- 1Laboratory of Inflammation Pharmacology and Immunometabolism, Institute of Pharmacology and Morphophysiology, Faculty of Veterinary Sciences, Universidad Austral de Chile, Valdivia, Chile
- 2Institute of Pharmacy, Faculty of Sciences, Universidad Austral de Chile, Valdivia, Chile
- 3Institute of Veterinary Clinical Sciences, Faculty of Veterinary Sciences, Universidad Austral de Chile, Valdivia, Chile
- 4Institute of Physiology, Faculty of Medicine, Universidad Austral de Chile, Valdivia, Chile
D-lactic acidosis is associated with fermentative disturbances and is often marked by elevated levels of D-lactic acid in the blood, ruminal fluid, and synovial fluid in cattle. D-lactic acidosis is linked to various inflammatory manifestations, and although the causative factors have been extensively explored, the exact pathogenesis of the associated inflammation remains elusive. Notably, less attention has been given to D-lactate, a stereoisomer found in the plasma of affected animals, which may lead to D-lactic acidosis. This review aims to highlight the evidence suggesting that D-lactate participates in the modulation of inflammatory processes and explore its potential effects on synoviocytes, polymorphonuclear neutrophils, macrophages, and T-cells. This comprehensive examination of D-lactate’s involvement in the inflammatory response process provides timely insights into the pathophysiological aspects of ruminal acidosis in cattle.
1 Introduction
Lactate exists as two stereoisomers, L-lactate and D-lactate, both of which play distinct roles in metabolism. L-lactate is the predominant form in human metabolism, is produced primarily during anaerobic glycolysis, and serves as a key intermediate in cellular energy homeostasis (1). In contrast, D-lactate, though less abundant, is produced by specific bacteria and has been associated with certain pathological conditions, such as D-lactic acidosis, particularly in patients with short bowel syndrome or gut dysbiosis (1–3).
Despite their structural similarities, these two isomers are metabolized through distinct enzymatic pathways. L-lactate is processed predominantly in the liver and heart through L-lactate dehydrogenase (LDH), whereas D-lactate metabolism occurs via D-2-hydroxyacid dehydrogenase, an enzyme present in fewer tissues with a different regulatory mechanism (2, 4, 5). This distinction is crucial since the accumulation of D-lactate can have neurotoxic effects, leading to symptoms such as confusion and ataxia (6).
Once exclusively considered a metabolic waste by-product, lactic acid is now acknowledged as a pleiotropic signal involved in diverse physiological and pathological conditions (1). Lactic acid is present as a lactate-conjugated base at a physiological pH of 7.4, and exists in two enantiomeric forms in mammals: L-lactate is produced during glycolysis when oxygen levels are low (3), while D-lactate is formed through the detoxification of methylglyoxal (2).
L-lactate is a naturally occurring enantiomer in mammals (4). In cattle blood, the normal range of L-lactate is 0.5–1 mM (7). In contrast, the D-lactate levels are in the micromolar ranges (8). Elevated L-lactate levels beyond this physiological range lead to hyperlactatemia, which is often triggered by various pathological processes are associated with deficient mitochondrial adenosine triphosphate (ATP) production (e.g., hypoxia), increased aerobic glycolysis (e.g., the Warburg effect, diabetic acidosis), or decreased lactate removal (9). Unlike hyperlactatemia, D-lactate accumulation, known as D-lactic acidosis, is observed in cases of ethylene glycol or propylene glycol poisoning (10). Dissimilar to L-lactate, D-lactate produced by alcohol dehydrogenase in the liver is not effectively metabolized by aldehyde dehydrogenases, leading to D-lactate accumulation (9, 11, 12). In humans with diabetic ketoacidosis, plasma D-lactate levels are significantly higher, reaching 3.44 ± 1.99 mM, compared to the 0.48 ± 0.56 mM in patients with diabetes without ketoacidosis (13, 14). D-Lactic acidosis can develop as a complication after surgical procedures such as small bowel resection, which is associated with short bowel syndrome and intestinal bypass surgery performed for the treatment of obesity (15, 16). The clinical manifestation of short bowel syndrome includes episodes of metabolic encephalopathy, similar to those observed in cases of ethanol intoxication (15).
The etiology of D-lactic acidosis in bovines is often due to disruptions in digestive fermentation caused by excessive grain and carbohydrate intake, or infectious diarrhea, commonly observed in neonatal calves (2, 7, 17). In addition, D-lactic acidosis in calves can be caused by a malfunction of the esophageal groove reflex, resulting in the diversion of milk into the reticulorumen (ruminal drinking) instead of direct delivery to the abomasum (18). The consequences of D-lactic acidosis in cattle have been described previously (7, 19). However, the role of D-lactate in the pathophysiology of associated diseases has not been the focus of attention. This review focuses on new advances in understanding D-lactate as a potential metabolic indicator associated with cellular damage.
Although the role of L-lactate in exercise, hypoxia, and metabolic diseases has been extensively studied, the physiological and pathophysiological roles of D-lactate remain poorly understood. Additionally, the clinical significance of D-lactate and its potential contributions to metabolic disorders beyond the known cases of D-lactic acidosis are still emerging research areas. This review aims to clarify these gaps by synthesizing current knowledge on the distinct roles and metabolic pathways of L-and D-lactate, focusing on the underexplored implications of D-lactate in health and disease.
2 Biochemistry and the metabolism of D-lactate
In mammals, D-lactate is produced through the breakdown of carbohydrates and lipids during the generation of methylglyoxal (MG) (Figure 1). MG is a byproduct of glycolysis and is formed by the fragmentation of dihydroxyacetone phosphate (DHAP) and glyceraldehyde 3-phosphate (G3P) (20). Triose phosphate isomerase metabolizes G3P into DHAP, and the conversion of DHAP to MG is catalyzed by methylglyoxal synthase (MS) (21). Moreover, MG can be formed by protein catabolism, which includes the synthesis of aminoacetone by aminoacetone synthetases, and from lipid metabolism via reactions facilitated by glycerol kinase and glycerol-3-phosphate dehydrogenase in both the kidneys and the liver. These processes connect glycolysis and lipid metabolism during MG production (22). The primary detoxification pathway for methylglyoxal is the ubiquitous glyoxalase system (23). This system consists of two enzymes, glyoxalase I (GLO1) and glyoxalase II (GLO2) and utilizes a catalytic amount of reduced glutathione (GSH) to form D-lactate (24).
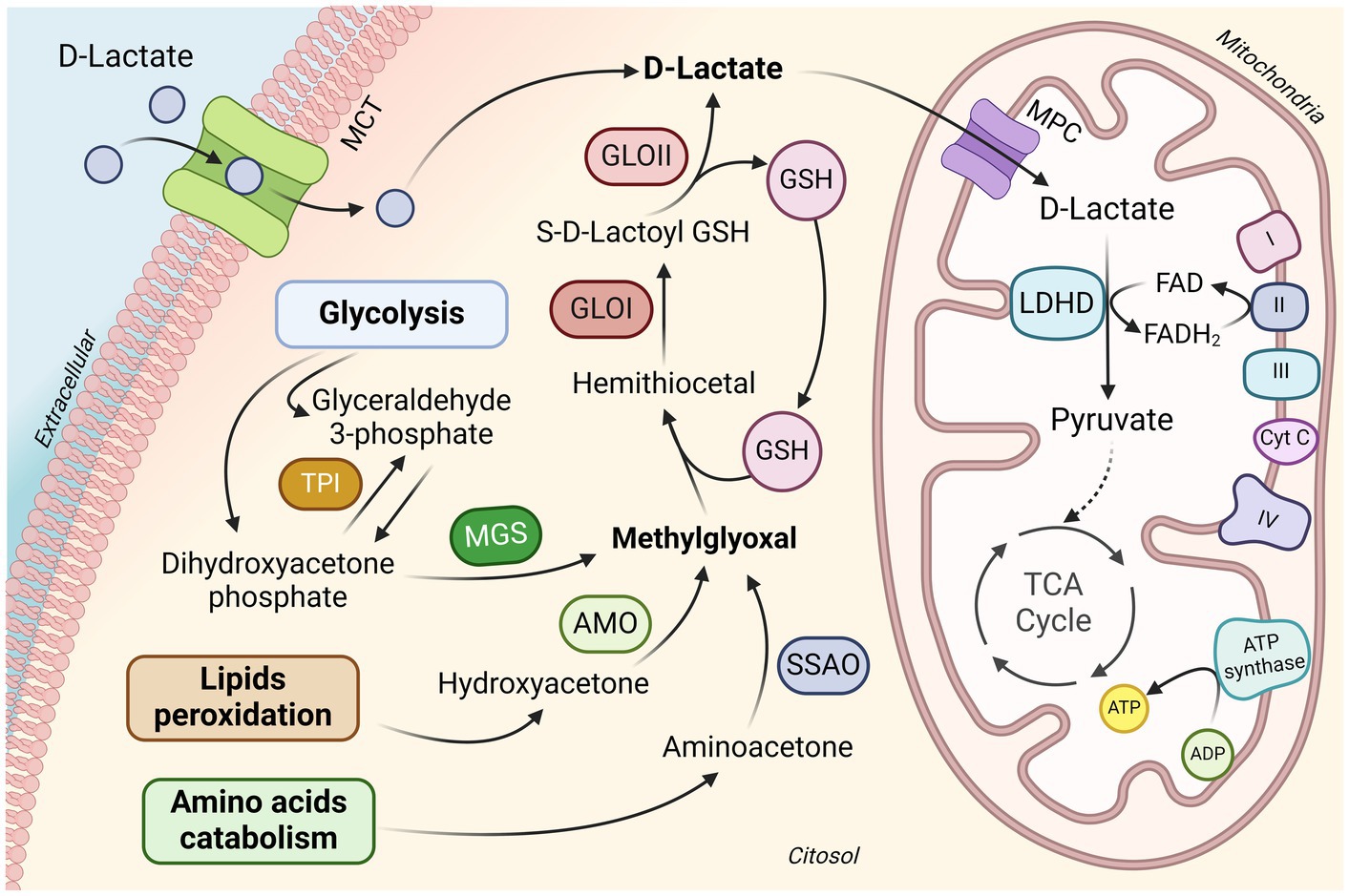
Figure 1. D-lactate metabolism in mammalian cells. D-lactate enters from the extracellular into the cytosol via MCT. Mammalian cells can also generate D-lactate during methylglyoxal metabolism. Methylglyoxal is mainly produced as a byproduct of glycolysis, although it can also be produced during lipid peroxidation and amino acid breakdown. Detoxification of methylglyoxal takes place in the cytosol by the glyoxalase system, consisting of the enzymes GLO1 and GLO2, which employ reduced GSH to form D-lactate. D-lactate metabolism occurs within the mitochondria, entering through MPC located in the inner mitochondrial membrane. Within the mitochondria, D-lactate is oxidized to pyruvate by LDHD, using FAD as a cofactor. Pyruvate continues its oxidation to enter the mitochondrial tricarboxylic acid cycle. MCT, monocarboxylate transporter; TPI, triose phosphate isomerase; MGS, methylglyoxal synthase; AMO, acetol monooxygenase; SSAO, semicarbazide-sensitive amine oxidase; GSH, glutathione; GLOI, glyoxalase 1; GLOII, glyoxalase II; MPC, mitochondrial pyruvate carrier; LDHD, D-lactate dehydrogenase; FAD/FADH2, flavin adenine dinucleotide; TCA, tricarboxylic acid. Figure created with BioRender.
