- 1Environmental Systems Graduate Group, University of California Merced, Merced, CA, United States
- 2Stillwater Sciences, Berkeley, CA, United States
Harmful algal blooms (HABs) degrade water quality through the production of potent toxins that pose critical management challenges, especially in water-scarce regions. This study examined how dissolved oxygen (DO) conditions at the sediment–water interface in reservoirs in Southern California, United States, affect internal nutrient loading, which can promote HABs through the release of nutrients and metals from sediment to overlaying water. Replicate 2-liter laboratory chamber experiments with minimally disturbed sediment and bottom water from shallow, hypereutrophic Lake Henshaw and moderately deep, meso-eutrophic Lake Wohlford were conducted at ~25°C for ~30 days under oxic (~ 8 mg/L DO), hypoxic (< 2 mg/L DO), and anoxic (0 mg/L DO) conditions. Anoxic conditions in Lake Henshaw chambers resulted in elevated fluxes of ammonia (366 mg-N/m2·d) and soluble reactive phosphorus (122 mg-P/m2·d) from sediment, while hypoxic conditions promoted the flux of manganese (14.9 mg/m2·d), iron (3.3 mg/m2·d), and some SRP (44 mg-P/m2·d). In Lake Wohlford, anoxic conditions resulted in sediment release of ammonia (82 mg-N/m2·d), soluble reactive phosphorus (67 mg-P/m2·d), manganese (24 mg/m2·d) and iron (1.9 mg/m2·d). Under oxic and hypoxic conditions, Lake Henshaw sediments exhibited some release of ammonia and soluble reactive phosphorus, while Lake Wohlford sediments exhibited negligible release. After adjusting for temperature, Lake Wohlford anoxic fluxes of ammonia and soluble reactive phosphorus appear consistent with other eutrophic systems (4–60 mg-N/m2⋅d and 10–53 mg-P/m2⋅d), while Lake Henshaw anoxic fluxes appear rather extreme. Results from Lake Henshaw suggest that different nitrogen to phosphorus ratios were released from sediment to overlaying water under hypoxia (very low ratio plus iron) versus anoxia (low ratio with high nutrient levels). This in turn could affect phytoplankton community composition and toxin production. Results are being used to help inform management strategies, such as dissolved oxygen or sediment amendments, to control internal nutrient loading and reduce HAB toxin production.
1 Introduction
The cycling of nutrients in lakes and reservoirs is important to assess, particularly since excess nutrients can trigger harmful algal blooms (HABs). Sediments may release nitrogen (N) and phosphorus (P), which are common limiting nutrients, as well as the key micronutrient iron (Fe), to overlying water through a process called internal nutrient loading (Zamparas and Zacharias, 2014; Welch and Dennis Cooke, 2005). Internal nutrient loading can stimulate phytoplankton growth and act as a positive feedback, as anoxic conditions, brought on by microbial decay of organic carbon in senesced phytoplankton, favor nutrient release from sediment which exacerbates phytoplankton growth and decay, and in turn stimulates anoxic conditions and enhances internal nutrient loading. HABs may occur naturally in fertile regions of the world with high background nutrient availability, but their increasing presence in a variety of lakes and reservoirs is linked to degradation of water bodies by human additions of N and P (Watson et al., 2015). Climate change will likely exacerbate HAB occurrence due to warming temperatures that favor the growth of cyanobacteria over other phytoplankton and increased rates of microbial activity that promotes sediment nutrient release to overlying water (Havens and Pearl, 2015). Understanding nutrient cycling in reservoirs is key to developing management strategies to promote climate-resilient water systems and maintain the integrity of water supplies (Sprague and Prenger-Berninghoff, 2019).
The dissolved oxygen (DO) content of water at the sediment–water interface is a key controller of nutrient and metal cycling in aquatic sediment (Liu et al., 2023; Osaka et al., 2021; Dadi et al., 2020, 2023). Under anoxic conditions, ammonium (NH4+) is one of the first compounds to be released (Beutel, 2006). This release of ammonium is a key component of internal nutrient loading and the eutrophication of N-limited and co-limited systems (Paerl, 2009; Conley et al., 2009). The oxidation of ammonia to nitrate can also exert an additional oxygen burden in aquatic systems, further exacerbating low-DO conditions. As oxidation–reduction (redox) potential continues to decline at the anoxic sediment–water interface, dissolved manganese (Mn), as Mn(II), and dissolved Fe, as Fe(II), are released into overlying water via microbial and chemical reductive dissolution of metal oxides (Davison, 1993). The reductive dissolution of Fe oxides also liberates phosphate (PO43−) from the sediment and contributes to an increase in P within the water column (Nünberg, 1994; Nürnberg, 1985). In eutrophic systems, mineralization of organic matter can also lead to sediment release of phosphate even under oxic conditions (Jensen and Andersen, 1992), but sediment P release rates are commonly higher under anoxic versus oxic conditions (Beutel, 2006; Osaka et al., 2021). Under highly reduced conditions, microbial sulfate (SO42−) reduction can lead to sulfide accumulation in bottom waters (Beutel et al., 2020), with the subsequent loss of Fe(II) from the water column via FeS precipitation (Balistrieri et al., 1992). The combined release of ammonia, phosphate, and Fe can stimulate HABs, which can produce potent toxins, such as microcsytin and anatoxin, that render water unsuitable for human consumption (Falconer and Humpage, 2005) and for recreational contact (Backer et al., 2010; Brooks et al., 2015). The release of reduced metals from the sediment also creates challenges for potable water management as Fe and Mn degrade water treatability and cause taste and odor issues (Khadse et al., 2015; Betancourt et al., 2010). Maintaining a consistent and safe water supply is particularly important for water resilience efforts in water-scarce regions like Southern California, USA, the focus of this study.
This study examined patterns of internal nutrient loading through assessment of nutrient and metal releases to water in experimental bench-top sediment-water chambers under different DO concentrations. The experiment was conducted using the traditional oxic and anoxic phases of typical chamber experiments, as well as a less studied hypoxic phase (DO <2 mg/L), which can show interesting and potentially ecologically important patterns of N and P cycling (Doig et al., 2016; Osaka et al., 2021). These findings suggest that hypoxia is an important condition that affects nutrient loading. Hypoxia is especially important to consider in shallow reservoirs because of the potential for water-column oscillations in DO concentration due to changing patterns of phytoplankton photosynthesis, microbial and algal respiration, and wind mixing (D’Autilia et al., 2004; Langman et al., 2010).
In this study, sites include a large, shallow, hypereutrophic reservoir (Lake Henshaw) and a downstream smaller, moderately deep, mesotrophic to eutrophic reservoir (Lake Wohlford), which serve as raw water sources for potable use and downstream shallow groundwater recharge, recreational contact uses (swimming, boating, fishing), and tribal cultural uses in Southern California. Both reservoirs are impacted by toxins produced by HABs that are stimulated, in part, by internal nutrient and metals loading, and are connected by the Escondido Canal. The objectives of this study were to, in the two contrasting study sites, evaluate: (1) the magnitude and spatial patterns of anoxic sediment release rates of nutrients and metals to overlaying water, and (2) the effects of DO conditions, including hypoxic conditions (DO <2 mg/L), on sediment nutrient and metals release rates. This paper is part of a larger study to evaluate options aimed at repressing HAB toxin production via potential short- and long-term in-lake management strategies such as algaecide application, sediment treatment (e.g., alum, lanthanum), source water control, and oxygenation (Stillwater Sciences, 2022).
2 Materials and methods
2.1 Study sites
Lake Henshaw (−116.7490275 W 33.2392639 N) and Lake Wohlford (−116.9978056 W 33.1715902 N) are raw water reservoirs in San Diego County, California, United States, on traditional homelands of the Cupeño, Kumeyaay, and Payómkawichum people (Figure 1). Lake Henshaw has a surface area at full pool of 915 ha (2,260 acres) with a maximum capacity of 64.1 million m3 (52,000 acre-feet), however the reservoir is rarely at maximum capacity (Figure 2A). Surface area has varied between 200–740 ha (490–1,820 acres) and capacity between 2.4–39.5 million m3 (1,910–32,030 acre-feet) from 2016 through 2023. The typical mean depth ranges from 2.4–6.0 m. Lake Wohlford has a maximum surface area of 91 ha (224 acres) with a potential capacity of 8.0 million m3 (6,460 acre-feet) (Figure 2B). The reservoir currently operates at a maximum surface area of 59 ha (145 acres) and a capacity of 3.4 million m3 (2,780 acre-feet) due to seismic concerns with the dam. Under the seismic restrictions, the maximum depth is ~16.0 m and the mean depth ~ 5.8 m.
