- 1Washington University School of Medicine, Saint Louis, MO, United States
- 2Airway & UC Fit Digital Health–Exercise Physiology Research Laboratory, David Geffen School of Medicine, University of California Los Angeles, Los Angeles, CA, United States
- 3Chobanian & Avedisian School of Medicine, Boston University, Boston, MA, United States
- 4Hackensack Meridian School of Medicine, Nutley, NJ, United States
- 5Northwell Orthopedics, New Hyde Park, NY, United States
While there has been a recent onslaught of traditional lab-based fitness measures in immersive virtual reality (IVR) exergaming research, there remains a paucity in the field-based fitness domain, which refers to assessments made outside a formal laboratory setting which are easier, cheaper, and have more practical application. This study aimed to assess changes in field-based fitness tests including the 1-mile run, 20-m dash, multiple single-leg hop-stabilization test, Abalakov jump, and 5-10-5 Pro Agility test during a 1-month workout protocol and to compare differences between groups assigned to either an IVR machine-directed exergaming platform or a traditional, self-directed cable-resistance training control (SELF). Eighteen (7 females) college-aged participants with little resistance training experience were randomized to IVR or SELF and worked out thrice weekly for 4 weeks (12 sessions). Wilcoxon rank-sum tests were performed for continuous variables to assess significance. Compared to SELF, the IVR group had significantly better performance improvements in 20 m dash (−0.1s vs. 0.0s, p = 0.022), 5-10-5 Pro Agility Test (−0.1s vs. −0.0s, p = 0.003), Abalakov Jump (5.8 cm vs. 2.0 cm, p = 0.0013), 1-Mile Run (−11.0s vs. −2.0s, p = 0.008), and Multiple Single-Leg Hop-Stabilization Test with their dominant (−9.0s vs. 1.0s, p = 0.0015) and non-dominant (−8.0s vs. 1.0s, p = 0.003) legs. This training study demonstrates that IVR exergaming, more so than those that traditionally resistance train (SELF), can improve many field-based fitness components including agility, balance and stability, speed/acceleration, cardiovascular endurance, and lower-body power.
1 Introduction
Exergaming, the gamification of exercise, has notably increased in popularity over the last decade and is a credible solution in motivating sedentary individuals to become more active (Warburton et al., 2007). Exergames, especially in conjunction with immersive virtual reality (IVR), aim to attenuate perceived exertion while maximizing exercise enjoyment, all while increasing motivation during the workout (Bonetti et al., 2010; Bronner et al., 2016; McClure and Schofield, 2020). Prior studies within this laboratory have shown the efficacy of IVR exergaming within both the commercial and home fitness sectors in young, apparently healthy populations (18–35 years) (Hu et al., 2021; Gomez et al., 2022; Mologne et al., 2022). Hu et al. showed that even a 30-min workout with the IVR exergame platform provided a highly intense workout, with mean average heart rate of 176 bpm, a mean max heart rate of 188 bpm, and a mean of 12.9 METs per session, without high levels of perceived exertion (Hu et al., 2021). A subsequent study revealed that the IVR exergame platform elicited myoelectric activity on par with traditional cable resistance training (Gomez et al., 2022). Most recently, during a 12-week randomized control training trial using the IVR exergaming platform against a volume-matched traditional resistance training control group, favorable adaptations in body composition, cardiometabolic measures, and muscular strength and endurance were heightened in the IVR group (Mologne et al., 2022).
Laboratory testing is common within the field of sport and exercise physiology. An effective lab testing program can identify an individual’s strengths and weaknesses, which allows for a more tailored and accurate approach in program design for achieving their fitness goals (Dolezal et al., 1997). Moreso, it can help an individual understand the physiology behind their improvements. However, lab testing is not always feasible since they are not accessible, require trained examiners using expensive equipment and interpretation of the data is left to the expert. Field-based fitness measures, however, are an excellent alternative due to low economic cost, ease of execution, absence of sophisticated technical equipment, and minimal time required to conduct them (Ruiz et al., 2011; Grant et al., 2014; Raghuveer et al., 2020; Prieto-González, 2022). These measures are common throughout the field of exercise physiology and are especially useful in an athletic environment (Hammami et al., 2022; Ben Ayed et al., 2023). Moreover, the benefits of using field-based measures can be seen in its wide adoption in a variety of organizations, such as the Presidential Youth Fitness Program, the United States Military, and the United States Police Force (“Army Combat Fitness Test, 2024; Police Officer Training - Preparing for the Physical Abilities Test,” 2016; Presidential Youth Fitness Program, 2024”). A more recent development in research regarding field-based fitness measures is the use of novel adaptive resistance mechanisms that alter a user’s resistance to maximize tension within a set. This has been shown to improve peak power and jump height in collegiate basketball players, and is a unique component of various exergaming platforms (Mologne et al., 2022; Yamamoto et al., 2023).
While the effects of this study’s IVR exergaming platform have been studied within the realm of laboratory-based fitness and performance measures, the effects on field-based fitness measures are lacking (Hu et al., 2021; Viana et al., 2021; Gomez et al., 2022; Mologne et al., 2022). The few prior studies that have been conducted show improvements in agility, balance, and speed as a result of exergaming interventions in populations such as the elderly, those with cognitive disabilities, and those with cancer diagnoses (Smits-Engelsman et al., 2017; de Oliveira et al., 2020; Peng et al., 2020). Smits-Engelsman and others saw significant improvements in functional strength, anaerobic fitness, and balance in children with Developmental Condition Disorder when training with a Nintendo Wii (Smits-Engelsman et al., 2017). Using a game on the Xbox Kinect, de Oliveira et al. found that use of an exergaming intervention led to significant improvements in muscle fatigue, pain, and function in cancer patients (de Oliveira et al., 2020). Finally, Peng et al. saw that an exergaming mat improved flexibility, strength, agility, and balance in elderly participants, which ultimately decreased their risk of falling (Peng et al., 2020). Yet, there remains no studies in which basic field-based fitness metrics are assessed in the general population after interventions with exergaming systems using IVR technology.
Several proposed mechanisms explaining improvements in physical performance attributed to exergaming have been posited. Fully-immersive virtual reality exergaming, specifically with a user donning a head-mounted- display (HMD), has been found to significantly enhance a user’s presence, motivation, and performance in exergaming requiring proprioceptive body movements (Born et al., 2019). Furthermore, an additive component that may enhance motivation, enjoyment, and exercise intensity is the presence of competition. The presence of a simulated competitor has been suggested to increase exercise intensity through increasing intrinsic motivation and, consequently, effort (Farrow et al., 2019). The exergaming platform used in the present study incorporates these elements through the use of fully-immersive virtual reality delivered via a HMD and simulated competitor throughout the duration of the exergame. Therefore, we propose that physical performance improvements will occur as a result of these components that enhance immersion, motivation, and effort.
This study aimed to assess changes in field-based fitness tests including the 1-mile run, 20-m dash, multiple single-leg hop-stabilization test, Abalakov jump, and 5-10-5 Pro Agility test during a 1-month workout protocol and to compare differences between groups assigned to either an IVR machine-directed exergaming platform or a self-directed conventional resistance training control. We hypothesize that there will be greater improvements in field-based fitness metrics after 1 month of IVR versus the conventional resistance training control.