In cattle, the shift from forage-based diets to concentrate-rich diets, which contain higher amounts of rapidly fermentable carbohydrates (such as during lactation or feedlot rearing), significantly alters the rumen fermentation process. The sudden intake of these carbohydrates, for example, due to sorting behavior, further drives the fermentation process to focus more on non-fibrous carbohydrates (25). In the rumen, these carbohydrates are rapidly fermented into short-chain fatty acids (SCFA) and lactate, leading to the accumulation of these acids and a decrease in ruminal pH below normal fermentation levels (i.e., pH < 5.6) (26). Here, D-Lactate is produced by the gut microbiota through lactate racemases, which are nickel-dependent enzymes present in halophilic archaea, such as Haloarcula marismortui and also in several commensal bacteria, including Lactobacillus and Clostridium (27). Many bacteria in the rumen, such as Streptococcus bovis and Lactobacillus, produce D-lactate (19, 28, 29). However, other microorganisms within this digestive environment, including Megasphaera elsdenii (M. elsdenii), Selenomonas ruminantium subsp. Lactilytica, and certain entodiniomorph protozoa, also utilize lactate (1, 30). Approximately 60–80% of DL-lactate fermentation in the rumen is attributed to M. elsdenii (28). Lactate-utilizing microorganisms are sensitive to a decrease in pH, which promotes the proliferation of lactate-producing bacteria and consequently induces ruminal D-lactic acidosis (19, 26).
In pre-weaning calves with neonatal diarrhea due to viral infections or Cryptosporidium, the prevalence of D-lactic acidosis seems to be linked to villous atrophy in the small intestine (31). This condition can lead to impaired digestion and absorption, subsequently fostering microbial fermentation of substrates in the large intestine (6, 31). In humans, D-lactic acidosis is frequently recognized as an outcome of short bowel syndrome, often arising after surgical intervention involving partial removal of the small intestine due to malignant tumors, diseases, or procedures such as jejunoileal bypass surgery (32, 33). A reduction in the length of the small intestine compromises the carbohydrate absorption capacity, leading to an elevated carbohydrate flow to bacteria in the colon. This increased influx may promote bacterial proliferation, creating an acidic environment that supports D-lactate production (32, 33).
Varying rates of D-lactate oxidation have been observed in bovine tissue analyses (34), with the highest oxidation rates observed in the kidney cortex, followed by the heart and liver, and muscle tissue displaying the lowest oxidation rates. Similarly, L-lactate oxidation was notably higher in the kidney cortex and heart, with the liver and muscle exhibiting similar rates. Notably, gluconeogenesis oxidation rates for D-and L-lactate are comparable at 0.1 mM lactate concentrations in kidney and liver. However, the proportional utilization of D-lactate relative to L-lactate declined as substrate concentrations increased. These findings emphasize the constrained capacity for D-lactate utilization by bovine tissues (35).
D-lactate metabolism is attributed to oxidation to pyruvate by D-Lactate dehydrogenase (LDHD) (36, 37). Two distinct categories of LDHDs exist: nicotinamide adenine dinucleotide (NAD)-dependent LDHDs and flavin adenine dinucleotide (FAD)-dependent LDHDs (38, 39). The NAD-dependent LDHDs, identified within certain bacterial strains, are classified within the D-isomer specific 2-hydroxyacid dehydrogenase superfamily. Nonetheless, the exact biological roles of these enzymes within bacterial systems remain elusive (40, 41). The FAD-dependent LDHDs were first identified in bacteria, archaea, yeasts, and plants (42–45). These enzymes do not share a common ancestry with either L-lactate dehydrogenases or NAD-dependent LDHDs. They are categorized within the 2-hydroxyacid dehydrogenase subfamily of the vanillyl alcohol oxidase/para-cresol methylhydroxylase (VAO/PCMH) flavoprotein family. All members of this subfamily share a conserved FAD-binding domain and a variable substrate-binding domain. Unlike its NAD-dependent counterparts, FAD is used as a cofactor instead of NAD (38, 39, 46). The FAD-dependent LDHDs have been identified in mammals, including humans, mice, and bovine (36, 38), exhibiting significant sequence resemblance to yeast LDHDs (36, 47, 48). This LDHD demonstrates elevated levels of expression in tissues characterized by high metabolic rates and abundant mitochondria (49), mostly located in the liver and kidney (50) and situated in the inner membrane of mitochondria (36, 47, 48).
3 D-lactate absorption
The solute carrier family 16 (SLC16) consists of 14 members categorized within the monocarboxylate transporter (MCT) family (51). These transmembrane proteins play a crucial role in facilitating the transport of lactate enantiomers and various other metabolically essential monocarboxylates, such as pyruvate, branched-chain oxoacids, SCFA, and ketone bodies, across cellular membranes (51, 52). The MCT1-4 group are proton-dependent transporters closely associated with the movement of by-products of the glycolysis cycle across the plasma membrane. These include lactate, pyruvate, and ketone bodies such as acetoacetate and β-hydroxybutyrate (51). The Km has been reported at 27.5 mM for D-lactate and 4.54 mM for L-lactate in Ehrlich-Lettre Tumor Cells (53). Furthermore, the uptake of D-lactate by MCT-1 is significantly lower than that of L-lactate, with both isomers displaying reciprocal inhibitory effects (54).
The absorption of L-lactate in the digestive tract of monogastric is through the MCT-1, that is localized in the apical membrane and basolateral membrane of the intestinal epithelium (55, 56). In ruminants, MCT1-4 are mostly expressed in the forestomach and large intestine epithelia (57–60). MCT-1 and MCT-2 are primarily localized in the basolateral membrane, with MCT-4 showing a slightly greater orientation towards the luminal side of the rumen epithelia (57, 59, 60). Therefore, lactate anions can enter the ruminal epithelial cells during cotransport with their protons (61). During ruminal acidosis, the concentrations of D-lactate in ruminal fluid reach 50–80 mM (7, 62, 63), suggesting that this condition may be key for MCTs D-lactate absorption. However, in contrast to SCFA, it has been suggested that lactate is more slowly absorbed (64). This could be involved to the higher Km of D-lactate (519 mM) than L-lactate (28 mM) observed with MCT-4 (65). Despite this, a decrease in ruminal pH due to excessive SCFA production promotes increased D-lactate accumulation through microbiome modifications (19, 26). In addition, has been reported that an acidic environment reported in ruminal acidosis can decrease the Km of MCT-4 for lactate (66). Moreover, the drop in ruminal pH facilitates the passive transport of lactate in its unprotonated form (lactate ion) through the ruminal wall, since the pKa of lactate is 3.84. At this pH, a significant portion of lactate remains unprotonated, allowing it to easily cross the ruminal wall. This process contributes to the development of metabolic acidosis in cattle.
Lactic acidosis becomes evident when plasma L-lactate concentrations exceed 4 mM, potentially resulting in a drop in blood pH to <7.35. Hyperlactatemia, whether mild or severe (i.e., progressing to lactic acidosis), can arise due to multiple factors such as sepsis, hemorrhagic shock, cardiac arrest, trauma, toxic exposure, ischemia, burns, diabetic ketoacidosis, certain types of cancer, and strenuous muscle activity (67). In bovines with ruminal acidosis, the blood lactate is >4 mmol/L after 24 h of ingestion of carbohydrates (7, 62), this becomes even more severe in calves, with concentrations exceeding 10 mM (31).
4 D-lactate as a potential proinflammatory agent
During ruminal acidosis, several inflammatory conditions associated with lameness are observed (68, 69). Laminitis is considered the main lesion in cattle with D-lactic acidosis, however, its etiology is still debated (19, 70). Intraruminal injection of lactic acid can induce laminitis in lambs (71, 72). Interestingly, arthritis and concurrent systemic conditions are observed in cattle with laminitis, suggesting a more diffuse inflammatory reaction (73). In fact, the experimental induction of laminitis associated with ruminal acidosis after oligofructose overload leads to an increase in the diameter of the tarsocrural joints (68, 69). Moreover, the concentration of polymorphonuclear leucocytes (PMNs) in the synovial fluid of several joints increases 24 h after induction of carbohydrate overload (68, 74). Additionally, 6 mM of D-lactate and an increase in pro-inflammatory molecules such as Interleukin (IL)-6, IL-1, prostaglandin E (PGE2), and matrix metalloproteinases (MMP)-9 were detected in the synovial fluid of affected bovines (74, 75). Elevated levels of lactate have also been identified in the synovial fluid of humans with various joint diseases such as septic arthritis (76, 77), osteoarthritis (78), osteonecrosis (79), rheumatoid arthritis (RA), and gout (80, 81). Particularly, fibroblast-like synoviocytes (FLS) in RA-affected joints exhibit an increased lactic acid production (82). This increased lactic acid concentration has been proposed to play a pivotal role in the intracellular signaling pathways regulating the production of pro-inflammatory cytokines and is considered a new potential therapeutic target (83–85). D-lactate can modulate the inflammatory response through different cells and mechanisms, contributing to the complex pathophysiology of inflammation observed in cattle. This section explores the distinct effects of D-lactate on fibroblast-like synoviocytes (FLS), polymorphonuclear cells (PMNs), macrophages, and T-cells, highlighting the specific pathways and responses involved in the inflammatory process.
4.1 Bovine fibroblast-like synoviocytes
Fibroblast-like synoviocytes (FLS) are critical to structure the intimal lining cellular layer of the synovium in diarthrodial joints and are responsible for regulating the composition of the synovial fluid and the extracellular matrix (86). The FLS also define and maintain the inflammatory environment during most arthropathies (87–89). Several antecedents suggest that D-lactate induces specific inflammatory responses in FLS. It has been shown that 5 mM D-lactate or sodium D-lactate induces the expression and production of IL-6 and IL-8 in bovine FLS, suggesting a potential role of D-lactate in joint inflammation during acute ruminal acidosis (90). Additionally, bFLS expresses mostly MCT-1 and its pharmacological inhibition with AR-C155858 was shown to significantly reduce IL-6 and IL-8 secretion induced by D-lactate (90). Furthermore, D-lactate induces the phosphorylation of p38 mitogen-activated protein kinase (MAPK), the extracellular signal-regulated kinase (ERK) 1/2 MAPK, Akt, and triggers the nuclear factor (NF)-κB pathway, which induces cytokine production in bFLS (90). D-lactate can also induce the accumulation of Hypoxia-inducible factor (HIF)-1α and in turn, the expression of IL-6. Moreover, the HIF-1 is closely linked with the PI3K/Akt and NF-κB pathway activation in bFLS (91) (Figure 2).
