- 1Research Group in Biotechnology Applied to Animal Health, Production and Conservation (SANIGEN), Laboratory of Biology and Molecular Genetics, Faculty of Veterinary Medicine, Universidad Nacional Mayor de San Marcos, Lima, Peru
- 2Asociación Equipo Primatológico del Perú (EPP), Iquitos, Peru
- 3Programa de Pós-Graduação Interunidades em Bioinformática, Instituto de Matemática e Estatística, Universidade de São Paulo, São Paulo, Brazil
Introduction: Extended-spectrum beta-lactamase (ESBL)-producing Enterobacteriaceae are on the WHO priority pathogens list because they are associated with high mortality, health-care burden, and antimicrobial resistance (AMR), a serious problem that threatens global public health and should be addressed through the One Health approach. Non-human primates (NHP) have a high risk of acquiring these antibiotic-resistant bacteria due to their close phylogenetic relationship with humans and increased anthropogenic activities in their natural environments. This study aimed to detect and analyze the genomes of ESBL-producing Escherichia coli (ESBL-producing E. coli) in NHP from the Peruvian Amazon.
Materials and methods: We collected a total of 119 fecal samples from semi-captive Saguinus labiatus, Saguinus mystax, and Saimiri boliviensis, and captive Ateles chamek, Cebus unicolor, Lagothrix lagothricha, and Sapajus apella in the Loreto and Ucayali regions, respectively. Subsequently, we isolated and identified E. coli strains by microbiological methods, detected ESBL-producing E. coli through antimicrobial susceptibility tests following CLSI guidelines, and analyzed their genomes using previously described genomic methods.
Results: We detected that 7.07% (7/99) of E. coli strains: 5.45% (3/55) from Loreto and 9.09% (4/44) from Ucayali, expressed ESBL phenotype. Genomic analysis revealed the presence of high-risk pandemic clones, such as ST10 and ST117, carrying a broad resistome to relevant antibiotics, including three blaCTX-M variants: blaCTX-M-15, blaCTX-M-55, and blaCTX-M-65. Phylogenomic analysis confirmed the clonal relatedness of high-risk lineages circulating at the human-NHP interface. Additionally, two ESBL-producing E. coli strains were identified as EPEC (eae) and ExPEC according to their virulence profiles, and one more presented a hypermucoviscous phenotype.
Discussion: We report the detection and genomic analysis of seven ESBL-producing E. coli strains carrying broad resistome and virulence factors in NHP from two regions of the Peruvian Amazon. Some of these strains are closely related to high-risk pandemic lineages previously reported in humans and domestic animals, highlighting the negative impact of anthropogenic activities on Amazonian wildlife. To our knowledge, this is the first documentation of ESBL-producing E. coli in NHP from the Amazon, underscoring the importance of adopting the One Health approach to AMR surveillance and minimizing the potential transmission risk of antibiotic-resistant bacteria at the human-NHP interface.
1 Introduction
The emergence and spread of antimicrobial resistance (AMR) represent a major threat to global public health, with low and middle-income countries (LMICs) disproportionately affected (1, 2). The AMR challenge in LMICs extended beyond medical treatments for the human population, as a high prevalence of antibiotic-resistant bacteria is observed in intensive livestock production due to the unregulated use of antibiotics as growth promoters, despite being prohibited in many countries, along with inadequate veterinary treatments (3). Antibiotic-resistant bacteria from healthcare and farm settings may run off into natural environments, eventually reaching wildlife. Consequently, there is an increased need to promote a One Health approach to comprehend and address the emergence and evolution of AMR (4, 5).
According to the World Health Organization (WHO), extended-spectrum beta-lactamase (ESBL) producing Enterobacteriaceae rank among the most critical antibiotic-resistant bacteria (6). These bacteria produce enzymes that hydrolyze beta-lactam antibiotics, including third- and fourth generation cephalosporins. The blaCTX-M gene family, the most widespread and clinically relevant ESBL enzyme, can be easily transmitted via plasmids, facilitating rapid spread and dissemination (7). There is evidence demonstrating the presence of ESBL-producing Escherichia coli (ESBL-producing E. coli) in the gut microbiomes of domestic and wild animals, with rapid dissemination observed in wildlife around the world (8–10).
Wild animals, including non-human primates (NHP), are generally not exposed to antibiotics; however, they can acquire antibiotic-resistant bacteria, such as ESBL-producing E. coli, through foraging and drinking in natural environments contaminated by anthropogenic sources (11). Moreover, forest fragmentation resulting from anthropogenic activities such as agriculture, livestock, and forestry, along with changes in the lifestyle of free-living NHP transitioning to captive and semi-captive conditions, can increase the interaction between humans and NHP and enhance the spread of these antibiotic-resistant bacteria (12).
Peru boasts an wide biodiversity of neotropical fauna residing in different regions of the Peruvian Amazon (13), including various NHP classified into 55 taxa comprising species and subspecies. Around 30% of these are designated as “threatened with extinction,” five as “near threatened” and five more as “data deficient,” according to the International Union for the Conservation of Nature (IUCN) (14). However, few studies have investigated AMR in Peruvian Amazon wildlife, and only one has reported antibiotic-resistant Enterobacteriaceae from semi-captive NHP of Ateles, Callicebus, and Lagothrix genus, with E. coli the most prevalent enterobacteria (15), but not detected their antimicrobial resistance genes (ARGs).
This study aimed to detect the presence of ESBL-producing E. coli strains in captive and semi-captive NHP from two regions of the Peruvian Amazon and analyze their genomes. We performed the isolation and identification of E. coli strains using microbiological methods, the phenotypic detection of ESBL-producing E. coli through antimicrobial susceptibility tests according to the Clinical and Laboratory Standard Institute (CLSI), identification of ARGs within the resistome, and the analysis of virulence genes using genomic approaches. Additionally, we conducted a phylogenomic analysis of high-risk lineages.
2 Materials and methods
2.1 Ethics approval statement
This study obtained authorization for scientific research purposes on wildlife outside Protected Natural Areas from the National Forestry and Wildlife Service of Peru (General Directorate Resolution No. RD-000031-2022-MIDAGRI-SERFOR-DGGSPFFS-DGSPFS). All procedures have undergone review and approval by the Ethics and Animal Welfare Committee of the Faculty of Veterinary Medicine of the Universidad Nacional Mayor de San Marcos (Authorization No. 2022-10).
2.2 Fecal sampling collection
In August 2022, we collected a total of 119 fecal samples from semi-captive and captive NHP in two regions of the Peruvian Amazon. Of these, 69 samples belonged to semi-captive NHP specimens, including Saimiri boliviensis (n = 26), Saguinus labiatus (n = 21), and Saguinus mystax (n = 23), that cohabit with humans in rainforests near urban–rural areas on Iquitos, Muyuy, and Padre islands, respectively, in the Loreto region of the northern Peruvian Amazon. Additionally, 50 samples were obtained from captive NHP specimens, including Ateles chamek (n = 14), Cebus unicolor (n = 11), Lagothrix lagothricha (n = 14), and Sapajus apella (n = 11), housed in a Rescue Center and a Zoo that are frequently visited by many local and foreign people, in the Ucayali region of the eastern Peruvian Amazon.
Briefly, we collected between 5 and 10 mg of recently excreted feces using a sterile disposable palette, avoiding the parts that had direct contact with the ground and other surfaces to minimize the risk of sample contamination. The samples were placed inside sterile fecal containers, labeled according to their characteristics (species, sampling region, and living conditions), packed in a cooler container with controlled refrigeration temperature (4°C), and sent to the Laboratory of the Biology and Molecular Genetics of the Faculty of Veterinary Medicine at the Universidad Nacional Mayor de San Marcos for processing using microbiological and molecular methods.
2.3 Microbiological isolation and identification of Escherichia coli strains
The fecal samples were diluted in sterile tubes with isotonic saline solution (0.9% NaCl) in a 1:1 ratio and gently mixed through vortexing. Subsequently, the samples were streaked onto MacConkey agar (Sigma-Aldrich, Germany) and incubated in an Incucell-ecoline incubator model (MMM Group, Germany) at 37°C for 24 h under aerobic conditions. On the following day, all lactose-positive colonies displaying a brick-red to rosaceous color surrounded by a zone of precipitated bile were selected and streaked onto Eosin-Methylene Blue (EMB) agar, followed by incubation under the same conditions. Colonies exhibiting a metallic green luster were then chosen and confirmed through IMViC (Indole, Methyl Red, Voges-Proskauer and Citrate test) biochemical tests (Merck, Germany). Additionally, a “String” test was also conducted on all strains with a mucoid appearance using sterile microbiological loops. This test involved inoculating our strains onto blood agar (Merck, Germany) with 5% sheep blood and incubating them at 37°C overnight, as previously described (16).