2 Materials and methods
2.1 Participants
Eighteen participants at the University of California at Los-Angeles (UCLA) and the surrounding community met the following inclusion criteria: i) apparently healthy men and women, ii) 18–35 years of age, and iii) history of resistance training <4 workouts/monthly the past 6 months. Exclusion criteria included: i) significant medical diagnoses, including cardiovascular, pulmonary, musculoskeletal, or metabolic disorders that may limit the ability to exercise or increase the cardiovascular risk of exercising, and ii) use of any drug or supplement known to enhance anabolic responses. All volunteers completed a pre-participation physical activity readiness questionnaire (PAR-Q) and an exercise history questionnaire. The UCLA Institutional Review Board reviewed and approved the study, and all participants gave their written informed consent.
2.2 Study design
This was a 4-week, single-blinded, randomized control trial using a parallel research design. Sedentary college-aged participants were randomly placed 1:1 into one of two groups: the intervention, IVR machine-directed training (IVR) or the control, self-directed conventional resistance training (SELF), by an investigator independent of the recruitment of participants using an online-generated random number program. Allocation was concealed with the use of consecutively numbered envelopes. The participants worked out thrice weekly (i.e., 12 total sessions) for 45–60 min. Participants were asked to refrain from additional vigorous activity for the course of the study (Figure 1).
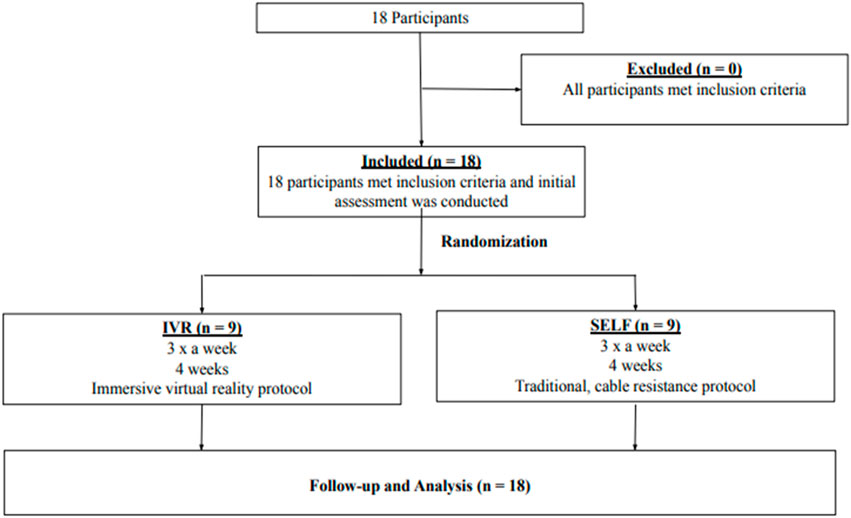
Figure 1. CONSORT diagram demonstrating participant flow throughout the study. IVR machine-directed training (IVR), Intervention; self-directed conventional resistance training (SELF), Control.
2.3 Interventions
Under the direction of the lab director at the UC Fit Digital Health-Exercise Physiology Research Laboratory at UCLA’s David Geffen School of Medicine, trained undergraduate research personnel monitored training and carried out all field-based fitness measurements. Participants were given the autonomy to train at any time three times per week preferably with a rest day between sessions. No controls on dietary intake were in place aside from the prohibition of any dietary supplement or directed weight-altering diet for the duration of the study. All participants were asked to refrain from performing any moderate-high intensity exercise 24 h prior to baseline and post-testing.
2.4 Study groups
2.4.1 Intervention group: BlackBox IVR exergaming (IVR)
The IVR-based cable resistance exergaming platform, Black Box VR, 2024 (Black Box VR®, Boise, ID, USA), employed a servo-based electromagnetic adaptive resistance mechanism (Figure 2). The system operated with a head-mounted-display (HMD) (Vive, Taipei, Taiwan), an automated support pad, and pair of resistance handles that adjust up and down on articulating carriages to automatically align at the correct height for all cable resistance exercises. The system’s HMD and wrist-worn sensors processed in-game data instantaneously to ensure synchronization between the user’s actions and the IVR gameplay. An in-game image of the virtual reality platform can be seen in Supplementary Image 1.
Copyrights owned by BlackBox Virtual Reality. Permission of use was acquired.
Per the company’s website, the exergame was akin to a typical, two-lane tower defense where the user’s goal was to defend their virtual crystal by fighting enemy units while simultaneously trying to destroy the opponent’s crystal. The execution of six cable resistance exercises corresponded to in-game attacks that could be used for offensive or defensive purposes. The damage delivered for each exercise (i.e., lat-pulldown, standing chest press, standing row, overhead press, stiff-leg deadlift, and squat) was dependent on the cable resistance level. Per the manufacturer, the exergame system’s proprietary software adjusted the resistance when 12–14 repetitions were completed per set at 60%–70% of the users automatically calculated the predicted one-rep maximum. These exercises were associated with an elemental category, such as water, fire, and air, allowing for each user to strategize which movements were optimal to perform against certain enemies (i.e., water attack in response to an enemy’s fire hero being deployed). In-game “heroes” designed to combat enemy units and damage the opponent’s crystal could be deployed by performing a sequence of hand movements. The gameplay strategy (i.e., exercise sequence, repetitions, sets) was entirely user dependent, which equated to training volumes that varied across participants.
2.4.2 Control group: self-directed (SELF)
It should be noted that during the IVR exergaming session, each study participant freely chooses their exercise, exercise sequence, and ultimately the training volume. Because of this, these variables (i.e., exercise choice and sequence, sets, repetitions, and time-to-complete) were computed by the machine’s integrated computer. This computed training program was suggested to participants to attempt to match overall load for control participants for each of the 12 training sessions to prevent training dose–response bias in the outcome measures.
The matched control participants completed their exercises using a cable/pulley-based crossover machine with dual-selectorized weight stacks (Figure 3). Both arms of the machine were spaced the same distance as the IVR machine and could be similarly adjusted vertically. This conventional cable machine allowed participants to complete the same six exercises as the IVR platform in near-identical movement patterns. Using an iPhone app (Airway & UCFit Digital Health-Exercise Physiology Research Laboratory, Los Angeles, CA, United States), participants tracked their sessions and training volume.
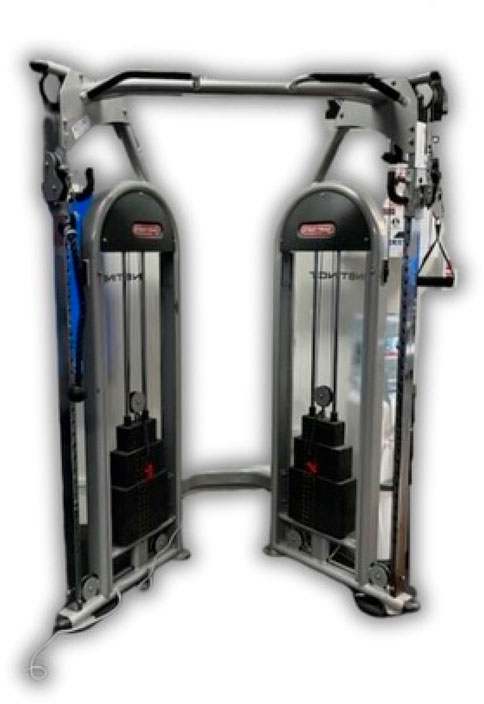
Figure 3. Conventional cable/pulley-based crossover machine with dual-selectorized weight stacks used by SELF group.
