- Reproductive Unit – Clinic for Small Animals, University of Veterinary Medicine Hannover, Foundation, Hannover, Germany
Chronic asymptomatic idiopathic orchitis (CAO) is an important but neglected cause of acquired infertility due to non-obstructive azoospermia (NOA) in male dogs. The similarity of the pathophysiology in infertile dogs and men supports the dog's suitability as a possible animal model for studying human diseases causing disruption of spermatogenesis and evaluating the role of spermatogonial stem cells (SSCs) as a new therapeutic approach to restore or recover fertility in cases of CAO. To investigate the survival of resilient stem cells, the expression of the protein gene product (PGP9.5), deleted in azoospermia like (DAZL), foxo transcription factor 1 (FOXO1) and tyrosine-kinase receptor (C-Kit) were evaluated in healthy and CAO-affected canine testes. Our data confirmed the presence of all investigated germ cell markers at mRNA and protein levels. In addition, we postulate a specific expression pattern of FOXO1 and C-Kit in undifferentiated and differentiating spermatogonia, respectively, whereas DAZL and PGP9.5 expressions were confirmed in the entire spermatogonial population. Furthermore, this is the first study revealing a significant reduction of PGP9.5, DAZL, and FOXO1 in CAO at protein and/or gene expression level indicating a severe disruption of spermatogenesis. This means that chronic asymptomatic inflammatory changes in CAO testis are accompanied by a significant loss of SSCs. Notwithstanding, our data confirm the survival of putative stem cells with the potential of self-renewal and differentiation and lay the groundwork for further research into stem cell-based therapeutic options to reinitialize spermatogenesis in canine CAO-affected patients.
1. Introduction
The most frequent clinical finding in infertile male dogs is azoospermia, the complete absence of spermatozoa in the ejaculate (1–4). More detailed, non-obstructive azoospermia (NOA) is most common in male dogs (3, 5–8), but also in other species, such as men (9–11). The role of immune cells in infertility due to NOA received increased attention across a number of species in recent years. In men, focal lymphocytic infiltrations have been detected in 25–30% of testicular biopsies from infertile patients (12–14). Further insights into molecular mechanisms of immune cell infiltration leading to the breakdown of spermatogenesis have been gained from studies on experimental autoimmune orchitis (EAO) in rodent models (12, 13). Immune-cell infiltration has also been described in infertile male dogs and—due to the lack of clinical signs—has been associated with spontaneous autoimmune orchitis (AIO), in mainly individual case reports (8, 15–19). These reports describe histopathological changes such as seminiferous tubular degeneration, atrophy, and parenchymal collapse along with immune cell infiltration in the testicular tissues of affected, however, otherwise asymptomatic dogs (8, 15–20). So far, only one publication identified concomitant autoimmune thyroiditis in 17 of 22 affected dogs of a closed beagle colony (15).
Recently, we characterized a larger collective of testicular biopsies from asymptomatic, but infertile canine NOA patients and confirmed severe histopathological alterations, such as Sertoli cell-only (SCO) syndrome, tubular shadows, and fibrosis, concomitantly with lymphoplasmacytic inflammation of both testes (8). Immune cells, namely plasma and T cells, invade not only the interstitium but also the seminiferous tubules (21). These immune cells overcome and disrupt the blood testicular barrier (22) as earlier described in men and EAO mice (12) and lead to focal or generalized inflammatory changes as well as to a corresponding arrest of spermatogenesis (8). These results provide empirical evidence for the postulate that chronic asymptomatic, possibly immune-cell mediated orchitis (CAO) is the cause of acquired infertility due to NOA in the dog. As there is still no certainty regarding the etiology of the disease and its development, CAO is considered to be “spontaneous” or rather idiopathic in the dog. With the onset of infertility, a poor prognosis and irreversible damage to the testicular tissue have to be assumed and previous studies failed to identify treatment options with significant changes in fertility outcome or even recovery or restoration of fertility, not only in dogs (23) but also in humans (24). In the case of infertility in men, assisted reproductive technologies, such as intrauterine insemination, in vitro fertilization, or intracytoplasmic sperm injection represent the last resort (25, 26).