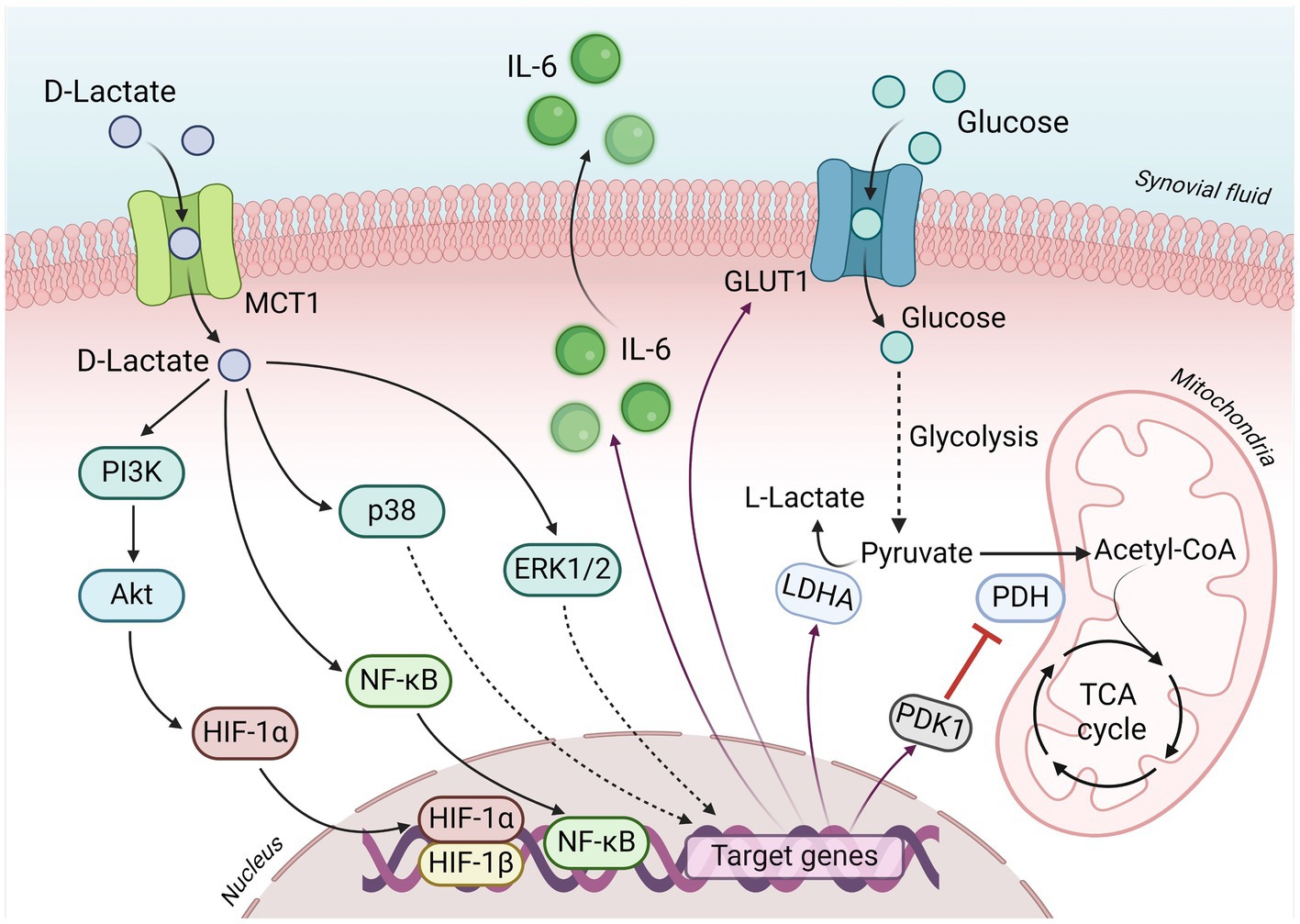
Figure 2. Key signaling events underlying D-lactate-induced metabolic reprogramming in bFLS. D-lactate enters bovine fibroblast-like synoviocytes (bFLS) through MCT-1 and induces the activation of the PI3K/Akt, p38 MAPK and ERK1/2 MAPK pathways, and the subsequent activation of the transcription factors NF-κB and HIF-1. Through these signaling pathways and transcription factors, D-lactate induces the synthesis and secretion of IL-6, an inflammatory marker characteristic of bovine polysynovitis associated with D-lactic acidosis. Additionally, the local inflammatory response is sustained thanks to the activation of HIF-1, which favors glycolytic metabolism by increasing the expression of GLUT1 (which increases the incorporation of glucose from the extracellular medium), PDK1 (blocking the mitochondrial use of pyruvate through the TCA cycle) and LDHA (ensuring glycolytic flux by favoring the oxidation of pyruvate to lactate). IL-6, interleukin 6; MCT1, monocarboxylate transporter 1; GLUT1, solute carrier family 2 (facilitated glucose transporter) member 1; PI3K, phosphatidyl inositol 3-kinase; Akt, protein kinase B; p38, p38 mitogen-activated protein kinase (MAPK); ERK1/2, extracellular signal-regulated kinase 1/2 MAPK; HIF-1α and HIF-1β, hypoxia inducible factor 1 alpha and beta subunits, respectively; NF-κB, nuclear factor kappa B; LDHA, lactate dehydrogenase A subunit; PDK1, pyruvate dehydrogenase kinase 1; PDH, pyruvate dehydrogenase; TCA, tricarboxylic acid. Figure created with BioRender.
L-lactate concentrations ranging from 10 to 40 mM have been detected in inflamed tissues of animals with diseases such as arthritis including synovial tissue (92). The RA-FLS express MCT-1 and MCT-4, which regulate lactate uptake and release from cells, respectively (93). An increase in glycolysis (thus lactate levels) has been detected in RA-FLS and is associated with inflammation (94, 95). Moreover, L-lactate can induce an elevation in glycolysis and enhance the glycolytic capacity within synovial fibroblasts (96). Additionally, L-lactate potentiates the tumor necrosis factor-alpha (TNF-α)-induced secretion of IL-6 in RA-FLS, indicating a potential activation of the HIF-1 pathway (96). Similarly, D-lactate can also increase the glycolysis augmenting glucose uptake and GLUT-1 expression in bFLS via HIF-1, suggesting a metabolic reprogramming associated with the inflammatory response (91). Moreover, this effect is additionally supported not only by an upregulation of glycolytic enzymes such as LDHA, but also by an upregulation of pyruvate dehydrogenase kinase (PDK)-1, which inhibits Pyruvate dehydrogenase (PDH), hindering the conversion of pyruvate to Acetyl-CoA, ultimately favoring the production of lactate (91) (Figure 1). A PDK-1 inhibitor, such as dichloroacetic acid (DCA), has been utilized to correct the pyruvate influx into the mitochondria, consequently enhancing oxidative phosphorylation and reducing lactate accumulation (97). Studies thus suggest the effectiveness of a PDK-1 inhibitor in reducing inflammation in collagen II-induced arthritis in female DBA/1 mice (98). In bFLS, in which TNF-α increases glycolysis and reduces pyruvate influx into the tricarboxylic acid (TCA) cycle and increasing IL-6 production, DCA effectively reduces the secretion of IL-6 (99). Additionally, metabolic disturbances characterized by increased levels of pyruvate, D-lactate, L-lactate and IL-6 have been detected in the synovial fluid of heifers with acute ruminal acidosis induced by oligofructose overload (75). Thus, the utilization of modifiers targeting glycolytic metabolism emerges as a novel strategy to treat joint inflammation (83–85, 100). This approach warrants attention and exploration within the context of livestock health, especially towards the prevention and management of metabolic-inflammatory diseases in cattle herds.
4.2 Polymorphonuclear leucocytes
Polymorphonuclear leucocytes (PMNs) constitute the primary and most abundant leukocyte population in cattle joints 24 h after induced ruminal acidosis (68, 74). PMNs perform various functions, including phagocytosis, generating reactive oxygen species (ROS), and forming neutrophil extracellular traps (NETs) (101, 102). The presence of NETs in synovial fluid is a characteristic of aseptic polysynovitis in cattle (74). While NETs are acknowledged as a beneficial microbicidal mechanism, they are also pathological biomarkers of early rheumatoid arthritis (103, 104). NETs serve as a source of citrullinated autoantigens, significantly amplifying the inflammatory response and triggering the production of inflammatory molecules such as IL-6, IL-8, and adhesion proteins (104). Bovine PMNs (bPMNs) exhibit an induction of NET release upon exposure to D-lactate in vitro, a process reliant on its cellular uptake facilitated by MCT-1 transporters, subsequently activating peptidyl arginine deiminase 4 (PAD-4) that induce the histone H4 citrullination (105). Moreover, the release of NET induced PMNs adhesion to bovine endothelial cells by increasing CD11b expression and L-selectin shedding (105) (Figure 3). Similarly, L-lactate has shown the capability to induce NET formation in human neutrophils. Inhibition of lactate dehydrogenase (LDH) activity markedly reduced NETosis induced by PMA and A23187, two well-described NADPH oxidase-dependent and-independent NETosis-inducing stimuli, respectively (106). PMA and A23187 can stimulate PKM2 dimerization and increase LDH activity, consequently triggering a Warburg effect in human neutrophils (106). In contrast, another study proposed that L-lactate generated during exercise may attenuate PMA-induced NET formation and ROS production (107).
