tree, except for a few isolates from litter that clustered together, suggesting their clonal nature. Overall, the genetic relatedness was higher among E. faecalis isolates that originated from similar habitats. The litter isolate (ID 2968 of ST82) from control group that harbored cytolysin genes showed some relationship with human isolates (Figure 6).
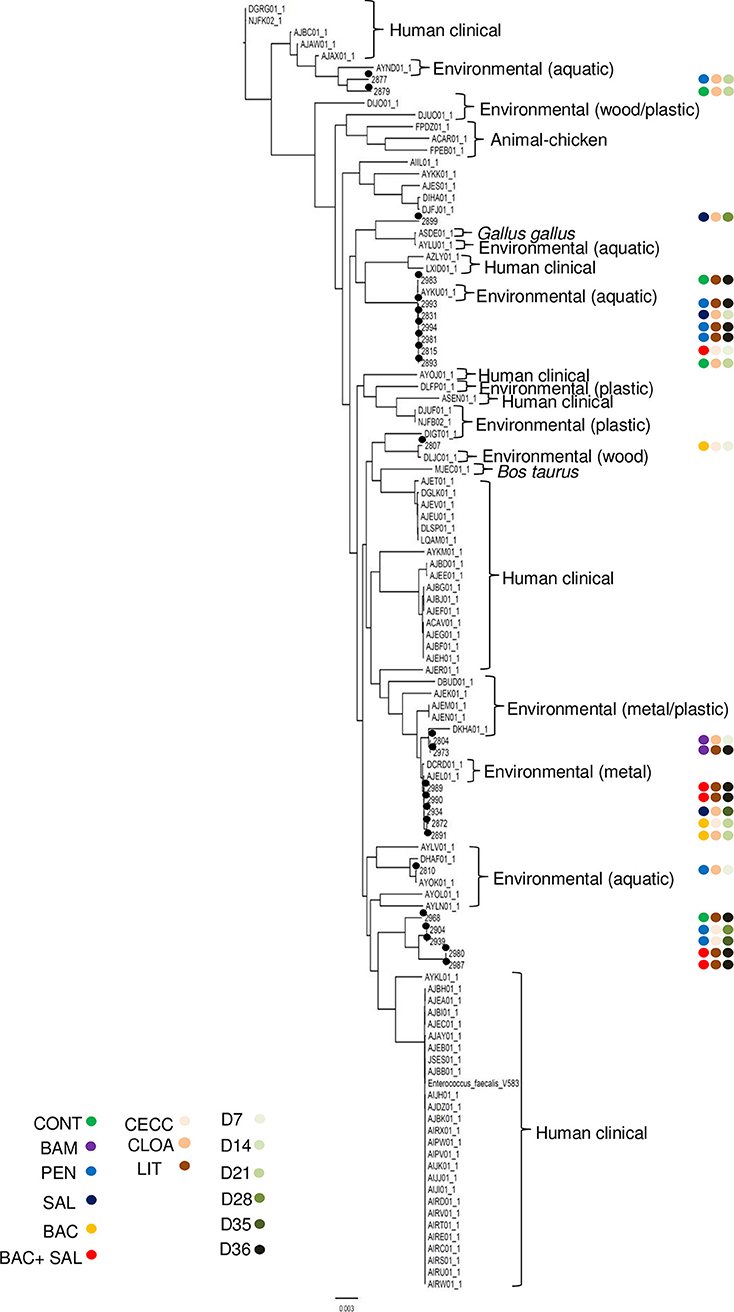
Figure 5. Phylogeny of E. faecalis based on core genome (>99%) single-nucleotide polymorphisms (SNPs) analysis using SNVPhyl. E. faecalis strain V583 was used as reference in the analysis. A Maximum-likelihood tree of 24 E. faecalis multi-resistant isolates obtained from broiler chickens in the present study (dark dots) and 81 isolates from human clinical (from patients with bloodstream and urinary infections), environment (aquatic, metal, plastic, wood) and animal sources (labeled). The E. faecalis strain V583 served as a common reference.
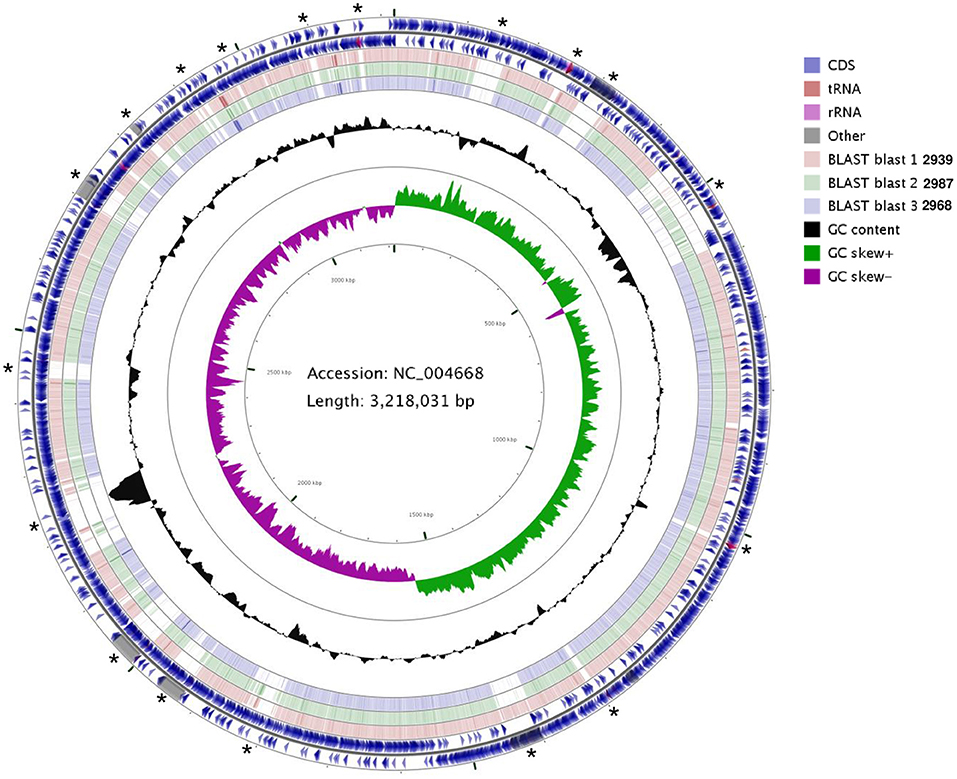
Figure 6. CGView: Comparison of genome from study isolates with an isolate of human clinical origin (accession # NZ_CP018071) using blastn. The contents of the feature rings (starting with the outermost ring) are as follows. Ring 1, 2: forward and reverse strand features read from the primary sequence GenBank file. Rings 3, 4, 5: Query genomes 2939, 2987, 2968 in fasta format. Rings 7, 8: GC content and GC skew. The gaps in the alignment represent the regions missing in the query genomes in comparison to reference genome.