Another approach to the prophylaxis and treatment of human male infertility is based on the derivation of spermatogonial stem cells (SSCs) from testicular tissues by ectopic or orthotopic transplantation to (re-) initialize spermatogenesis (27). Despite the controversial discussion about whether “stemness potential” is restricted to a particular cell type or dynamically distributed in all types of spermatogonia (28, 29), SSCs derive from primordial germ cells during embryonic development and support tissue maintenance through balancing self-renewal and differentiation as the basic requirement for lifelong spermatogenesis (30–35). Thus, an increasing number of studies examine the use of stem cells for regenerative and reproductive science in domestic animals, too (36). In addition, since the first successful SSC transplantation (SSCT) in a dog (37), there has been a growing number of publications focusing on the efficiency and improvement of SSCT (38–42). Although studies over the past two decades provided important information on specific male germ cell biomarkers in rodents, data regarding dogs are limited (43–50).
Important biomarkers studied in relation to germ cell renewal and differentiation for maintenance of spermatogenesis are PGP9.5 (protein gene product 9.5, also known as UCHL-1), DAZL (DAZ-like, deleted in azoospermia), FOXO1 (foxo transcription factor 1), and the tyrosine-kinase receptor C-Kit (also known as CD117) and its ligand (stem cell factor, SCF). Ubiquitin-dependent proteolysis has been implicated in the control of mammalian gametogenesis (51, 52), and the deubiquitinating enzyme PGP9.5 plays an important role in apoptosis, meiosis, and mitotic proliferation in germ cells (53). Results from earlier studies demonstrated an exclusive expression of PGP9.5 in spermatogonia across species, including mice (51), boars (53, 54), bulls (55), and dogs (43, 47). Different from this, DAZL expression is described in various germ cell populations in several vertebrates and invertebrates (56–58), including dogs (45, 49), pigs (53, 59), and mice (60), and is crucial for the initiation of translation during gametogenesis. FOXO1 and C-Kit expression, however, is limited to specific germ cells within the spermatogonial population. FOXO1 expression is restricted to undifferentiated spermatogonia with stem cell potential in the adult testis of various species, including the dog (50). It controls maintenance and differentiation through a specific network of transcriptional targets, including the receptor tyrosine kinase Ret (61). C-Kit expression is linked to the differentiation of type A spermatogonia (62) in several species, including dogs (45, 63–65).
However, despite the growing number of publications focusing on specific male germ cell biomarkers for the identification of SSCs in several species, there have been no attempts to evaluate a stem cell-based therapeutic approach for the treatment of CAO in male dogs up to now. Furthermore, data regarding the survival of viable stem cell populations in the respective canine testicular tissues are currently completely missing. Consequently, the purpose of this study was to investigate the expression of the canine biomarkers PGP9.5, DAZL, FOXO1, and C-Kit in CAO-affected testicular tissue. We hypothesized that SSCs survive in the canine CAO testis despite the histopathological alterations and lymphoplasmacytic infiltration associated with the ongoing disease. However, since the pathological conditions disturb the complex paracrine, molecular, and cellular interaction between SSCs and the “SSC niche”, we assumed a reduced number of SSCs in the case of CAO compared with normal, healthy testis obtained from normospermic canine patients. Furthermore, the aim was not only to detect but also to characterize the surviving spermatogonial population and to compare our findings to previous studies on canine germ cell development.
2. Material and methods
Permission for all samples was received by the respective authorities. The collection of CAO samples for diagnostic purposes was approved by the Dyreforsøgstilsynet Fødevarestyrelsen and by the animal ethics committee of the University of Veterinary Medicine Hannover, Foundation. Testis samples from control animals were taken from dogs that were presented for routine castration for other than health issues. All owners agreed on further use of samples for research purposes.
2.1. Animals and grouping
Twenty-five sexually mature, clinically healthy male dogs were included in this study. Fifteen dogs were assigned to the CAO group (CAO) after confirmation of permanent azoospermia. Dogs had a mean age of 5.5 ± 1.9 years (2.6–9.6 years) and represented the following breeds: Collie: n = 3, Cairn Terrier, Coton de Tulear, Beagle, and German Shepherd Dog: n = 3, and Iceland sheepdog, Jack Russel Terrier, Labrador Retriever, Miniature Poodle, Welsh Corgi Pembroke, and Cane Corso: n = 1 each. Part of the dogs were also included in previous studies (8, 66). The remaining ten dogs with a mean age of 4.2 ± 2.8 years (1 – 8.9 years) served as a healthy control group (CG) with normospermic ejaculates according to the reference values (67, 68). Animals of the control group belonged to six different breeds (n = 1 each: Boxer, Havanese, Malteser, Boston Terrier, and Chihuahua; and n = 3 Beagle), and two male dogs were mongrels.