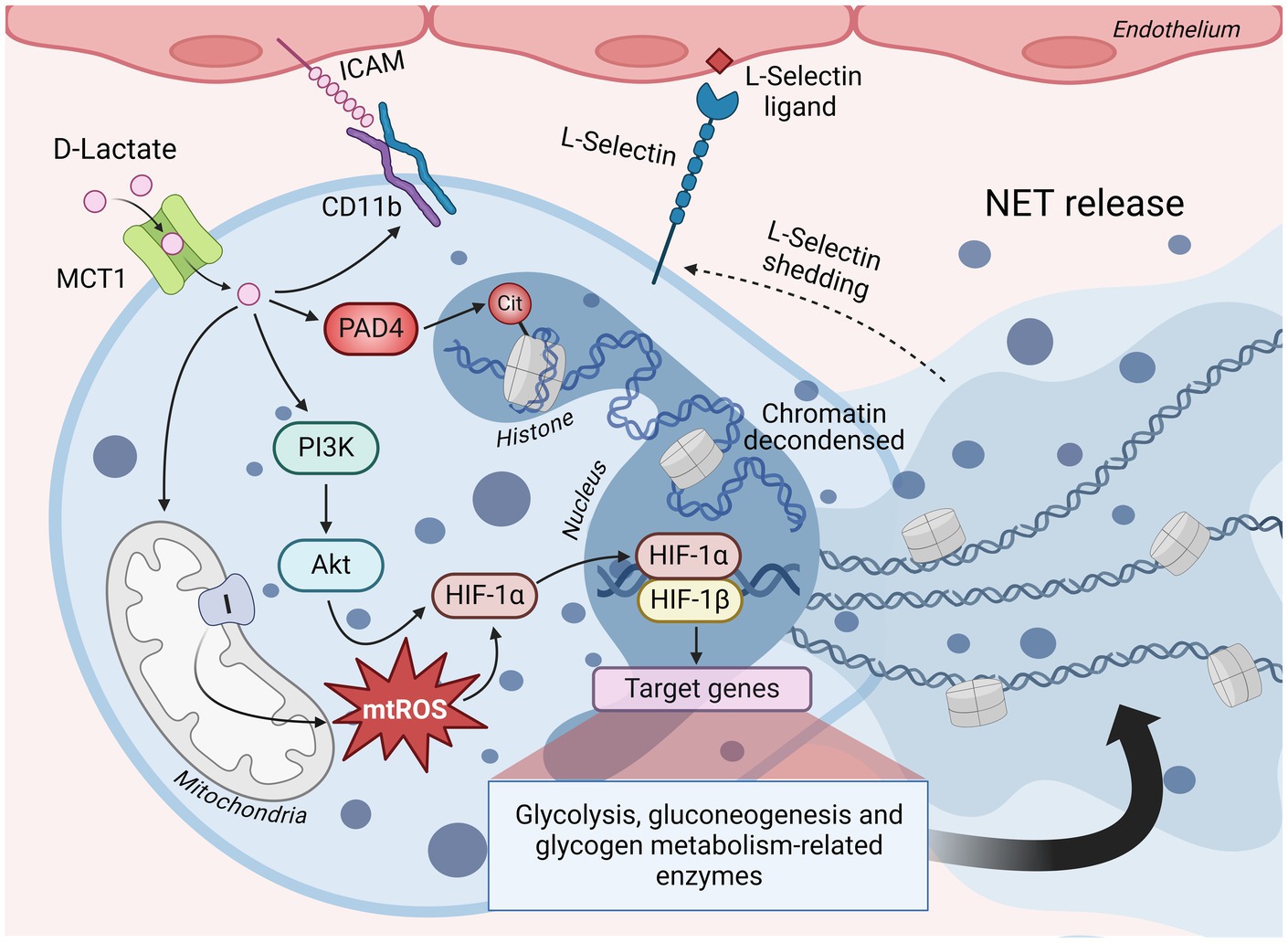
Figure 3. D-lactate-induced NET formation is sustained through metabolic reprogramming of bPMN. Bovine polymorphonuclear neutrophils (bPMN) incorporate D-lactate through MCT-1, which induces the activation of the PI3K/Akt signaling pathway and the subsequent stabilization of HIF-1α. Additionally, D-lactate triggers the activation of PAD4, which catalyzes the transformation of arginine residues to citrulline in histones, leading to chromatin decondensation. HIF-1 activation is primarily responsible for the metabolic reprogramming necessary to energetically sustain NET release, through the overexpression of enzymes associated with glycolysis, gluconeogenesis and glycogen metabolism. D-lactate also increases the production of mtROS, mainly through mitochondrial complex I, a molecular mechanism necessary to induce the stabilization of HIF-1α and, consequently, the greater transcriptional activity of HIF-1. Furthermore, D-lactate promotes bPMN adhesion to endothelial cells through a mechanism involving the increased expression of CD11b and shedding of L-selectin. MCT1, monocarboxylate transporter 1; PI3K, phosphatidylinositol 3-kinase; Akt, protein kinase B; HIF-1α and HIF-1β, hypoxia inducible factor 1 alpha and beta subunits, respectively; mtROS, mitochondrial reactive oxygen species; PAD4, peptidyl arginine deiminase 4; Cit, citrulline; ICAM, intercellular adhesion molecule; NET, neutrophil extracellular traps. Figure created with BioRender.
D-lactate demonstrates the capacity to stabilize HIF-1α in a PI3K/Akt pathway-dependent manner and increase the levels of LDHA and PDK-1 in bPMNs (108). Untargeted metabolomic analysis showed that D-lactate amplifies glycolysis and gluconeogenesis in bPMNs. Furthermore, inhibiting glycolysis using 2-DG and 3PO, which are inhibitors of hexokinase and 6-phosphofructo-2-kinase/fructose-2,6-bisphosphatase 3, respectively, resulted in a reduction of NET formation (109) (Figure 3). Additionally, D-lactate stimulates glycogenolysis in bPMNs, which is vital for NET release. This critical attribute was corroborated by the noticeable inhibition observed with CP-91,149, a glycogen phosphorylase inhibitor, emphasizing the significance of glycogen reserves as an energy source pivotal for this mechanism (109).
Mitochondria are the powerhouse of the cell and are responsible of ATP production. The electron transport chain (ETC), located in the inner mitochondrial membrane, consists of four main complexes (I-IV) that sequentially transfer electrons from NADH and FADH2 to oxygen to form water (110, 111). As electrons move through these complexes, protons are pumped from the mitochondrial matrix to the intermembrane space by complexes I, III and IV, generating a proton gradient (ΔpH) and an electrical potential (Δψm) (110, 112). Together, these components form the electrochemical potential known as the proton motive force (Δp). The ATP synthase (Complex V) uses the energy from this to cause proton flow back into the matrix to synthesize ATP from ADP and inorganic phosphate (Pi) in a process known as oxidative phosphorylation (111, 113). This mechanism efficiently converts energy from nutrients into ATP, which is the primary energy currency of the cell (111).
Earlier electron microscopy studies usually failed to identify classic mitochondria in neutrophils (114, 115). This suggested that neutrophil mitochondrial function may be primarily confined to apoptotic processes, due to their apparent low mitochondrial count and modest oxidative phosphorylation (OXPHOS) activity (115). However, our previous studies showed that bPMNs possess a functional and complex network of mitochondria (116) with similar characteristics to those described in human PMNs (114). Evidence suggests that mitochondria contribute to the inflammatory process via mitochondrial reactive oxygen species (mtROS) production. This hypothesis is supported by the increase of mtROS in bPMNs by PAF (116). PAF-induced NET release is inhibited by rotenone, a complex I inhibitor (117). Interestingly, mtROS can be generated by complexes I and III of the respiratory chain (118, 119), which serve as the primary source of ROS in neutrophils, particularly in response to NOX-independent stimuli such as calcium ionophores (118).
Interestingly, D-lactate also increases mtROS in bPMNs and its scavenging with mitoTEMPO effectively reduces NET formation (108). In this context, mtROS have been described as key mechanisms for NET release in several diseases and as potential therapeutic targets (120–123). Overactivation of the type I interferon signaling pathway induces ROS and mtROS production, leading to NETosis in the neutrophils of patients with primary Sjögren’s syndrome (124) and a lupus mouse model (123). The mechanism of mtROS induction by D-lactate in blood bPMNs remains unknown; however, it is plausible that it involves metabolic reprogramming with elevation in the expression of hexokinases 2 and 3, as well as phosphofructokinase. This may coincide with the elevated levels of glucose and glucose-6-phosphate detected in the cytosol (109). Lactate can increase glucose metabolism and induce ROS production in chondrocytes via NADPH oxidase 4 (125). Furthermore, preconditioning fibroblasts in a culture medium that relies exclusively on lactate as a fuel source leads to a transition from OXPHOS to glycolysis. This metabolic shift is partly facilitated by the ROS-mediated stabilization of HIF-1α (126). Similarly, mtROS is required for HIF-1α stabilization by hypoxia in human neutrophils (127) and in bPMNs induced by D-lactate under normoxia (108). Moreover, the upregulation of glycolysis in neutrophils promotes enhancements in mtROS production through the glycerol 3-phosphate shuttle (127).
4.3 Macrophages
L-lactate can induce lactylation and post-translational modifications (PTMs), which serve as potential mechanisms to regulate inflammation (128). This process involves the inhibition of signaling pathways and the alteration of histones, resulting in the reduction of inflammatory macrophages that drive M2-like polarization towards a reparative phenotype (128, 129). Additionally, evidence also suggests that D-lactate may not directly induce histone lactylation (130). Instead, it can indirectly facilitate the lactylation of cytosolic proteins through S-D-(R)-Lactylglutathione, an intermediate in the glyoxalase pathway. This compound can react non-enzymatically, resulting in lysine D-lactylation (K(D-la)) and PTMs of glycolytic enzymes (131). These differences may explain some of the discrepancies in the response of lactate enantiomers to inflammation observed in bFLS in vitro (90). In contrast, D-lactate has been observed to promote histone deacetylase (HDAC) protein gene expression, potentially serving as a crucial transcriptional regulator (132, 133). This association potentially connects the impact of metabolic disturbances to alterations in gene transcription of proinflammatory proteins in cattle. However, further research is required to confirm this hypothesis. Recent findings suggest that D-lactate can induce the transformation of M2 macrophages into M1 macrophages. This modulation would occur by inhibiting the PI3K/Akt pathway while concurrently activating the NF-κB pathway (134). These observations suggest that D-lactate could potentially act as an agonist on TLR2 and TLR9 receptors, increasing the inducible nitric oxide synthase (iNOS), TNF-α, and IL-12, thereby exerting influence over the polarization of macrophages towards a pro-inflammatory phenotype (134). TLR2 can activate the PI3K/Akt pathway and NK-κB via MyD88/TAK1 and the expression of pro-inflammatory cytokines (135). Activation of TLR9 induces ERK1/2 and Akt pathways and the IL-6 and TNF-α production in macrophages (136). This finding supports the potential role of D-lactate as a danger-associated metabolite. In contrast, the role of D-lactate in macrophage function could be a more complex scenario. D-lactate exhibits anti-inflammatory effects in experimental models of colitis and endotoxemia, through a specific receptor known as GPR81 (formerly hydroxycarboxylic acid receptor 1, HCAR1) (137). Moreover, D-lactate interfered with M1 polarization provide survival advantage in acute inflammation (137).
4.4 T-cells
D-lactate influences T-cell responses by modulating metabolic and signaling pathways that are crucial for their activation, proliferation, and cytokine production (138). Moreover, the interaction of D-lactate with MCTs, particularly MCT-1, highlights its ability to influence T-cell metabolism and function through direct uptake and utilization (138). Unlike L-lactate, which primarily supports glycolysis, D-lactate bypasses cytosolic LDH metabolism, enhances mitochondrial OXPHOS and promote a shift towards energy production through the TCA cycle (139). Additionally, human D-lactate dehydrogenase specifically transfers electrons from D-lactate to cytochrome c, supporting mitochondrial membrane polarization (140). This metabolic shift allows T-cells to sustain prolonged activation and function under conditions of metabolic stress. The TCA cycle and OXPHOS play central roles in regulating T cell-mediated inflammation by providing energy, generating key metabolites, and influencing the differentiation and function of T cell subsets (141). In fact, TCA cycle flux in Th1 and Th17 cells, which controls the elevated succinate/α-ketoglutarate (α-KG) ratio, promotes proinflammatory responses by stabilizing HIF-1α and increasing the expression of inflammatory cytokines such as IL-17 (141, 142). Moreover, mitochondrial activity, including the electron transport chain (ETC), generates ROS that act as signaling molecules to modulate T-cell fate and function (142). These metabolic processes are pivotal in maintaining immune homeostasis, and their disruption contributes to the pathogenesis of autoimmune diseases, highlighting potential therapeutic targets for inflammation modulation (141, 142).