Discussion
Enterococcal species are opportunistic pathogens in both humans and animals (Jett et al., 1994) and their ability to acquire antibiotic resistance presents a challenge for infection control. The ecology of AMR in enterococci in relation with antimicrobial usage in poultry is not well-characterized. In this study, the effect of in-feed antimicrobials on the diversity and promotion of AMR as well as relationship between resistance phenotypes and genotypes among enterococci isolated from broiler chickens were investigated.
Of the 95 identified colonies E. faecium was the most predominant species across different treatment groups, sources (cloacae, ceca, or litter) and sampling days. These findings were consistent with previous studies (Yoshimura et al., 2000; Diarra et al., 2010). In contrast, other studies (Kaukas et al., 1986; Aarestrup et al., 2000a) have found that E. faecalis was the most predominant species from poultry. Furthermore, E. cecorum has been reported to be the most abundant species in the intestines of 3–5 week old broilers (Devriese et al., 1991; Gong et al., 2002), but this species was not identified in our study. The difference in the occurrence of species between studies may be due to differences in rearing conditions, source of chicks, sampling strategies, isolation and identification procedures, geographic disparities, or the use of medicated diets (Hayes et al., 2004). Susceptibility data of the 95 enterococci in this study showed a high proportion of resistant isolates from birds fed diets containing BAC. In agreement with an earlier study (Diarra et al., 2010), multiresistance was frequently detected among E. faecium and E. faecalis isolates that accounted for 61 and 25.3% of total enterococci population, respectively.
Bacitracin has been commonly used in poultry production to control infections caused by Clostridium perfringens (Diarra and Malouin, 2014). Our data showed that of the 59 isolates carrying the bcr gene, 93.3 and 93.4% were from birds fed diets containing BAC and SAL + BAC, confirming the relationship between bacitracin usage and the selection of isolates harboring the corresponding resistant determinant. The resistance to flavomycin has been described as an intrinsic property of enterococci (Aslam et al., 2012; Barros et al., 2012; Diarra and Malouin, 2014). The comparative genomics analysis of resistance and susceptible population in this study suggests the role of cell surface proteins as a possible resistance mechanism to this antimicrobial. Bambermycin (flavomycin) in feed was also found to promote flavomycin resistance in enterococci.
In the present study, ~60% of total isolates were found to be resistant to erythromycin; however, a significantly higher frequency of resistance to erythromycin (83%) and tylosin (79%) were observed in E. faecalis. Studies have reported resistance to MLSB in enterococci involving 23S rRNA methylation as well as active efflux and inactivating enzymes (Hollenbeck and Rice, 2012; Jaglic et al., 2012). In our study, the co-resistance was also frequently observed in broilers receiving BAC. Approximately 22% of enterococci were simultaneously resistant to erythromycin, lincomycin, and quinupristin-dalfopristin, suggesting an acquired MLSB related co-resistance. The streptogramin A resistance genes vatD or vatE were not detected in this study as in a previous study (Weisblum, 1985). Interestingly, the macrolide resistance erm gene was found to be associated with the tet gene, which was located on a transposable element in the majority of E. faecalis and E. faecium isolates. These transposable elements encode a full complement of machinery for conjugation as well as regulatory systems to control excision from the chromosome, suggesting that conjugative transfer of ARGs to a new bacterial host happen in the broiler gut (Roberts and Mullany, 2009; Wozniak and Waldor, 2010).
Overall, only 28.4% of the 95 enterococci were resistant to penicillin. However, a significant difference was found between E. faecium (38%) and E. faecalis (8%) isolated from poultry in the frequency of resistance to penicillin. It is well-known that penicillin resistance in E. faecium can arise due to low affinity or binding (due to point mutations in the C-terminal of pbp5 gene) to penicillin-binding protein 5 (PBP5), as well as expression of β-lactamase (Hollenbeck and Rice, 2012). Other PBPs such as PBP3r, which shares similarities with PBP2, which in turn confers resistance to methicillin in staphylococci, have also been reported to confer resistance to penicillin in other enterococci species (Rice et al., 2001). In the present study, analysis of pbp5 gene of the isolates resistant to penicillin, revealed various point mutations in the C-terminal when compared to penicillin-susceptible isolates, which is consistent with previous reports (Ligozzi et al., 1996; Klibi et al., 2008). In spite of no β-lactamase genes being detected, a gene cluster homolog to the adaptive region of mecA, responsible for resistance to antibiotics such as methicillin, penicillin and other penicillin-like antibiotics (Stapleton and Taylor, 2002), was found in 24 (41.4%) and 6 (25%) of the E. faecium and E. faecalis isolates, respectively. However, no association was found between this gene cluster and resistance to PEN, as only 7 (25.9%) of the 35 isolates resistant to penicillin were positive for this mecA-like cluster. The characterization of this gene cluster may elucidate its role in β-lactam resistance in enterococci.
Resistance to linezolid has been documented among enterococci from poultry (Diarra and Malouin, 2014). Different mechanisms for linezolid resistance have been described, with the most common being mutations in genes encoding the 23S rRNA (Eliopoulos et al., 2004; Miller et al., 2014). We observed a G2576T mutation in 23S rRNA among the resistance isolates (position 2576 refers to the nucleotide position relative to 23S rRNA in E. coli). This particular mutation impedes the linezolid binding site and confers resistance. The importance of mannose phosphotransferase (ManPTS) in linezolid resistance in E. faecium has been recently reported (Geldart and Kaznessis, 2017), however no such observation was noted in this study.