2.2. Study design, sample collection, and processing
Detailed clinical and histological procedures to identify azoospermia, to verify non-obstructive azoospermia (NOA), to rule out bacterial infectious causes, and to verify immune cell infiltration (CAO) had been described earlier (8, 21). Briefly, clinical and andrological examinations including semen collection and analysis, ultrasound of the genital tract, and determination of the alkaline phosphatase (AlP) in seminal plasma (>5000 U/l) were performed to confirm NOA. Further bacteriological examination of the ejaculate and serological testing for Brucella canis was used to rule out infection, and endocrine analysis (testosterone/estradiol-17ß/thyroxin/free thyroxin/cTSH/thyroid antibodies) was used to rule out pretesticular origin of NOA. Scissor biopsies from both testes were obtained for histological assessment, and CAO was confirmed in all included dogs (8). Samples of the CAO group were further differentiated based on the histological appearance, namely the arrest of spermatogenesis: early arrest (n = 5) with Sertoli-cell only (SCO) or spermatogenesis arrested on spermatogonia; and late arrest (n = 10) with spermatogenesis arrested on spermatocytes or later stages of spermatogenesis. Testicular tissues in CG (n = 10) were collected during surgical castration performed upon the owner‘s request due to other reasons than testicular disease.
2.3. Preparation of testicular tissues
The preparation of testicular tissues for histology, mRNA, and protein extraction/analysis was as previously described (69). Briefly, the samples were partly placed in RNAlater® (Qiagen GmbH, Hilden, Germany) and frozen at−80°C, while the other part was fixed in Bouin's solution for 24 h, washed several times in 70% ethanol and subsequently embedded in paraffin.
2.4. Quantitative real-time PCR
Isolation of total RNA from the RNAlater®-immersed testicular tissues of each dog was performed using TRI reagent® (Sigma Aldrich, St. Louis, Missouri, USA) following the manufacturer's instructions. RNA concentration and quality were measured using a NanoPhotometer® NP80 (IMPLEN, Munich, Germany). All RNA samples were treated with Protector RNase Inhibitor (Sigma Aldrich) and stored at-80°C until used. Full-length first strand cDNA-synthesis was performed using 200 ng/μl RNA and the RevertAidFirst Strand cDNA Synthesis kit (#K1622, Thermo