D-lactate has been shown to increase mitochondrial ATP production and support pyruvate entry into the TCA cycle, enhancing the energetic capacity of T-cells (143, 144). This metabolic reprogramming favors Th2 polarization, characterized by cytokine production such as IL-4 and IL-13 (138). These cytokines not only modulate inflammatory pathways, but also play a critical role in modulating macrophage polarization from a pro-inflammatory M1 phenotype to an anti-inflammatory M2 phenotype, as discussed previously (145). In addition to its metabolic effects, D-lactate may act as a signaling molecule that synergizes with inflammatory stimuli, such as PMA/ionomycin, to amplify cytokine production in HUT-78 T-cells (138). Notably, this includes its capacity to enhance the expression of Th2 cytokines, which are implicated in the regulation of synovitis and systemic inflammation (145). However, the potential effects of D-lactate on the T-subset of cells could be more complex and require more studies to extend our knowledge of its role in the inflammatory process in cattle.
5 Conclusion
Several evidence suggest that D-lactate may enhance glycolytic activity in PMNs, FLS, and macrophages, disrupt cellular function, and that can induce an inflammatory response. Given that an increase in D-lactate is a characteristic of metabolic disorders associated with pathological processes in livestock, including lameness, it may be a relevant factor during the initial steps leading to the inflammatory response. D-lactate may exert pleiotropic effects depending on the affected tissue, as its metabolism relies on LDHD. This implies a more intricate scenario in cells expressing low levels of LDHD, which potentially interferes with mitochondrial function. In addition, the effect of D-lactate on the increase of Th2 cytokine expression may contribute to inflammatory modulation. This aspect warrants further investigation to elucidate the complexities associated with the impact of D-lactate on cellular processes and its potential implications for various pathological conditions in livestock.
Author contributions
RB: Writing – original draft. CM: Writing – review & editing. PA: Writing – review & editing. MN: Writing – review & editing. JQ: Writing – review & editing. GM: Writing – review & editing. JG: Writing – review & editing. SB: Writing – review & editing. MC: Writing – review & editing.
Funding
The author(s) declare that financial support was received for the research, authorship, and/or publication of this article. This work was supported by FONDECYT regular 1210754 and Postdoctoral FONDECYT 3230482.
Conflict of interest
The authors declare that this review was conducted without any commercial or financial relationships that could be construed as potential conflicts of interest.
The author(s) declared that they were an editorial board member of Frontiers, at the time of submission. This had no impact on the peer review process and the final decision.
Generative AI statement
The authors declare that no Generative AI was used in the creation of this manuscript.
Publisher’s note
All claims expressed in this article are solely those of the authors and do not necessarily represent those of their affiliated organizations, or those of the publisher, the editors and the reviewers. Any product that may be evaluated in this article, or claim that may be made by its manufacturer, is not guaranteed or endorsed by the publisher.
References
1. Manosalva, C, Quiroga, J, Hidalgo, AI, Alarcon, P, Anseoleaga, N, Hidalgo, MA, et al. Role of lactate in inflammatory processes: friend or foe. Front Immunol. (2021) 12:808799. doi: 10.3389/fimmu.2021.808799
2. Ewaschuk, JB, Naylor, JM, and Zello, GA. D-lactate in human and ruminant metabolism. J Nutr. (2005) 135:1619–25. doi: 10.1093/jn/135.7.1619
3. Gladden, LB. Lactate metabolism: a new paradigm for the third millennium. J Physiol. (2004) 558:5–30. doi: 10.1113/jphysiol.2003.058701
4. Ferguson, BS, Rogatzki, MJ, Goodwin, ML, Kane, DA, Rightmire, Z, and Gladden, LB. Lactate metabolism: historical context, Prior misinterpretations, and current understanding. Eur J Appl Physiol. (2018) 118:691–728. doi: 10.1007/s00421-017-3795-6
5. Ling, B, Peng, F, Alcorn, J, Lohmann, K, Bandy, B, and Zello, GA. D-lactate altered mitochondrial energy production in rat brain and heart but not liver. Nutr Metab (Lond). (2012) 9:20120201. doi: 10.1186/1743-7075-9-6
6. Lorenz, I, and Gentile, A. D-lactic acidosis in neonatal ruminants. Vet Clin North Am Food Anim Pract. (2014) 30:317–331, v. doi: 10.1016/j.cvfa.2014.03.004
7. Dunlop, RH, and Hammond, PB. D-lactic acidosis of ruminants. Ann N Y Acad Sci. (1965) 119:1109–32. doi: 10.1111/j.1749-6632.1965.tb47466.x
8. Pereira, MN, Garrett, EF, Oetzel, GR, and Armentano, LE. Partial replacement of forage with nonforage Fiber sources in lactating cow diets. I. Performance and health. J Dairy Sci. (1999) 82:2716–30. doi: 10.3168/jds.s0022-0302(99)75528-1
9. Kamel, KS, Oh, MS, and Halperin, ML. L-lactic acidosis: pathophysiology, classification, and causes; emphasis on biochemical and metabolic basis. Kidney Int. (2020) 97:75–88. doi: 10.1016/j.kint.2019.08.023
10. Kraut, JA, and Kurtz, I. Toxic alcohol ingestions: clinical features, diagnosis, and management. Clin J Am Soc Nephrol. (2008) 3:208–25. doi: 10.2215/CJN.03220807
11. Bianchetti, D, Amelio, GS, Lava, SAG, Bianchetti, MG, Simonetti, GD, Agostoni, C, et al. D-lactic acidosis in humans: systematic literature review. Pediatr Nephrol. (2018) 33:673–81. doi: 10.1007/s00467-017-3844-8
12. Christopher, MM, Eckfeldt, JH, and Eaton, JW. Propylene glycol ingestion causes D-lactic acidosis. Lab Investig. (1990) 62:114–8.
13. Bo, J, Li, W, Chen, Z, Wadden, DG, Randell, E, Zhou, H, et al. D-lactate: a novel contributor to metabolic acidosis and high anion gap in diabetic ketoacidosis. Clin Chem. (2013) 59:1406–7. doi: 10.1373/clinchem.2013.208777
14. Lu, J, Zello, GA, Randell, E, Adeli, K, Krahn, J, and Meng, QH. Closing the anion gap: contribution of D-lactate to diabetic ketoacidosis. Clin Chim Acta. (2011) 412:286–91. doi: 10.1016/j.cca.2010.10.020
15. McHale, C, Keating, E, O'Donovan, H, and Slattery, E. D-lactic acidosis presenting as metabolic encephalopathy in a patient with short bowel syndrome. BMJ Case Rep. (2021) 14:e241102. doi: 10.1136/bcr-2020-241102
16. Zhang, DL, Jiang, ZW, Jiang, J, Cao, B, and Li, JS. D-lactic acidosis secondary to short bowel syndrome. Postgrad Med J. (2003) 79:110–2. doi: 10.1136/pmj.79.928.110
17. Ewaschuk, JB, Naylor, JM, Palmer, R, Whiting, SJ, and Zello, GA. D-lactate production and excretion in diarrheic calves. J Vet Intern Med. (2004) 18:744–7. doi: 10.1892/0891-6640(2004)18<744:dpaeid>2.0.co;2
18. Gentile, A, Sconza, S, Lorenz, I, Otranto, G, Rademacher, G, Famigli-Bergamini, P, et al. D-lactic acidosis in calves as a consequence of experimentally induced ruminal acidosis. J Vet Med A Physiol Pathol Clin Med. (2004) 51:64–70. doi: 10.1111/j.1439-0442.2004.00600.x
19. Hernandez, J, Benedito, JL, Abuelo, A, and Castillo, C. Ruminal acidosis in feedlot: from Aetiology to prevention. ScientificWorldJournal. (2014) 2014:702572:1–8. doi: 10.1155/2014/702572
20. Angeloni, C, Zambonin, L, and Hrelia, S. Role of methylglyoxal in Alzheimer's disease. Biomed Res Int. (2014) 2014:238485. doi: 10.1155/2014/238485
21. Desai, KM, Chang, T, Wang, H, Banigesh, A, Dhar, A, Liu, J, et al. Oxidative stress and aging: is methylglyoxal the hidden enemy? Can J Physiol Pharmacol. (2010) 88:273–84. doi: 10.1139/Y10-001
22. Kalapos, MP. Where does plasma methylglyoxal originate from? Diabetes Res Clin Pract. (2013) 99:260–71. doi: 10.1016/j.diabres.2012.11.003
23. Thornalley, PJ. The glyoxalase system in health and disease. Mol Asp Med. (1993) 14:287–371. doi: 10.1016/0098-2997(93)90002-u
24. Cai, M, Wan, J, Cai, K, Song, H, Wang, Y, Sun, W, et al. Understanding the contribution of lactate metabolism in Cancer Progress: a perspective from isomers. Cancers (Basel). (2022) 15:87. doi: 10.3390/cancers15010087
25. Elmhadi, ME, Ali, DK, Khogali, MK, and Wang, H. Subacute ruminal acidosis in dairy herds: microbiological and nutritional causes, consequences, and prevention strategies. Anim Nutr. (2022) 10:148–55. doi: 10.1016/j.aninu.2021.12.008
26. Nocek, JE. Bovine acidosis: implications on laminitis. J Dairy Sci. (1997) 80:1005–28. doi: 10.3168/jds.S0022-0302(97)76026-0
27. Zhou, XZ, Zhang, N, Hossain, F, Kandalai, S, Tian, HY, and Zheng, QF. Biosynthesis of D/L-lactate from methylglyoxal. Tetrahedron. (2022):127. doi: 10.1016/j.tet.2022.133087
28. Nagaraja, TG, and Titgemeyer, EC. Ruminal acidosis in beef cattle: the current microbiological and nutritional outlook. J Dairy Sci. (2007) 90:E17–38. doi: 10.3168/jds.2006-478
29. He, B, Fan, Y, and Wang, H. Lactate uptake in the rumen and its contributions to subacute rumen acidosis of goats induced by high-grain diets. Front Vet Sci. (2022) 9:964027. doi: 10.3389/fvets.2022.964027
30. Bramley, E, Lean, IJ, Fulkerson, WJ, Stevenson, MA, Rabiee, AR, and Costa, ND. The definition of acidosis in dairy herds predominantly fed on pasture and concentrates. J Dairy Sci. (2008) 91:308–21. doi: 10.3168/jds.2006-601