2.5 Demographic measures
Anthropometry: Body mass was measured on a calibrated medical scale (accuracy ±0.1 kg), and height was determined using a precision stadiometer (Seca, Hanover, MD, United States; accuracy ±0.01 m). Participants were instructed to remove unnecessary clothing and accessories prior to being weighed, as well as remove their shoes prior to taking their height measurements.
Body Composition: Body fat percentage was measured using a validated octipolar, multi-frequency, multi-segmental bioelectrical impedance analyzer (BIA) (InBody Co., Seoul, Korea Republic) (Dolezal et al., 2013). To ensure accuracy, participants adhered to standard pre-measurement BIA guidelines recommended by the American Society of Exercise Physiologists (Heyward, 2001). Briefly, the test was performed after at least 3 hours of fasting and voiding, with participants instructed to remain hydrated and not exercise 2 h before testing. After investigators explained the procedure, the participant stood upright with their feet on two metallic footpads while holding a handgrip with both hands. The instrument measured resistance and reactance using proprietary algorithms.
2.6 Field-based fitness measures
Five field-based fitness tests were performed on an outdoor 400-m track at UCLA. Participants were instructed to be well rested, not workout 24 h prior, and to come hydrated with running shoes. This test battery followed the sequence below and included a 20 min recovery between each test which resulted in a total test time of under 90 min.
2.6.1 Multiple single-leg hop-stabilization test (MSLHST)
The Multiple Single-Leg Hop-Stabilization Test (MSLHST) is a dynamic and static balance assessment tool. The setup and scoring for the MSLHST followed the protocol set by Sawle et al. (Sawle et al., 2017). Potential test scores were determined by the sum of the balance scores and landing scores, which would range from 0 to 30 and 0-100, respectively. These scores were determined by the errors committed during a given balance or landing period, wherein a lower score would indicate less errors (hence, more balance) and a higher score would indicate more errors. Briefly, standardized verbal instructions were given by a researcher during the MSLHST setup and prior to the allowed practice attempts. The MSLHST required 10 numbered boxes to be set up in a sequenced order with the distance between each of the boxes determined by the participants’ height. Participants were instructed to keep their hands on their iliac crests throughout the duration of the test. Upon starting the test, participants were asked to hop according to the series of boxes in numerical order. A metronome, which represented an auditory cue every second, set the pace of the test. Once landing on each box, participants were instructed to try to remain balanced in this position for a total of 5 seconds, which was counted aloud by the researcher. One practice attempt was given to the participant for familiarity, then the participant tested with both their dominant and non-dominant leg, in random order. Scoring was assessed based on the balance period and the landing period to determine a relative measure of balance.
2.6.2 20-M sprint test
The 20-m sprint test is widely used in sports and fitness to evaluate a person’s linear acceleration and speed (Binnie et al., 2013). Two cones were set up 20 m apart from one another with the first cone being positioned 0.3 m ahead of the starting line, as this method has been recommended by experts in the field (Altmann et al., 2015). All participants were instructed to use a standing split-stance starting position with their dominant leg forward. Participants were counted down ‘3–2 - 1- GO’ and accelerated maximally to the finish line upon hearing the ‘GO’ signal. One timekeeper, using a smartphone timer, was positioned at the finish line to time the sprint. A 3-min recovery period was given between each of the three trials. The average of the three trials was recorded.
2.6.3 5-10-5 Pro Agility test
The 5-10-5 Pro Agility test is used to assess a person’s change of direction (i.e., agility), speed, and leg strength. Moran et al. has a detailed description, however for the sake of brevity, we summarize the key parts of setup below (Moran et al., 2018). A distance of 10 m was measured and bisected with a cone, which represented the test’s starting point. The timing of the test started when the participant began moving to their left or right. The participant was instructed to run 5 m either direction and touch one of the end-line cones before immediately reversing their course to run 10 m to the opposite end. The participant would subsequently touch the opposite ended cone and change direction a final time. The test was concluded after the participant sprinted the 5 m back through the initial starting position (Figure 4). One timekeeper, using a smartphone timer, was positioned at the start/finish cone to time the trial. A 3-min recovery period was given between each of the three trials. The average of the three trials was recorded.
2.6.4 Abalakov Jump
Using a previously validated electronic jump mat (Probotics, Inc., Huntsville, AL, United States) participants were instructed to stand on the mat with their feet at hip-width and perform an Abalakov jump (Leard et al., 2007; Rodríguez-Rosell et al., 2017b). Participants were allowed to prepare for the jump by bending their knees and loading their subsequent arm swing before performing an Abalakov jump for maximal height. The jump height was computed using the computer interfaced with the jump mat. A total of three trials were conducted with rest intervals of 30 s between trials, and the average of the three trials was recorded. Jump height was calculated based on “hang time,” defined as the time s) from the feet leaving the mat to their return and the following equation: Ht = t^2 * 1.227 where t is hang-time in seconds, and 1.227 is a constant derived from the acceleration of gravity (Harman et al., 1991).
2.6.5 1-Mile run
The 1-mile run test measures cardiovascular fitness and the time to completion is correlated with the gold-standard VO2max laboratory assessments, as seen with a Cronbach’s alpha of 0.873 and an R = 0.88 in adults aged 18-25 (Cureton et al., 1995; Trinh, 2019). The 1-mile run was conducted on a university track (UCLA, Los Angeles, CA). Participants ran as quickly as they could to complete four laps, with each lap equaling 400 m while trying to keep a fast, consistent pace. Times were recorded using a hand-timer that started at an athlete’s first movement and ended when they passed the 1,600 m mark, marking the end of their fourth lap (Lunt et al., 2013).
2.7 Statistical analysis
A power analysis was performed using data from Marián et al. (Marián et al., 2016). Using unpublished data from our lab, we deemed an improvement of 1 inch (2.2 cm) to constitute a meaningful difference. A sample size of at least 12 was calculated to achieve a power of 80% with α = 0.05.
Descriptive statistics are presented as the median and interquartile range (IQR). Given the small sample size in each group combined with some distributions’ significant deviation from normality, a non-parametric approach was utilized. Wilcoxon rank-sum tests and signed-rank tests were performed for continuous variables between groups and within groups, respectively. A chi-squared test was executed for the sole categorical variable (sex). The Benjamini–Hochberg procedure was employed to maintain the familywise error rate. Statistical significance was determined by α = 0.05 and all tests were two-tailed. Effect sizes were calculated. All data were exported to IBM SPSS Statistics for Windows, version 22 (IBM Corp., Armonk, N.Y., USA) for analysis.
2.8 Approval
The study was approved on 20 August 2022 by the Institutional Board Review of UCLA with IRB number (11–003190).