2.4 Antimicrobial susceptibility testing of Escherichia coli strains
Antimicrobial susceptibility testing was conducted using the Kirby-Bauer disk diffusion method, following the CLSI recommendations (17). In brief, E. coli strains were diluted in sterile tubes with distilled water to a turbidity of 0.5 on the McFarland standard scale. They were then transferred with sterile swabs onto Petri dishes containing Mueller-Hinton (MH) agar (Millipore, Germany). To determine the ESBL phenotype, a screening test was conducted using ceftazidime (30 μg), cefotaxime (30 μg), ceftriaxone (30 μg), and aztreonam (30 μg) antibiotic disks (Oxoid, United Kingdom). The plates were incubated for 18 h at 37°C under aerobic conditions, and the results were interpreted according to CLSI guidelines.
The following day, a confirmation test based on the Jarlier Method was conducted on all suspected strains. This involved placing an amoxicillin/clavulanic acid (20/10 μg) antibiotic disk in the center of a Petri dish with MH agar previously inoculated, surrounded by the antibiotic disks tested earlier. The dishes were then incubated under the same conditions, and strains that formed a distorted inhibition halo were considered ESBL-positive (18).
Additionally, the resistance profile phenotype was evaluated using oxytetracycline (30 μg), nitrofurantoin (300 μg), levofloxacin (5 μg), gentamicin (10 μg), trimethoprim/sulfamethoxazole (1.25/23.75 μg), and chloramphenicol (30 μg) antibiotics disks. Multidrug-resistant (MDR) strains were identified as those showing resistance to three or more classes of the antibiotics tested, following the definition provided by Magiorakos et al. (19).
2.5 Whole genome sequencing and assembly of ESBL-producing Escherichia coli strains
Total genomic DNA from all ESBL-producing E. coli strains was extracted, and paired-end libraries were sequenced on the Illumina MiSeq platform (Illumina, San Diego, CA, United States) using kit v3 (600-cycle) with 2 × 250 bp reads. To ensure data quality, the obtained FastQ files were trimmed using Trimmomatic (20), and quality assessment was conducted using FastQC (21). The cleaned reads were then submitted to the Enterobase database (22) for further analysis. Assembly, Multilocus sequence type (MLST), Clermont type, Hierarchical Clustering of core genome MLST (HierCC) clusters, and serotype statistics were retrieved from the Enterobase platform. The seven ESBL strains sequenced in this study have been deposited under the BioProject PRJNA992559 of the National Center for Biotechnology Information (NCBI).
2.6 Identification of sequence of interest: ARGs, virulence factors, capsule loci, and plasmids
To identify ARGs, we employed the AMRFinderPlus database (23) within the abriTAMR v 1.0.14 pipeline, applying a 90% identity and 90% coverage cutoff (24). Plasmid replicons, virulence genes, and Klebsiella capsule synthesis loci (K-locus) were identified from whole-genome data using the PlasmidFinder database (25), Virulence Factor Database (26) and Kaptive 2.0.7 (27), respectively. Additionally, gene cluster comparison figures were generated using Clinker v 0.0.27 (28).
2.7 Phylogenetic analysis
The phylogenomic analysis of the E. coli genomes obtained in this study and of the genetically related sequences deposited in Enterobase was conducted according to the HierCC HC50 or MLST scheme. Core alignment was performed using Snippy v 4.6.0.1 Gubbins (29) was employed to detect and eliminate recombinant regions. Maximum likelihood phylogenetic reconstruction was carried out using IQ-TREE v 2.0 (30) with GTR + G + I model and 1,000 bootstrap replicates. The resulting tree was visualized using the ggtree package (31) in the R environment v4.3 (32). Additionally, the pairwise SNP distance between genomes, derived from the free-recombination core genome alignment was calculated using SNP-dists v0.6 (see text footnote 1).
3 Results
3.1 Detection and resistome characterization of ESBL-producing Escherichia coli strains
Among 119 fecal samples obtained from NHP in two regions of the Peruvian Amazon, we isolated 99 E. coli strains. Of these, 55 strains were from semi-captive NHP in Loreto, and 44 were from captive NHP in Ucayali. Antimicrobial susceptibility tests revealed a similar resistance profile in samples from both regions. Approximately 45% of strains from both Loreto and Ucayali exhibited resistance to oxytetracycline and trimethoprim-sulfamethoxazole. Similarly, the proportion of strains resistant to chloramphenicol and gentamicin was below 10%. Additionally, 7.07% (7/99) of all strains—around 5.45% (3/55) from Loreto and 9.09% (4/44) from Ucayali—were identified as ESBL-producing E. coli (Figure 1A).
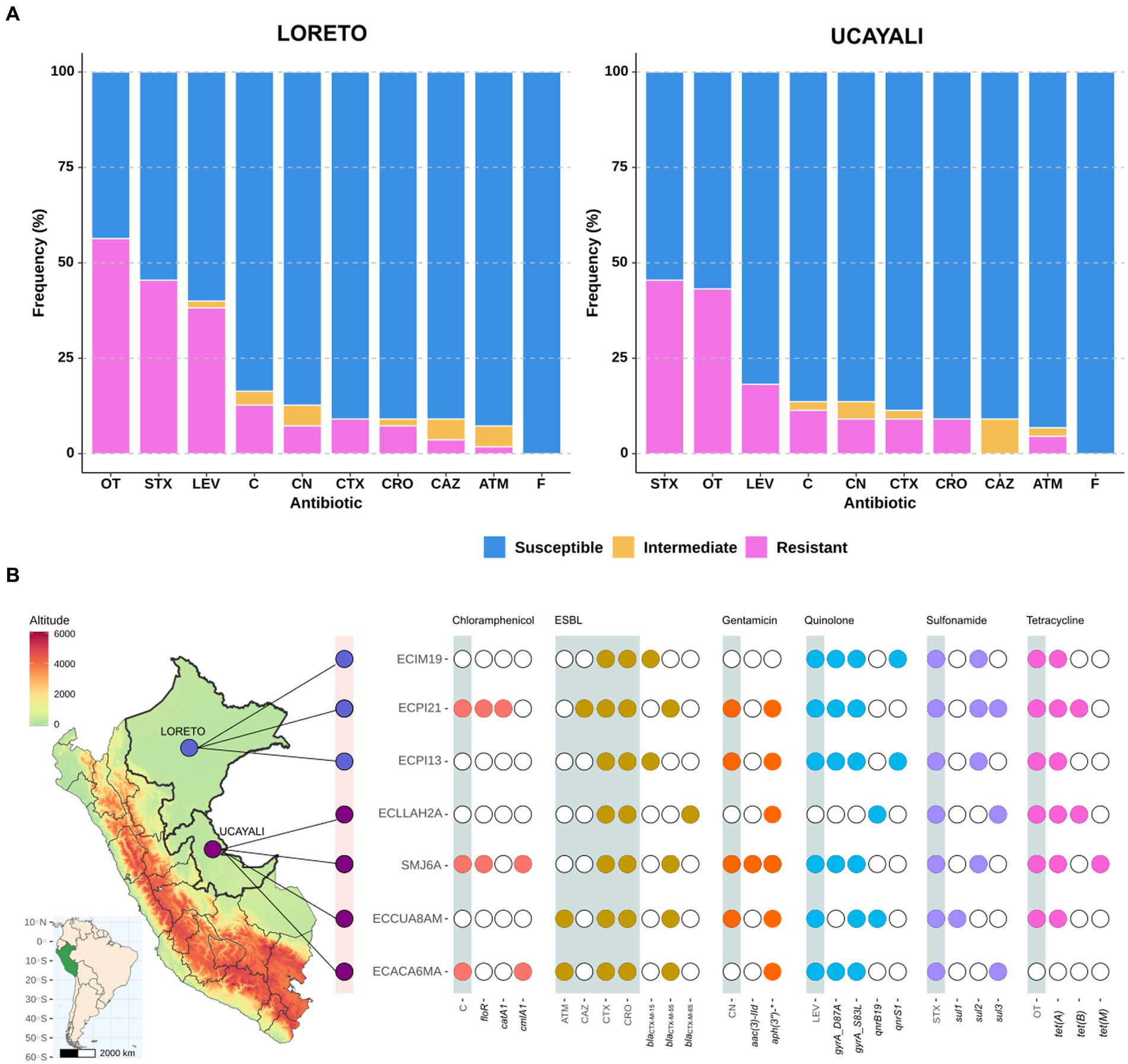
Figure 1. (A) AMR profile of Escherichia coli strains isolated from NHP in Loreto (n = 55) and Ucayali (n = 44) regions of the northeastern Peruvian Amazon; ATM, Aztreonam; C, Chloramphenicol; CAZ, Ceftazidime; CRO, Ceftriaxone; CTX, Cefotaxime; GEN, Gentamicin; LEV, Levofloxacin; STX, Trimethoprim-sulfamethoxazole; OT, Oxytetracycline; and F, Nitrofurantoin. (B) Identification map of Loreto (light purple) and Ucayali (dark purple) regions coupled to a diagram showing AMR phenotype distribution (gray shades) associated with the presence (color points)/absence (white points) of ARGs in seven genomes of ESBL-producing E. coli strains isolated from NHP.