31. Trefz, FM, Lorch, A, Feist, M, Sauter-Louis, C, and Lorenz, I. Metabolic acidosis in neonatal calf diarrhea-clinical findings and theoretical assessment of a simple treatment protocol. J Vet Intern Med. (2012) 26:162–70. doi: 10.1111/j.1939-1676.2011.00848.x
32. Halverson, J, Gale, A, and Lazarus, C. D-lactic acidosis and other complications of intestinal bypass surgery. Arch Intern Med. (1984) 144:357–60. doi: 10.1001/archinte.1984.00350140181025
33. Kowlgi, NG, and Chhabra, L. D-lactic acidosis: an Underrecognized complication of short bowel syndrome. Gastroenterol Res Pract. (2015) 2015:476215:1–8. doi: 10.1155/2015/476215
34. Harmon, DL, Britton, RA, and Prior, RL. Influence of diet on glucose turnover and rates of gluconeogenesis, oxidation and turnover of D-(−)-lactate in the bovine. J Nutr. (1983) 113:1842–50. doi: 10.1093/jn/113.9.1842
35. Harmon, DL, Britton, RA, and Prior, RL. In vitro rates of oxidation and gluconeogenesis from L(+)- and D(−)lactate in bovine tissues. Comp Biochem Physiol B. (1984) 77:365–8. doi: 10.1016/0305-0491(84)90344-4
36. Jin, S, Chen, X, Yang, J, and Ding, J. Lactate dehydrogenase D is a general dehydrogenase for D-2-Hydroxyacids and is associated with D-lactic acidosis. Nat Commun. (2023) 14:6638. doi: 10.1038/s41467-023-42456-3
37. Kalapos, MP. Methylglyoxal in living organisms: chemistry, biochemistry, toxicology and biological implications. Toxicol Lett. (1999) 110:145–75. doi: 10.1016/s0378-4274(99)00160-5
38. Cristescu, ME, and Egbosimba, EE. Evolutionary history of D-lactate dehydrogenases: a Phylogenomic perspective on functional diversity in the fad binding oxidoreductase/transferase type 4 family. J Mol Evol. (2009) 69:276–87. doi: 10.1007/s00239-009-9274-x
39. Cristescu, ME, Innes, DJ, Stillman, JH, and Crease, TJ. D-and L-lactate dehydrogenases during invertebrate evolution. BMC Evol Biol. (2008) 8:268. doi: 10.1186/1471-2148-8-268
40. Deka, RK, Liu, WZ, Norgard, MV, and Brautigam, CA. Biophysical and biochemical characterization of Tp0037, a D-lactate dehydrogenase, supports an Acetogenic energy conservation pathway in Treponema Pallidum. MBio. (2020) 11:e02249-20. doi: 10.1128/mBio.02249-20
41. Furukawa, N, Miyanaga, A, Nakajima, M, and Taguchi, H. Structural basis of sequential allosteric transitions in tetrameric D-lactate dehydrogenases from three gram-negative Bacteria. Biochemistry. (2018) 57:5388–406. doi: 10.1021/acs.biochem.8b00557
42. Chelstowska, A, Liu, Z, Jia, Y, Amberg, D, and Butow, RA. Signalling between mitochondria and the nucleus regulates the expression of a new D-lactate dehydrogenase activity in yeast. Yeast. (1999) 15:1377–91. doi: 10.1002/(SICI)1097-0061(19990930)15:13<1377::AID-YEA473>3.0.CO;2-0
43. Engqvist, M, Drincovich, MF, Flugge, UI, and Maurino, VG. Two D-2-Hydroxy-acid dehydrogenases in Arabidopsis Thaliana with catalytic capacities to participate in the last reactions of the methylglyoxal and Beta-oxidation pathways. J Biol Chem. (2009) 284:25026–37. doi: 10.1074/jbc.M109.021253
44. Futai, M. Membrane D-lactate dehydrogenase from Escherichia Coli. Purification and properties. Biochemistry. (1973) 12:2468–74. doi: 10.1021/bi00737a016
45. Gleason, FH, and Nolan, RA. D(−)-Lactate Dehydrogenase in Lower Fungi. Science. (1966) 152:1272–3. doi: 10.1126/science.152.3726.1272
46. Ewing, TA, Fraaije, MW, Mattevi, A, and van Berkel, WJH. The Vao/Pcmh Flavoprotein family. Arch Biochem Biophys. (2017) 632:104–17. doi: 10.1016/j.abb.2017.06.022
47. de Bari, L, Atlante, A, Guaragnella, N, Principato, G, and Passarella, S. D-lactate transport and metabolism in rat liver mitochondria. Biochem J. (2002) 365:391–403. doi: 10.1042/BJ20020139
48. Flick, MJ, and Konieczny, SF. Identification of putative mammalian D-lactate dehydrogenase enzymes. Biochem Biophys Res Commun. (2002) 295:910–6. doi: 10.1016/s0006-291x(02)00768-4
49. Drabkin, M, Yogev, Y, Zeller, L, Zarivach, R, Zalk, R, Halperin, D, et al. Hyperuricemia and gout caused by missense mutation in D-lactate dehydrogenase. J Clin Invest. (2019) 129:5163–8. doi: 10.1172/JCI129057
50. Georganas, C, Liu, H, Perlman, H, Hoffmann, A, Thimmapaya, B, and Pope, RM. Regulation of Il-6 and Il-8 expression in rheumatoid arthritis synovial fibroblasts: the dominant role for Nf-kappa B but not C/Ebp Beta or C-Jun. J Immunol. (2000) 165:7199–206. doi: 10.4049/jimmunol.165.12.7199
51. Felmlee, MA, Jones, RS, Rodriguez-Cruz, V, Follman, KE, and Morris, ME. Monocarboxylate transporters (Slc16): function, regulation, and role in health and disease. Pharmacol Rev. (2020) 72:466–85. doi: 10.1124/pr.119.018762
52. Halestrap, AP. Monocarboxylic acid transport. Compr Physiol. (2013) 3:1611–43. doi: 10.1002/cphy.c130008
53. Carpenter, L, and Halestrap, AP. The kinetics, substrate and inhibitor specificity of the lactate transporter of Ehrlich-lettre tumour cells studied with the intracellular Ph Indicator Bcecf. Biochem J. (1994) 304:751–60. doi: 10.1042/bj3040751
54. Tamai, I, Takanaga, H, Maeda, H, Sai, Y, Ogihara, T, Higashida, H, et al. Participation of a proton-cotransporter, Mct1, in the intestinal transport of monocarboxylic acids. Biochem Biophys Res Commun. (1995) 214:482–9. doi: 10.1006/bbrc.1995.2312
55. Shimoyama, Y, Kirat, D, Akihara, Y, Kawasako, K, Komine, M, Hirayama, K, et al. Expression of Monocarboxylate transporter 1 (Mct1) in the dog intestine. J Vet Med Sci. (2007) 69:599–604. doi: 10.1292/jvms.69.599
56. Sivaprakasam, S, Bhutia, YD, Yang, S, and Ganapathy, V. Short-chain fatty acid transporters: role in colonic homeostasis. Compr Physiol. (2017) 8:299–314. doi: 10.1002/cphy.c170014
57. Kirat, D, Inoue, H, Iwano, H, Hirayama, K, Yokota, H, Taniyama, H, et al. Expression and distribution of Monocarboxylate transporter 1 (Mct1) in the gastrointestinal tract of calves. Res Vet Sci. (2005) 79:45–50. doi: 10.1016/j.rvsc.2004.11.007
58. Kirat, D, and Kato, S. Monocarboxylate transporter 1 (Mct1) mediates transport of short-chain fatty acids in bovine Caecum. Exp Physiol. (2006) 91:835–44. doi: 10.1113/expphysiol.2006.033837
59. Kirat, D, Matsuda, Y, Yamashiki, N, Hayashi, H, and Kato, S. Expression, cellular localization, and functional role of Monocarboxylate transporter 4 (Mct4) in the gastrointestinal tract of ruminants. Gene. (2007) 391:140–9. doi: 10.1016/j.gene.2006.12.020
60. Kirat, D, Sallam, KI, and Kato, S. Expression and cellular localization of Monocarboxylate transporters (Mct2, Mct7, and Mct8) along the cattle gastrointestinal tract. Cell Tissue Res. (2013) 352:585–98. doi: 10.1007/s00441-013-1570-5
61. Aschenbach, JR, Penner, GB, Stumpff, F, and Gabel, G. Ruminant nutrition symposium: role of fermentation acid absorption in the regulation of ruminal Ph. J Anim Sci. (2011) 89:1092–107. doi: 10.2527/jas.2010-3301
62. Harmon, DL, Britton, RA, Prior, RL, and Stock, RA. Net portal absorption of lactate and volatile fatty acids in steers experiencing glucose-induced acidosis or fed a 70% concentrate diet ad libitum. J Anim Sci. (1985) 60:560–9. doi: 10.2527/jas1985.602560x
63. Moller, PD, Diernaes, L, Sehested, J, Hyldgaard-Jensen, J, and Skadhauge, E. Absorption and fate of L-and D-lactic acid in ruminants. Comp Biochem Physiol A Physiol. (1997) 118:387–8. doi: 10.1016/s0300-9629(96)00325-8
64. Williams, VJ, and Mackenzie, DD. The absorption of lactic acid from the Reticulo-rumen of the sheep. Aust J Biol Sci. (1965) 18:917–34. doi: 10.1071/bi9650917
65. Manning Fox, JE, Meredith, D, and Halestrap, AP. Characterisation of human Monocarboxylate transporter 4 substantiates its role in lactic acid efflux from skeletal muscle. J Physiol. (2000) 529 Pt 2:285–93. doi: 10.1111/j.1469-7793.2000.00285.x
66. Dimmer, KS, Friedrich, B, Lang, F, Deitmer, JW, and Broer, S. The low-affinity Monocarboxylate transporter Mct4 is adapted to the export of lactate in highly glycolytic cells. Biochem J. (2000) 350:219–27. doi: 10.1042/bj3500219
67. Adeva-Andany, M, Lopez-Ojen, M, Funcasta-Calderon, R, Ameneiros-Rodriguez, E, Donapetry-Garcia, C, Vila-Altesor, M, et al. Comprehensive review on lactate metabolism in human health. Mitochondrion. (2014) 17:76–100. doi: 10.1016/j.mito.2014.05.007
68. Danscher, AM, Enemark, HL, Andersen, PH, Aalbaek, B, and Nielsen, OL. Polysynovitis after Oligofructose overload in dairy cattle. J Comp Pathol. (2010) 142:129–38. doi: 10.1016/j.jcpa.2009.09.001
69. Danscher, AM, Enemark, JM, Telezhenko, E, Capion, N, Ekstrom, CT, and Thoefner, MB. Oligofructose overload induces lameness in cattle. J Dairy Sci. (2009) 92:607–16. doi: 10.3168/jds.2008-1271
70. Passos, LT, Bettencourt, AF, Ritt, LA, Canozzi, MEA, and Fischer, V. Systematic review of the relationship between rumen acidosis and laminitis in cattle. Res Vet Sci. (2023) 161:110–7. doi: 10.1016/j.rvsc.2023.06.001
71. Mgasa, MN. Bovine Pododermatitis Aseptica Diffusa (laminitis) Aetiology, pathogenesis, treatment and control. Vet Res Commun. (1987) 11:235–41. doi: 10.1007/BF00570921
72. Morrow, LL, Tumbleson, ME, Kintner, LD, Pfander, WH, and Preston, RL. Laminitis in lambs injected with lactic acid. Am J Vet Res. (1973) 34:1305–7.