3 Results
Anthropometric and field-based fitness test results are presented in Table 1. At baseline, no differences existed between groups in age (median = 20.0 years, interquartile range = 2.0 years; p = 0.489), or sex (n = 7 females total; p = 0.629). Additionally, there were no significant differences in baseline body mass (p = 0.546) nor in body fat percentage (p = 0.340) between the IVR and SELF groups. In both groups, average session time (30 min) was identical and every participant completed all 12 sessions. The IVR group had a non-significant decrease trend in body mass. Both groups experienced decreases in body fat %; however, IVR experienced a significantly greater loss in body fat % compared to the SELF (−1.9 (0.8%) vs. −0.6 (1.2%), p = 0.003).
SELF participants experienced no significant differences between pre-testing and after 4 weeks for the 20m dash, 5-10-5 Pro Agility Test, Abalakov Jump, 1-Mile Run, and MSLHST for both dominant and non-dominant legs. IVR participants experienced significant differences in the 5-10-5 Pro Agility Test, Abalakov Jump, 1-Mile Run, and MSLHST for their non-dominant legs. Although 20 m dash and MSLHST for the dominant leg were not significant, these measurements trended towards improvement in the IVR group.
Compared to SELF, the IVR group had significantly better improvements from baseline to 4 weeks as a result of training in the 20 m Dash (−0.1 (0.1s) vs. 0.0 (0.1s), p = 0.022), 5-10-5 Pro Agility Test (−0.1 (0.2s) vs. 0.0 (0.1s), p = 0.003), the Abalakov Jump (5.8 (3.7 cm) vs. 2.0 (2.0 cm), p = 0.0013), the 1-Mile Run (−11.0 (7.0s) vs. −2.0 (14.0s), p = 0.008), and the MSLHST for dominant (−9.0 (12.0s) vs. 1.0 (5.0s), p = 0.015) and non-dominant legs (−8.0 (11.0s) vs. −1.0 (10.0s), p = 0.003).
Table 1 displays data for both the SELF and IVR groups. Comparison of differences between pre- and post-testing within the group (SELF or IVR) can be seen in the “P-within” column. Comparison of differences between pre-and post-testing between the two groups (SELF vs. IVR) can be seen in the “P-between” column.
4 Discussion
The current study aimed to assess the role of IVR exergaming on a multitude of field-based fitness tests and we believe it is the first to compare results to those that traditionally resistance train. These results showed that 1 month of IVR training compared to the matched control, traditional resistance-trained group was markedly better in improving agility (i.e., 5-10-5 agility test and multiple single-leg hop-stabilization test), balance and stability (i.e., multiple single-leg hop-stabilization test), speed/acceleration (i.e., 20-m sprint test), cardiovascular endurance (i.e., 1-mile time), and lower-body power (i.e., Abalakov jump). Prior studies have been conducted that have assessed only a few of these field-based fitness metrics following an exergaming intervention, with varying results (Smits-Engelsman et al., 2017; de Oliveira et al., 2020; Peng et al., 2020). However, these studies observed different populations than the current study. We posit that observed improvements in field-based fitness measures seen by IVR participants, especially the larger improvements in comparison to the control group, were an outcome of the immersive virtual reality nature of the exergaming system.
More specifically, we posit that these results were a product of a decrease in perceived exertion as well as the adaptive resistance mechanisms within the IVR machine. A benefit of virtual reality within the realm of exercise is that a heightened enjoyment may attenuate perceived exertion, which ultimately pushes individuals to work closer to fatigue (Giakoni-Ramírez et al., 2023). In the past, our laboratory has observed high rates of workout satisfaction in conjunction with significant differences in rates of perceived exertion when comparing IVR exergaming to traditional, cable-based strength training (Hu et al., 2021; Mologne et al., 2022). We believe this to be an integral reason why the IVR group had a heightened response to training, as participants were “distracted” by the gamification and immersion into virtual reality, hence tolerating a higher intensity and pushing themselves closer to full muscle fatigue than the control group.
We also believe the adaptive resistance mechanism of the exergaming platform effectuated strength outcomes in the study participants. Hu et al. and Mologne et al. elaborate on this IVR system, explaining that this system dynamically changes the cable resistance within a set to maximize tension on the user’s muscles. This adaptive intra-set technology pushes users to near failure every set, maximizing strength gains (Hu et al., 2021; Mologne et al., 2022). Furthermore, this adaptive mechanism automatically promotes progressive overload, as the IVR system progresses their load as the user is able to exert more force, even without the user’s awareness. This, in turn, promotes a higher degree of strength, even when control subjects are instructed to lift a similar load. We believe that because this is done without the user’s awareness, participants are more inclined to lift to failure rather than aim for a repetition number, one that may not truly indicate their strength level nor achieve maximal effort. This is seen in the Mologne et al. study, which saw IVR users experience significantly improved upper and lower body strength compared to their traditionally trained counterparts (Mologne et al., 2022). This technology is also being used in other platforms and similar results have been observed in our lab (Yamamoto et al., 2023).
We posit that the combination of heightened enjoyment, attenuated perceived exertion, and the adaptive mechanism of the exergaming system ultimately allowed for a prolonged tolerance to heavier resistance loads in the IVR group compared to the control. In doing so, we believe these components subsequently lead to an increase in strength manifested as improvements in field-based fitness metrics.
4.1 Agility
IVR participants experienced increased agility and change-of-direction (COD), as noted by a median decrease of 0.1s in the 5-10-5 m test. We posit that this is likely a result of increased lower body strength. Our prior study shows that IVR elicits greater strength gains for both squat and deadlift than conventional cable resistance training despite IVR participants having significantly lower rates of perceived exertion compared to the control (Mologne et al., 2022). As aforementioned, we believe that user’s attenuated perceived exertion and the exergaming platforms unique algorithm optimize training to “near failure” and prior research has shown that near-failure training can elicit muscle activation similar to complete failure, while still minimizing injury (Santanielo et al., 2020). We believe that it is the synergistic qualities of immersive virtual reality and the adaptive algorithm that therefore play a role in why the IVR group experienced heightened strength results, and subsequently, significantly greater results in other field-based metrics such as agility.
As such, increased agility is often associated with increased lower limb strength, especially among competitive athletes (Hammami et al., 2018; Sonoda et al., 2018). In a recent review article, Suchomel and others observed that 35 studies, 78% of the total studied, noted a moderate or greater relationship between muscular strength and COD ability, with 27 (60%) showing at least a large relationship (Suchomel et al., 2016). More specifically, resistance training has been shown to be one of the most effective ways to decrease time in the 5-10-5 Agility Test (Forster et al., 2022). Exergaming may have a role as well. A study by Smits-Engelsman et al. displayed that children, both with and without developmental coordination disorder, improved in their agility, as tested by the 10-step stair test, after completing an exergaming intervention with the Nintendo Wii Fit (Smits-Engelsman et al., 2017) However, as noted in the cited Suchomel review, there are significant limitations to COD testing (Suchomel et al., 2016). This may attenuate the actual effects that the immersive virtual reality exergaming system had on participants’ agility and may be more noticeable in activities like sports themselves rather than metric tests.