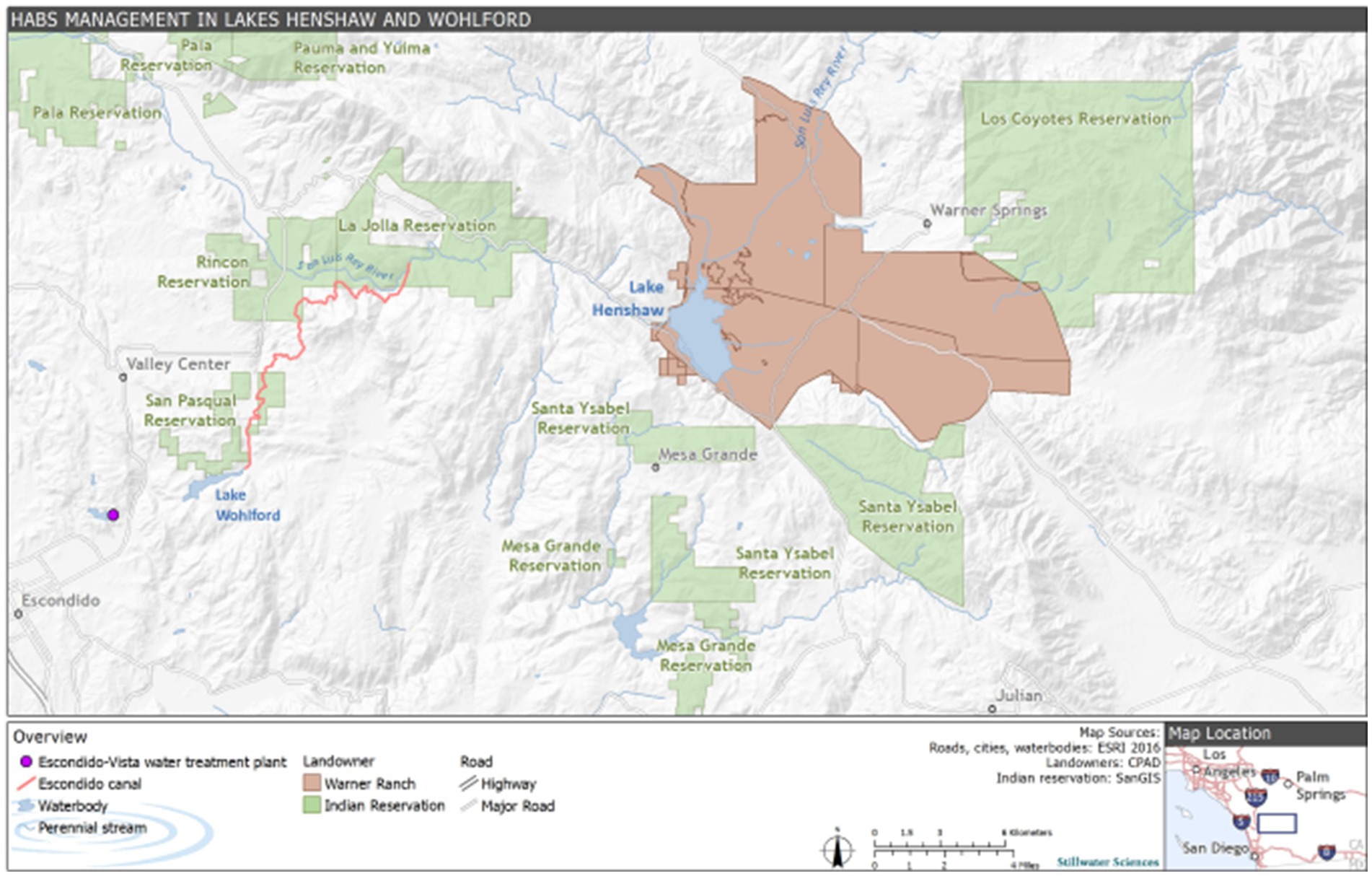
Figure 1. Site description map including Lake Henshaw and Lake Wohlford in the context of local indigenous tribes and the Escondido-Vista water treatment plant.
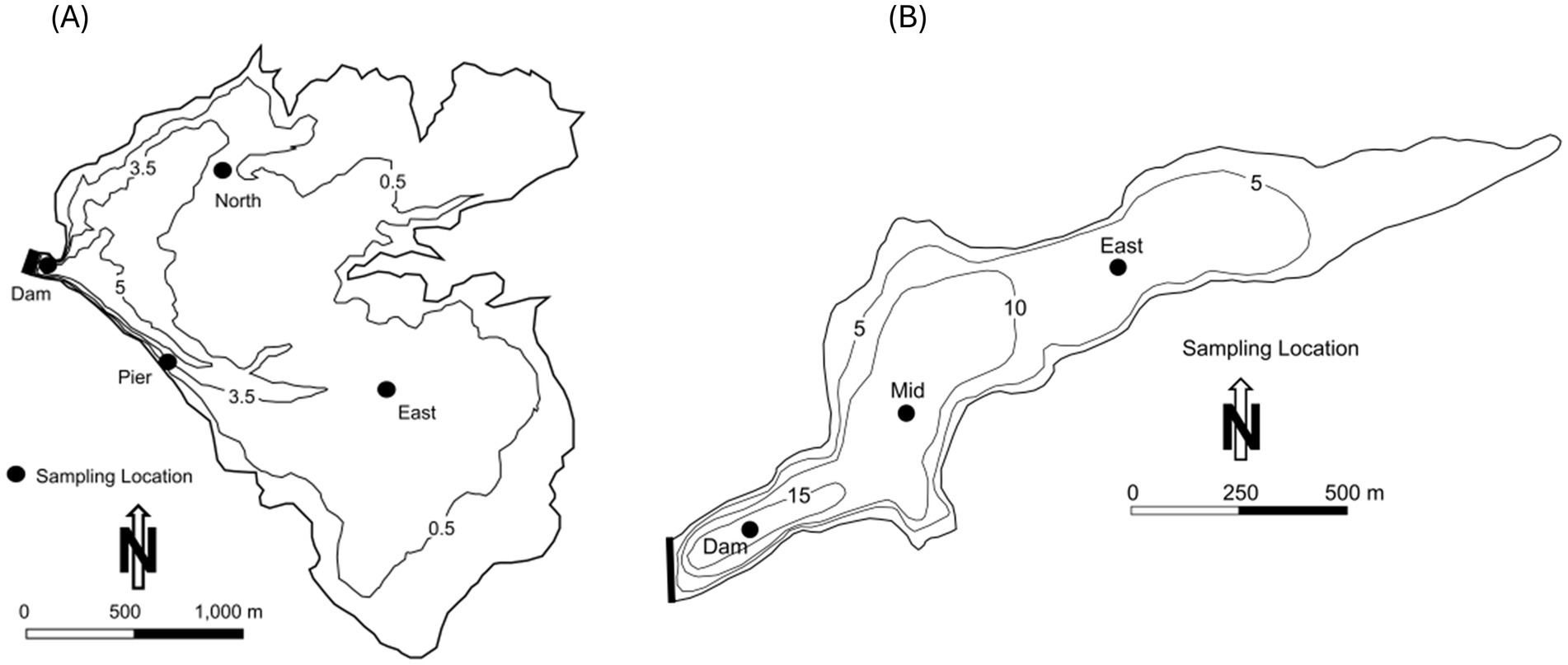
Figure 2. Bathymetry map and sampling locations for Lake Henshaw and Lake Wohlford. Depth contours are in meters (m). (A) Bathymetry map for Lake Henshaw for surface elevation of ~812.5 m (B) Bathymetry map for Lake Wohlford for surface elevation of ~445.1 m.
Water from Lake Henshaw flows via the 19.5 km Escondido Canal to Lake Wohlford, which provides water for over 250,000 people through the City of Escondido and Vista Irrigation District. Both reservoirs are important to local recreation and fishing, and Lake Henshaw has particular importance as a source of water to the San Luis Rey River for recreational and tribal cultural uses by the La Jolla Band of Luiseño Indians. Releases from Lake Henshaw also recharge groundwater underlying the downstream Rincon, Pauma, and Pala Reservations and provide water used for agricultural, domestic, and business purposes by those Bands as well as other residents, businesses, and landowners in the Upper San Luis Rey River Basin. Southern California water resiliency is improved by local water storage in these two reservoirs, thus reducing the dependence on water imported from the Colorado River or Northern California. As is typical for Southern California’s Mediterranean climate, the area receives around 36 cm of rain annually, with the majority of precipitation occurring in the winter months. As a result, external nutrient loading due to runoff in the summer and fall is negligible. Current estimates of external loading to Lake Henshaw include pumping of groundwater from the upstream Warner Ranch Wellfield, which introduces 70 kilograms (kg) of P per month, primarily in the form of bioavailable phosphate, to the lake during dry periods (spring through fall) when the wellfield is pumping. Despite this, internal nutrient loading is currently understood to be the largest sauce of nutrients to the lake and thus plays a critical role in sustaining summer and fall HABs (Stillwater Sciences, 2022). While data corresponding to the oxygenation and thermal stratification patterns of Lake Henshaw are limited, the lake likely experiences periods of hypoxic or anoxic conditions in the late spring, summer, and early fall. Lake Wohlford is generally a mixed system that does not experience strong thermal stratification due to an artificial aeration system that, while not introducing much oxygen to the lake, does provide physical mixing. Despite this system, brief periods of low oxygen conditions have been noted in spring, summer, and fall (Stillwater Sciences, 2022). Given the time of year of sampling, oxygen conditions were likely hypoxic in both lakes.
2.2 Field collection of sediment and water
Sediment and bottom water were collected for incubation from Lake Henshaw and Lake Wohlford based on Beutel et al. (2008, 2020) in June 2021 and July 2021, respectively. An Ekman dredge was lowered from the sampling boat to the lake bottom. Once partially submerged in sediment, the dredge was triggered to close and then brought to the surface. Sediment was cohesive and plugged the dredge resulting in a sampling of an intact sediment–water interface sample. A polycarbonate tube (9 cm in diameter and 25 cm in height) was carefully inserted into the sediment and then capped at the bottom by hand, after which a supporting base and top were added. Each chamber contained ~5 cm of sediment and ~ 4 cm of bottom water. Additional bottom water was collected with a Van Dorn Bottle and slowly dripped into the chamber to fill the remaining headspace. At each reservoir, four chambers were collected from three sites, Dam, North, and Pier in Lake Henshaw (Figure 1) and Dam, Mid, and East in Lake Wohlford (Figure 2). Additional sediment samples were collected at the chamber sampling sites at both lakes, and an additional shallow site in Lake Henshaw (East), to enhance the spatial coverage of the sediment analysis. For the sediment samples, the top ~2 cm of sediment was removed from the Ekman dredge and placed in a clean sample bag. Chambers and sediment samples were kept on ice and transported to the University of California, Merced laboratory and then frozen until analysis.
2.3 Experimental incubations
The 12 chambers from each lake were incubated in the dark at ~25°C to imitate the warm and low-light conditions of these Southern California lake bottoms in summer (Figs. S-1 and S-2). These 12 chambers and the associated sediment were carried through the entirety of the experiment. The chambers were incubated under three DO phases: oxic, hypoxic, and anoxic. The oxic and hypoxic phases lasted for nine days each. The anoxic phase lasted eleven days for Lake Henshaw and twelve days for Lake Wohlford. Water samples were taken on days 0, 3, 6, 9/9, 12, 15, 18/18, 21, 24, 27, and 29 (Henshaw) or 30 (Wohlford). At the end of each phase, the chambers were refilled with bottom water and resampled to collect a sample to start the new phase, as represented with a slash above for days 9 and 18. Chambers were bubbled with air (~21% oxygen) during the oxic phase, dinitrogen gas with 4% oxygen and 400 parts per million (ppm) carbon dioxide for pH control during the hypoxic phase, and dinitrogen gas with 400 ppm carbon dioxide during the anoxic phase. Additional descriptions of field sample collection and experimental incubation methodology are available in Beutel et al. (2008, 2020).