Whole genome sequencing was performed on the seven ESBL-producing E. coli strains to investigate ARGs (Figure 1B). We identified three variants of blaCTM-X: blaCTX-M-15 (two strains), blaCTX-M-55 (four strains), and blaCTX-M-65 (one strain). A broad resistome was also detected, conferring resistance to chloramphenicol (floR, catA1, and cmlA1), aminoglycosides (aac(3″)-IId, aadA, aph(3″)-IIa, aph(6″)-Id), quinolones (qnrB19, qnrS1), sulfonamides (sul1, sul2, and sul3), and tetracyclines (tetA, tetB, and tetM). Point mutations on the gyr gene (gyrA_S83L and gyrA_D87A) associated with quinolones resistance were also identified (Figure 1B). Additionally, genes encoding resistance against fosfomycin (fos3A) and lincomycin (lnuG), as well as a mutation in the nfsA gene (nfsA_R15C) associated with nitrofurantoin resistance, were detected (Supplementary Figure 1).
Among our seven ESBL-producing E. coli strains, five sequence types (ST) were identified: ST10, ST117, ST752, ST7176, and ST12254 (Table 1). Three of these strains (ECIM19, ECPI13, and SMJ6A) belong to the ST10 sequence type, a high-risk pandemic lineage previously reported in other hosts from South America. Additional genomic characteristics are detailed in Table 1 and Supplementary Table 1.
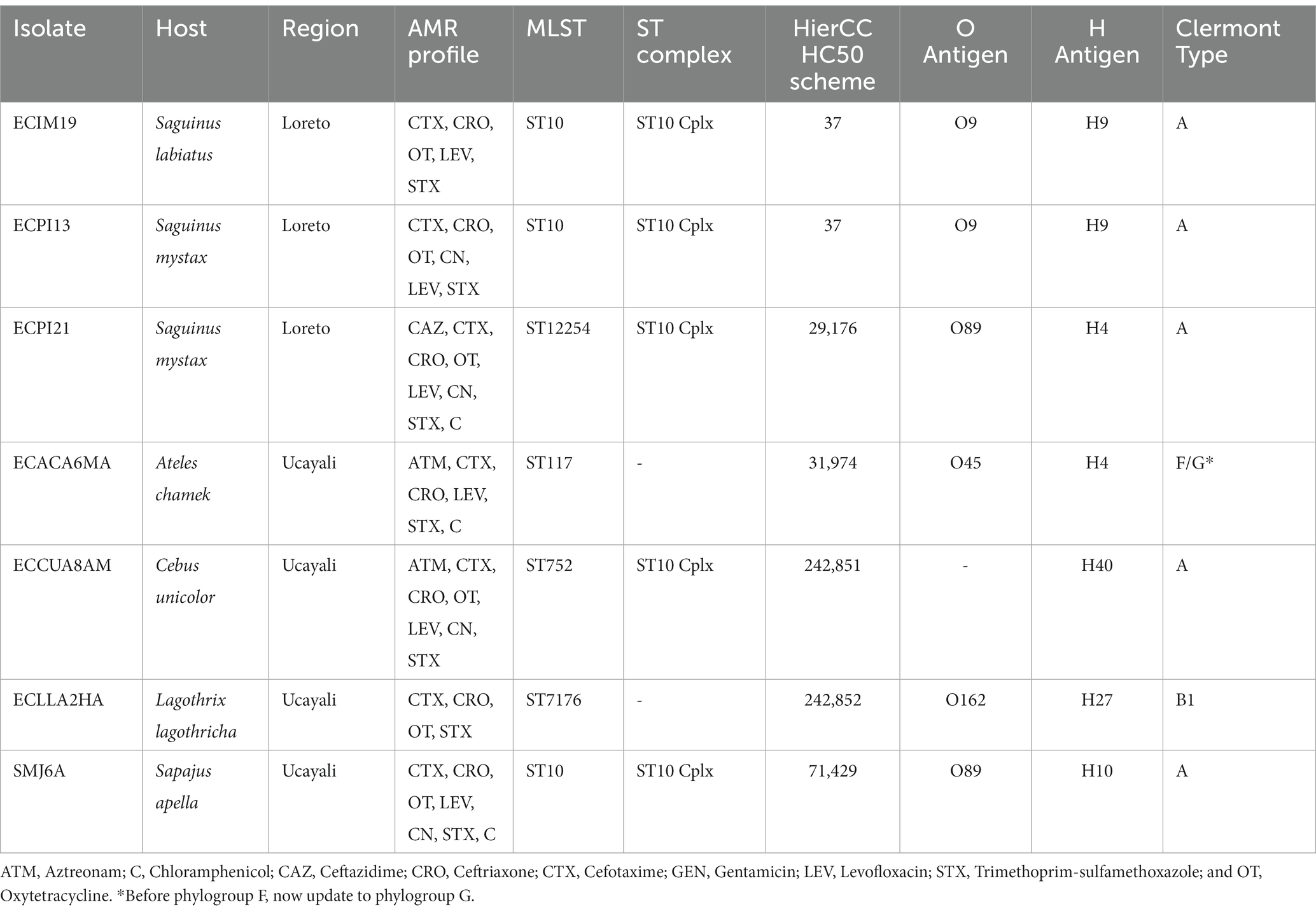
Table 1. Metadata summary of the seven ESBL-producing Escherichia coli genomes isolated from semi-captive and captive NHP in Loreto and Ucayali regions of the Peruvian Amazon.
3.2 Phylogenomic analysis of ESBL-producing Escherichia coli strains
Phylogenomic analysis of the HC50_37 cluster, which includes the ECIM19 strain from S. labiatus and the ECPI13 strain from S. mystax, revealed a SNP distance ranging from 0 to 519 SNPs between strains within this cluster. The majority of strains (n = 58/99) in this cluster harbored blaCTX-M genes, indicating the presence of a high-risk CTX-M-producing E. coli sublineage with a broad host and geographic distribution (Figure 2A). Interestingly, the genomes of the ECIM19 and ECPI13 strains were remarkably similar, differing by only one SNP in the core genome alignment. This close genetic proximity suggests that both strains belong to the same transmission cluster, despite being recovered from two different monkey species (S. labiatus and S. mystax, respectively) on islands located 26.5 km away from each other (Figure 2B). Differences in genetic content between both strains rule out possible laboratory contamination; the ECIM19 strain differentially presents an IncFII plasmid and aph(3)-Ib and aph(6)-Id resistance genes that ECM13 strain lacks (Figure 2C).