4.2 Balance/stability
The IVR participants of this study had significantly improved balance, as noted by improvements in the MSLHST for both their dominant and non-dominant legs, after a month of exergaming intervention. This was not observed in the SELF group. One explanation for the increase in balance may once again be a result of an increase in lower-body strength that is elicited from the exergaming system (Mologne et al., 2022). A recent meta-analysis and systematic review by Muehlbauer et al. found that there are associations between lower body strength and balance (Muehlbauer et al., 2015). It is plausible that the improvements in balance seen in the IVR were a result of increased lower-body strength.
Another possible explanation for this increase in balance is the use of immersive virtual reality in training. While often in settings of neurological deficit and rehabilitation, IVR has been shown to be beneficial in improving patient balance (Mao et al., 2014). Additionally, recent systematic reviews have shown that virtual reality-based training enhanced functional balance in elderly individuals compared to other traditional protocols (Sadeghi et al., 2021; Liu et al., 2022). While the participants of the current study did not have conditions similar to the aforementioned studies, their proprioception was still trained with the IVR components during each session, which is a critical part of balance (Pan et al., 2011). That is, virtual reality enabled participants to train both their muscles and their proprioception simultaneously.
4.3 Speed/acceleration
Participants in the IVR group experienced significantly improved 20 m dash times compared to the SELF group, indicating an increase in speed and acceleration. It has been well-documented that muscular strength, especially with the lower body, plays a large role in power generation and sprint speed (Delecluse, 1997; Cronin and Hansen, 2005; Comfort et al., 2012; Rodríguez-Rosell et al., 2017a; Yáñez-García et al., 2022). A systematic review and meta-analysis by Seitz and others note that not only is sprint speed associated with lower body strength increase, but also that there is a large correlation between squat strength effect size and sprint effect size (Seitz et al., 2014). However, it must be noted that sprinting is a very complex movement and that while muscular strength was improved, sprinting is unique in that there are other factors that contribute to sprint speed other than force production, such as stride frequency and the technical aspect of running (Lockie et al., 2011). With a lack of translatable functionality between the in-game movements of the IVR system and high-intensity sprinting, little improvement in this measure was observed compared to no changes observed in SELF.
4.4 Cardiovascular endurance
Both the IVR and SELF groups experienced improvements in their 1-Mile Run time, with the IVR group’s decrease in time being significantly more (−11.0s vs. −2.0s, p = 0.008). This improvement is likely to be the result of improved cardiometabolic metrics as well as improved muscular strength and endurance. In a recent systematic review and meta-analysis, it was found that generally resistance training not only improved muscular strength in endurance athletes, but also running economy, and had direct improvements in performance for distances between 1,500 and 10,000 m (Alcaraz-Ibañez and Rodríguez-Pérez, 2018). This is generally observed across scientific literature - resistance training is beneficial for endurance performance (Balsalobre-Fernández et al., 2016; Blagrove et al., 2018).
The difference between groups might be due to improved VO2max elicited by the IVR exergaming protocol. In a prior study conducted by our laboratory, we also observed that training with an IVR system improved VO2max and rVO2max, which were likely a result of improved cardiovascular adaptations and muscle mass, respectively (Cornelissen et al., 2011; Ozaki et al., 2013; Mologne et al., 2022). This improvement in VO2max is also posited to be a result of the gamification in the IVR exergaming system, as participants are engaging in other movements during their “rest” period; yet, participants may not even realize they are still exerting themselves when doing so because of this immersive component. VO2max may account for up to 81.3% of the variance in endurance performance, as an athlete’s VO2 max sets their upper limit for endurance performance and an athlete cannot operate at higher than 100% of their VO2max for sustained times (Bassett and Howley, 2000; McLaughlin et al., 2010). It is probable that IVR participants’ improved performance in the 1-mile run as a result of an increased VO2max in addition to the muscular adaptations, and a more notable decrease in time may also be seen with a larger training period.
4.5 Power
Lastly, while both SELF and IVR groups were observed to have significantly improved power as noted by their improvements in the Abalakov jump, the IVR group had a significantly higher improvement (5.8 cm vs. 2.0 cm, p = 0.0013). We posit that this is once more a product of the significantly increased lower body strength that is a result of the exergaming system (Mologne et al., 2022). 1-RM maxes in squats, especially in proportion to body weight, have been shown to be significantly correlated with countermovement jump (Nuzzo et al., 2008). Similarly, in a systematic review and meta-analysis, it was shown that 78% of studies found at least a moderate relationship between muscular strength and jump height (Suchomel et al., 2016). It is very plausible that the improved height is a result of increased lower body strength.
4.6 Limitations
This study is not without limitations. First, this study had a small sample size, which may cause a potential Type 2 error as a result of being underpowered. The low sample size may have been influenced by outliers or other significant results that may not have been observed. Similarly, the study’s population only included sedentary college individuals and it is unknown if the same results would be seen in a more active population or a different age demographic. The study was limited to only 4 weeks of training - a longer training protocol may have induced more notable changes than experienced in 4 weeks. Another limitation of this study is the use of hand-timers, which has been shown to possible underreport sprint times (Mann et al., 2015). This may have caused increased variability in hand-recorded times. Lastly, as aforementioned, some tests to assess field-based fitness, such as change of direction tests, may not be completely indicative of what they are testing. Decreases in change of direction test times may be underestimated as a result of increased strength as most tests primarily test one measurement of strength, while change of direction has been shown to be a product of eccentric, concentric, dynamic, and isometric strength simultaneously (Spiteri et al., 2014; Suchomel et al., 2016). Despite this, the 5-10-5 Pro Agility test is still a widely validated metric of change of direction with high test-retest reliability and correlation to other change of direction tests, which is why it was used in the present study (Stewart et al., 2014).
5 Conclusion
While lab measures are common within the field of sport and exercise physiology, field-based metrics are just as imperative. They are cheap and easy to conduct, while displaying tangible improvements to individuals, whether for athletic competition training or for general health. They also offer a similar understanding of physiology behind fitness improvements as lab tests do. Given the shown benefits and wide adoption of field-based fitness measures within society, it is important to study them within new exercise modalities, such as IVR exergaming. This training study demonstrates that an immersive virtual reality exergaming platform, more so than those that traditionally resistance train, improve many field-based fitness components including agility, balance and stability, speed/acceleration, cardiovascular endurance, and lower-body power. These findings, coupled with our previous studies using this specific IVR exergaming platform that showed large improvements in upper and lower body muscular strength and endurance, highlight the potential benefits of using immersive virtual reality as a fun and engaging exercise modality.
Data availability statement
The raw data supporting the conclusions of this article will be made available by the authors, without undue reservation.
Ethics statement
The studies involving humans were approved by Institutional Board Review of UCLA with IRB number (11-003190). The studies were conducted in accordance with the local legislation and institutional requirements. The participants provided their written informed consent to participate in this study.