Water samples were collected from chambers using a clean syringe and preserved using standard methods (APHA, 2005). A sample of 10 mL volume was preserved for nutrients (total ammonia, nitrate + nitrite, and soluble reactive P [SRP]) by filtering with a prewashed 0.45 μm filter and freezing. A sample of 20 mL volume was preserved for total Fe and total Mn by acidifying to 1% with metals-grade nitric acid and refrigerating. If sulfide odors were present, 60 mL of water was sampled into a container with 0.25% 2 normal zinc acetate and sodium hydroxide and refrigerated. DO, pH, and redox potential were monitored every 3–6 days by inserting a calibrated probe (HACH HQ40d meter with IntelliCAL ORP-REDOX, IntelliCAL pH, and IntelliCAL LDO101 probes) into a top port of the chamber. The calibration of probes was checked every 6 days against water-saturated air for DO, and with standard reference solutions for pH and redox potential. Measured redox potential was converted to Eh based on APHA (2005). During anoxic phase sampling and monitoring, the chamber headspace was flushed with dinitrogen gas to avoid oxygen contamination.
2.4 Water and sediment quality analysis
Water samples were analyzed at the University of California, Merced Environmental Analytical Laboratory. Total ammonia (comprised of ammonium and ammonia, and hereafter referred to as ammonia), nitrate + nitrite (hereafter referred to as nitrate presuming negligible nitrite), and SRP were measured using standard colorimetric methods (Lachat QuikChem 8,500 auto analyzer) (APHA, 2005). The reporting limit was 0.25 mg-N/L for ammonia, 0.05 mg-N/L for nitrate, and 0.1 mg-P/L for SRP. Total Fe and Mn were measured using inductively coupled plasma optical emission spectrometry (Perkin-Elmer Optima 5,300 DV) (APHA, 2005). The reporting limit for total Fe and Mn was 0.01 mg/L. Quality assurance measures included analytical duplicates, bottle blanks, and analytical ongoing precision and recovery samples. Total sulfide samples were analyzed by iodometric titration with a detection limit of 0.4 mg/L (APHA, 2005). See Supplemental material for entire chamber water concentration dataset for Lake Henshaw (Supplementary Table S1) and Lake Wohlford (Supplementary Table S2).
Sediment samples, four from Lake Henshaw and three for Lake Wohlford, were characterized for water content (heated at 103°C overnight) and loss on ignition (LOI) (combusted at 500°C for 4 h) (APHA, 2005). Carbon (C) and N content of the sediment was determined through the dry combustion method (Chatterjee et al., 2009) using a COSTECH 410 Elemental Analyzer coupled with ThermoFisher Delta V+ Isotope Ratio Mass Spectrometer, housed in the Stable Isotope Ecosystem Laboratory of University of California, Merced. Sediment sub-samples were sent to TestAmerica in Sacramento, California, USA, a certified analytical laboratory, for analysis of total aluminum (Al), total Mn, total Fe, and total P (TP). Standard sediment digestion and digestant analysis methods were used (APHA, 2005).
2.5 Flux calculations and data analysis
Rates of mass flux from sediment to overlaying water were calculated for ammonia, nitrate, SRP, total Fe, and total Mn. The rates were calculated for each 3-day sampling event using the equation:
where F (mg/m2·d) is flux, C2 (g/m3) is the concentration at the end of the event, C1 (g/m3) is the concentration at start of the event, V (m3) is the water volume at the start of the event, t2 (d) is the time at the end of the event, t1 (d) is the time at the start of the event, and A (m2) is sediment area. Chamber water height was measured at each sampling time to estimate the ratio of V over A and t2 minus t1 was typically 3 days. For statistical assessment, mean fluxes were first calculated using equation 1 for each phase (“mean phase fluxes”) in each chamber as an average of the 3-day fluxes measured for that phase (i.e., the mean flux for a 9-day oxic phase in a given chamber was the average of the three 3-day fluxes measured in that phase). Mean phase fluxes from quadruplicate chambers (i.e., n = 4) were then assessed for statistical differences between phases (oxic, hypoxic, and anoxic) for a given site (Dam, North and Pier in Lake Henshaw; Dam, Mid and East in Lake Wohlford) using a Student’s paired t-test. Mean phase fluxes for a given phase (oxic, hypoxic or anoxic) were also assessed for statistical differences between sites, within reservoirs (inter-site comparison), and between reservoirs (intra-site comparison), using a Student’s independent t-test. Statistical significance was assigned at p < 0.05.
3 Results
3.1 Sediment quality
The quality of the sediments from the two reservoirs was fairly similar (Table 1). Sediments from both sites had relatively high water content (~70–90%) and LOI (~11–18%). Values ranged 4.9–8.6% for C content and 0.46–0.95% for N content. Sediment total Fe concentrations ranged from 42,779–126,231 mg/kg dry weight (dw), and total Mn concentrations ranged from 813–2,970 mg/kg dw. Sediment TP concentrations at both sites were relatively high and ranged from 979–4,010 mg/kg dw. For all samples, the C:LOI ratio was ~0.5 while the N:P mass ratio was ~2–5. The Fe:P mass ratio, a coarse indicator of a sediment’s ability to sequester P under oxic conditions, ranged ~25–45.
3.2 Pattern of chamber water quality
In Lake Henshaw chamber water, DO concentration was ~7.5–8 mg/L in the oxic phase and ~ 2 mg/L in the hypoxic phase (Figure 3; Table 2; Supplementary Table S1). pH was stable through the experiment ranging from 8.2–8.8. Eh was ~340 mV during the oxic and hypoxic phases but decreased dramatically with the onset of anoxia to around <100–150 mV by the end of the anoxic phase. Ammonia concentration typically increased during the oxic phase to 15–20 mg-N/L, decreased to ~5 mg-N/L during the hypoxic phase, then showed a continuous increase during the anoxic phase to high concentrations, ranging from 25–35 mg-N/L. Nitrate was typically present late in the oxic phase and during the hypoxic phase at concentrations of ~5 mg-N/L and coincided with decreases in ammonia. Nitrate disappeared early in the anoxic phase. SRP concentrations typically increased during all DO phases and showed elevated concentrations late in the anoxic phase, ranging from 10–15 mg-P/L. Mn concentration generally decreased during the oxic phase, then showed a steady increase during the hypoxic phase to ~1 mg/L and stayed around this value for the hypoxic phase. Fe concentration typically was below 0.3 mg/L and showed a minor decrease during the oxic phase and minor increase during the hypoxic phase. Peak concentrations of ammonia, SRP and Mn were higher at the Dam site compared to the other sites. For example, ammonia concentrations at the end of the anoxic phase were 32–52 mg-N/L at the Dam site, 20–33 mg-N/L at the North site, and 11–19 mg-N/L at the Pier site. Sulfide was detected in Lake Henshaw chambers during the anoxic phase at concentrations of 0.7–3.3 mg/L. In the anoxic phase, fine black particles appeared in chamber water and on chamber surfaces, suggesting the formation of FeS precipitate (Fig. S-1). Concentrations of sulfide and total Fe in chamber water were indicative of FeS precipitation based on the estimated FeS solubility product for chamber water (Beutel et al., 2020).
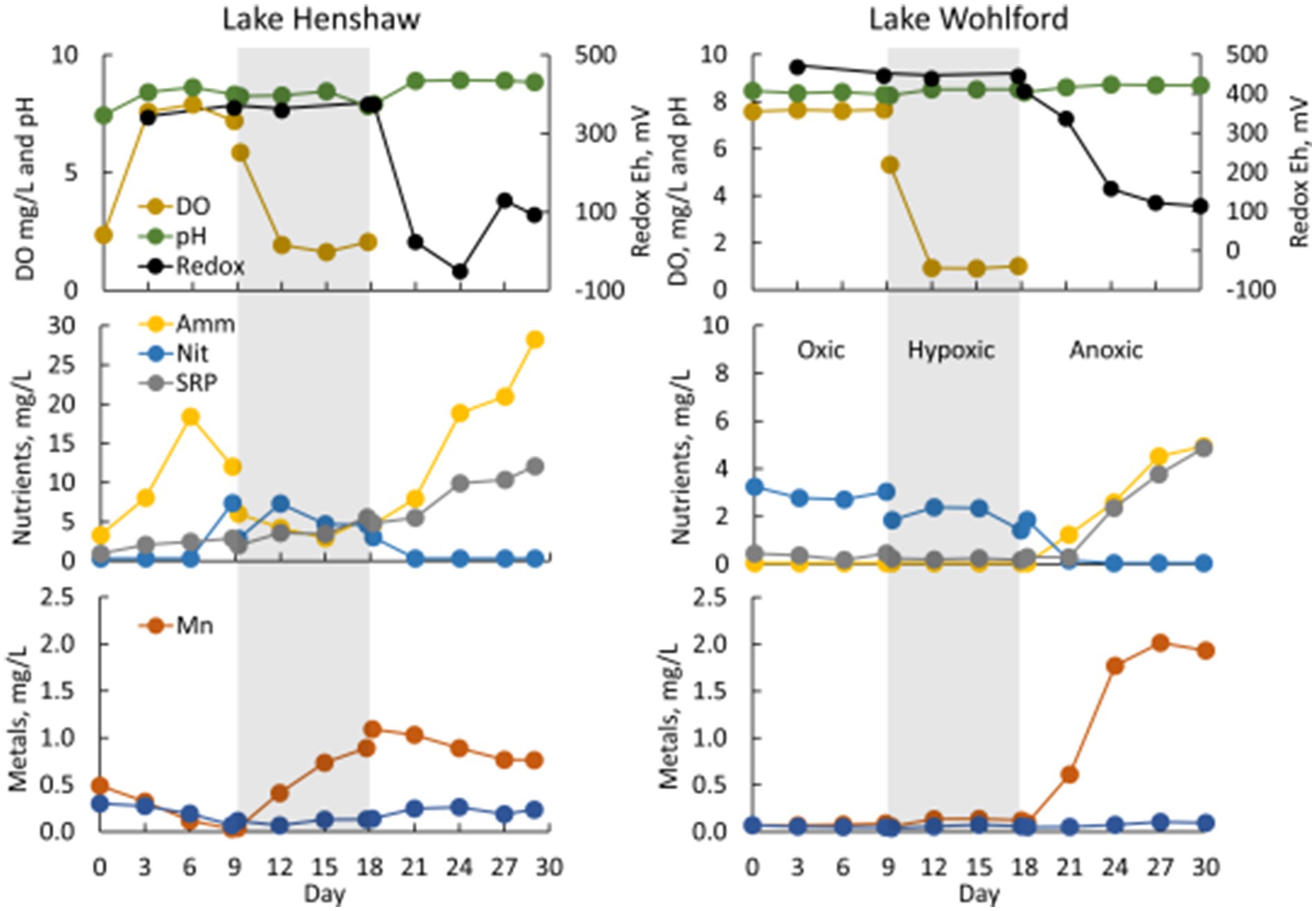
Figure 3. Water quality in select chambers from Lake Henshaw (North chamber replicate 2, left) and Lake Wohlford (East chamber replicate 3, right). Top: dissolved oxygen (DO), pH, and redox Eh. Middle: ammonia (Amm), nitrate plus nitrite (Nit), and soluble reactive phosphorus (SRP). Bottom: total manganese (Mn) and total iron (Fe). Shading distinguishes oxygen phases: oxic days 0–9, hypoxic days 9–18 (shaded), anoxic after day 18. Note difference in y-axis scale on nutrient plots.