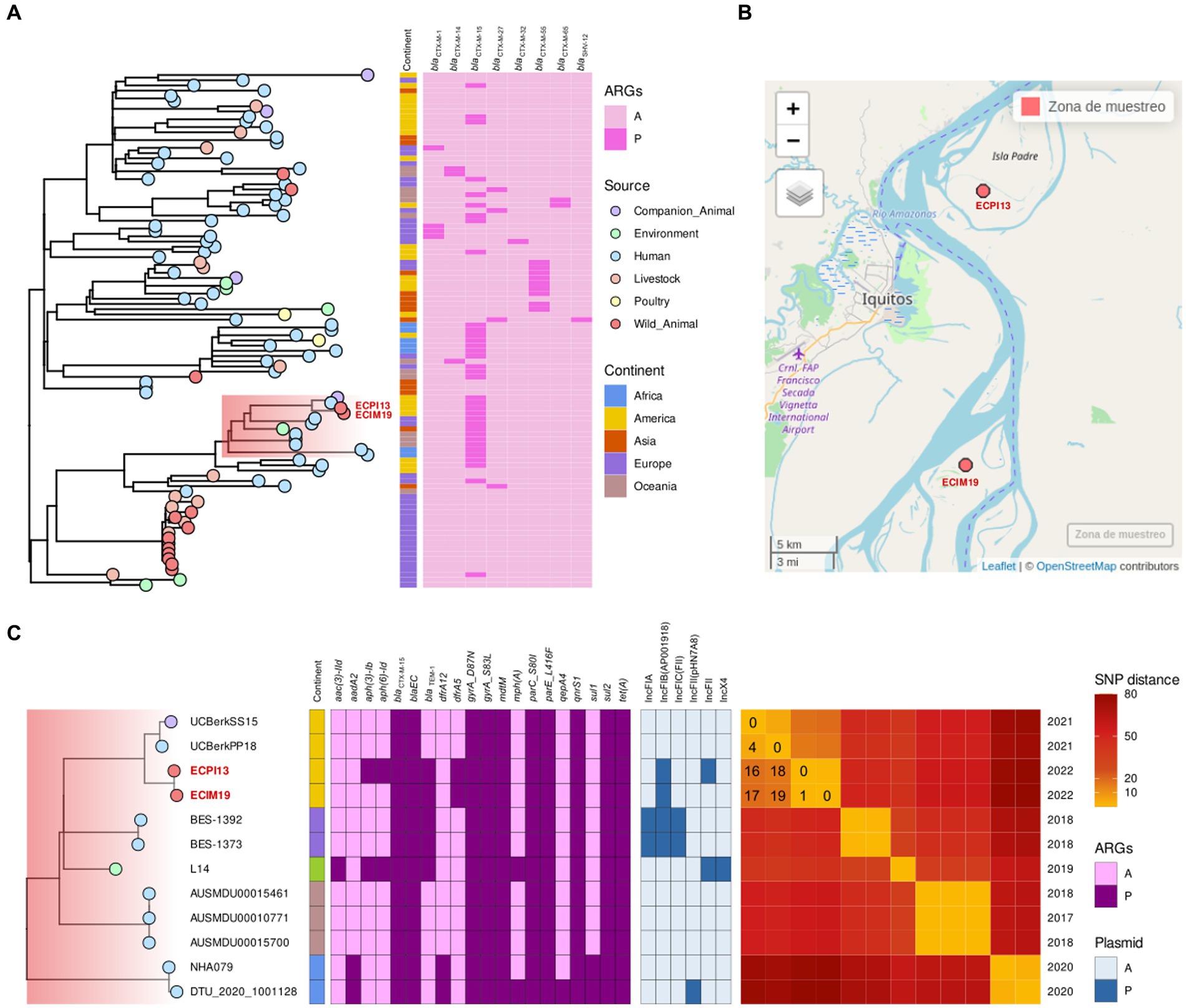
Figure 2. (A) Phylogenetic tree of Escherichia coli ST10 HierCC HC50_37 group downloaded from Enterobase, including ECIM19 and ECPI13 genomes (names in red), showing the source of strains (points), continent reported (squares), and presence (dark pink square)/absence (light pink square) of of blaCTX-M variants. (B) Map of the sampling locations of ECIM19 and ECPI13 isolates in the Loreto region. (C) Subclade highlighted in “A,” including ECIM19 and ECPI13 genomes with closely related genomes, coupled to binary heatmaps, indicating the presence/absence of ARGs and plasmid replicon types, and an SNPs distance heatmap, specifying the divergent SNPs number and the isolation year.
Furthermore, within the subclade (highlighted in red) that includes monkey strains, there are two additional closely related strains: one from a human and one from a dog isolated in Quito, Ecuador, in 2021 (33). The SNP distance between our strains and Ecuadorian strains ranged from 16 to 19 SNPs, respectively, (Figure 2C). All members of this subclade contained the blaCTX-M-15 gene and other ARGs such as qnrS1, sul2, and tet(A). These data provided compelling evidence that a CTM-M-15-producing E. coli clonal strain is widely disseminated among different hosts, including NHP and humans, in geographically separated locations from South America.
Phylogenetic analysis of the SMJ6A strain from S. apella and 19 genomes from HC50_71429 cluster shows that the SMJ6A strain was the most related to FP209CP strain from a pigeon (Columba livia) recovered in Oceania, with a distance of 506 SNPs (Supplementary Figure 2A). Similar results were obtained for the HC50_29176 cluster, where the ECPI21 was closely related to Ecuadorian strains from domestic dogs and swine with SNP distance of 94 and 96 SNPs, respectively, and shared the blaCTX-M-55 gene (Supplementary Figure 2B).
Finally, the phylogenetic analysis of the ST7176 lineage revealed that the ECLLAH2A strain from L. lagothricha was related to two poultry strains from the United States (Supplementary Figure 2C). Unlike the other strains, ECLLAH2A strain was the only one carrying the blaCTX-M-65 gene, while the other strains in the cluster showed the blaCTX-M-55 variant.
3.3 Genetic context of blaCTX-M genes
The genetic context of the blaCTX-M genes is illustrated in Figure 3. The ECIM19 and ECPI13 strains carrying the blaCTX-M-15 variant were associated with the ISEcp1 insertion sequence, identical to the one found in the chromosome of the E. coli 10R strain obtained from a turkey cloacal swab in China (Figure 3A). Additionally, the ECACA6MA, ECCUA8AM, ECPI21, and SMJ6A strains showed the presence of the blaTEM-1 gene adjacent to the ESBL-coding gene blaCTX-M-55 (Figure 3B). Unfortunately, due to the limitations of short-read sequencing, we could not assemble full plasmid sequences and explore the presence of mobile genetic elements surrounding the blaCTX-M-55 and blaCTX-M-65 variants in our strains.
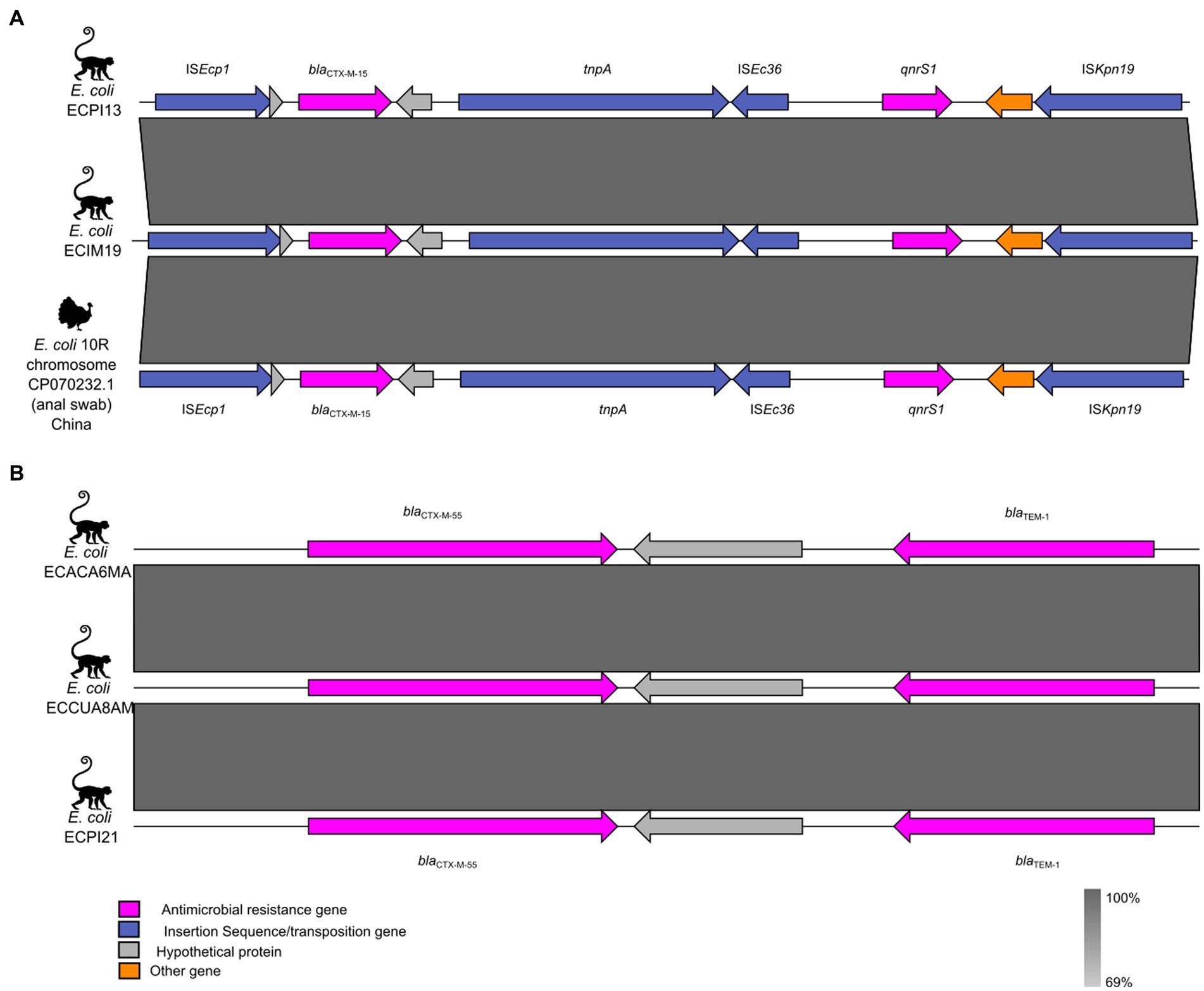
Figure 3. Genetic context of the blaCTX-M variants in ESBL-producing Escherichia coli from NHP. (A) Genetic environment of blaCTX-M-15 and its neighboring mobile genetic elements. (B) Genetic environment of blaCTX-M-55. The ARGs, insertion sequences, and hypothetical proteins are represented by pink, blue, and gray arrows, respectively.