Author contributions
MM: Conceptualization, Methodology, Writing–original draft. TY: Conceptualization, Data curation, Methodology, Writing–original draft. MV: Conceptualization, Methodology, Writing–original draft. AB: Conceptualization, Data curation, Methodology, Writing–review and editing. RL: Data curation, Methodology, Writing–review and editing. TN: Conceptualization, Data curation, Methodology, Writing–review and editing. AD: Conceptualization, Data curation, Methodology, Writing–review and editing. JF: Conceptualization, Data curation, Methodology, Writing–review and editing. EN: Formal Analysis, Software, Writing–review and editing. BD: Conceptualization, Investigation, Methodology, Project administration, Supervision, Writing–review and editing.
Funding
The author(s) declare that no financial support was received for the research, authorship, and/or publication of this article.
Conflict of interest
The authors declare that the research was conducted in the absence of any commercial or financial relationships that could be construed as a potential conflict of interest.
Publisher’s note
All claims expressed in this article are solely those of the authors and do not necessarily represent those of their affiliated organizations, or those of the publisher, the editors and the reviewers. Any product that may be evaluated in this article, or claim that may be made by its manufacturer, is not guaranteed or endorsed by the publisher.
References
Alcaraz-Ibañez, M., and Rodríguez-Pérez, M. (2018). Effects of resistance training on performance in previously trained endurance runners: a systematic review. J. Sports Sci. 36, 613–629. doi:10.1080/02640414.2017.1326618
Altmann, S., Hoffmann, M., Kurz, G., Neumann, R., Woll, A., and Haertel, S. (2015). Different starting distances affect 5-m sprint times. J. Strength Cond. Res. 29, 2361–2366. doi:10.1519/JSC.0000000000000865
Army Combat Fitness Test (2024). Army combat fitness test [WWW document], army combat Fit. Test. Available at: https://www.army.mil/acft (Accessed January 21, 24).
Balsalobre-Fernández, C., Santos-Concejero, J., and Grivas, G. V. (2016). Effects of strength training on running economy in highly trained runners: a systematic review with meta-analysis of controlled trials. J. Strength Cond. Res. 30, 2361–2368. doi:10.1519/JSC.0000000000001316
Bassett, D. R., and Howley, E. T. (2000). Limiting factors for maximum oxygen uptake and determinants of endurance performance. Med. Sci. Sports Exerc. 32, 70–84. doi:10.1097/00005768-200001000-00012
Ben Ayed, K., Hammami, R., Gene-Morales, J., Ajailia, A., Werfelli, H., Rebai, H., et al. (2023). High-intensity forward-backward plyometrics after the warm-up entail better sprint and change-of-direction performance than low-intensity side-to-side plyometrics. Mot. control 28, 63–77. doi:10.1123/mc.2023-0050
Binnie, M. J., Peeling, P., Pinnington, H., Landers, G., and Dawson, B. (2013). Effect of surface-specific training on 20-m sprint performance on sand and grass surfaces. J. Strength Cond. Res. 27, 3515–3520. doi:10.1519/JSC.0b013e31828f043f
Black Box VR (2024). Fitness - immersive Gym and gamified fitness experience [WWW document]. Available at: https://www.blackbox-vr.com/(Accessed January 21, 24).
Blagrove, R. C., Howatson, G., and Hayes, P. R. (2018). Effects of strength training on the physiological determinants of middle- and long-distance running performance: a systematic review. Sports Med. Auckl. N. Z. 48, 1117–1149. doi:10.1007/s40279-017-0835-7
Bonetti, A. J., Drury, D. G., Danoff, J. V., and Miller, T. A. (2010). Comparison of acute exercise responses between conventional video gaming and isometric resistance exergaming. J. Strength Cond. Res. 24, 1799–1803. doi:10.1519/JSC.0b013e3181bab4a8
Born, F., Abramowski, S., and Masuch, M. (2019). Exergaming in VR: the impact of immersive embodiment on motivation, performance, and perceived exertion. IEEE. doi:10.1109/VS-Games.2019.8864579
Bronner, S., Pinsker, R., Naik, R., and Noah, J. A. (2016). Physiological and psychophysiological responses to an exer-game training protocol. J. Sci. Med. Sport 19, 267–271. doi:10.1016/j.jsams.2015.03.003
Comfort, P., Bullock, N., and Pearson, S. J. (2012). A comparison of maximal squat strength and 5-10-and 20-meter sprint times, in athletes and recreationally trained men. J. Strength Cond. Res. 26, 937–940. doi:10.1519/JSC.0b013e31822e5889
Cornelissen, V. A., Fagard, R. H., Coeckelberghs, E., and Vanhees, L. (2011). Impact of resistance training on blood pressure and other cardiovascular risk factors: a meta-analysis of randomized, controlled trials. Hypertens. Dallas Tex 58, 950–958. doi:10.1161/HYPERTENSIONAHA.111.177071
Cronin, J. B., and Hansen, K. T. (2005). Strength and power predictors of sports speed. J. Strength Cond. Res. 19, 349–357. doi:10.1519/14323.1
Cureton, K. J., Sloniger, M. A., O’Bannon, J. P., Black, D. M., and McCormack, W. P. (1995). A generalized equation for prediction of &OV0312;O2peak from 1-mile run/walk performance. Med. Sci. Sports Exerc. 27, 445–451. doi:10.1249/00005768-199503000-00023
Delecluse, C. (1997). Influence of strength training on sprint running performance. Current findings and implications for training. Sports Med. Auckl. N. Z. 24, 147–156. doi:10.2165/00007256-199724030-00001
de Oliveira, P. F., Alves, R. da S., Iunes, D. H., de Carvalho, J. M., Borges, J. B. C., Menezes, F. da S., et al. (2020). Effect of exergaming on muscle strength, pain, and functionality of shoulders in cancer patients. Games Health J. 9, 297–303. doi:10.1089/g4h.2019.0113
Dolezal, B., Lau, M. J., Abrazado, M., Storer, T., and Cooper, C. (2013). Validity of two commercial grade bioelectrical impedance analyzers for measurement of body fat percentage. J. Exerc. Physiol. Online 16, 74–83.
Dolezal, B. A., Thompson, C. J., Schroeder, C. A., Haub, M. D., Haff, G. G., Comeau, M. J., et al. (1997). Laboratory testing to improve athletic performance. J 19, 20. doi:10.1519/1073-6840(1997)019<0020:lttiap>2.3.co;2
Farrow, M., Lutteroth, C., Rouse, P. C., and Bilzon, J. L. J. (2019). Virtual-reality exergaming improves performance during high-intensity interval training. Eur. J. Sport Sci. 19 (6), 719–727. doi:10.1080/17461391.2018.1542459
Forster, J. W. D., Uthoff, A. M., Rumpf, M. C., and Cronin, J. B. (2022). Training to improve pro-agility performance: a systematic review. J. Hum. Kinet. 85, 35–51. doi:10.2478/hukin-2022-0108
Giakoni-Ramírez, F., Godoy-Cumillaf, A., Espoz-Lazo, S., Duclos-Bastias, D., and Del Val Martín, P. (2023). Physical activity in immersive virtual reality: a scoping review. Healthc. Basel. 11 (11), 1553. doi:10.3390/healthcare11111553
Gomez, D., Browne, J., Almalouhi, A., Abundex, M., Hu, J., Nason, S., et al. (2022). Muscle activity during immersive virtual reality exergaming incorporating an adaptive cable resistance system. Int. J. Exerc. Sci. 15, 261–275.