73. Andersson, L, and Liberg, P. Blood serum and synovial fluid in bovine laminitis and arthritis, with particular reference to the protein composition. Acta Vet Scand. (1980) 21:567–77. doi: 10.1186/BF03546844
74. Hidalgo, AI, Carretta, MD, Alarcon, P, Manosalva, C, Muller, A, Navarro, M, et al. Pro-inflammatory mediators and neutrophils are increased in synovial fluid from heifers with acute ruminal acidosis. BMC Vet Res. (2019) 15:225. doi: 10.1186/s12917-019-1974-x
75. Alarcon, P, Hidalgo, AI, Manosalva, C, Cristi, R, Teuber, S, Hidalgo, MA, et al. Metabolic disturbances in synovial fluid are involved in the onset of synovitis in heifers with acute ruminal acidosis. Sci Rep. (2019) 9:5452. doi: 10.1038/s41598-019-42007-1
76. Kortekangas, P, Peltola, O, Toivanen, A, and Aro, HT. Synovial fluid L-lactic acid in acute arthritis of the adult knee joint. Scand J Rheumatol. (1995) 24:98–101. doi: 10.3109/03009749509099292
77. Riordan, T, Doyle, D, and Tabaqchali, S. Synovial fluid lactic acid measurement in the diagnosis and Management of Septic Arthritis. J Clin Pathol. (1982) 35:390–4. doi: 10.1136/jcp.35.4.390
78. Gerster, JC, and Gobelet, C. Synovial fluid lactic acid in acute and chronic pyrophosphate Arthropathy and in osteoarthritis. Clin Rheumatol. (1988) 7:197–9. doi: 10.1007/BF02204454
79. Huffman, KM, Bowers, JR, Dailiana, Z, Huebner, JL, Urbaniak, JR, and Kraus, VB. Synovial fluid metabolites in osteonecrosis. Rheumatology (Oxford). (2007) 46:523–8. doi: 10.1093/rheumatology/kel302
80. Gobelet, C, and Gerster, JC. Synovial fluid lactate levels in septic and non-septic Arthritides. Ann Rheum Dis. (1984) 43:742–5. doi: 10.1136/ard.43.5.742
81. Lenski, M, and Scherer, MA. Analysis of synovial inflammatory markers to differ infectious from gouty arthritis. Clin Biochem. (2014) 47:49–55. doi: 10.1016/j.clinbiochem.2013.10.019
82. Yang, XY, Zheng, KD, Lin, K, Zheng, G, Zou, H, Wang, JM, et al. Energy metabolism disorder as a contributing factor of rheumatoid arthritis: a comparative proteomic and Metabolomic study. PLoS One. (2015) 10:e0132695. doi: 10.1371/journal.pone.0132695
83. Wang, Q, Asenso, J, Xiao, N, Gao, J, Xiao, F, Kuai, J, et al. Lactic acid regulation: a potential therapeutic option in rheumatoid arthritis. J Immunol Res. (2022) 2022:2280973–11. doi: 10.1155/2022/2280973
84. Yi, O, Lin, Y, Hu, M, Hu, S, Su, Z, Liao, J, et al. Lactate metabolism in rheumatoid arthritis: pathogenic mechanisms and therapeutic intervention with natural compounds. Phytomedicine. (2022) 100:154048. doi: 10.1016/j.phymed.2022.154048
85. Zou, Y, Zeng, S, Huang, M, Qiu, Q, Xiao, Y, Shi, M, et al. Inhibition of 6-Phosphofructo-2-kinase suppresses fibroblast-like Synoviocytes-mediated synovial inflammation and joint destruction in rheumatoid arthritis. Br J Pharmacol. (2017) 174:893–908. doi: 10.1111/bph.13762
86. Nemeth, T, Nagy, G, and Pap, T. Synovial fibroblasts as potential drug targets in rheumatoid arthritis, where do we stand and where shall we go? Ann Rheum Dis. (2022) 81:1055–64. doi: 10.1136/annrheumdis-2021-222021
87. Han, D, Fang, Y, Tan, X, Jiang, H, Gong, X, Wang, X, et al. The emerging role of fibroblast-like Synoviocytes-mediated synovitis in osteoarthritis: an update. J Cell Mol Med. (2020) 24:9518–32. doi: 10.1111/jcmm.15669
88. Nygaard, G, and Firestein, GS. Restoring synovial homeostasis in rheumatoid arthritis by targeting fibroblast-like Synoviocytes. Nat Rev Rheumatol. (2020) 16:316–33. doi: 10.1038/s41584-020-0413-5
89. Yoshitomi, H. Regulation of immune responses and chronic inflammation by fibroblast-like Synoviocytes. Front Immunol. (2019) 10:1395. doi: 10.3389/fimmu.2019.01395
90. Manosalva, C, Quiroga, J, Teuber, S, Cardenas, S, Carretta, MD, Moran, GG, et al. D-lactate increases cytokine production in bovine fibroblast-like Synoviocytes via Mct1 uptake and the Mapk, Pi3k/Akt, and Nfkappab pathways. Animals (Basel). (2020) 10:2105. doi: 10.3390/ani10112105
91. Quiroga, J, Alarcon, P, Manosalva, C, Teuber, S, Taubert, A, Hermosilla, C, et al. Metabolic reprogramming and inflammatory response induced by D-lactate in bovine fibroblast-like Synoviocytes depends on Hif-1 activity. Front Vet Sci. (2021) 8:625347. doi: 10.3389/fvets.2021.625347
92. Pucino, V, Certo, M, Bulusu, V, Cucchi, D, Goldmann, K, Pontarini, E, et al. Lactate buildup at the site of chronic inflammation promotes disease by inducing Cd4(+) T cell metabolic rewiring. Cell Metab. (2019) 30:1055–1074.e8. doi: 10.1016/j.cmet.2019.10.004
93. Payen, VL, Mina, E, Van Hee, VF, Porporato, PE, and Sonveaux, P. Monocarboxylate transporters in cancer. Mol Metab. (2020) 33:48–66. doi: 10.1016/j.molmet.2019.07.006
94. Ahn, JK, Kim, S, Hwang, J, Kim, J, Kim, KH, and Cha, HS. Gc/Tof-Ms-based Metabolomic profiling in cultured fibroblast-like Synoviocytes from rheumatoid arthritis. Joint Bone Spine. (2016) 83:707–13. doi: 10.1016/j.jbspin.2015.11.009
95. de Oliveira, PG, Farinon, M, Sanchez-Lopez, E, Miyamoto, S, and Guma, M. Fibroblast-like Synoviocytes glucose metabolism as a therapeutic target in rheumatoid arthritis. Front Immunol. (2019) 10:1743. doi: 10.3389/fimmu.2019.01743
96. Pucino, V, Nefla, M, Gauthier, V, Alsaleh, G, Clayton, SA, Marshall, J, et al. Differential effect of lactate on synovial fibroblast and macrophage effector functions. Front Immunol. (2023) 14:1183825. doi: 10.3389/fimmu.2023.1183825
97. Schoenmann, N, Tannenbaum, N, Hodgeman, RM, and Raju, RP. Regulating mitochondrial metabolism by targeting pyruvate dehydrogenase with Dichloroacetate, a metabolic messenger. Biochim Biophys Acta Mol basis Dis. (2023) 1869:166769. doi: 10.1016/j.bbadis.2023.166769
98. Bian, L, Josefsson, E, Jonsson, IM, Verdrengh, M, Ohlsson, C, Bokarewa, M, et al. Dichloroacetate alleviates development of collagen ii-induced arthritis in female dba/1 mice. Arthritis Res Ther. (2009) 11:R132. doi: 10.1186/ar2799
99. Manosalva, C, Alarcon, P, Quiroga, J, Teuber, S, Carretta, MD, Bustamante, H, et al. Bovine tumor necrosis factor-alpha increases Il-6, Il-8, and Pge2 in bovine fibroblast-like Synoviocytes by metabolic reprogramming. Sci Rep. (2023) 13:3257. doi: 10.1038/s41598-023-29851-y
100. Wen, ZH, Sung, CS, Lin, SC, Yao, ZK, Lai, YC, Liu, YW, et al. Intra-articular lactate dehydrogenase a inhibitor Oxamate reduces experimental osteoarthritis and nociception in rats via possible alteration of glycolysis-related protein expression in cartilage tissue. Int J Mol Sci. (2023) 24:10770. doi: 10.3390/ijms241310770
101. Bassel, LL, and Caswell, JL. Bovine neutrophils in health and disease. Cell Tissue Res. (2018) 371:617–37. doi: 10.1007/s00441-018-2789-y
102. Xie, L, Ma, Y, Opsomer, G, Pascottini, OB, Guan, Y, and Dong, Q. Neutrophil extracellular traps in cattle health and disease. Res Vet Sci. (2021) 139:4–10. doi: 10.1016/j.rvsc.2021.06.019
103. Wigerblad, G, and Kaplan, MJ. Neutrophil extracellular traps in systemic autoimmune and autoinflammatory diseases. Nat Rev Immunol. (2023) 23:274–88. doi: 10.1038/s41577-022-00787-0
104. Xiang, M, Yin, M, Xie, S, Shi, L, Nie, W, Shi, B, et al. The molecular mechanism of neutrophil extracellular traps and its role in bone and joint disease. Heliyon. (2023) 9:e22920. doi: 10.1016/j.heliyon.2023.e22920
105. Alarcon, P, Manosalva, C, Conejeros, I, Carretta, MD, Munoz-Caro, T, Silva, LMR, et al. D(−) lactic acid-induced adhesion of bovine neutrophils onto endothelial cells is dependent on neutrophils extracellular traps formation and Cd11b expression. Front Immunol. (2017) 8:975. doi: 10.3389/fimmu.2017.00975
106. Awasthi, D, Nagarkoti, S, Sadaf, S, Chandra, T, Kumar, S, and Dikshit, M. Glycolysis dependent lactate formation in neutrophils: a metabolic link between Nox-dependent and independent Netosis. Biochim Biophys Acta Mol basis Dis. (2019) 1865:165542. doi: 10.1016/j.bbadis.2019.165542
107. Shi, Y, Shi, H, Nieman, DC, Hu, Q, Yang, L, Liu, T, et al. Lactic acid accumulation during exhaustive exercise impairs release of neutrophil extracellular traps in mice. Front Physiol. (2019) 10:709. doi: 10.3389/fphys.2019.00709
108. Quiroga, J, Alarcon, P, Ramirez, MF, Manosalva, C, Teuber, S, Carretta, MD, et al. D-lactate-induced Etosis in cattle Polymorphonuclear leucocytes is dependent on the release of mitochondrial reactive oxygen species and the Pi3k/Akt/Hif-1 and Gsk-3beta pathways. Dev Comp Immunol. (2023) 145:104728. doi: 10.1016/j.dci.2023.104728
109. Quiroga, J, Alarcon, P, Manosalva, C, Teuber, S, Carretta, MD, and Burgos, RA. D-lactate-triggered extracellular trap formation in cattle Polymorphonuclear leucocytes is glucose metabolism dependent. Dev Comp Immunol. (2022) 135:104492. doi: 10.1016/j.dci.2022.104492
110. Peng, S, Gao, J, Stojkov, D, Yousefi, S, and Simon, HU. Established and emerging roles for mitochondria in neutrophils. Immunol Rev. (2023) 314:413–26. doi: 10.1111/imr.13158
111. Zotta, A, O'Neill, LAJ, and Yin, M. Unlocking potential: the role of the Electron transport chain in Immunometabolism. Trends Immunol. (2024) 45:259–73. doi: 10.1016/j.it.2024.02.002