In Lake Wohlford chamber water, DO concentration was ~7–8 mg/L in the oxic phase and ~ 1 mg/L in the hypoxic phase, which was lower than that observed in Lake Henshaw chambers (~2 mg/L) (Figure 3; Table 3). pH was stable through the experiment ranging from 8.4–8.8. Eh remained steady during the oxic and hypoxic phases at ~420 mV and dropped with the onset of anoxia to ~50–200 mV depending on the site. Ammonia concentration was negligible during oxic and hypoxic phases, then showed a continuous increase during the anoxic phase, typically ranging from 2–6 mg-N/L by the end of the incubation depending on site. Nitrate was present during the oxic and hypoxic phases, ranging from ~0.5–3 mg-N/L. Nitrate disappeared early in the anoxic phase. SRP concentrations were typically <1 mg-P/L during the oxic and hypoxic phases and increased during the anoxic phase, ranging from ~3–5 mg-P/L by the end of the incubation. Mn concentration was generally low (< 1 mg/L) during the oxic and hypoxic phases and increased during the anoxic phase, ranging from ~0–3 mg/L by the end of the incubation. Fe concentration typically was <0.1 mg/L in the oxic and hypoxic phases and increased slightly in the anoxic phase. Sulfide was not detected throughout the incubation in Lake Wohlford chamber water. Peak concentrations of ammonia, SRP and Mn were higher at the Dam and Mid sites compared to the East site. For example, ammonia concentrations at the end of anoxic phase were 5–6 mg-N/L at the Dam site, 3–6 mg-N/L at the Mid site, and 2–3 mg-N/L at the East site.
3.3 Patterns of nutrient and metals fluxes
Lake Henshaw exhibited high fluxes of nutrients and metals, with the highest fluxes generally occurring under hypoxic and anoxic conditions (Figure 4; Table 2). Aside from the oxic Dam chambers which showed an elevated mean ammonia flux, values were relatively low (<53 mg-N/m2·d) or negative (ammonia loss) at all sites during oxic and hypoxic phases. Mean ammonia flux was higher in the anoxic phase (212–366 mg-N/m2·d) compared to other phases, and the highest anoxic flux was in Dam chambers. Mean SRP flux was lowest (9.3–41 mg-P/m2·d) during the oxic phase, higher during the hypoxic phase (38–51 mg-P/m2·d), and very high during the anoxic phase (91–122 mg-P/m2·d), with Dam chambers again having the highest mean flux. Mean Mn flux was negative under oxic conditions (−7.1 to −4.9 mg/m2·d), consistent with Mn concentrations decreasing in chamber waters during this portion of the experiment. In Dam and North chambers, mean Mn flux was highest under hypoxic conditions (14.9–16 mg/m2·d) and lowest under anoxic conditions (1.8–6.4 mg/m2·d). In contrast, mean Mn flux was highest during the anoxic phase in Pier chambers (8.7 mg/m2·d), indicating that Pier sediments were less susceptible to becoming reduced under hypoxic conditions compared to the other sites. Fe flux was low or negative under oxic conditions (−2.4 to 0.9 mg/m2·d) but increased under hypoxic conditions (−0.8 to 3.3 mg/m2·d). The highest mean Fe flux was observed under hypoxic conditions in the North chambers (3.3 mg/m2·d), while the Dam chambers had the highest mean anoxic Fe flux (2.4 mg/m2·d).
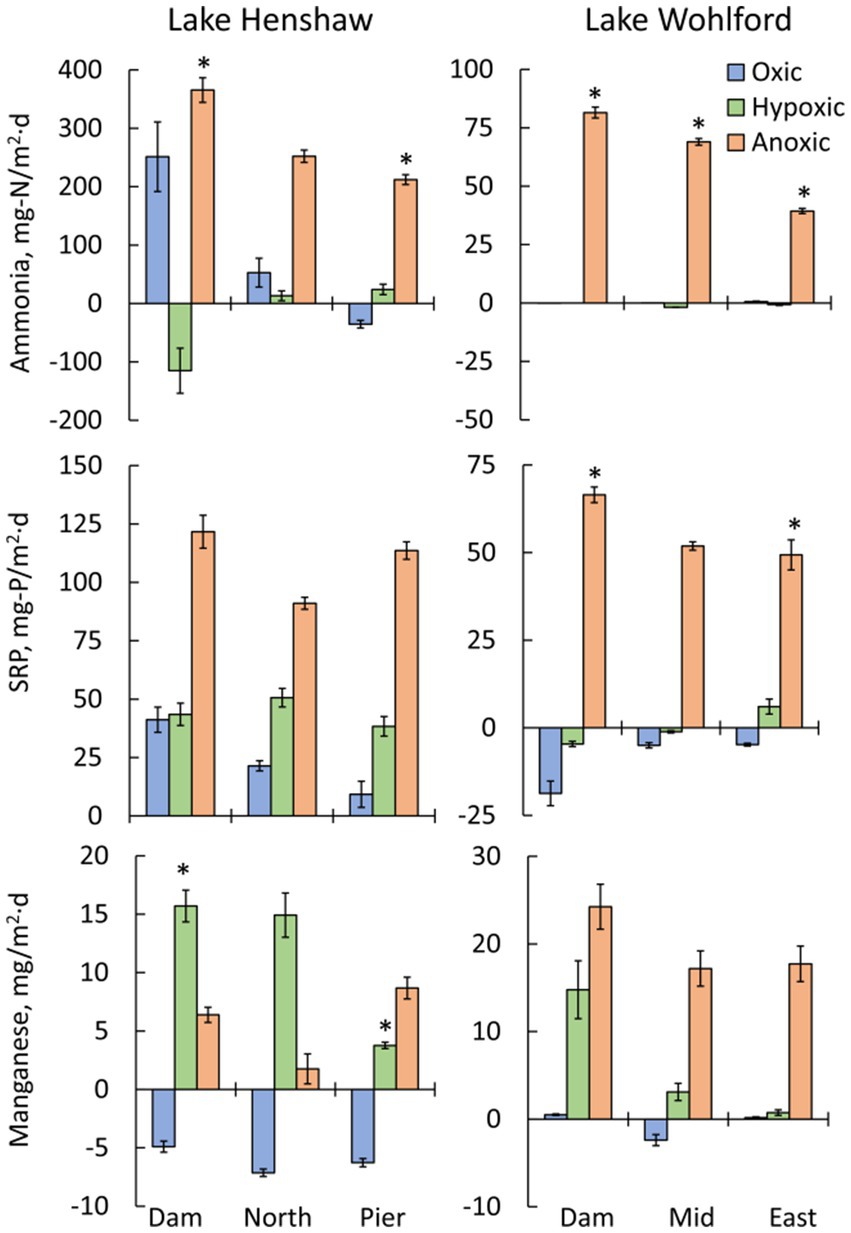
Figure 4. Mean fluxes of nutrients and metals by oxygen phase (oxic, hypoxic and anoxic) and by site in chambers from Lake Henshaw (left) and Lake Wohlford (right). Top: ammonia flux. Middle: soluble reactive phosphorus (SRP) flux. Bottom: total manganese flux. Note difference in y-axis scale on ammonia and SRP plots. Error bars are plus/minus one standard error from quadruplicate chambers (n = 4). Statistical differences are shown for select inter-site comparisons, with an asterisk (*) indicating significant differences (p < 0.05 of a Student’s independent t-test; see Methods Section 2.5).
Lake Wohlford had lower fluxes of ammonia, SRP and Mn compared to Lake Henshaw, with fluxes predominantly occurring under anoxic conditions and highest in Dam chambers (Figure 4; Table 3). Mean ammonia flux was negligible under oxic and hypoxic conditions but elevated under anoxic conditions (39–82 mg-N/m2·d). Mean SRP flux was also low or negative (SRP loss) under oxic and hypoxic conditions and high during anoxic conditions (49–67 mg-P/m2·d). Mean Mn flux was relatively high in Dam chambers under both hypoxic and anoxic conditions (15–24 mg/m2·d). In contrast, mean Mn flux in Mid and East chambers was elevated mainly during anoxic conditions (17–18 mg/m2·d), indicating that these sediments were less susceptible to becoming reduced under hypoxic conditions compared to the Dam site. Fluxes of Fe were relatively low but appeared higher (1–2 mg/m2·d) under hypoxic and/or anoxic conditions in Mid and East chambers.
3.4 Differences in fluxes across treatments and sites
In Lake Henshaw chambers, differences in mean fluxes between DO phases for a given site showed varied patterns of significance (Table 2). At some sites oxic/hypoxic phases were significantly different from the anoxic phase, while at other sites the oxic phase was significantly different from the hypoxic/anoxic phases. Mean ammonia release was significantly different between the oxic/hypoxic and anoxic phases for the North and Pier chambers. For SRP, Dam and Pier chambers showed significant difference between the oxic/hypoxic phases and anoxic phase, and North chambers showed significant difference between all three DO phases. The Lake Henshaw mean Mn fluxes were significantly different between the oxic and the hypoxic/anoxic phases at all sites.
In Lake Wohlford Chambers, in general oxic/hypoxic phase fluxes were significantly different from the anoxic phase fluxes (Table 3). Mean ammonia flux for the Dam and East chambers showed significant differences in oxic/hypoxic phases and anoxic phase, while Mid chambers showed significant differences between oxic, hypoxic, and anoxic phases. All sites showed significant differences between oxic/hypoxic phases and anoxic phase for SRP. For Mn, Dam and Mid chambers showed significant differences between the oxic and anoxic phases, while East chambers showed significant differences between the oxic/hypoxic phases and anoxic phase.