Resistance to glycopeptide antibiotics like vancomycin has generated considerable research interest during the last few decades, as vancomycin is among the drugs of last resort to treat infections in humans caused by enterococci. As expected, all E. gallinarum and one E. casseliflavus isolated in this study carried the vanC operon, which is an intrinsic property of these enterococci species (Reid et al., 2001). The absence of vancomycin resistance and its associated genes in E. faecium and E. faecalis in the present study was expected and in agreement with an earlier study in the USA (Butaye et al., 2001). In contrast, resistance to vancomycin in enterococci was linked to the use of avoparcin in Europe (Aarestrup et al., 2000b).
Regardless of feed groups, 44 and 24% of the isolates were resistant to kanamycin. The prevalence of resistance to streptomycin (MIC > 2048) was relatively higher than resistance to other aminoglycosides, as only 14 isolates (14.8%) showed high level (HL) of resistance to this antibiotic, in agreement with others studies (Aarestrup et al., 2000a; Hayes et al., 2004; Tremblay et al., 2011). The aminoglycoside-modifying aac (6′)-Ii gene, encoding a 6′-N-aminoglycoside acetyltransferase found in almost all (98.5%) our aminoglycoside resistant isolates, has been reported to be chromosomally located in E. faecium and to confer resistance to synergism between cell-wall acting antibiotics and aminoglycosides (Eliopoulos et al., 2004). All 58 E. faecium and all four E. gallinarum harbored the aac(6′)-Ii gene. This gene was predominantly detected in isolates from birds fed BAC (86.7%) and those fed BAC and SAL (81.3%).
In the present study, the tetM gene was co-located with ermB on a conjugative transposon related to the Tn916-like family, thus the use of MLSB antimicrobials could co-select for tetracycline resistance in broilers as concluded in a previous study (Cauwerts et al., 2007). Moreover, the Tn552 transposons were found to be associated with a tellurite resistance gene, and the Czc efflux system which mediates resistance to heavy metals such as cobalt, zinc, and cadmium (Dressler et al., 1991). The genetic map of Tn5252 was found to be somewhat similar to a streptococcal conjugative transposon carrying the β-lactamase class C family (100% sequence similarity to serine hydrolase). Several open reading frames (ORFs) also showed sequence homologies to DNA processing genes such as trsE-like transmembrane ATPase, integrases, excisionases, and transcriptional regulators responsible for conjugal transfer of these elements (Alarcon-Chaidez et al., 1997). The detected transposons and plasmids in the studied enterococci suggest their potential to disseminate ARGs associated with them, which could contribute to the mobilization of AMR.
A total of 17 virulence genes were examined in all 95 enterococcal genomes, including factors involved in bacterial replication, host-colonization and tissue damage and modulation of the host inflammatory system (Jett et al., 1994; Ike, 2017; Pillay et al., 2018). E. faecalis harbored a greater number of virulence genes (15 of 17 detected genes) than E. faecium, in agreement with a previous study (Champagne et al., 2011). The two virulence genes, esp and asa1, which are generally associated with human infections (Hallgren et al., 2009), were detected in none of the enterococci studied. E. faecalis has been reported to be associated with pulmonary hypertension syndrome and endocarditis in broilers of all ages (Tankson et al., 2001, 2002). Except E. faecium and a few E. faecalis, the studied isolates were phylogenetically unrelated to human clinical isolates, suggesting that studies on their virulence potential in humans and even in poultry are warranted.
This longitudinal study provided useful information on the distribution of antimicrobial-resistant and virulent genotypes of enterococci from broiler chickens fed with different antimicrobial agents compared to control birds. In-depth generated genomic data could be used in AMR risk analysis and modeling to further improve understanding of AMR emergency and transmission, which could help to design production practices to mitigate antimicrobial resistance in broilers.
Conclusions
Multiple drug resistant enterococci continue to create issues for human health, and their presence in animals raised for food may threaten the sustainability and safety of food production. Data from this study showed that the use of BAC and BAM in feed significantly promoted the resistance phenotype and genotype of enterococci that persisted in broilers. The persistence of AMR enterococci specifically in litter could have an impact on the environment and subsequently on food safety when resistance genes are transferred to pathogenic bacteria. It is imperative to understand the molecular ecology of AMR enterococci in order to control their dissemination in poultry production. Despite the limited number of studied isolates, a correlation was found between WGS data and phenotype. Although a few discrepancies were noted for drugs for which enterococci show intrinsic resistance, our study is overall a critical step in advancing the use of WGS in resistance phenotype prediction among Gram-positive bacteria.
Author Contributions
MD and ET conceptualized the study design. Whole genome sequencing was performed by NG and KA. Bioinformatics analyses and AST was performed by MR, JP, and MD. XY, JP, RZ, and TM contributed to experimental design. MD applied the statistical tests. MR and MD wrote the manuscript with input from all coauthors. MD was the principle investigator, who provided overall guidance, mentorship, and resources throughout the scope of this project.
Conflict of Interest Statement
The authors declare that the research was conducted in the absence of any commercial or financial relationships that could be construed as a potential conflict of interest.
Acknowledgments
This work was financially supported by Agriculture and Agri-Food Canada through the Sustainable Agriculture Environmental Systems (SGES) Program and the Federal Genomics Research and Development Initiative to mitigate antimicrobial resistance (GRDI-AMR) project (PSS #1858) of Government of Canada. The funders had no role in study design, data collection and analysis, or preparation of the manuscript. We thank F. Diarrassouba, H. Rempel and J. Proudfoot (Agassiz Research and Development Centre, AAFC, Agassiz, BC, Canada) and members of the Integrated Rapid Infectious Disease Analysis (IRIDA) team for their assistance.