112. Vorobjeva, NV, Chelombitko, MA, Sud'ina, GF, Zinovkin, RA, and Chernyak, BV. Role of mitochondria in the regulation of effector functions of granulocytes. Cells. (2023) 12:2210. doi: 10.3390/cells12182210
113. Kumar, S, and Dikshit, M. Metabolic insight of neutrophils in health and disease. Front Immunol. (2019) 10:2099. doi: 10.3389/fimmu.2019.02099
114. Fossati, G, Moulding, DA, Spiller, DG, Moots, RJ, White, MR, and Edwards, SW. The mitochondrial network of human neutrophils: role in chemotaxis, phagocytosis, respiratory burst activation, and commitment to apoptosis. J Immunol. (2003) 170:1964–72. doi: 10.4049/jimmunol.170.4.1964
115. Maianski, NA, Geissler, J, Srinivasula, SM, Alnemri, ES, Roos, D, and Kuijpers, TW. Functional characterization of mitochondria in neutrophils: a role restricted to apoptosis. Cell Death Differ. (2004) 11:143–53. doi: 10.1038/sj.cdd.4401320
116. Quiroga, J, Alarcon, P, Manosalva, C, Taubert, A, Hermosilla, C, Hidalgo, MA, et al. Glycolysis and mitochondrial function regulate the radical oxygen species production induced by platelet-activating factor in bovine Polymorphonuclear leukocytes. Vet Immunol Immunopathol. (2020) 226:110074. doi: 10.1016/j.vetimm.2020.110074
117. Quiroga, J, Alarcon, P, Manosalva, C, Taubert, A, Hermosilla, C, Hidalgo, MA, et al. Mitochondria-derived Atp participates in the formation of neutrophil extracellular traps induced by platelet-activating factor through purinergic signaling in cows. Dev Comp Immunol. (2020) 113:103768. doi: 10.1016/j.dci.2020.103768
118. Vorobjeva, NV, and Chernyak, BV. Netosis: molecular mechanisms, role in physiology and pathology. Biochemistry (Mosc). (2020) 85:1178–90. doi: 10.1134/S0006297920100065
119. Yin, M, and O'Neill, LAJ. The role of the Electron transport chain in immunity. FASEB J. (2021) 35:e21974. doi: 10.1096/fj.202101161R
120. Douda, DN, Khan, MA, Grasemann, H, and Palaniyar, N. Sk3 channel and mitochondrial Ros mediate Nadph oxidase-independent Netosis induced by calcium influx. Proc Natl Acad Sci USA. (2015) 112:2817–22. doi: 10.1073/pnas.1414055112
121. Dunham-Snary, KJ, Surewaard, BG, Mewburn, JD, Bentley, RE, Martin, AY, Jones, O, et al. Mitochondria in human neutrophils mediate killing of Staphylococcus Aureus. Redox Biol. (2022) 49:102225. doi: 10.1016/j.redox.2021.102225
122. Fortner, KA, Blanco, LP, Buskiewicz, I, Huang, N, Gibson, PC, Cook, DL, et al. Targeting mitochondrial oxidative stress with Mitoq reduces net formation and kidney disease in lupus-prone Mrl-Lpr mice. Lupus Sci Med. (2020) 7:e000387. doi: 10.1136/lupus-2020-000387
123. Lood, C, Blanco, LP, Purmalek, MM, Carmona-Rivera, C, De Ravin, SS, Smith, CK, et al. Neutrophil extracellular traps enriched in oxidized mitochondrial DNA are Interferogenic and contribute to lupus-like disease. Nat Med. (2016) 22:146–53. doi: 10.1038/nm.4027
124. Peng, Y, Wu, X, Zhang, S, Deng, C, Zhao, L, Wang, M, et al. The potential roles of type I interferon activated neutrophils and neutrophil extracellular traps (Nets) in the pathogenesis of primary Sjogren's syndrome. Arthritis Res Ther. (2022) 24:170. doi: 10.1186/s13075-022-02860-4
125. Huang, YF, Wang, G, Ding, L, Bai, ZR, Leng, Y, Tian, JW, et al. Lactate-upregulated Nadph-dependent Nox4 expression via Hcar1/Pi3k pathway contributes to Ros-induced osteoarthritis chondrocyte damage. Redox Biol. (2023) 67:102867. doi: 10.1016/j.redox.2023.102867
126. Kozlov, AM, Lone, A, Betts, DH, and Cumming, RC. Lactate preconditioning promotes a Hif-1alpha-mediated metabolic shift from Oxphos to glycolysis in Normal human diploid fibroblasts. Sci Rep. (2020) 10:8388. doi: 10.1038/s41598-020-65193-9
127. Willson, JA, Arienti, S, Sadiku, P, Reyes, L, Coelho, P, Morrison, T, et al. Neutrophil Hif-1alpha stabilization is augmented by mitochondrial Ros produced via the glycerol 3-phosphate shuttle. Blood. (2022) 139:281–6. doi: 10.1182/blood.2021011010
128. Zhang, D, Tang, Z, Huang, H, Zhou, G, Cui, C, Weng, Y, et al. Metabolic regulation of gene expression by histone Lactylation. Nature. (2019) 574:575–80. doi: 10.1038/s41586-019-1678-1
129. Chen, L, Huang, L, Gu, Y, Cang, W, Sun, P, and Xiang, Y. Lactate-Lactylation hands between metabolic reprogramming and immunosuppression. Int J Mol Sci. (2022) 23:11943. doi: 10.3390/ijms231911943
130. Xin, Q, Wang, H, Li, Q, Liu, S, Qu, K, Liu, C, et al. Lactylation: a passing fad or the future of posttranslational modification. Inflammation. (2022) 45:1419–29. doi: 10.1007/s10753-022-01637-w
131. Moreno-Yruela, C, Zhang, D, Wei, W, Baek, M, Liu, W, Gao, J, et al. Class I histone deacetylases (Hdac1-3) are histone lysine Delactylases. Sci Adv. (2022) 8:eabi6696. doi: 10.1126/sciadv.abi6696
132. Latham, T, Mackay, L, Sproul, D, Karim, M, Culley, J, Harrison, DJ, et al. Lactate, a product of glycolytic metabolism, inhibits histone deacetylase activity and promotes changes in gene expression. Nucleic Acids Res. (2012) 40:4794–803. doi: 10.1093/nar/gks066
133. Wagner, W, Ciszewski, WM, and Kania, KD. L-and D-lactate enhance DNA repair and modulate the resistance of cervical carcinoma cells to anticancer drugs via histone deacetylase inhibition and Hydroxycarboxylic acid receptor 1 activation. Cell Commun Signal. (2015) 13:36. doi: 10.1186/s12964-015-0114-x
134. Han, S, Bao, X, Zou, Y, Wang, L, Li, Y, Yang, L, et al. D-lactate modulates M2 tumor-associated macrophages and remodels immunosuppressive tumor microenvironment for hepatocellular carcinoma. Sci Adv. (2023) 9:eadg2697. doi: 10.1126/sciadv.adg2697
135. Zhao, G, Gao, Y, Zhang, J, Zhang, H, Xie, C, Nan, F, et al. Toll-like receptor 2 signaling pathway activation contributes to a highly efficient inflammatory response in Japanese encephalitis virus-infected mouse microglial cells by proteomics. Front Microbiol. (2022) 13:989183. doi: 10.3389/fmicb.2022.989183
136. Xu, X, Wang, G, Ai, L, Shi, J, Zhang, J, and Chen, YX. Melatonin suppresses Tlr9-triggered Proinflammatory cytokine production in macrophages by inhibiting Erk1/2 and Akt activation. Sci Rep. (2018) 8:15579. doi: 10.1038/s41598-018-34011-8
137. Yan, Y, Li, X, Yang, Q, Zhang, H, Hettinga, K, Li, H, et al. Dietary D-lactate intake facilitates inflammatory resolution by modulating M1 macrophage polarization. Mol Nutr Food Res. (2022) 66:e2200196. doi: 10.1002/mnfr.202200196
138. Wagner, W, Ciszewski, W, Kania, K, and Dastych, J. Lactate stimulates Il-4 and Il-13 production in activated hut-78 T lymphocytes through a process that involves Monocarboxylate transporters and protein Hyperacetylation. J Interf Cytokine Res. (2016) 36:317–27. doi: 10.1089/jir.2015.0086
139. Cai, X, Ng, CP, Jones, O, Fung, TS, Ryu, KW, Li, D, et al. Lactate activates the mitochondrial Electron transport chain independently of its metabolism. Mol Cell. (2023) 83:3904–3920.e7. doi: 10.1016/j.molcel.2023.09.034
140. To TLMcCoy, JG, Ostriker, NK, Sandler, LS, Mannella, CA, and Mootha, VK. Pmf-Seq: a highly scalable screening strategy for linking genetics to mitochondrial bioenergetics. Nat Metab. (2024) 6:687–96. doi: 10.1038/s42255-024-00994-0
141. Lin, L, Ren, R, Xiong, Q, Zheng, C, Yang, B, and Wang, H. Remodeling of T-cell mitochondrial metabolism to treat autoimmune diseases. Autoimmun Rev. (2024) 23:103583. doi: 10.1016/j.autrev.2024.103583
142. Chen, X, Sunkel, B, Wang, M, Kang, S, Wang, T, Gnanaprakasam, JNR, et al. Succinate dehydrogenase/complex ii is critical for metabolic and epigenetic regulation of T cell proliferation and inflammation. Sci Immunol. (2022) 7:eabm8161. doi: 10.1126/sciimmunol.abm8161
143. Beier, UH, Jiao, J, Xiao, H, Angelin, A, Wallace, DC, Levine, M, et al. Learning from Cancer: using D-lactate for therapeutic immunosuppression. J Immunol. (2016) 196:140.15. doi: 10.4049/jimmunol.196.Supp.140.15
144. Cai, WW, Yu, Y, Zong, SY, and Wei, F. Metabolic reprogramming as a key regulator in the pathogenesis of rheumatoid arthritis. Inflamm Res. (2020) 69:1087–101. doi: 10.1007/s00011-020-01391-5
Keywords: bovine, D-lactate, inflammation, ruminal acidosis, lameness
Citation: Burgos RA, Manosalva C, Alarcón P, Navarro M, Quiroga J, Morán G, Gallastegui J, Brauchi S and Carretta MD (2024) The D-lactate enigma: exploring the inflammatory influence of D-lactate in cattle. Front. Vet. Sci. 11:1509399. doi: 10.3389/fvets.2024.1509399
Edited by:
Zhicheng Peng, University of Pennsylvania, United StatesReviewed by:
Qiushi Xu, Heilongjiang Bayi Agricultural University, ChinaMengxu Ge, Harvard Medical School, United States
Copyright © 2024 Burgos, Manosalva, Alarcón, Navarro, Quiroga, Morán, Gallastegui, Brauchi and Carretta. This is an open-access article distributed under the terms of the Creative Commons Attribution License (CC BY). The use, distribution or reproduction in other forums is permitted, provided the original author(s) and the copyright owner(s) are credited and that the original publication in this journal is cited, in accordance with accepted academic practice. No use, distribution or reproduction is permitted which does not comply with these terms.
*Correspondence:Rafael Agustín Burgos, cmJ1cmdvczFAdWFjaC5jbA==