An inter-site comparison of nutrient and metal fluxes at both sites showed some spatial patterns (Figure 4). The largest mean fluxes of ammonia, SRP and Mn in Lake Henshaw were observed in the Dam chambers. Mean anoxic flux of ammonia was significantly different between Dam and Pier chambers. Mean hypoxic flux of Mn was also significantly different between Dam and Pier chambers. The largest metal and nutrient fluxes were also seen in the Dam chambers at Lake Wohlford. Mean hypoxic ammonia flux was significantly different between all three chamber sites. Dam and Mid chambers also had significantly different fluxes for SRP under anoxic conditions. An intra-site comparison between reservoirs showed that both anoxic ammonia flux and anoxic SRP flux were significantly higher in Lake Henshaw compared to Lake Wohlford when comparing Dam versus Dam chambers, North versus Mid chambers, and Pier versus East chambers.
4 Discussion
4.1 Sediment quality
Sediment quality was similar between the two reservoirs. The C:LOI ratio of approximately 0.5 is consistent with organic matter of phytoplankton origin which is typically ~50% C (Reynolds, 2006). The N:P ratio is lower than typical phytoplankton organic matter (OM) of ~10:1, indicative of a sink for N in OM via nitrification and denitrification (Vanni et al., 2011). Fe:P ratio, a coarse indicator of sediment’s ability to retain P under oxidized conditions via sorption to Fe(III) oxides, ranged from 23.9 to 46.4 and was generally above 30. Fe:P ratios above 30 have been observed to correspond with low aerobic Fe release, depending on the sulfur content of sediment since FeS precipitation can be a sink for Fe (Wang et al., 2018). Fe in the sediment was higher than Mn, likely because Mn has a loss mechanism via reductive dissolution to Mn(II), which does not easily re-oxidize to particulate and settle out, and instead can wash out of the system (Davison, 1993). The greatest values for LOI and % C were near the Dam for both reservoirs. This is a common observation in reservoirs, likely because of sediment “focusing” of allochthonous organic matter into deeper regions, combined with lower rates of organic matter degradation in deeper anoxic sediments (Thornton et al., 1990; Blais and Kalff, 1995; Beutel et al., 2020).
There appeared to be a linkage between sediment quality and sediment nutrient and metals release in both reservoirs. The pattern was especially clear for Lake Wohlford where LOI, %N, %C, TP, and Mn concentration in sediment all increased with sample site depth (Table 1), as did ammonia and SRP anoxic release rates and Mn hypoxic and anoxic release rates (Figure 4). Mn release, which is a good indicator of the onset of reduced conditions at the sediment–water interface since Mn oxides are very susceptible to reductive dissolution (Davison, 1993), also showed clear spatial trends in both lakes. Mn release occurred earlier (i.e., during hypoxic conditions) and at higher magnitudes in deeper sites, indicating the more organic-rich sediments at deeper sites had greater potential to become reduced during low DO conditions. Lake Henshaw also appeared to exhibit spatial patterns of SRP release under oxic and hypoxic conditions, with levels particularly elevated during both phases at the Dam site (Figure 4). This result corresponds with sediment P fractionation results in Lake Henshaw, which indicated that labile, reductant-soluble, and total P in sediment generally increased with sampling site depth, as did concentrations of labile and total organic matter (Meis et al., 2012; Stillwater Sciences, 2023). Thus, deeper sediment in Lake Henshaw appeared to have greater pools of labile and redox-sensitive P, elevated organic matter, and a stronger tendency to become reduced. This resulted in elevated potential to release P during oxic and hypoxic conditions.
Chamber sediment from both sites exhibited interesting biological characteristics worth highlighting. Early in the anoxic phase of Lake Henshaw incubations, chamber sediment became covered with a white, filamentous mat (Supplementary Figure S1). This has been observed in other chamber incubations and was likely the growth of Beggiatoa spp., bacteria capable of oxidizing sulfide to whitish elemental sulfur under micro-aerophilic conditions (Beutel et al., 2020). Lake Wohlford sediments had greater macrobenthos densities, observed especially at the start of the anoxic phase when worms were out of their burrows attempting to breathe under low DO conditions (Supplementary Figure S2). The higher density of respiring macrobenthos may account for the apparently contradictory observation of lower steady state DO concentrations under hypoxic conditions (Aller et al., 2001) in meso-eutrophic Lake Wohlford (~1 mg/L DO) compared to hypereutrophic Lake Henshaw (~2 mg/L) (Figure 3).
4.2 Magnitude of anoxic nutrient fluxes
Both lakes had relatively high magnitude of anoxic ammonia fluxes (212–366 mg-N/m2⋅d for Lake Henshaw; 39–82 mg-N/m2⋅d for Lake Wohlford, both at 25°C) compared to previous studies. At Hodges Reservoir (San Diego, California, USA) and Lake Elsinore (Riverside, California, USA), both hypereutrophic systems, ammonia fluxes ranged from 80–110 mg-N/m2⋅d at 15°C and 40–120 mg-N/m2⋅d at 27°C (Beutel, 2006, 2000). At hypereutrophic Lake Kevätön (Siilinjärvi, Finland), anoxic ammonia fluxes ranged between 20–30 mg-N/m2⋅d at 16°C and 35–45 mg-N/m2⋅d at 23°C (Liikanen et al., 2002). Ammonia fluxes at Lake Biwa (Shiga, Japan) ranged from 17–18 mg-N/m2⋅d when incubated at 9°C (Osaka et al., 2021). At eutrophic San Vicente Reservoir in Southern California, anoxic ammonia fluxes ranged from 17–69 mg-N/m2⋅d at 12°C (Beutel et al., 2007). Using a theta value (θ) of 1.07 for N mineralization (Kadlec and Reddy, 2001; Liikanen et al., 2002; van Luijn et al., 1999), we can normalize the ammonia release rates to 20°C and then compare using the conventional rate equation:
where R1 (mg-N/m2⋅d) is the rate of release after normalization, R2 (mg-N/m2⋅d) is the rate of release during the experiment, T1 (°C) is 20°C, and T2 (°C) is the temperature at which the experiment took place. After normalization to 20°C, findings suggest that Lake Henshaw’s ammonia fluxes are extreme compared to other reservoirs, while those in Lake Wohlford are consistent with previous studies (Table 4).
Like ammonia fluxes, SRP fluxes from anoxic sediment from both reservoirs were relatively high (91–122 mg-P/m2⋅d for Lake Henshaw; 49–67 mg-P/m2⋅d for Lake Wohlford, both at 25°C). These high rates may not sustain themselves over time as sediments become depleted in Fe-bound phosphate. A review of SRP concentration trends in chamber water during the 12-day anoxic phase (Supplementary Tables S1, S2) indicates consistent SRP accumulation in Lake Henshaw chambers, but deceleration of SRP accumaution in some Lake Wohlford chambers (e.g., Mid-2, Mid-3, Mid-4 and East-1). P fluxes from anoxic sediment of hypereutrophic North American reservoirs reportedly range from 10–30 mg-P/m2⋅d (Nünberg, 1994). However, these measurements likely occurred under cooler conditions (~ 14°C or less). Another study used slightly warmer conditions when assessing anoxic sediment phosphate flux (~24–27°C) and found the release rate in hypereutrophic Lake Elsinore to range between 8–27 mg-P/m2⋅d (Beutel, 2000). Under cooler conditions (12–15°C), anoxic SRP releases were found to range from 17–33 mg-P/m2d in hypereutrophic Lake Hodges and 10–28 mg-P/m2⋅d in eutrophic San Vicente Reservoir (Beutel et al., 2007, 2020). SRP fluxes in hypereutrophic Clear Lake (Clearlake, USA) were found to range between 9–27 mg-P/m2⋅d at 15°C and between 13–22 mg-P/m2⋅d at 23°C (Framstead, 2022). Like ammonia, P release from aquatic sediments is temperature sensitive (Duan and Kaushal, 2013), with a reported θ value of ~1.10 for anoxic P release from lake sediment (Jensen and Andersen, 1992; Liikanen et al., 2002; Nürnberg, 2009; Wu et al., 2013). Using Equation 2, we can normalize the SRP release rates to 20°C, where appropriate, and compare. After normalization to 20°C, findings suggest that Lake Henshaw’s SRP fluxes are extreme compared to other reservoirs, while those in Lake Wohlford are consistent with previous studies (Table 5).
The reported θ value for TP release from anoxic lake sediment (1.10) merits further discussion due to the multiple processes associated with P cycling and variability of estimates of this parameter within the literature. The release of P from sediment is controlled by biotic processes (e.g., mineralization; microbial reductive dissolution of Fe oxides) and abiotic processes (e.g., diffusion rates; reductive dissolution of Fe oxides by sulfide) that may have differing temperature sensitivities (Lovley, 1995; Melton et al., 2014; Chen et al., 2016). Increased temperature within a system has been found to increase Fe reducing bacterial activity and thus P release from the sediment (Holdren and Armstrong, 1980; Jiang et al., 2008; Dadi et al., 2020; Liu et al., 2023). Geobacteraceae, a family of bacteria with subgroups Geobacter and Desulfuromonas, have been identified through rRNA sequencing and phylogenetic analysis as common Fe-reducing bacteria in sediments that are temperature sensitive (Lonergan et al., 1996; Zhao et al., 2021). A handful of studies report Q10 values, the factor by which the rate increases with a 10°C increase in temperature, for Fe reduction in aquatic sediment ranging from ~1.4–4.0 (Chen et al., 2014; Schilling et al., 2018). This is equivalent to a θ value of 1.03–1.15 and, presuming Fe reduction is a primary mechanism driving anoxic P release, suggests that a value of 1.10 is reasonable for comparison of release rates at different temperatures. Additionally, abiotic processes, such as the reduction of Fe by sulfides, are also responsive to temperature as they are secondarily influenced by bacterial respiration (Chen et al., 2014). The sensitivity of P release to temperature, as well as ammonia release (discussed above), means that the effect of water temperature on internal nutrient loading rates is an important factor to consider in reservoir management, particularly as water managers plan for the impacts of climate change on lakes and reservoirs.