Supplementary Material
The Supplementary Material for this article can be found online at: https://www.frontiersin.org/articles/10.3389/fsufs.2018.00083/full#supplementary-material
Table S1. List of E. faecalis reference genomes.
Table S2. List of E. faecium reference genomes.
Figure S1. Amino acid sequence alignment of enterococci pbp5 and Staphylococcus mecA proteins.
Figure S2. Species specific clustering of each species by WGS based SNV analysis.
References
Aarestrup, F. M. (2000). Occurrence, selection and spread of resistance to antimicrobial agents used for growth promotion for food animals in Denmark. APMIS Suppl. 101, 1–48. doi: 10.1111/j.1600-0463.2000.tb05380.x
Aarestrup, F. M., Agerso, Y., Gerner-Smidt, P., Madsen, M., and Jensen, L. B. (2000a). Comparison of antimicrobial resistance phenotypes and resistance genes in Enterococcus faecalis and Enterococcus faecium from humans in the community, broilers, and pigs in Denmark. Diagn. Microbiol. Infect. Dis. 37, 127–137. doi: 10.1016/S0732-8893(00)00130-9
Aarestrup, F. M., Kruse, H., Tast, E., Hammerum, A. M., and Jensen, L. B. (2000b). Associations between the use of antimicrobial agents for growth promotion and the occurrence of resistance among Enterococcus faecium from broilers and pigs in Denmark, Finland, and Norway. Microb. Drug Resist. 6, 63–70. doi: 10.1089/mdr.2000.6.63
Abat, C., Huart, M., Garcia, V., Dubourg, G., and Raoult, D. (2016). Enterococcus faecalis urinary-tract infections: do they have a zoonotic origin? J. Infect. 73, 305–313. doi: 10.1016/j.jinf.2016.07.012
Alarcon-Chaidez, F., Sampath, J., Srinivas, P., and Vijayakumar, M. N. (1997). TN5252: a model for complex streptococcal conjugative transposons. Adv. Exp. Med. Biol. 418, 1029–1032. doi: 10.1007/978-1-4899-1825-3_242
Ammerlaan, H. S. M., Harbarth, S., Buiting, A. G. M., Crook, D. W., Fitzpatrick, F., Hanberger, H., et al. (2013). Secular trends in nosocomial bloodstream infections: antibiotic-resistant bacteria increase the total burden of infection. Clin. Infect. Dis. 56, 798–805. doi: 10.1093/cid/cis1006
Aslam, M., Diarra, M. S., Checkley, S., Bohaychuk, V., and Masson, L. (2012). Characterization of antimicrobial resistance and virulence genes in Enterococcus spp. isolated from retail meats in Alberta, Canada. Int. J. Food Microbiol. 156, 222–230. doi: 10.1016/j.ijfoodmicro.2012.03.026
Bankevich, A., Nurk, S., Antipov, D., Gurevich, A. A., Dvorkin, M., Kulikov, A. S., et al. (2012). SPAdes: a new genome assembly algorithm and its applications to single-cell sequencing. J. Comput. Biol. 19, 455–477. doi: 10.1089/cmb.2012.0021
Barros, R. D., Vieira, S. L., Favero, A., Taschetto, D., Mascarello, N. C., and Cemin, H. S. (2012). Reassessing flavophospholipol effects on broiler performance. Rev. Bras. Zoot. 41, 2458–2462. doi: 10.1590/S1516-35982012001200011
Benson, D. A., Cavanaugh, M., Clark, K., Karsch-Mizrachi, I., Lipman, D. J., Ostell, J., et al. (2013). GenBank. Nucleic Acids Res. 41, D36–D42. doi: 10.1093/nar/gks1195
Bertels, F., Silander, O. K., Pachkov, M., Rainey, P. B., and Van Nimwegen, E. (2014). Automated reconstruction of whole-genome phylogenies from short-sequence reads. Mol. Biol. Evol. 31, 1077–1088. doi: 10.1093/molbev/msu088
Beukers, A. G., Zaheer, R., Goji, N., Amoako, K. K., Chaves, A. V., Ward, M. P., et al. (2017). Comparative genomics of Enterococcus spp. isolated from bovine feces. BMC Microbiol 17:52. doi: 10.1186/s12866-017-0962-1
Bi, D., Xu, Z., Harrison, E. M., Tai, C., Wei, Y., He, X., et al. (2012). ICEberg: a web-based resource for integrative and conjugative elements found in Bacteria. Nucleic Acids Res. 40, D621–D626. doi: 10.1093/nar/gkr846
Billington, E. O., Phang, S. H., Gregson, D. B., Pitout, J. D., Ross, T., Church, D. L., et al. (2014). Incidence, risk factors, and outcomes for Enterococcus spp. blood stream infections: a population-based study. Int. J. Infect. Dis. 26, 76–82. doi: 10.1016/j.ijid.2014.02.012
Boehm, A. B., and Sassoubre, L. M. (2014). “Enterococci as indicators of environmental fecal contamination,” in Enterococci: From Commensals to Leading Causes of Drug Resistant Infection [Internet], eds M. S. Gilmore, D. B. Clewell, Y. Ike and N. Shankar (Boston, MA: Massachusetts Eye and Ear Infirmary).
Bortolaia, V., and Guardabassi, L. (2015). “Zoonotic transmission of antimicrobial resistant enterococci: a threat to public health or an overemphasised risk?” in Zoonoses - Infections Affecting Humans and Animals: Focus on Public Health Aspects, ed A. Sing (Dordrecht: Springer Netherlands), 407–431.