Grant, C., Janse van Rensburg, D., Pepper, M., du Toit, P., Wood, P., Ker, J., et al. (2014). The correlation between the health-related fitness of healthy participants measured at home as opposed to fitness measured by sport scientists in a laboratory. South Afr. Fam. Pract. 56, 235–239. doi:10.1080/20786190.2014.953888
Hammami, M., Negra, Y., Billaut, F., Hermassi, S., Shephard, R. J., and Chelly, M. S. (2018). Effects of lower-limb strength training on agility, repeated sprinting with changes of direction, leg peak power, and neuromuscular adaptations of soccer players. J. Strength Cond. Res. 32, 37–47. doi:10.1519/JSC.0000000000001813
Hammami, R., Gene-Morales, J., Nebigh, A., Rebai, H., and Colado, J. C. (2022). Speed improves with eccentric hamstring training in athletes of different maturity status. Pediatr. Exerc Sci. 34 (2), 99–107. doi:10.1123/pes.2021-0080
Harman, E. A., Rosenstein, M. T., Frykman, P. N., Rosenstein, R. M., and Kraemer, W. J. (1991). Estimation of human power output from vertical jump. J. Strength Cond. Res. 5, 116–120. doi:10.1519/00124278-199108000-00002
Heyward, V. (2001). Body composition ASEP METHODS RECOMMENDATION: BODY COMPOSITION ASSESSMENT. JEPonline J. Exerc. Physiologyonline Official J. Am. Soc. Exerc. Physiologists (ASEP) Int. Electron. J. 4. https://www.asep.org/asep/asep/HeywardFinal.pdf.
Hu, J., Browne, J. D., Arnold, M. T., Robinson, A., Heacock, M. F., Ku, R., et al. (2021). Physiological and metabolic requirements, and user-perceived exertion of immersive virtual reality exergaming incorporating an adaptive cable resistance system: an exploratory study. Games Health J. 10, 361–369. doi:10.1089/g4h.2021.0031
Leard, J. S., Cirillo, M. A., Katsnelson, E., Kimiatek, D. A., Miller, T. W., Trebincevic, K., et al. (2007). Validity of two alternative systems for measuring vertical jump height. J. Strength Cond. Res. 21, 1296–1299. doi:10.1519/R-21536.1
Liu, W., Hu, Y., Li, J., and Chang, J. (2022). Effect of virtual reality on balance function in children with cerebral palsy: a systematic review and meta-analysis. Front. Public Health 10, 865474. doi:10.3389/fpubh.2022.865474
Lockie, R. G., Murphy, A. J., Knight, T. J., and Janse de Jonge, X. A. K. (2011). Factors that differentiate acceleration ability in field sport athletes. J. Strength Cond. Res. 25, 2704–2714. doi:10.1519/JSC.0b013e31820d9f17
Lunt, H., Roiz De Sa, D., Roiz De Sa, J., and Allsopp, A. (2013). Validation of one-mile walk equations for the estimation of aerobic fitness in British military personnel under the age of 40 years. Mil. Med. 178, 753–759. doi:10.7205/MILMED-D-12-00369
Mann, J. B., Ivey, P. J., Brechue, W. F., and Mayhew, J. L. (2015). Validity and reliability of hand and electronic timing for 40-yd sprint in college football players. J. Strength Cond. Res. 29, 1509–1514. doi:10.1519/JSC.0000000000000941
Mao, Y., Chen, P., Li, L., and Huang, D. (2014). Virtual reality training improves balance function. Neural Regen. Res. 9, 1628–1634. doi:10.4103/1673-5374.141795
Marián, V., Katarína, L., Dávid, O., Matúš, K., and Simon, W. (2016). Improved maximum strength, vertical jump and sprint performance after 8 Weeks of jump squat training with individualized loads. J. Sports Sci. Med. 15, 492–500.
McClure, C., and Schofield, D. (2020). Running virtual: the effect of virtual reality on exercise. J. Hum. Sport Exerc. 15, 861–870. doi:10.14198/jhse.2020.154.13
McLaughlin, J. E., Howley, E. T., Bassett, D. R., Thompson, D. L., and Fitzhugh, E. C. (2010). Test of the classic model for predicting endurance running performance. Med. Sci. Sports Exerc. 42, 991–997. doi:10.1249/MSS.0b013e3181c0669d
Mologne, M. S., Hu, J., Carrillo, E., Gomez, D., Yamamoto, T., Lu, S., et al. (2022). The efficacy of an immersive virtual reality exergame incorporating an adaptive cable resistance system on fitness and cardiometabolic measures: a 12-week randomized controlled trial. Int. J. Environ. Res. Public. Health 20, 210. doi:10.3390/ijerph20010210
Moran, J., Parry, D. A., Lewis, I., Collison, J., Rumpf, M. C., and Sandercock, G. R. H. (2018). Maturation-related adaptations in running speed in response to sprint training in youth soccer players. J. Sci. Med. Sport 21, 538–542. doi:10.1016/j.jsams.2017.09.012
Muehlbauer, T., Gollhofer, A., and Granacher, U. (2015). Associations between measures of balance and lower-extremity muscle strength/power in healthy individuals across the lifespan: a systematic review and meta-analysis. Sports Med. Auckl. N. Z. 45, 1671–1692. doi:10.1007/s40279-015-0390-z
Nuzzo, J. L., McBride, J. M., Cormie, P., and McCaulley, G. O. (2008). Relationship between countermovement jump performance and multijoint isometric and dynamic tests of strength. J. Strength Cond. Res. 22, 699–707. doi:10.1519/JSC.0b013e31816d5eda
Ozaki, H., Loenneke, J. P., Thiebaud, R. S., and Abe, T. (2013). Resistance training induced increase in VO2max in young and older subjects. Eur. Rev. Aging Phys. Act. 10, 107–116. doi:10.1007/s11556-013-0120-1
Pan, H., Eng, H., and Li, Y. (2011). Effects of load-controlled proprioceptive training on lower extremity motor and balance function of stroke patients 26, 1025–1028. doi:10.3969/j.issn.1001-1242.2011.11.008
Peng, H.-T., Tien, C.-W., Lin, P.-S., Peng, H.-Y., and Song, C.-Y. (2020). Novel mat exergaming to improve the physical performance, cognitive function, and dual-task walking and decrease the fall risk of community-dwelling older adults. Front. Psychol. 11, 1620. doi:10.3389/fpsyg.2020.01620
Police Officer Training - Preparing for the Physical Abilities Test (2016). Police officer training - preparing for the physical Abilities test [WWW document]. U. S. Capitol Police. Available at: https://www.uscp.gov/police-officer-training-preparing-physical-abilities-test (Accessed January 21, 24).
Presidential Youth Fitness (2024). Presidential youth fitness program | health.gov [WWW document]. Available at: https://health.gov/our-work/nutrition-physical-activity/presidents-council/programs-awards/presidential-youth-fitness-program (Accessed January 21, 24).