Both depth and trophic status are important factors controlling nutrient dynamics in reservoirs. Lake Henshaw is a shallow, hypereutrophic reservoir, while Lake Wohlford is a moderately deep, meso-eutrophic reservoir. Lake Henshaw chambers exhibited higher nutrient and metal fluxes under a greater variety of DO conditions, while fluxes in Lake Wohlford chambers occurred mainly during the anoxic phase (Figure 4). Shallow lakes are known to pose management challenges due to the longevity of internal nutrient loading and elevated P released from the sediment into the photic zone (Welch and Dennis Cooke, 2005; Søndergaard et al., 2012). Within shallow lakes, organic matter quicky deposits at the sediment–water interface, where it drives respiration and undergoes mineralization and decay (Beutel, 2003). Trophic status is also an important factor controlling the magnitude of sediment nutrient and metals release in reservoirs, as nutrient loading leads to greater phytoplankton production and organic matter deposition into bottom waters, providing both the organic matter to drive respiration that results in anoxia and a source of nutrients to be recycled. As was observed in this study, anoxic release rates from lake and reservoir sediments for both ammonia and P have been shown to increase with increasing trophic status. Values are typically >15–30 mg/m2·d for hypereutrophic systems and < 5 mg/m2·d for mesotrophic systems (Beutel, 2006; Nünberg, 1994). This phenomenon was also apparent within each study reservoir. As discussed in the above section, both reservoirs showed a general trend in which sediments with higher organic and nutrient content exhibited higher anoxic release rates of nutrients and metals. Together, the shallow depth and elevated trophic status of Lake Henshaw combine to make this site especially susceptible to high rates of internal nutrient and metals recycling.
4.3 Patterns of nutrient release of under different oxygenation phases in Lake Henshaw
Shallow lakes like Lake Henshaw are dynamic systems with variations across spatial and temporal scales (Scheffer, 2004). Spatially, they vary in depth and commonly exhibit extensive shallow areas and relatively small deeper zones (Padisák and Reynolds, 2003). Temporally, the DO of the water column may fluctuate between oxic during the day, anoxic during the night, and hypoxic during dusk and dawn associated with patterns of photosynthesis and respiration (Van Duin and Lijklema, 1989). Additionally, changes in wind patterns may cause periods of high water column mixing during windy conditions or temporary thermal stratification during calm, warm conditions, thereby shifting the lake between oxic, hypoxic, and anoxic conditions in time and space (Søndergaard et al., 2023). Nutrient limitation may also shift spatially or temporally between key limiting nutrients such as N or P (Maberly et al., 2020). In the context of our experiment, we attempted to assess this variability by assessing sediment nutrient release dynamics at multiple sites and under multiple oxygenation regimes indicative of typical temporal patterns that affect oxygen levels in the water column (e.g., day vs. night or calm vs. windy).
Based on our experimental results from Lake Henshaw, particularly the shallower North and Pier sites that represent most of the lake area, different incubation phases (oxic, hypoxic and anoxic) showed different patterns of N and P release (Figure 5; Table 6), which in turn could affect phytoplankton dynamics. Under oxic conditions, sediment was a large source of nitrate likley as a byproduct of biological nitrification of ammonia. Sediments appeared to be a small sink (Pier site) or source (North site) of ammonia to overlaying water. Phosphate was released likely as the result of organic matter mineralization, but under oxic conditions some phosphate may have been retained in sediment via sorption to Fe oxides in surficial sediment. This led to a relatively high N:P ratio in nutrient sediment release to overlaying water (10:1; Table 6). It is important to note that this ratio is composed of dissolved inorganic nitrogen and SRP, which can be representative inorganic dissolved phosphorus, though has limitations (Jarvie et al., 2002). Under hypoxic conditions, N cycling shifted with denitrification appearing to dominate and sediments becoming a large sink for nitrate and a continued source of ammonia. Lower redox potential appeared to promote the microbial reductive dissolution of metal oxides, resulting in some Mn and Fe release. Phosphate release was enhanced as sorbed phosphate was likely released from dissolving Fe oxides. In contrast to oxic conditions, hypoxic conditions led to a very low N:P ratio in nutrient sediment release to overlaying water (0:45; Table 6). Under anoxic conditions, both ammonia and SRP release increased dramatically, likley because of suppressed nitrification coupled with enhanced reductive dissolution of Fe oxides released sorbed phosphate. While Mn release continued, reduced conditions led to sulfide release and the loss of Fe via FeS precipitation. Anoxic conditions led to high levels of N and P release and a relatively low N:P ratio in nutrient sediment release to overlaying water (1.8:1; Table 6).
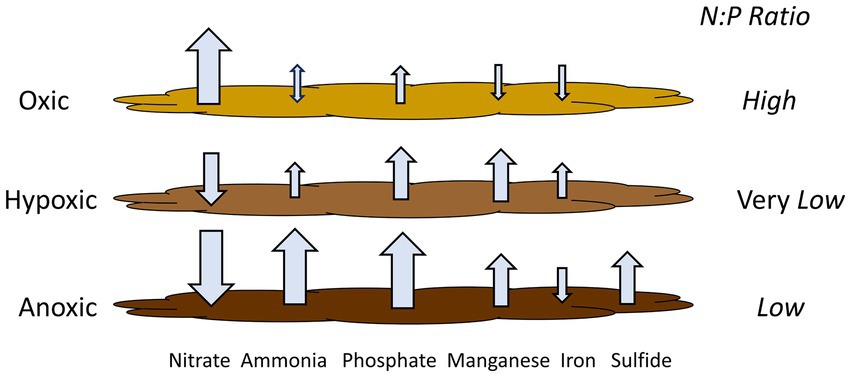
Figure 5. Release rates of monitored compounds under the various oxygen regimes considered with N:P ratios. Ratio are based on dissolved inorganic nitrogen and SRP in nutrient fluxes to overlaying water. Hypoxic conditions may lead to elevated phosphate flux relative to nitrogen flux from sediment, as well as some iron flux. This yields internal nutrient loading with a very low N:P ratio, which, combined with iron, could shift lake ecology so that cyanobacteria constitute a large portion of phytoplankton and may be a component in triggering HABs.
Nutrient release dynamics under hypoxic conditions, with elevated release of P relative to N and the release of Fe, may be of particular ecological relevance. Other studies have shown low N release and elevated P release under hypoxic conditions (Hayakawa et al., 2015; Gerling et al., 2016; Doig et al., 2016; Osaka et al., 2021). In chamber incubations with sediment from mesotrophic Lake Biwa, Japan, Osaka et al. (2021) found that ammonia concentration in overlaying water increased with decreasing DO along the oxic to anoxic spectrum, while phosphate concentration in water increased when DO was below 1.5 mg/L. In addition, studies have shown that decreases in N relative to P can shift lake ecology such that cyanobacteria make up a large portion of phytoplankton. In eutrophic Lake Okeechobee, Florida, USA, the N:P ratio decreased due to a decrease in inflow water N:P and a decrease in P assimilation within the lake, leading to an increase in N2-fixing cyanobacteria populations (Havens et al., 2003). Increased in-lake P concentration in Lake Peipsi, Estonia/Russia, doubled the biomass of N2-fixing cyanobacteria contribution to the phytoplankton community (Nõges et al., 2004). Finally, the presence of Fe in combination with low N:P ratio may be a key component in triggering HABs, as cyanobacteria have a higher Fe requirement compared to eukaryotic phytoplankton (Molot et al., 2014).
The differing dynamics of internal loading under varying DO conditions may, in part, explain the stochastic nature of HAB toxins production in Lake Henshaw, a common phenomenon in lakes and reservoirs (Brooks et al., 2015). Cyanobacteria in lakes can either be N2 fixing or non-N2 fixing. In the case of Lake Henshaw, the reservoir supports three dominant genera of cyanobacteria: non-N2-fixing Microcystis and Planktothrix and N2-fixing Dolichospermum (formerly Anabaena). In general, microcystin production tended to co-occur with high cell density of Microcystis and Planktothrix, while anatoxin-a production tended to co-occur with high cell density of Dolichospermum (Stillwater Sciences, 2022). While absolute concentrations of N and P (i.e., independent of N:P ratios) are also important to the growth and dominance of cyanobacteria, the low N:P ratio and Fe-rich conditions associated with hypoxic conditions could advantage Dolichospermum (Kramer et al., 2022), while the moderate N:P ratio and high N and P concentrations associated with anoxic conditions could advantage Microcystis and Planktothrix (Gobler et al., 2016), thus leading to different patterns of toxin production under hypoxic versus anoxic conditions. Relatively higher levels of ammonium release under anoxic conditions may further support cyanobacterial synthesis of microcystin, an N-rich molecule (Gobler et al., 2016; Wagner et al., 2021). Keeping in mind the dynamic nature of shallow lakes, shifts between oxic, hypoxic and anoxic conditions can occur frequently over time (e.g., oxic during the day when photosynthesis is dominant, but hypoxic at night when respiration is dominant) and space (e.g., oxic shallow sediment but hypoxic or anoxic deeper sediment). Thus, patterns of N:P released from sediments likely vary on small temporal and spatial scales in shallow lakes. This in turn may drive spatial and temporal patterns of cyanobacterial dominance by N2 fixing or non-N2 fixing cyanobacteria, which tend to have a different toxin production profile.
4.4 Management implications in Lake Henshaw, Lake Wohlford, and beyond
HABs pose a serious threat to the water quality of scarce water resources in places like Southern California, USA. DO is an important factor controlling biogeochemical cycling in reservoirs and influences internal nutrient loading and HAB events. To inform lake management efforts, this study used replicate sediment-water chambers collected from shallow, hypereutrophic Lake Henshaw and deep, meso-eutrophic Lake Wohlford, to assess how oxic, hypoxic, and anoxic conditions affect nutrient and metal cycling at the sediment–water interface. Sediment release of ammonia, SRP and redox-sensitive metals (Fe and Mn) was highest under anoxic conditions, with levels in Lake Henshaw exceeding levels typically seen in the literature. In Lake Henshaw, substantial P release was also observed under oxic and hypoxic conditions, and substantial Mn release was observed under hypoxic conditions. In contrast, release of nutrients and metals was negligible in Lake Wohlford under oxic and hypoxic conditions. At both sites there was a spatial pattern in which organic and nutrient content and anoxic release rates were highest in deeper sediment.
Water quality management in eutrophic reservoirs requires a complex consideration of biogeochemical processes and DO conditions, and management of both Lake Henshaw and Lake Wohlford will need to be adaptive and comprehensive to protect these critical water resources. Based in part on this study, managers at Lake Henshaw are implementing a comprehensive water quality management plan that includes the use of hydrogen peroxide for HABs control and sediment amendments to control internal P loading. A lake oxygenation field trial, based on the use of pure oxygen gas (Beutel and Horne, 1999), with the capacity to deliver up to 5,000 kg/d of dissolved oxygen to lake waters is also in the planning stages. At Lake Wohlford, managers are considering installation of a bottom water oxygenation system when the reservoir’s dam is replaced in future years.
Data availability statement
The datasets presented in this study can be found in online repositories. The names of the repository/repositories and accession number(s) can be found in the article/Supplementary material.