3.4 Detection of virulent and hypermucoviscous ESBL-producing Escherichia coli strains
We identified 33 virulence genes in all seven ESBL-producing E. coli strains (Figure 4A). The genome of the ECCUA8AM strain from C. unicolor harbored the locus of enterocyte effacement (LEE) pathogenic island, containing eae, tir, espA, and espB genes that are associated with the Enteropathogenic E. coli (EPEC) pathotype (34). Additionally, we detected ireA, iroN, iss, iutA, astA, and tsh genes in the genome of ECACA6MA isolated from A. chamek, genes previously associated with Extraintestinal Pathogenic E. coli (ExPEC) pathotype in NHP (35).
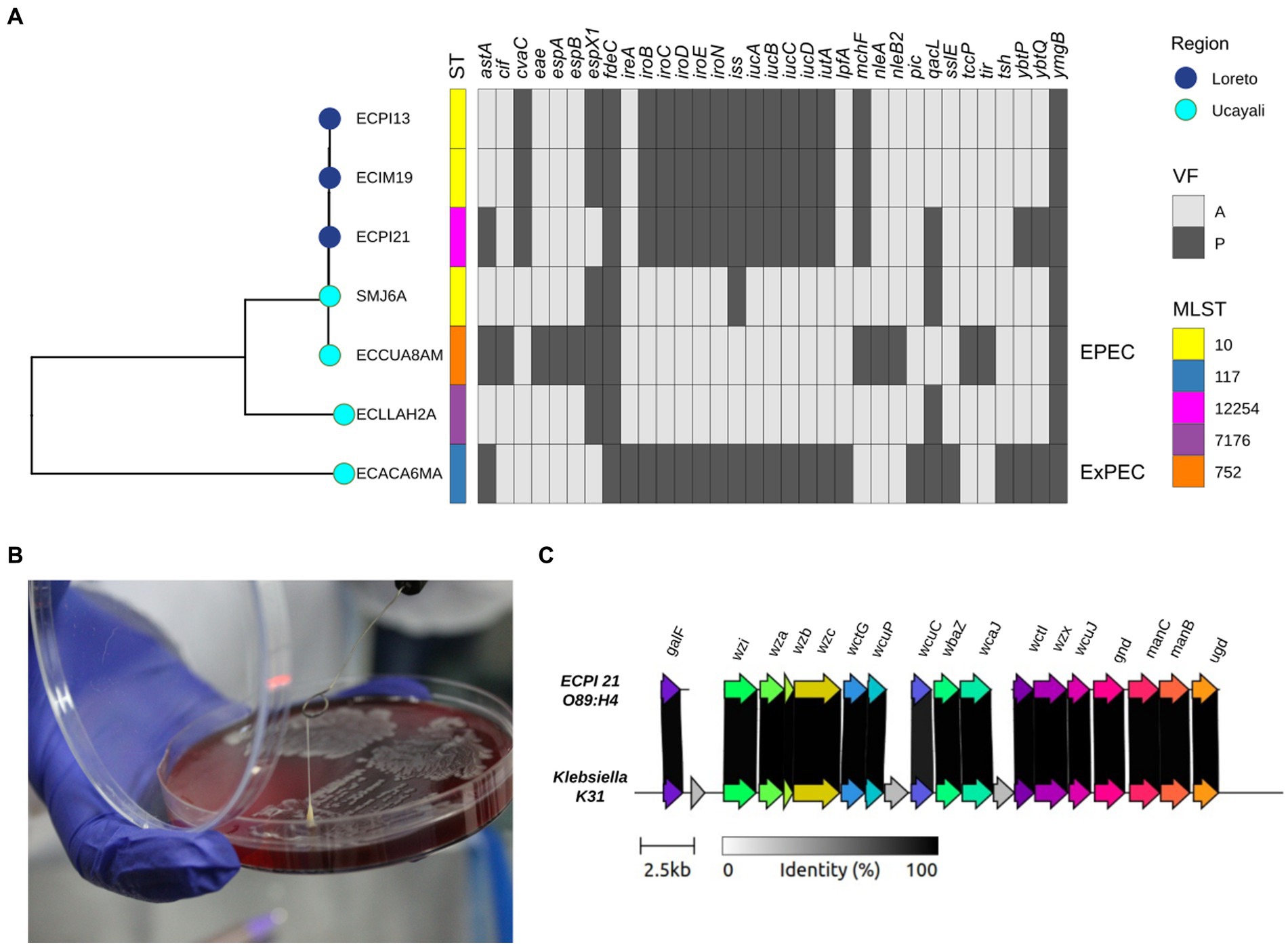
Figure 4. (A) Phylogenetic tree of seven ESBL-producing Escherichia coli strains from NHP coupled to a binary heatmap of presence (dark gray)/absence (light gray) of virulence genes (n = 33). (B) HMV phenotype of the ECPI21 strain isolated from Saguinus mystax represented by a positive “string test” (> 6 cm). (C) Capsule locus structure of HMV phenotype ECPI21 strain (O89:H4). The gray shading indicates the level of identity between ECPI21 and Klebsiella K31 reference capsule locus, which ranges from 98.10 to 100% nucleotide identity.
The ECPI21 strain from S. mystax exhibited a hypermucoviscous (HMV) phenotype, as evidenced by the “string” effect of approximately 6 cm when adherent to a microbiological loop (Figure 4B). This characteristic resembles the HMV phenotype observed in certain virulent Klebsiella pneumoniae strains, providing an advantage for invasive infections (16, 36). While the identification of HMV strains in E. coli is uncommon, it has been described in livestock and clinical strains (37, 38). Analysis of the ECPI21 genome identified a highly conserved Klebsiella-capsule type K31 (98.86% identity; Figure 4C).
4 Discussion
We report the detection and genomic analysis of seven ESBL-producing E. coli strains in semi-captive and captive NHP from two regions of the Peruvian Amazon. ESBL-producing Enterobacteriaceae are critical priority resistant pathogens and were not previously reported in NHP from South America. A recent study found MDR-Enterobacteriaceae in samples taken from free-living black capuchin monkeys (Sapajus nigritus) in Brazil, identifying resistant strains to beta-lactam antibiotics, but no ESBL-production was detected (39). Our ESBL-producing E. coli strains were found to have broad resistome to relevant antibiotics (Table 1). These findings are consistent with previous reports of MDR and ESBL-producing Enterobacteriaceae isolated from fish, soils and aquatic environments in the Amazon ecosystems (40–42).
The most prevalent lineage in our dataset was the ST10 clone, a well-known high-risk pandemic lineage associated with infections in humans and has been found in livestock and meat in Peru (43, 44) Other STs identified in this study, such as ST117, have been found in emerging ExPEC strains of foodborne E. coli, posing a risk to human health (45, 46). ST752 has been associated with poultry in Europe and the United States and is considered predominant in chicken populations (47, 48). ST7176 has been detected in porcine E. coli strains carrying the blaCTX-M-55 variant (49).
The CTX-M family is the most widespread and clinically relevant ESBL enzyme (7). We identified three blaCTX-M variants in our ESBL strains, with blaCTX-M-55 gene being the most common. Interestingly, recent surveillance studies have reported that this variant is also predominant in E. coli strains from pigs, cows, and chickens in Lima-Peru (43, 44, 50), suggesting that anthropogenic activities may serve as potential drivers of CTX-M-producing E. coli strains into the Amazonian wildlife. Conversely, blaCTX-M-15 and blaCTX-M-65 variants were found to a lesser extent in CTX-M-producing E. coli strains from livestock and bats in Peru (43, 50).