Prieto-González, P. (2022). Relationship between specific field-based physical fitness test results and selected health biomarkers in college-aged males: a cross-sectional study. Int. J. Environ. Res. Public. Health 19, 14498. doi:10.3390/ijerph192114498
Raghuveer, G., Hartz, J., Lubans, D., Takken, T., Wiltz, J., Mietus-Snyder, M., et al. (2020). Cardiorespiratory fitness in youth – an important marker of health: a scientific statement from the American heart association. Circulation 142, e101–e118. –e118. doi:10.1161/CIR.0000000000000866
Rodríguez-Rosell, D., Franco-Márquez, F., Mora-Custodio, R., and González-Badillo, J. J. (2017a). Effect of high-speed strength training on physical performance in young soccer players of different ages. J. Strength Cond. Res. 31, 2498–2508. doi:10.1519/JSC.0000000000001706
Rodríguez-Rosell, D., Mora-Custodio, R., Franco-Márquez, F., Yáñez-García, J. M., and González-Badillo, J. J. (2017b). Traditional vs. Sport-specific vertical jump tests: reliability, validity, and relationship with the legs strength and sprint performance in adult and teen soccer and basketball players J. Strength Cond. Res. 31, 196–206. doi:10.1519/JSC.0000000000001476
Ruiz, J. R., Castro-Piñero, J., España-Romero, V., Artero, E. G., Ortega, F. B., Cuenca, M. M., et al. (2011). Field-based fitness assessment in young people: the ALPHA health-related fitness test battery for children and adolescents. Br. J. Sports Med. 45, 518–524. doi:10.1136/bjsm.2010.075341
Sadeghi, H., Jehu, D. A., Daneshjoo, A., Shakoor, E., Razeghi, M., Amani, A., et al. (2021). Effects of 8 Weeks of balance training, virtual reality training, and combined exercise on lower limb muscle strength, balance, and functional mobility among older men: a randomized controlled trial. Sports Health 13, 606–612. doi:10.1177/1941738120986803
Santanielo, N., Nóbrega, S. R., Scarpelli, M. C., Alvarez, I. F., Otoboni, G. B., Pintanel, L., et al. (2020). Effect of resistance training to muscle failure vs non-failure on strength, hypertrophy and muscle architecture in trained individuals. Biol. Sport 37, 333–341. doi:10.5114/biolsport.2020.96317
Sawle, L., Freeman, J., and Marsden, J. (2017). INTRA-RATER RELIABILITY OF THE MULTIPLE SINGLE-LEG HOP-STABILIZATION TEST AND RELATIONSHIPS WITH AGE, LEG DOMINANCE AND TRAINING. Int. J. Sports Phys. Ther. 12, 190–198.
Seitz, L. B., Reyes, A., Tran, T. T., Saez de Villarreal, E., and Haff, G. G. (2014). Increases in lower-body strength transfer positively to sprint performance: a systematic review with meta-analysis. Sports Med. Auckl. N. Z. 44, 1693–1702. doi:10.1007/s40279-014-0227-1
Smits-Engelsman, B. C. M., Jelsma, L. D., and Ferguson, G. D. (2017). The effect of exergames on functional strength, anaerobic fitness, balance and agility in children with and without motor coordination difficulties living in low-income communities. Hum. Mov. Sci. 55, 327–337. doi:10.1016/j.humov.2016.07.006
Sonoda, T., Tashiro, Y., Suzuki, Y., Kajiwara, Y., Zeidan, H., Yokota, Y., et al. (2018). Relationship between agility and lower limb muscle strength, targeting university badminton players. J. Phys. Ther. Sci. 30, 320–323. doi:10.1589/jpts.30.320
Spiteri, T., Nimphius, S., Hart, N. H., Specos, C., Sheppard, J. M., and Newton, R. U. (2014). Contribution of strength characteristics to change of direction and agility performance in female basketball athletes. J. Strength Cond. Res. 28, 2415–2423. doi:10.1519/JSC.0000000000000547
Stewart, P. F., Turner, A. N., and Miller, S. C. (2014). Reliability, factorial validity, and interrelationships of five commonly used change of direction speed tests. Scand. J. Med. Sci. Sports 24, 500–506. doi:10.1111/sms.12019
Suchomel, T. J., Nimphius, S., and Stone, M. H. (2016). The importance of muscular strength in athletic performance. Sports Med. Auckl. N. Z. 46, 1419–1449. doi:10.1007/s40279-016-0486-0
Trinh, C. M. (2019). Predicting VO2max from 1- and 1.5-mile runs. Int. J. Exerc. Sci. 2 (11). https://digitalcommons.wku.edu/ijesab/vol2/iss11/8.
Viana, R. B., de Oliveira, V. N., Dankel, S. J., Loenneke, J. P., Abe, T., da Silva, W. F., et al. (2021). The effects of exergames on muscle strength: a systematic review and meta-analysis. Scand. J. Med. Sci. Sports 31, 1592–1611. doi:10.1111/sms.13964
Warburton, D. E. R., Bredin, S. S. D., Horita, L. T. L., Zbogar, D., Scott, J. M., Esch, B. T. A., et al. (2007). The health benefits of interactive video game exercise. Appl. Physiol. Nutr. Metab. Physiol. Appl. Nutr. Metab. 32, 655–663. doi:10.1139/H07-038
Yamamoto, T., Pandit, B., Viggiano, M., McCabe, C., Mickelsen, E., Geralds, C., et al. (2023). Inclusion of a connected adaptive resistance exercise machine in a 6-week training regimen with collegiate basketball players: a double-blind randomized controlled trial. Sci. J. Sport Perform. 2, 540–552. doi:10.55860/YBCW4009
Yáñez-García, J. M., Rodríguez-Rosell, D., Mora-Custodio, R., and González-Badillo, J. J. (2022). Changes in muscle strength, jump, and sprint performance in young elite basketball players: the impact of combined high-speed resistance training and plyometrics. J. Strength Cond. Res. 36, 478–485. doi:10.1519/JSC.0000000000003472
Keywords: immersive virtual reality, exergaming, field-based fitness tests, exercise physiology, strength training
Citation: Mologne MS, Yamamoto T, Viggiano M, Blatney AE, Lechner RJ, Nguyen TH, Doyle A, Farrales JP, Neufeld EV and Dolezal BA (2024) Field-based fitness measures improve via an immersive virtual reality exergaming platform: a randomized controlled trial. Front. Virtual Real. 5:1290711. doi: 10.3389/frvir.2024.1290711
Received: 07 September 2023; Accepted: 29 February 2024;
Published: 21 March 2024.
Edited by:
Jorge Peña, University of California, Davis, United StatesReviewed by:
Camren Allen, University of California, Davis, United StatesJavier Gene-Morales, University of Valencia, Spain
Copyright © 2024 Mologne, Yamamoto, Viggiano, Blatney, Lechner, Nguyen, Doyle, Farrales, Neufeld and Dolezal. This is an open-access article distributed under the terms of the Creative Commons Attribution License (CC BY). The use, distribution or reproduction in other forums is permitted, provided the original author(s) and the copyright owner(s) are credited and that the original publication in this journal is cited, in accordance with accepted academic practice. No use, distribution or reproduction is permitted which does not comply with these terms.
*Correspondence: Mitchell S. Mologne, mmologne@gmail.com