Braga, J. F. V., Leal, C. a .G , Silva, C. C., Fernandes, A. A., Martins, N., et al. (2018). Genetic diversity and antimicrobial resistance profile of Enterococcus faecalis isolated from broilers with vertebral osteomyelitis in Southeast Brazil. Avian Pathol. 47, 14–22. doi: 10.1080/03079457.2017.1359403
Butaye, P., Devriese, L. A., and Haesebrouck, F. (2001). Differences in antibiotic resistance patterns of Enterococcus faecalis and Enterococcus faecium strains isolated from farm and pet animals. Antimicrob. Agents Chemother. 45, 1374–1378. doi: 10.1128/AAC.45.5.1374-1378.2001
Byappanahalli, M. N., Nevers, M. B., Korajkic, A., Staley, Z. R., and Harwood, V. J. (2012). Enterococci in the environment. Microbiol. Mol. Biol. Rev. 76, 685–706. doi: 10.1128/MMBR.00023-12
Carattoli, A., Zankari, E., Garcia-Fernandez, A., Voldby Larsen, M., Lund, O., Villa, L., et al. (2014). In silico detection and typing of plasmids using PlasmidFinder and plasmid multilocus sequence typing. Antimicrob. Agents Chemother. 58, 3895–3903. doi: 10.1128/AAC.02412-14
Cauwerts, K., Decostere, A., De Graef, E. M., Haesebrouck, F., and Pasmans, F. (2007). High prevalence of tetracycline resistance in Enterococcus isolates from broilers carrying the erm(B) gene. Avian Pathol. 36, 395–399. doi: 10.1080/03079450701589167
Champagne, J., Diarra, M. S., Rempel, H., Topp, E., Greer, C. W., Harel, J., et al. (2011). Development of a DNA microarray for enterococcal species, virulence, and antibiotic resistance gene determinations among isolates from poultry. Appl. Environ. Microbiol. 77, 2625–2633. doi: 10.1128/AEM.00263-11
Chin, C. S., Alexander, D. H., Marks, P., Klammer, A. A., Drake, J., Heiner, C., et al. (2013). Nonhybrid, finished microbial genome assemblies from long-read SMRT sequencing data. Nat. Methods 10, 563–569. doi: 10.1038/nmeth.2474
Cipars (2008). Canadian Integrated Program for Antimicrobial Resistance Surveillance Annual Report. Ottawa: Health Canada. Available online at: https://www.canada.ca/en/public-health/services/surveillance/canadian-integrated-program-antimicrobial-resistance-surveillance-cipars/cipars-2008-annual-report.html
CLSI (2015). Performance Standards for Antimicrobial Susceptibility Testing. Document M100-S25; Twenty-fifth informational supplement. Wayne, PA: Clinical and Laboratory Standards Institute.
Davis, J. J., Boisvert, S., Brettin, T., Kenyon, R. W., Mao, C., Olson, R., et al. (2016). Antimicrobial resistance prediction in PATRIC and RAST. Sci. Rep. 6:27930. doi: 10.1038/srep27930
De Kraker, M. E., Jarlier, V., Monen, J. C., Heuer, O. E., Van De Sande, N., and Grundmann, H. (2013). The changing epidemiology of bacteraemias in Europe: trends from the European Antimicrobial Resistance Surveillance System. Clin. Microbiol. Infect. 19, 860–868. doi: 10.1111/1469-0691.12028
Devriese, L. A., Hommez, J., Wijfels, R., and Haesebrouck, F. (1991). Composition of the enterococcal and streptococcal intestinal flora of poultry. J. Appl. Bacteriol. 71, 46–50. doi: 10.1111/j.1365-2672.1991.tb04585.x
Diarra, M. S., and Malouin, F. (2014). Antibiotics in Canadian poultry productions and anticipated alternatives. Front. Microbiol. 5:282. doi: 10.3389/fmicb.2014.00282
Diarra, M. S., Rempel, H., Champagne, J., Masson, L., Pritchard, J., and Topp, E. (2010). Distribution of antimicrobial resistance and virulence genes in Enterococcus spp. and characterization of isolates from broiler chickens. Appl. Environ. Microbiol. 76, 8033–8043. doi: 10.1128/AEM.01545-10
Diarra, M. S., Silversides, F. G., Diarrassouba, F., Pritchard, J., Masson, L., Brousseau, R., et al. (2007). Impact of feed supplementation with antimicrobial agents on growth performance of broiler chickens, Clostridium perfringens and enterococcus counts, and antibiotic resistance phenotypes and distribution of antimicrobial resistance determinants in Escherichia coli isolates. Appl. Environ. Microbiol. 73, 6566–6576. doi: 10.1128/AEM.01086-07
Dressler, C., Kues, U., Nies, D. H., and Friedrich, B. (1991). Determinants encoding resistance to several heavy metals in newly isolated copper-resistant bacteria. Appl. Environ. Microbiol. 57, 3079–3085.
Dubin, K., and Pamer, E. G. (2017). Enterococci and their interactions with the intestinal microbiome. Microbiol. Spectr. 5:BAD-0014-2016. doi: 10.1128/microbiolspec.BAD-0014-2016
ECDC (2011). European Centre for Disease Prevention and Control Publishes Annual Epidemiological Report 2011. Eurosurveillance 16, 20012.