Author contributions
SD: Formal analysis, Investigation, Methodology, Visualization, Writing – original draft. MB: Conceptualization, Formal analysis, Investigation, Methodology, Visualization, Writing – original draft. NR-M: Investigation, Methodology, Writing – review & editing. MS: Conceptualization, Funding acquisition, Project administration, Supervision, Writing – review & editing.
Funding
The author(s) declare financial support was received for the research, authorship, and/or publication of this article. This project was funded in part by Stillwater Sciences and the Environmental Systems Graduate Group at UC Merced. This project was funded via an applied research award to the University of California from Stillwater Sciences financed by Vista Irrigation District and City of Escondido.
Acknowledgments
We would like to thank the following for support during this project: Mr. Don Smith and the staff at Vista Irrigation District; Mr. Reed Harlan and the staff at the City of Escondido; Dr. Liying Zhao and staff at the U.C. Merced Environmental Analytical Laboratory. We also thank the reviewers for their constructive comments on the manuscript. The views expressed herein are solely those of the authors and do not represent the official policies or positions of any supporting entity.
Conflict of interest
The authors declare that the research was conducted in the absence of any commercial or financial relationships that could be construed as a potential conflict of interest.
The reviewer RL declared a shared affiliation with the authors SD, MB, and NR-M to the handling editor at the time of review.
Publisher’s note
All claims expressed in this article are solely those of the authors and do not necessarily represent those of their affiliated organizations, or those of the publisher, the editors and the reviewers. Any product that may be evaluated in this article, or claim that may be made by its manufacturer, is not guaranteed or endorsed by the publisher.
Supplementary material
The Supplementary material for this article can be found online at: https://www.frontiersin.org/articles/10.3389/frwa.2024.1474057/full#supplementary-material
References
Aller, R. C., et al. (2001). “Transport and reaction in the bioirrigated zone” in The Benthic Boundary Layer: Transport Processes and Biogeochemistry. eds. Sprague and Watson (New York: Oxford University Press).
APHA (2005). Standard methods for the examination of water and wastewater. 21st Edn: American Public Health Association/American Water Works Association/Water Environment Federation. Washington, DC: American Public Health Association.
Backer, L. C., McNeel, S. V., Barber, T., Kirkpatrick, B., Williams, C., Irvin, M., et al. (2010). Recreational exposure to microcystins during algal blooms in two California lakes. Toxicon 55, 909–921. doi: 10.1016/j.toxicon.2009.07.006
Balistrieri, L. S., Murray, J. W., and Paul, B. (1992). The cycling of iron and manganese in the water column of Lake Sammamish, Washington. Limnol. Oceanogr. 37, 510–528. doi: 10.4319/lo.1992.37.3.0510
Betancourt, C., Jorge, F., Suárez, R., Beutel, M., and Gebremariam, S. (2010). Manganese sources and cycling in a tropical eutrophic water supply reservoir, Paso Bonito reservoir, Cuba. Lake Reserv. Manag. 26, 217–226. doi: 10.1080/07438141.2010.519856
Beutel, M. W. (2000). Report to Montgomery Watson and Alex Horne associates. California: Lake Elsinore Sediment-water Interface Study.
Beutel, M. W. (2003). Hypolimnetic anoxia and sediment oxygen demand in California drinking water reservoirs. Lake Reserv. Manag. 19, 208–221. doi: 10.1080/07438140309354086
Beutel, M. W. (2006). Inhibition of Ammonia release from anoxic Profundal sediments in lakes using Hypolimnetic oxygenation. Ecol. Eng. 28, 271–279. doi: 10.1016/j.ecoleng.2006.05.009
Beutel, M., Fuhrmann, B., Herbon, G., Chow, A., Brower, S., and Pasek, J. (2020). Cycling of methylmercury and other redox-sensitive compounds in the profundal zone of a hypereutrophic water supply reservoir. Hydrobiologia 847, 4425–4446. doi: 10.1007/s10750-020-04192-3
Beutel, M. W., and Horne, A. J. (1999). A review of the effects of hypolimnetic oxygenation on Lake and reservoir water quality. Lake Reserv. Manag. 15, 285–297. doi: 10.1080/07438149909354124
Beutel, M., et al. (2007). Evaluation of hypolimnetic oxygen demand in a large eutrophic raw water reservoir, San Vicente reservoir, calif. J. Environ. Eng. 133, 130–138. doi: 10.1061/(asce)0733-9372(2007)133:2(130).
Beutel, M. W., et al. (2008). Effects of aerobic and anaerobic conditions on P, N, Fe, Mn, and HG accumulation in waters overlaying Profundal sediments of an oligo-mesotrophic Lake. Water Res. 42, 1953–1962. doi: 10.1016/j.watres.2007.11.027
Blais, J. M., and Kalff, J. (1995). The influence of Lake morphometry on sediment focusing. Limnol. Oceanogr. 40, 582–588. doi: 10.4319/lo.1995.40.3.0582
Brooks, B. W., Lazorchak, J. M., Howard, M. D. A., Johnson, M.-V. V., Morton, S. L., Perkins, D. A. K., et al. (2015). Are harmful algal blooms becoming the greatest inland water quality threat to public health and aquatic ecosystems? Environ. Toxicol. Chem. 35, 6–13. doi: 10.1002/etc.3220
Chatterjee, A., Lal, R., Wielopolski, L., Martin, M. Z., and Ebinger, M. H. (2009). Evaluation of different soil carbon determination methods. Crit. Rev. Plant Sci. 28, 164–178. doi: 10.1080/07352680902776556
Chen, M., Li, X. H., He, Y. H., Song, N., Cai, H. Y., Wang, C., et al. (2016). Increasing sulfate concentrations result in higher sulfide production and phosphorous mobilization in a shallow eutrophic freshwater lake. Water Res. 96, 94–104. doi: 10.1016/j.watres.2016.03.030
Chen, M., Ye, T. R., Krumholz, L. R., and Jiang, H. L. (2014). Temperature and cyanobacterial bloom biomass influence phosphorous cycling in eutrophic lake sediments. PLoS One 9. doi: 10.1371/journal.pone.0093130
Conley, D. J., Paerl, H. W., Howarth, R. W., Boesch, D. F., Seitzinger, S. P., Havens, K. E., et al. (2009). Controlling eutrophication: nitrogen and phosphorus. Science 323, 1014–1015. doi: 10.1126/science.1167755
D’Autilia, R., Falcucci, M., Hull, V., and Parrella, L. (2004). Short time dissolved oxygen dynamics in shallow water ecosystems. Ecol. Model. 179, 297–306. doi: 10.1016/j.ecolmodel.2004.02.009
Dadi, T., Rinke, K., and Friese, K. (2020). Trajectories of sediment-water interactions in reservoirs as a result of temperature and oxygen conditions. Water 12:1065. doi: 10.3390/w12041065
Dadi, T., Schultze, M., Kong, X., Seewald, M., Rinke, K., and Friese, K. (2023). Sudden eutrophication of an aluminum Sulphate treated Lake due to abrupt increase of internal phosphorus loading after three decades of Mesotrophy. Water Res. 235:119824. doi: 10.1016/j.watres.2023.119824
Davison, W. (1993). Iron and manganese in lakes. Earth Sci. Rev. 34, 119–163. doi: 10.1016/0012-8252(93)90029-7
Doig, L. E., North, R. L., Hudson, J. J., Hewlett, C., Lindenschmidt, K. E., and Liber, K. (2016). Phosphorus release from sediments in a River-Valley reservoir in the northern Great Plains of North America. Hydrobiologia 787, 323–339. doi: 10.1007/s10750-016-2977-2
Duan, S. W., and Kaushal, S. S. (2013). Warming increases carbon and nutrient fluxes from sediments in streams across land use. Biogeosciences 10, 1193–1207. doi: 10.5194/bg-10-1193-2013
Falconer, I., and Humpage, A. (2005). Health risk assessment of cyanobacterial (blue-green algal) toxins in drinking water. Int. J. Environ. Res. Public Health 2, 43–50. doi: 10.3390/ijerph2005010043
Framstead . Measuring internal phosphorus loads: importance of accounting for seasonality in sediment phosphorus pools in hypereutrophic Clear Lake, CA. (2022). UC Davis, Master’s Thesis
Gerling, A. B., Munger, Z. W., Doubek, J. P., Hamre, K. D., Gantzer, P. A., Little, J. C., et al. (2016). Whole-catchment manipulations of internal and external loading reveal the sensitivity of a century-old reservoir to hypoxia. Ecosystems 19, 555–571. doi: 10.1007/s10021-015-9951-0
Gobler, C. J., Burkholder, J. A. M., Davis, T. W., Harke, M. J., Johengen, T., Stow, C. A., et al. (2016). The dual role of nitrogen supply in controlling the growth and toxicity of cyanobacterial blooms. Harmful Algae 54, 87–97. doi: 10.1016/j.hal.2016.01.010
Havens, K. E., James, R. T., East, T. L., and Smith, V. H. (2003). N:P ratios, light limitation, and cyanobacterial dominance in a subtropical Lake impacted by non-point source nutrient pollution. Environ. Pollut. 122, 379–390. doi: 10.1016/s0269-7491(02)00304-4
Havens, K. E., and Pearl, H. W. (2015). Climate change at a crossroad for control of harmful algal blooms. Environ. Sci. Technol. 49, 12605–12606. doi: 10.1021/acs.est.5b03990
Hayakawa, A., Ikeda, S., Tsushima, R., Ishikawa, Y., and Hidaka, S. (2015). Spatial and temporal variations in nutrients in water and riverbed sediments at the mouths of rivers that enter Lake Hachiro, a shallow eutrophic lake in Japan. Catena 133, 486–494. doi: 10.1016/j.catena.2015.04.009
Holdren, G. C., and Armstrong, D. E. (1980). Factors affecting phosphorus release from intact Lake sediment cores. Environ. Sci. Technol. 14, 79–87. doi: 10.1021/es60161a014
Jarvie, H. P., Withers, J. A., and Neal, C. (2002). Review of robust measurement of phosphorus in river water: sampling, storage, fractionation and sensitivity. Hydrol. Earth Syst. Sci. 6, 113–131. doi: 10.5194/hess-6-113-2002