Strikingly, we identified two CTX-M-15-producing E. coli ST10 differed from each other by only one SNP across the entire recombination-free core genome. This genetic similarity is noteworthy, as both strains were sampled from two different monkey species, S. mystax and S. labiatus, located 26.5 km apart. The phylogenetic analysis also revealed that two CTX-M-15-producing E. coli from Quito, Ecuador (33), were, on average, 18 SNP distant from our strains. Several studies have demonstrated clonal expansion as a key mechanism for understanding the spread of MDR E. coli in diverse hosts and environments. The evidence suggests that specific MDR and CTX-M-producing E. coli lineages can spread among subjects geographically separated over an extended period with minimal variation in the core genome (51–53).
The remarkable genome similarity of CTX-M-15-producing E. coli ST10 supports the idea of clonal expansion of this lineage among semi-captive monkey populations in the Peruvian Amazon rainforest and other hosts in South America. The source of this clonal spread in the primate population remains unknown, with the most plausible explanation being transmission from humans to NHP populations. Due to the expansion of human settlements in natural NHP habitats in Loreto (Muyuy and Padre islands), the interaction between both hosts has become frequent, increasing the probability of pathogen transmission. Alternatively, there is growing evidence that antimicrobial resistance has impacted Amazonian soils and aquatic ecosystems (40, 42, 54); these environments could serve as reservoirs for AMR that wildlife such as NHP may acquire.
Two ESBL-producing E. coli strains isolated in captive NHP from the Ucayali carried virulence factors typically associated with the EPEC and ExPEC pathotypes. The EPEC pathotype is characterized by its ability to cause attaching-effacing (A/E) lesions, leading to diarrhea and dysentery in humans and livestock (55). We found genes associated with the locus of enterocyte effacement (LEE) pathogenic island in the ECCUA8AM strain isolated from C. unicolor. Previously, the EPEC pathotype was identified in captive young Aotus sp. specimens in Loreto, exhibiting diarrhea in some cases and apparently healthy in others, supporting the hypothesis of NHP as an important reservoir of pathogenic E. coli (56).
On the other hand, ExPEC is known to cause extraintestinal diseases in humans and has been isolated from NHP, although it remains unclear whether it causes disease in NHP (35). The ECACA6MA strain isolated from A. chamek, an endangered species according to the IUCN (57), contains genes associated with the ExPEC pathotype. Despite being pathogenic forms of E. coli, these strains were obtained from apparently healthy animals, suggesting that these NHP could act as reservoirs for those E. coli pathotypes and negatively impact the health of naïve NHP and Amazonian human populations.
The HMV phenotype observed in the ECPI21 strain from S. mystax may be associated with the conserved Klebsiella-K31 capsular sequence in its genome, since K31 capsular type has been linked to HMV K. pneumoniae in various hosts (16, 58, 59). Functional assays are necessary to confirm the association between K31 capsule type and the HMV phenotype observed in ECPI21. The coexistence of ESBL and HMV phenotypes should be a significant concern for public health due to their potentially enhanced virulence (16, 36–38).
In conclusion, we report the isolation of MDR and ESBL-producing E. coli from semi-captive and captive NHP in two regions of the Peruvian Amazon. Genomic analysis revealed three different blaCTX-M gene variants (blaCTX-M-15, blaCTX-M-55, and blaCTX-M-65) and a wide resistome conferring resistance to relevant antibiotics. Furthermore, two strains were characterized as EPEC and ExPEC pathotypes according to their virulence factors, and one more presented HMV phenotype. Most ESBL-producing E. coli strains were assigned to the high-risk pandemic ST10 sequence type, and two of these were closely relatedness to high-risk pandemic lineages previously reported in humans and domestic animals.
This diversity suggests potential environmental pollution resulting from human activities associated with the use of antimicrobial compounds. The presence of pathogenic strains carrying a broad resistome in NHP facilitates the persistence and rapid spread of critical priority ESBL-producing E. coli, which may have a negative impact on the conservation of the Amazonian wildlife and their natural environments. This underscores the importance of adopting the One Health approach in the AMR surveillance, with the aim of minimizing the potential risk of transmission of antibiotic-resistant bacteria and anticipating the emergence and spread of zoonotic and anthroponotic diseases at the human-NHP interface.
Data availability statement
The original contributions presented in the study are publicly available. This data can be found at: https://www.ncbi.nlm.nih.gov/; PRJNA992559.
Ethics statement
The animal study was approved by Comité de Ética y Bienestar Animal—CEBA—UNMSM (Authorization No. 2022-10) and Servicio Nacional Forestal y de Fauna Silvestre (SERFOR) with Directorate Resolution No. RD-000031-2022-MIDAGRI-SERFOR-DGGSPFFS-DGSPFS. The study was conducted in accordance with the local legislation and institutional requirements.
Author contributions
JB-G: Conceptualization, Formal Analysis, Methodology, Writing – original draft, Writing – review & editing. TS-E: Formal analysis, Methodology, Writing – review & editing. CR: Formal analysis, Methodology, Writing – review & editing. DC: Formal analysis, Methodology, Writing – review & editing. YI: Formal analysis, Methodology, Writing – review & editing. LL: Conceptualization, Formal analysis, Funding acquisition, Writing – original draft, Writing – review & editing. RR: Supervision, Writing – review & editing. LM: Conceptualization, Formal analysis, Funding acquisition, Supervision, Writing – original draft, Writing – review & editing.
Funding
The author(s) declare financial support was received for the research, authorship, and/or publication of this article. This study was funded by PROCIENCIA-CONCYTEC, through its executing unit ProCiencia-Proyectos de Investigación Básica 2021-01, Contract number No. 076-2021-FONDECYT.
Acknowledgments
The authors are very grateful to Carlos Ique Guerrero (1953-2021) for his fundamental contribution to the preparation of the research project of this study and to the staff of the Instituto Veterinario de Investigaciones Tropicales y de Altura de Iquitos (IVITA-Iquitos) for their disinterested support in field activities.
Conflict of interest
The authors declare that the research was conducted in the absence of any commercial or financial relationships that could be construed as a potential conflict of interest.
The reviewer FS shares an affiliation to University of Sao Paolo with the author DC.
Publisher’s note
All claims expressed in this article are solely those of the authors and do not necessarily represent those of their affiliated organizations, or those of the publisher, the editors and the reviewers. Any product that may be evaluated in this article, or claim that may be made by its manufacturer, is not guaranteed or endorsed by the publisher.