Eliopoulos, G. M., Meka, V. G., and Gold, H. S. (2004). Antimicrobial Resistance to Linezolid. Clin. Infect. Dis. 39, 1010–1015. doi: 10.1086/423841
Fisher, K., and Phillips, C. (2009). The ecology, epidemiology and virulence of Enterococcus. Microbiology 155, 1749–1757. doi: 10.1099/mic.0.026385-0
Geldart, K., and Kaznessis, Y. N. (2017). Characterization of class IIa bacteriocins resistance in Enterococcus faecium. Antimicrob. Agents Chemother. 61:e02033-16. doi: 10.1128/AAC.02033-16
Gilmore, M. S. (2002). The Enterococci : Pathogenesis, Molecular Biology, and Antibiotic Resistance. Washington, DC: ASM Press. doi: 10.1128/9781555817923
Golinska, E., Tomusiak, A., Gosiewski, T., Wiecek, G., Machul, A., Mikolajczyk, D., et al. (2013). Virulence factors of Enterococcus strains isolated from patients with inflammatory bowel disease. World J. Gastroenterol. 19, 3562–3572. doi: 10.3748/wjg.v19.i23.3562
Gong, J., Forster, R. J., Yu, H., Chambers, J. R., Wheatcroft, R., Sabour, P. M., et al. (2002). Molecular analysis of bacterial populations in the ileum of broiler chickens and comparison with bacteria in the cecum. FEMS Microbiol. Ecol. 41, 171–179. doi: 10.1111/j.1574-6941.2002.tb00978.x
Grant, J. R., Arantes, A. S., and Stothard, P. (2012). Comparing thousands of circular genomes using the CGView Comparison Tool. BMC Genomics 13:202. doi: 10.1186/1471-2164-13-202
Grohmann, E., Muth, G., and Espinosa, M. (2003). Conjugative plasmid transfer in gram-positive bacteria. Microbiol. Mol. Biol. Rev. 67, 277–301. doi: 10.1128/MMBR.67.2.277-301.2003
Hallgren, A., Claesson, C., Saeedi, B., Monstein, H. J., Hanberger, H., and Nilsson, L. E. (2009). Molecular detection of aggregation substance, enterococcal surface protein, and cytolysin genes and in vitro adhesion to urinary catheters of Enterococcus faecalis and E. faecium of clinical origin. Int. J. Med. Microbiol. 299, 323–332. doi: 10.1016/j.ijmm.2008.10.001
Hancock, L. E., and Perego, M. (2004). The Enterococcus faecalis fsr two-component system controls biofilm development through production of gelatinase. J. Bacteriol. 186, 5629–5639. doi: 10.1128/JB.186.17.5629-5639.2004
Hayes, J. R., English, L. L., Carr, L. E., Wagner, D. D., and Joseph, S. W. (2004). Multiple-antibiotic resistance of Enterococcus spp. isolated from commercial poultry production environments. Appl. Environ. Microbiol. 70, 6005–6011. doi: 10.1128/AEM.70.10.6005-6011.2004
He, Q., Hou, Q., Wang, Y., Li, J., Li, W., Kwok, L. Y., et al. (2018). Comparative genomic analysis of Enterococcus faecalis: insights into their environmental adaptations. BMC Genomics 19:527. doi: 10.1186/s12864-018-4887-3
Hegstad, K., Mikalsen, T., Coque, T. M., Werner, G., and Sundsfjord, A. (2010). Mobile genetic elements and their contribution to the emergence of antimicrobial resistant Enterococcus faecalis and Enterococcus faecium. Clin. Microbiol. Infect. 16, 541–554. doi: 10.1111/j.1469-0691.2010.03226.x
Hirt, H., Greenwood-Quaintance, K. E., Karau, M. J., Till, L. M., Kashyap, P. C., Patel, R., et al. (2018). Enterococcus faecalis sex pheromone cCF10 enhances conjugative plasmid transfer in vivo. MBio 9:e00037-18. doi: 10.1128/mBio.00037-18
Hollenbeck, B. L., and Rice, L. B. (2012). Intrinsic and acquired resistance mechanisms in enterococcus. Virulence 3, 421–433. doi: 10.4161/viru.21282
Ike, Y. (2017). Pathogenicity of Enterococci. Nippon. Saikingaku Zasshi 72, 189–211. doi: 10.3412/jsb.72.189
Jaglic, Z., Vlkova, H., Bardon, J., Michu, E., Cervinkova, D., and Babak, V. (2012). Distribution, characterization and genetic bases of erythromycin resistance in staphylococci and enterococci originating from livestock. Zoonoses Public Health 59, 202–211. doi: 10.1111/j.1863-2378.2011.01434.x
Jett, B. D., Huycke, M. M., and Gilmore, M. S. (1994). Virulence of enterococci. Clin. Microbiol. Rev. 7, 462–478. doi: 10.1128/CMR.7.4.462
Jeukens, J., Kukavica-Ibrulj, I., Emond-Rheault, J. G., Freschi, L., and Levesque, R. C. (2017). Comparative genomics of a drug-resistant Pseudomonas aeruginosa panel and the challenges of antimicrobial resistance prediction from genomes. FEMS Microbiol. Lett. 364:fnx161. doi: 10.1093/femsle/fnx161
Joensen, K. G., Scheutz, F., Lund, O., Hasman, H., Kaas, R. S., Nielsen, E. M., et al. (2014). Real-time whole-genome sequencing for routine typing, surveillance, and outbreak detection of verotoxigenic Escherichia coli. J. Clin. Microbiol. 52, 1501–1510. doi: 10.1128/JCM.03617-13
Jolley, K. A., Bliss, C. M., Bennett, J. S., Bratcher, H. B., Brehony, C., Colles, F. M., et al. (2012). Ribosomal multilocus sequence typing: universal characterization of bacteria from domain to strain. Microbiology 158, 1005–1015. doi: 10.1099/mic.0.055459-0
Kaukas, A., Hinton, M., and Linton, A. H. (1986). Changes in the faecal enterococcal population of young chickens and its effect on the incidence of resistance to certain antibiotics. Lett. Appl. Microbiol. 2, 5–8. doi: 10.1111/j.1472-765X.1986.tb01503.x
Klibi, N., Sáenz, Y., Zarazaga, M., Ben Slama, K., Masmoudi, A., Ruiz-Larrea, F., et al. (2008). Polymorphism in pbp5 gene detected in clinical Enterococcus faecium strains with different ampicillin MICs from a Tunisian Hospital. J. Chemother. 20, 436–440. doi: 10.1179/joc.2008.20.4.436