Jensen, H. S., and Andersen, F. O. (1992). Importance of temperature, nitrate, and ph for phosphate release from aerobic sediments of four shallow, Eutrophic Lakes. Limnol. Oceanogr. 37, 577–589. doi: 10.4319/lo.1992.37.3.0577
Jiang, X., Jin, X., Yao, Y., Li, L., and Wu, F. (2008). Effects of biological activity, light, temperature and oxygen on phosphorus release processes at the sediment and water interface of Taihu Lake, China. Water Res. 42, 2251–2259. doi: 10.1016/j.watres.2007.12.003
Kadlec, R. H., and Reddy, K. R. (2001). Temperature effects in treatment wetlands. Water Environ. Res. 73, 543–557. doi: 10.2175/106143001x139614
Khadse, G. K., Patni, P. M., and Labhasetwar, P. K. (2015). Removal of iron and manganese from drinking water supply. Sustain. Water Res. Manag. 1, 157–165. doi: 10.1007/s40899-015-0017-4
Kramer, B. J., Jankowiak, J. G., Nanjappa, D., Harke, M. J., and Gobler, C. J. (2022). Nitrogen and phosphorus significantly alter growth, nitrogen fixation, anatoxin-a content, and the transcriptome of the bloom-forming cyanobacterium, dolichospermum. Front. Microbiol. 13. doi: 10.3389/fmicb.2022.955032
Langman, O. C., Hanson, P. C., Carpenter, S. R., and Hu, Y. H. (2010). Control of dissolved oxygen in northern temperate lakes over scales ranging from minutes to days. Aquat. Biol. 9, 193–202. doi: 10.3354/ab00249
Liikanen, A., et al. (2002). Effects of temperature and oxygen availability on greenhouse gas and nutrient dynamics in sediment of a eutrophic mid-boreal lake. Biogeochemistry 59, 269–286. doi: 10.1023/a:1016015526712
Liu, B., Zhang, X., Tong, Y., Ao, W., Wang, Z., Zhu, S., et al. (2023). Quantification of nutrient fluxes from sediments of Lake Hulun, China: implications for plateau Lake management. Sustain. For. 15:8680. doi: 10.3390/su15118680
Lonergan, D. J., Jenter, H. L., Coates, J. D., Phillips, E. J., Schmidt, T. M., and Lovley, D. R. (1996). Phylogenetic analysis of dissimilatory Fe(III)-reducing bacteria. J. Bacteriol. 178, 2402–2408. doi: 10.1128/jb.178.8.2402-2408.1996
Lovley, D. R. (1995). Microbial reduction of iron, manganese, and other metals. Adv. Agron., 175–231. doi: 10.1016/s0065-2113(08)60900-1
Maberly, S. C., Pitt, J.-A., Davies, P. S., and Carvalho, L. (2020). Nitrogen and phosphorus limitation and the Management of Small Productive Lakes. Inland Waters 10, 159–172. doi: 10.1080/20442041.2020.1714384
Meis, S., Spears, B. M., Maberly, S. C., O’Malley, M. B., and Perkins, R. G. (2012). Sediment amendment with Phoslock® in Clatto reservoir (Dundee, UK): investigating changes in sediment elemental composition and phosphorus fractionation. J. Environ. Manag. 93, 185–193. doi: 10.1016/j.jenvman.2011.09.015
Melton, E. D., Swanner, E. D., Behrens, S., Schmidt, C., and Kappler, A. (2014). The interplay of microbially mediated and abiotic reactions in the biogeochemical Fe cycle. Nat. Rev. Microbiol. 12, 797–808. doi: 10.1038/nrmicro3347
Molot, L. A., Watson, S. B., Creed, I. F., Trick, C. G., McCabe, S. K., Verschoor, M. J., et al. (2014). A novel model for Cyanobacteria bloom formation: the critical role of anoxia and ferrous Iron. Freshw. Biol. 59, 1323–1340. doi: 10.1111/fwb.12334
Nõges, T. T., et al. (2004). The impact of changes in nutrient loading on cyanobacterial dominance in Lake Peipsi (Estonia/ Russia). Arch. Hydrobiol. 160, 261–279. doi: 10.1127/0003-9136/2004/0160-0261
Nünberg, G. K. (1994). Phosphorus release from anoxic sediments: what we know and how we can deal with it. Limnetica 10, 1–4. doi: 10.23818/limn.10.01
Nürnberg, G. K. (1985). Availability of phosphorus upwelling from iron-rich anoxic hypolimnia. Arch. Hydrobiol. 104, 459–476. doi: 10.1127/archiv-hydrobiol/104/1985/459
Nürnberg, G. K. (2009). Assessing internal phosphorus load – problems to be solved. Lake Reserv. Manag. 25, 419–432. doi: 10.1080/00357520903458848
Osaka, K.'i., Yokoyama, R., Ishibashi, T., and Goto, N. (2021). Effect of dissolved oxygen on nitrogen and phosphorus fluxes from lake sediments and their thresholds based on incubation using a simple and stable dissolved oxygen control method. Limnol. Oceanogr. Methods 20, 1–14. doi: 10.1002/lom3.10466
Padisák, J., and Reynolds, C. S. (2003). Shallow Lakes: the absolute, the relative, the functional and the pragmatic. Hydrobiologia 506-509, 1–11. doi: 10.1023/b:hydr.0000008630.49527.29
Paerl, H. W. (2009). Controlling eutrophication along the freshwater–marine continuum: dual nutrient (N and p) reductions are essential. Estuar. Coasts 32, 593–601. doi: 10.1007/s12237-009-9158-8
Schilling, K., Borch, T., Rhoades, C. C., and Pallud, C. E. (2018). Temperature sensitivity of microbial Fe(III) reduction kinetics in subalpine wetland soils. Biogeochemistry 142, 19–35. doi: 10.1007/s10533-018-0520-4
Søndergaard, M., Bjerring, R., and Jeppesen, E. (2012). Persistent internal phosphorus loading during summer in shallow eutrophic lakes. Hydrobiologia 710, 95–107. doi: 10.1007/s10750-012-1091-3
Søndergaard, M., Nielsen, A., Johansson, L. S., and Davidson, T. A. (2023). Temporarily summer-Stratified Lakes are common: profile data from 436 lakes in lowland Denmark. Inland Waters 13, 153–166. doi: 10.1080/20442041.2023.2203060
Sprague, T., and Prenger-Berninghoff, K. (2019). “Introduction to California cases” in Building Resilience and Planning for Extreme Water-Related Events. ed. R. C. Brears (Cham, Switzerland: Palgrave Macmillan).
Stillwater Sciences (2022). “Lake Henshaw and Lake Wohlford harmful algal blooms management and mitigation plan,” in Technical report prepared by Stillwater Sciences. Berkeley, California for Vista Irrigation District, Vista, California and City of Escondido, California.
Stillwater Sciences (2023). “Phosphorus fractionation in Lake Henshaw sediments to inform phosphorus sequestration as a management strategy for harmful algal blooms,” in Technical memorandum prepared by Stillwater Sciences. (Berkeley, California for Vista Irrigation District, Vista, California and City of Escondido, California).
Thornton, K. W., Kimmel, B. L., and Payne, F. E. (1990). Reservoir limnology: ecological perspectives. New York: Wiley.
Van Duin, E. H. S., and Lijklema, L. (1989). Modelling photosynthesis and oxygen in a shallow, hypertrophic Lake. Ecol. Model. 45, 243–260. doi: 10.1016/0304-3800(89)90074-4
van Luijn, F., Boers, P. C. M., Lijklema, L., and Sweerts, J. P. R. A. (1999). Nitrogen fluxes and processes in sandy and muddy sediments from a shallow eutrophic Lake. Water Res. 33, 33–42. doi: 10.1016/s0043-1354(98)00201-2
Vanni, M. J., Renwick, W. H., Bowling, A. M., Horgan, M. J., and Christian, A. D. (2011). Nutrient stoichiometry of linked catchment-lake systems along a gradient of land use. Freshw. Biol. 56, 791–811. doi: 10.1111/j.1365-2427.2010.02436.x
Wagner, N. D., Quach, E., Buscho, S., Ricciardelli, A., Kannan, A., Naung, S. W., et al. (2021). Nitrogen form, concentration, and micronutrient availability affect microcystin production in cyanobacterial blooms. Harmful Algae 103:102002. doi: 10.1016/j.hal.2021.102002
Wang, J., Chen, J., Guo, J., Sun, Q., and Yang, H. (2018). Combined FE/P and Fe/S ratios as a practicable index for estimating the release potential of internal-P in freshwater sediment. Environ. Sci. Pollut. Res. 25, 10740–10751. doi: 10.1007/s11356-018-1373-z
Watson, S. B., Whitton, B. A., Higgins, S. N., Paerl, H. W., Brooks, B. W., and Wehr, J. D. (2015). “Chapter 20—harmful algal blooms” in Freshwater algae of North America: ecology and classification. eds. J. D. Wehr, R. G. Sheath, and J. Patrick Kociolek Massachusetts: Elsevier).
Welch, E. B., and Dennis Cooke, G. (2005). Internal phosphorus loading in shallow lakes: importance and control. Lake Reserv. Manag. 21, 209–217. doi: 10.1080/07438140509354430
Wu, Y., Wen, Y., Zhou, J., and Wu, Y. (2013). Phosphorus release from lake sediments: effects of ph, temperature and dissolved oxygen. KSCE J. Civ. Eng. 18, 323–329. doi: 10.1007/s12205-014-0192-0
Zamparas, M., and Zacharias, I. (2014). Restoration of eutrophic freshwater by managing internal nutrient loads. A review. Sci. Total Environ. 496, 551–562. doi: 10.1016/j.scitotenv.2014.07.076
Keywords: harmful algal blooms, internal nutrient loading, ammonia, phosphorus, iron
Citation: Defeo S, Beutel MW, Rodal-Morales N and Singer M (2024) Sediment release of nutrients and metals from two contrasting eutrophic California reservoirs under oxic, hypoxic and anoxic conditions. Front. Water. 6:1474057. doi: 10.3389/frwa.2024.1474057
Edited by:
Yang Song, University of Michigan, United StatesReviewed by:
Jeffrey Cornwell, University of Maryland, United StatesRenjian Li, University of California, Santa Barbara, United States
Copyright © 2024 Defeo, Beutel, Rodal-Morales and Singer. This is an open-access article distributed under the terms of the Creative Commons Attribution License (CC BY). The use, distribution or reproduction in other forums is permitted, provided the original author(s) and the copyright owner(s) are credited and that the original publication in this journal is cited, in accordance with accepted academic practice. No use, distribution or reproduction is permitted which does not comply with these terms.
*Correspondence: Shelby Defeo, c2RlZmVvQHVjbWVyY2VkLmVkdQ==