Supplementary material
The Supplementary material for this article can be found online at: https://www.frontiersin.org/articles/10.3389/fvets.2023.1340428/full#supplementary-material
Footnotes
References
1. Murray, CJL, Ikuta, KS, Sharara, F, Swetschinski, L, Robles Aguilar, G, Gray, A, et al. Global burden of bacterial antimicrobial resistance in 2019: a systematic analysis. Lancet. (2022) 399:629–55. doi: 10.1016/S0140-6736(21)02724-0
2. Sulis, G, Sayood, S, and Gandra, S. Antimicrobial resistance in low- and middle-income countries: current status and future directions. Expert Rev Anti-Infect Ther. (2022) 20:147–60. doi: 10.1080/14787210.2021.1951705
3. Van Boeckel, TP, Pires, J, Silvester, R, Zhao, C, Song, J, Criscuolo, NG, et al. Global trends in antimicrobial resistance in animals in low- and middle-income countries. Science. (2019) 365:eaaw1944. doi: 10.1126/science.aaw1944
4. Hernando-Amado, S, Coque, TM, Baquero, F, and Martínez, JL. Defining and combating antibiotic resistance from one health and global health perspectives. Nat Microbiol. (2019) 4:1432–42. doi: 10.1038/s41564-019-0503-9
5. Ikhimiukor, OO, Odih, EE, Donado-Godoy, P, and Okeke, IN. A bottom-up view of antimicrobial resistance transmission in developing countries. Nat Microbiol. (2022) 7:757–65. doi: 10.1038/s41564-022-01124-w
6. Tacconelli, E, Carrara, E, Savoldi, A, Harbarth, S, Mendelson, M, Monnet, DL, et al. Discovery, research, and development of new antibiotics: the WHO priority list of antibiotic-resistant bacteria and tuberculosis. Lancet Infect Dis. (2018) 18:318–27. doi: 10.1016/S1473-3099(17)30753-3
7. Castanheira, M, Simner, PJ, and Bradford, PA. Extended-spectrum β -lactamases: an update on their characteristics, epidemiology and detection. JAC-Antimicrob Resist. (2021) 3:dlab092. doi: 10.1093/jacamr/dlab092
8. Palmeira, JD, Cunha, MV, Carvalho, J, Ferreira, H, Fonseca, C, and Torres, RT. Emergence and spread of Cephalosporinases in wildlife: a review. Animals. (2021) 11:1765. doi: 10.3390/ani11061765
9. Sano, E, Esposito, F, Fontana, H, Fuga, B, Cardenas-Arias, A, Moura, Q, et al. One health clones of multidrug-resistant Escherichia coli carried by synanthropic animals in Brazil. One Health. (2023) 16:100476. doi: 10.1016/j.onehlt.2022.100476
10. Benavides, JA, Salgado-Caxito, M, Opazo-Capurro, A, González Muñoz, P, Piñeiro, A, Otto Medina, M, et al. ESBL-producing Escherichia coli carrying CTX-M genes circulating among livestock, dogs, and wild mammals in small-scale farms of Central Chile. Antibiotics. (2021) 10:510. doi: 10.3390/antibiotics10050510
11. Guenther, S, Ewers, C, and Wieler, LH. Extended-spectrum beta-lactamases producing E. coli in wildlife, yet another form of environmental pollution? Front Microbiol. (2011) 2:246. doi: 10.3389/fmicb.2011.00246
12. Weiss, D, Wallace, RM, Rwego, IB, Gillespie, TR, Chapman, CA, Singer, RS, et al. Antibiotic-resistant Escherichia coli and class 1 Integrons in humans, domestic animals, and wild Primates in rural Uganda. Appl Environ Microbiol. (2018) 84:e01632–18. doi: 10.1128/AEM.01632-18
13. Pacheco Torres, VR, Diaz, S, Graham Angeles, LA, Flores-Quispe, M, Calizaya-Mamani, G, Ruelas, D, et al. Lista actualizada de la diversidad de los mamíferos del Perú y una propuesta para su actualización. Rev Peru Biol. (2021) 28:e21019. doi: 10.15381/rpb.v28i4.21019
14. Shanee, S, Fernández-Hidalgo, L, Allgas, N, Vero, V, Bello-Santa Cruz, R, Bowler, M, et al. Threat analysis of forest fragmentation and degradation for peruvian primates. Diversity. (2023) 15:276. doi: 10.3390/d15020276
15. Medina, GC, Morales, CS, and Navarrete, ZM. Resistencia Antibiótica de Enterobacterias Aisladas de Monos (Ateles, Callicebus y Lagothrix) en Semicautiverio en un Centro de Rescate, Perú. Rev Investig Vet Perú. (2017) 28:418–25. doi: 10.15381/rivep.v28i2.13073
16. Fang, C-T, Chuang, Y-P, Shun, C-T, Chang, S-C, and Wang, J-T. A novel virulence gene in Klebsiella pneumoniae strains causing primary liver abscess and septic metastatic complications. J Exp Med. (2004) 199:697–705. doi: 10.1084/jem.20030857
17. Clinical and Laboratory Standards Institute (CLSI) (2021). Performance standards for antimicrobial susceptibility testing 31st edition CLSI supplement M100. Available at: https://clsi.org/standards/products/microbiology/documents/m100/
18. Drieux, L, Brossier, F, Sougakoff, W, and Jarlier, V. Phenotypic detection of extended-spectrum β-lactamase production in Enterobacteriaceae: review and bench guide. Clin Microbiol Infect. (2008) 14:90–103. doi: 10.1111/j.1469-0691.2007.01846.x
19. Magiorakos, A-P, Srinivasan, A, Carey, RB, Carmeli, Y, Falagas, ME, Giske, CG, et al. Multidrug-resistant, extensively drug-resistant and pandrug-resistant bacteria: an international expert proposal for interim standard definitions for acquired resistance. Clin Microbiol Infect. (2012) 18:268–81. doi: 10.1111/j.1469-0691.2011.03570.x
20. Bolger, AM, Lohse, M, and Usadel, B. Trimmomatic: a flexible trimmer for Illumina sequence data. Bioinformatics. (2014) 30:2114–20. doi: 10.1093/bioinformatics/btu170
21. Andrews, S (2010). FastQC A Quality control tool for high throughput sequence data. Available at: https://www.bioinformatics.babraham.ac.uk/projects/fastqc/
22. Zhou, Z, Alikhan, N-F, Mohamed, K, Fan, Y, and The Agama Study Group Achtman, M. The EnteroBase user’s guide, with case studies on Salmonella transmissions, Yersinia pestis phylogeny, and Escherichia core genomic diversity. Genome Res. (2020) 30:138–52. doi: 10.1101/gr.251678.119
23. Feldgarden, M, Brover, V, Gonzalez-Escalona, N, Frye, JG, Haendiges, J, Haft, DH, et al. AMRFinderPlus and the reference gene catalog facilitate examination of the genomic links among antimicrobial resistance, stress response, and virulence. Sci Rep. (2021) 11:12728. doi: 10.1038/s41598-021-91456-0
24. Sherry, NL, Horan, KA, Ballard, SA, Gonçalves Da Silva, A, Gorrie, CL, Schultz, MB, et al. An ISO-certified genomics workflow for identification and surveillance of antimicrobial resistance. Nat Commun. (2023) 14:60. doi: 10.1038/s41467-022-35713-4
25. Carattoli, A, and Hasman, H (2020). “PlasmidFinder and in silico pMLST: identification and typing of plasmid replicons in whole-genome sequencing (WGS)” in Horizontal Gene Transfer. (ed.) F CruzDe La (New York, NY: Springer US), 285–294
26. Liu, B, Zheng, D, Jin, Q, Chen, L, and Yang, J. VFDB 2019: a comparative pathogenomic platform with an interactive web interface. Nucleic Acids Res. (2019) 47:D687–92. doi: 10.1093/nar/gky1080
27. Lam, MMC, Wick, RR, Judd, LM, Holt, KE, and Wyres, KL. Kaptive 2.0: updated capsule and lipopolysaccharide locus typing for the Klebsiella pneumoniae species complex. Microb Genomics. (2022) 8. doi: 10.1099/mgen.0.000800
28. Gilchrist, CLM, and Chooi, Y-H. Clinker & clustermap. Js: automatic generation of gene cluster comparison figures. Bioinformatics. (2021) 37:2473–5. doi: 10.1093/bioinformatics/btab007
29. Croucher, NJ, Page, AJ, Connor, TR, Delaney, AJ, Keane, JA, Bentley, SD, et al. Rapid phylogenetic analysis of large samples of recombinant bacterial whole genome sequences using Gubbins. Nucleic Acids Res. (2015) 43:e15. doi: 10.1093/nar/gku1196
30. Minh, BQ, Schmidt, HA, Chernomor, O, Schrempf, D, Woodhams, MD, Von Haeseler, A, et al. IQ-TREE 2: new models and efficient methods for phylogenetic inference in the genomic era. Mol Biol Evol. (2020) 37:1530–4. doi: 10.1093/molbev/msaa015
31. Yu, G, Smith, DK, Zhu, H, Guan, Y, and Lam, TT. Ggtree: an r package for visualization and annotation of phylogenetic trees with their covariates and other associated data. Methods Ecol Evol. (2017) 8:28–36. doi: 10.1111/2041-210X.12628
32. R Core Team (2022). R: A language and environment for statistical computing. R Foundation for Statistical Computing, Vienna, Austria. Available at: https://www.R-project.org/
33. Amato, HK, Loayza, F, Salinas, L, Paredes, D, Garcia, D, Sarzosa, S, et al. Risk factors for extended-spectrum beta-lactamase (ESBL) producing E. coli carriage among children in a food animal producing region of Quito, Ecuador. Epidemiology. (2022). doi: 10.1101/2022.11.14.22282279