Larsen, M. V., Cosentino, S., Rasmussen, S., Friis, C., Hasman, H., Marvig, R. L., et al. (2012). Multilocus sequence typing of total-genome-sequenced bacteria. J. Clin. Microbiol. 50, 1355–1361. doi: 10.1128/JCM.06094-11
Ligozzi, M., Pittaluga, F., and Fontana, R. (1996). Modification of penicillin-binding protein 5 associated with high-level ampicillin resistance in Enterococcus faecium. Antimicrob. Agents Chemother. 40, 354–357. doi: 10.1128/AAC.40.2.354
Macesic, N., Polubriaginof, F., and Tatonetti, N. P. (2017). Machine learning: novel bioinformatics approaches for combating antimicrobial resistance. Curr. Opin. Infect. Dis. 30, 511–517. doi: 10.1097/QCO.0000000000000406
Magoc, T., and Salzberg, S. L. (2011). FLASH: fast length adjustment of short reads to improve genome assemblies. Bioinformatics 27, 2957–2963. doi: 10.1093/bioinformatics/btr507
Marshall, B. M., and Levy, S. B. (2011). Food animals and antimicrobials: impacts on human health. Clin. Microbiol. Rev. 24, 718–733. doi: 10.1128/CMR.00002-11
Mason, A., Foster, D., Bradley, P., Golubchik, T., Doumith, M., Gordon, N. C., et al. (2018). Accuracy of different bioinformatics methods in detecting antibiotic resistance and virulence factors from Staphylococcus aureus whole genome sequences. J. Clin. Microbiol. 56:e01815-17. doi: 10.1128/JCM.01815-17
McArthur, A. G., Waglechner, N., Nizam, F., Yan, A., Azad, M. A., Baylay, A. J., et al. (2013). The comprehensive antibiotic resistance database. Antimicrob. Agents Chemother. 57, 3348–3357. doi: 10.1128/AAC.00419-13
McDermott, P. F., Tyson, G. H., Kabera, C., Chen, Y., Li, C., Folster, J. P., et al. (2016). Whole-genome sequencing for detecting antimicrobial resistance in nontyphoidal salmonella. Antimicrob. Agents Chemother. 60, 5515–5520. doi: 10.1128/AAC.01030-16
Miller, W. R., Munita, J. M., and Arias, C. A. (2014). Mechanisms of antibiotic resistance in enterococci. Expert Rev. Anti Infect. Ther. 12, 1221–1236. doi: 10.1586/14787210.2014.956092
Neuert, S., Nair, S., Day, M. R., Doumith, M., Ashton, P. M., Mellor, K. C., et al. (2018). Prediction of phenotypic antimicrobial resistance profiles from whole genome sequences of non-typhoidal Salmonella enterica. Front. Microbiol. 9:592. doi: 10.3389/fmicb.2018.00592
Nhung, N. T., Cuong, N. V., Thwaites, G., and Carrique-Mas, J. (2016). Antimicrobial usage and antimicrobial resistance in animal production in Southeast Asia: a review. Antibiotics 5:e37. doi: 10.3390/antibiotics5040037
Petkau, A., Mabon, P., Sieffert, C., Knox, N. C., Cabral, J., Iskander, M., et al. (2017). SNVPhyl: a single nucleotide variant phylogenomics pipeline for microbial genomic epidemiology. Microb. Genom. 3:e000116. doi: 10.1099/mgen.0.000116
Pightling, A. W., Pettengill, J. B., Luo, Y., Baugher, J. D., Rand, H., and Strain, E. (2018). Interpreting whole-genome sequence analyses of foodborne bacteria for regulatory applications and outbreak investigations. Front. Microbiol. 9:1482. doi: 10.3389/fmicb.2018.01482
Pillay, S., Zishiri, O. T., and Adeleke, M. A. (2018). Prevalence of virulence genes in Enterococcus species isolated from companion animals and livestock. Onderstepoort J. Vet. Res. 85, e1–e8. doi: 10.4102/ojvr.v85i1.1583
Pinholt, M., Larner-Svensson, H., Littauer, P., Moser, C. E., Pedersen, M., Lemming, L. E., et al. (2015). Multiple hospital outbreaks of vanA Enterococcus faecium in Denmark, 2012–13, investigated by WGS, MLST and PFGE. J. Antimicrob. Chemother. 70, 2474–2482. doi: 10.1093/jac/dkv142
Poulsen, L. L., Bisgaard, M., Son, N. T., Trung, N. V., An, H. M., and Dalsgaard, A. (2012). Enterococcus faecalis clones in poultry and in humans with urinary tract infections, Vietnam. Emerging Infect. Dis. 18, 1096–1100. doi: 10.3201/eid1807.111754
Rehman, M. A., Yin, X., Lepp, D., Laing, C., Ziebell, K., Talbot, G., et al. (2017). Genomic analysis of third generation cephalosporin resistant Escherichia coli from dairy cow manure. Vet. Sci. 4:e57. doi: 10.3390/vetsci4040057
Reid, K. C., Cockerill, I. F., and Patel, R. (2001). Clinical and epidemiological features of Enterococcus casseliflavus/flavescens and Enterococcus gallinarum bacteremia: a report of 20 cases. Clin. Infect. Dis. 32, 1540–1546. doi: 10.1086/320542
Rice, L. B., Carias, L. L., Hutton-Thomas, R., Sifaoui, F., Gutmann, L., and Rudin, S. D. (2001). Penicillin-binding protein 5 and expression of ampicillin resistance in Enterococcus faecium. Antimicrob. Agents Chemother. 45, 1480–1486. doi: 10.1128/AAC.45.5.1480-1486.2001
Roberts, A. P., and Mullany, P. (2009). A modular master on the move: the Tn916 family of mobile genetic elements. Trends Microbiol. 17, 251–258. doi: 10.1016/j.tim.2009.03.002
Salipante, S. J., Sengupta, D. J., Cummings, L. A., Land, T. A., Hoogestraat, D. R., and Cookson, B. T. (2015). Application of whole-genome sequencing for bacterial strain typing in molecular epidemiology. J. Clin. Microbiol. 53, 1072–1079. doi: 10.1128/JCM.03385-14
Seemann, T. (2014). Prokka: rapid prokaryotic genome annotation. Bioinformatics 30, 2068–2069. doi: 10.1093/bioinformatics/btu153
Sekse, C., Holst-Jensen, A., Dobrindt, U., Johannessen, G. S., Li, W., Spilsberg, B., et al. (2017). High throughput sequencing for detection of foodborne pathogens. Front. Microbiol. 8:2029. doi: 10.3389/fmicb.2017.02029