34. Deng, W, Puente, JL, Gruenheid, S, Li, Y, Vallance, BA, Vázquez, A, et al. Dissecting virulence: systematic and functional analyses of a pathogenicity island. Proc Natl Acad Sci. (2004) 101:3597–602. doi: 10.1073/pnas.0400326101
35. Clayton, JB, Danzeisen, JL, Trent, AM, Murphy, T, and Johnson, TJ. Longitudinal characterization of Escherichia coli in healthy captive non-human Primates. Front Vet Sci. (2014) 1:24. doi: 10.3389/fvets.2014.00024
36. Shon, AS, Bajwa, RPS, and Russo, TA. Hypervirulent (hypermucoviscous) Klebsiella pneumoniae: a new and dangerous breed. Virulence. (2013) 4:107–18. doi: 10.4161/viru.22718
37. Harris, S, Piotrowska, MJ, Goldstone, RJ, Qi, R, Foster, G, Dobrindt, U, et al. Variant O89 O-antigen of E. coli is associated with group 1 capsule loci and multidrug resistance. Front Microbiol. (2018) 9:2026. doi: 10.3389/fmicb.2018.02026
38. Neumann, B, Lippmann, N, Wendt, S, Karlas, T, Lübbert, C, Werner, G, et al. Recurrent bacteremia with a hypermucoviscous Escherichia coli isolated from a patient with perihilar cholangiocarcinoma: insights from a comprehensive genome-based analysis. Ann Clin Microbiol Antimicrob. (2022) 21:28. doi: 10.1186/s12941-022-00521-7
39. Zaniolo, MM, Santos, ICD, Barbosa, LN, Pachaly, EMV, Caetano, ICDS, Lopes, KFC, et al. Antimicrobial resistance and extended-Spectrum Beta-lactamase production in Enterobacteria isolated from free-living Primates. Vect Borne Zoo Dis. (2020) 20:513–6. doi: 10.1089/vbz.2019.2552
40. Freitas, DY, Araújo, S, Folador, ARC, Ramos, RTJ, Azevedo, JSN, Tacão, M, et al. Extended Spectrum Beta-lactamase-producing gram-negative Bacteria recovered from an Amazonian Lake near the City of Belém, Brazil. Front Microbiol. (2019) 10:364. doi: 10.3389/fmicb.2019.00364
41. Cerdeira, L, Monte, DFM, Fuga, B, Sellera, FP, Neves, I, Rodrigues, L, et al. Genomic insights of Klebsiella pneumoniae isolated from a native Amazonian fish reveal wide resistome against heavy metals, disinfectants, and clinically relevant antibiotics. Genomics. (2020) 112:5143–6. doi: 10.1016/j.ygeno.2020.09.015
42. Lemos, LN, Pedrinho, A, Vasconcelos, ATRD, Tsai, SM, and Mendes, LW. Amazon deforestation enriches antibiotic resistance genes. Soil Biol Biochem. (2021) 153:108110. doi: 10.1016/j.soilbio.2020.108110
43. Murray, M, Salvatierra, G, Dávila-Barclay, A, Ayzanoa, B, Castillo-Vilcahuaman, C, Huang, M, et al. Market chickens as a source of antibiotic-resistant Escherichia coli in a Peri-Urban Community in Lima, Peru. Front Microbiol. (2021) 12:635871. doi: 10.3389/fmicb.2021.635871
44. Carhuaricra, D, Duran Gonzales, CG, Rodríguez Cueva, CL, Ignacion León, Y, Silvestre Espejo, T, Marcelo Monge, G, et al. Occurrence and genomic characterization of mcr-1-harboring Escherichia coli isolates from chicken and pig farms in Lima. Peru Antibiotics. (2022) 11:1781. doi: 10.3390/antibiotics11121781
45. Manges, AR, Geum, HM, Guo, A, Edens, TJ, Fibke, CD, and Pitout, JDD. Global Extraintestinal pathogenic Escherichia coli (ExPEC) lineages. Clin Microbiol Rev. (2019) 32:e00135–18. doi: 10.1128/CMR.00135-18
46. Xia, F, Jiang, M, Wen, Z, Wang, Z, Wang, M, Xu, Y, et al. Complete genomic analysis of ST117 lineage extraintestinal pathogenic Escherichia coli (ExPEC) to reveal multiple genetic determinants to drive its global transmission: ST117 E. coli as an emerging multidrug-resistant foodborne ExPEC with zoonotic potential. Transbound Emerg Dis. (2022) 69:3256–73. doi: 10.1111/tbed.14678
47. Zhang, S, Yang, G, Huang, Y, Zhang, J, Cui, L, and Wu, Q. Prevalence and characterization of atypical Enteropathogenic Escherichia coli isolated from retail foods in China. J Food Prot. (2018) 81:1761–7. doi: 10.4315/0362-028X.JFP-18-188
48. Tiwari, SK, Van Der Putten, BCL, Fuchs, TM, Vinh, TN, Bootsma, M, Oldenkamp, R, et al. Genome-wide association reveals host-specific genomic traits in Escherichia coli. BMC Biol. (2023) 21:76. doi: 10.1186/s12915-023-01562-w
49. Nguyen, MN, Hoang, HTT, Xavier, BB, Lammens, C, Le, HT, Hoang, NTB, et al. Prospective one health genetic surveillance in Vietnam identifies distinct blaCTX-M-harbouring Escherichia coli in food-chain and human-derived samples. Clin Microbiol Infect. (2021) 27:1515.e1–8. doi: 10.1016/j.cmi.2021.01.006
50. Benavides, JA, Godreuil, S, Opazo-Capurro, A, Mahamat, OO, Falcon, N, Oravcova, K, et al. Long-term maintenance of multidrug-resistant Escherichia coli carried by vampire bats and shared with livestock in Peru. Sci Total Environ. (2022) 810:152045. doi: 10.1016/j.scitotenv.2021.152045
51. Price, LB, Johnson, JR, Aziz, M, Clabots, C, Johnston, B, Tchesnokova, V, et al. The epidemic of extended-Spectrum-β-lactamase-producing Escherichia coli ST131 is driven by a single highly pathogenic subclone, H 30-Rx. MBio. (2013) 4:e00377. doi: 10.1128/mBio.00377-13
52. Haenni, M, Beyrouthy, R, Lupo, A, Châtre, P, Madec, J-Y, and Bonnet, R. Epidemic spread of Escherichia coli ST744 isolates carrying mcr-3 and blaCTX-M-55 in cattle in France. J Antimicrob Chemother. (2018) 73:533–6. doi: 10.1093/jac/dkx418
53. Zhi, S, Banting, G, Stothard, P, Ashbolt, NJ, Checkley, S, Meyer, K, et al. Evidence for the evolution, clonal expansion and global dissemination of water treatment-resistant naturalized strains of Escherichia coli in wastewater. Water Res. (2019) 156:208–22. doi: 10.1016/j.watres.2019.03.024
54. Alves, J, Dias, L, Mateus, J, Marques, J, Graças, D, Ramos, R, et al. Resistome in Lake Bolonha, Brazilian Amazon: identification of genes related to resistance to broad-Spectrum antibiotics. Front Microbiol. (2020) 11:67. doi: 10.3389/fmicb.2020.00067
55. Kaper, JB, Nataro, JP, and Mobley, HLT. Pathogenic Escherichia coli. Nat Rev Microbiol. (2004) 2:123–40. doi: 10.1038/nrmicro818
56. Sánchez, PN, Arias, BI, Gálvez, CH, Carranza, V, and Romaina, RA. Escherichia coli Enteropatógena en Crías de Primate Aotus (Aotidae) con Diarrea en Cautiverio. Rev Investig Vet Perú. (2015) 26:657. doi: 10.15381/rivep.v26i4.11205
57. Mitman, S, Rosenbaum, M, Bello, R, Knapp, C, Nutter, F, and Mendoza, P. Challenges to IUCN guideline implementation in the rehabilitation and release of trafficked Primates in Peru. Prim Conserv Newsl J IUCNSSC Prim Spec Group. (2021) 35:87–102.
58. Cubero, M, Grau, I, Tubau, F, Pallarés, R, Dominguez, MA, Liñares, J, et al. Hypervirulent Klebsiella pneumoniae clones causing bacteraemia in adults in a teaching hospital in Barcelona, Spain (2007–2013). Clin Microbiol Infect. (2016) 22:154–60. doi: 10.1016/j.cmi.2015.09.025
Keywords: antimicrobial resistance, extended-spectrum beta-lactamase, Escherichia coli, non-human primates, Peruvian Amazon, molecular epidemiology, multilocus sequence typing, phylogenomic
Citation: Bazalar-Gonzales J, Silvestre-Espejo T, Rodríguez Cueva C, Carhuaricra Huamán D, Ignación León Y, Luna Espinoza L, Rosadio Alcántara R and Maturrano Hernández L (2024) Genomic insights into ESBL-producing Escherichia coli isolated from non-human primates in the Peruvian Amazon. Front. Vet. Sci. 10:1340428. doi: 10.3389/fvets.2023.1340428
Edited by:
Victor Martinez, University of Chile, ChileReviewed by:
Fábio Sellera, University of São Paulo, BrazilJuan David Valencia Bacca, Wake Forest University, United States
Nicolás Galarce, Andres Bello University, Chile
Copyright © 2024 Bazalar-Gonzales, Silvestre-Espejo, Rodríguez Cueva, Carhuaricra Huamán, Ignación León, Luna Espinoza, Rosadio Alcántara and Maturrano Hernández. This is an open-access article distributed under the terms of the Creative Commons Attribution License (CC BY). The use, distribution or reproduction in other forums is permitted, provided the original author(s) and the copyright owner(s) are credited and that the original publication in this journal is cited, in accordance with accepted academic practice. No use, distribution or reproduction is permitted which does not comply with these terms.
*Correspondence: Lenin Maturrano Hernández, YW1hdHVycmFub2hAdW5tc20uZWR1LnBl
†These authors have contributed equally to this work