Semedo, T., Santos, M. A., Lopes, M. F., Figueiredo Marques, J. J., Barreto Crespo, M. T., and Tenreiro, R. (2003). Virulence factors in food, clinical and reference Enterococci: a common trait in the genus? Syst. Appl. Microbiol. 26, 13–22. doi: 10.1078/072320203322337263
Simonsen, G. S., Haaheim, H., Dahl, K. H., Kruse, H., Lovseth, A., Olsvik, O., et al. (1998). Transmission of VanA-type vancomycin-resistant enterococci and vanA resistance elements between chicken and humans at avoparcin-exposed farms. Microb. Drug Resist. 4, 313–318. doi: 10.1089/mdr.1998.4.313
Stapleton, P. D., and Taylor, P. W. (2002). Methicillin resistance in Staphylococcus aureus: mechanisms and modulation. Sci. Prog. 85, 57–72. doi: 10.3184/003685002783238870
Tankson, J. D., Thaxton, J. P., and Vizzier-Thaxton, Y. (2001). Pulmonary hypertension syndrome in broilers caused by Enterococcus faecalis. Infect. Immun. 69, 6318–6322. doi: 10.1128/IAI.69.10.6318-6322.2001
Tankson, J. D., Thaxton, J. P., and Vizzier-Thaxton, Y. (2002). Morphological changes in heart and lungs of broilers experiencing pulmonary hypertension syndrome caused by Enterococcus faecalis. Poult. Sci. 81, 365–370. doi: 10.1093/ps/81.3.365
Torres, C., Alonso, C. A., Ruiz-Ripa, L., León-Sampedro, R., Del Campo, R., and Coque, T. M. (2018). Antimicrobial resistance in Enterococcus spp. of animal origin. Microbiol. Spectr. 6:ARBA-0032-2018. doi: 10.1128/microbiolspec.ARBA-0032-2018
Tremblay, C. L., Letellier, A., Quessy, S., Boulianne, M., Daignault, D., and Archambault, M. (2011). Multiple-antibiotic resistance of Enterococcus faecalis and Enterococcus faecium from cecal contents in broiler chicken and turkey flocks slaughtered in Canada and plasmid colocalization of tetO and ermB genes. J. Food Prot. 74, 1639–1648. doi: 10.4315/0362-028X.JFP-10-451
Tyson, G. H., Mcdermott, P. F., Li, C., Chen, Y., Tadesse, D. A., Mukherjee, S., et al. (2015). WGS accurately predicts antimicrobial resistance in Escherichia coli. J. Antimicrob. Chemother. 70, 2763–2769. doi: 10.1093/jac/dkv186
Tyson, G. H., Nyirabahizi, E., Crarey, E., Kabera, C., Lam, C., Rice-Trujillo, C., et al. (2018a). Prevalence and antimicrobial resistance of enterococci isolated from retail meats in the United States, 2002 to 2014. Appl. Environ. Microbiol. 84:e01902-17. doi: 10.1128/AEM.01902-17
Tyson, G. H., Sabo, J. L., Rice-Trujillo, C., Hernandez, J., and Mcdermott, P. F. (2018b). Whole-genome sequencing based characterization of antimicrobial resistance in Enterococcus. Pathog. Dis. 76:fty018. doi: 10.1093/femspd/fty018
Weisblum, B. (1985). Inducible resistance to macrolides, lincosamides and streptogramin type B antibiotics: the resistance phenotype, its biological diversity, and structural elements that regulate expression–a review. J. Antimicrob. Chemother. 16(Suppl A), 63–90. doi: 10.1093/jac/16.suppl_A.63
Werner, G., Coque, T. M., Hammerum, A. M., Hope, R., Hryniewicz, W., Johnson, A., et al. (2008). Emergence and spread of vancomycin resistance among enterococci in Europe. Euro Surveill. 13:19046. Available online at: http://www.eurosurveillance.org
Wozniak, R. A., and Waldor, M. K. (2010). Integrative and conjugative elements: mosaic mobile genetic elements enabling dynamic lateral gene flow. Nat. Rev. Microbiol. 8, 552–563. doi: 10.1038/nrmicro2382
Yoshimura, H., Ishimaru, M., Endoh, Y. S., and Kojima, A. (2000). Antimicrobial susceptibilities of enterococci isolated from faeces of broiler and layer chickens. Lett. Appl. Microbiol. 31, 427–432. doi: 10.1046/j.1365-2672.2000.00842.x
Yost, C. K., Diarra, M. S., and Topp, E. (2011). “Animals and humans as sources of fecal indicator bacteria,” in The Fecal Bacteria, eds M. Sadowsky and R. Whitman (Washington, DC: Amercan Society for Microbiology Press), 67–91.
Zankari, E., Hasman, H., Cosentino, S., Vestergaard, M., Rasmussen, S., Lund, O., et al. (2012). Identification of acquired antimicrobial resistance genes. J. Antimicrob. Chemother. 67, 2640–2644. doi: 10.1093/jac/dks261
Keywords: enterococci, broiler chickens, antimicrobial resistance (AMR), whole genome sequencing (WGS), AMR genotypes, AMR phenotypes
Citation: Rehman MA, Yin X, Zaheer R, Goji N, Amoako KK, McAllister T, Pritchard J, Topp E and Diarra MS (2018) Genotypes and Phenotypes of Enterococci Isolated From Broiler Chickens. Front. Sustain. Food Syst. 2:83. doi: 10.3389/fsufs.2018.00083
Received: 24 August 2018; Accepted: 20 November 2018;
Published: 13 December 2018.
Edited by:
Stephen Forsythe, Foodmicrobe.com, United KingdomReviewed by:
Juan Wang, South China Agricultural University, ChinaCangliang Shen, West Virginia University, United States
Copyright © 2018 Rehman, Yin, Zaheer, Goji, Amoako, McAllister, Pritchard, Topp and Diarra. This is an open-access article distributed under the terms of the Creative Commons Attribution License (CC BY). The use, distribution or reproduction in other forums is permitted, provided the original author(s) and the copyright owner(s) are credited and that the original publication in this journal is cited, in accordance with accepted academic practice. No use, distribution or reproduction is permitted which does not comply with these terms.
*Correspondence: Moussa S. Diarra, bW91c3NhLmRpYXJyYUBjYW5hZGEuY2E=