Fisher, Waltham, MA, USA), including DNase treatment, according to the manufacturer's protocol. In order to determine the expression of PGP9.5, DAZL, FOXO1, and C-Kit, primer sets for RT-qPCR were developed using known sequences available from GenBank (Table 1). Glyceraldehyde-3-phosphate dehydrogenase (GAPDH), hypoxanthine guanine phosphoribosyltransferase (HPRT), and β-actin were evaluated for use as housekeeping genes for endogenous control. Finally, GAPDH and HPRT were chosen as reference genes as they showed the slightest variation in mRNA expression.
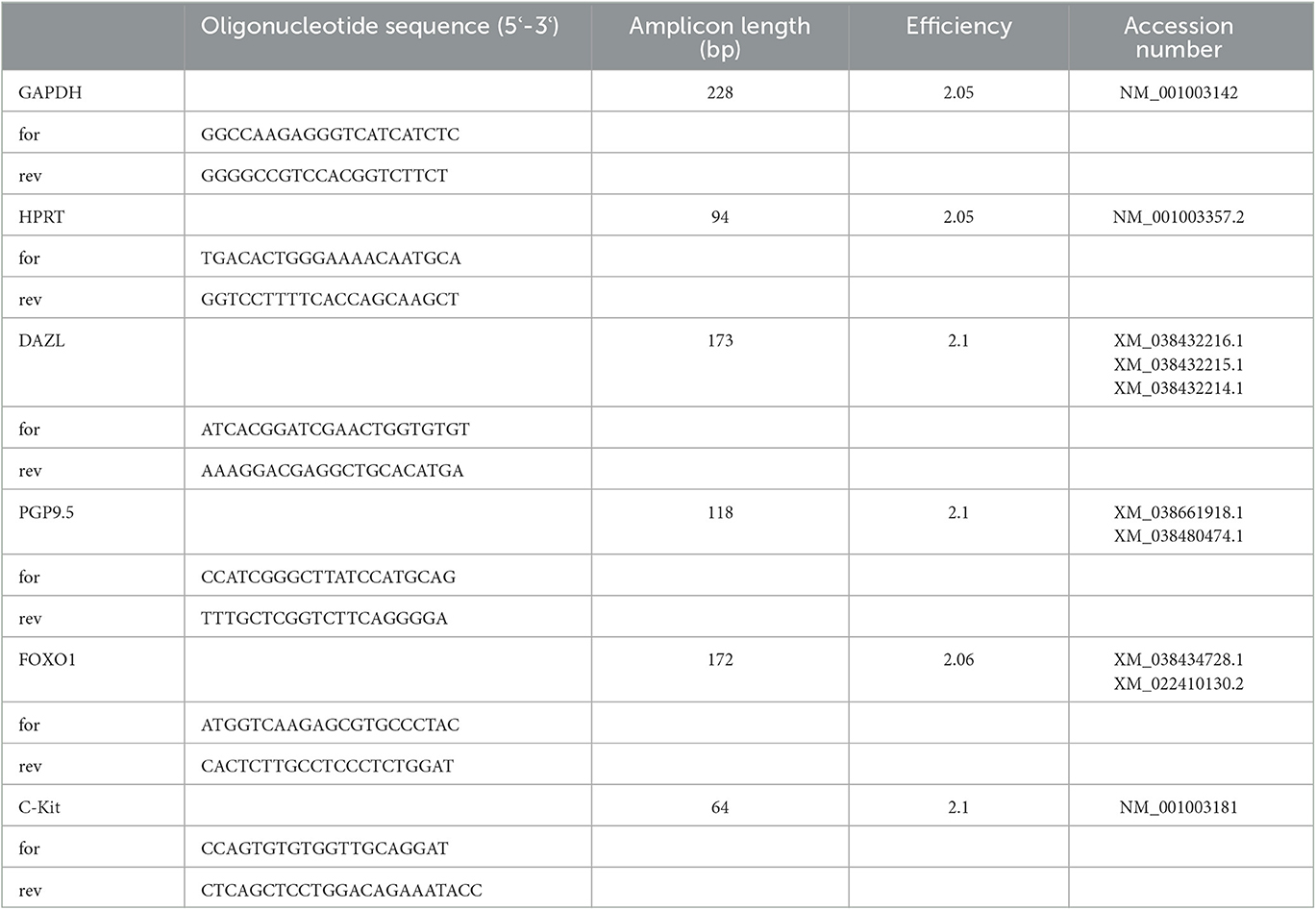
Table 1. Sequences of primers for RT-PCR and RT-qPCR, amplicon length, efficiency, and accession number (for, forward; rev, reverse).
Relative mRNA expression (RT-qPCR) was investigated as described earlier (66, 70): cDNA was added to 8μl of FastStart Essential DNA Green Master (Roche Diagnostics GmbH, Mannheim, Germany), 1μl of the forward and reverse primer (10pmol) (Table 1), and 2μl of sterile Aqua bidest. RT-qPCR was performed with the following cycling conditions for all genes except for FOXO1 and HPRT: 95°C for 10 min, followed by 45 cycles of 95°C for 10 s, 60°C for 10 s, 72°C for 10 s, and melting curve with 80°C. For FOXO1 and HPRT, cycling conditions were slightly modified with the annealing temperature being 57°C. All samples were run in triplicates using a LightCycler®96 real-time PCR system (Software version 1.1.0.1320, Roche Diagnostics GmbH, Mannheim, Germany). Calculation of PCR efficiencies of target and reference genes was performed by using a relative standard curve derived from a triplet RT-qPCR run of a 2-fold dilution series (1:2- 1:128) of pooled cDNA samples, whereas the efficiency (E) was E= 10(-1/m) with “m” being the slope of the linear regression line. For the evaluation of the RT-qPCR results, the efficiency-corrected relative quantification according to Pfaffl (2001) (71) was modified and extended taking both reference genes into account as described previously (72). The specificity of the primers for PGP9.5, DAZL, FOXO1, and C-Kit used in RT-qPCR was checked by using BLAST (https://blast.ncbi.nlm.nih.gov/Blast.cgi), and the results were confirmed by sequencing of PCR products (Microsynth AG, Balgach, Switzerland).
2.5. Immunohistochemistry and evaluation of PGP9.5, DAZL, FOXO1, and C-Kit staining
The paraffin-embedded tissue was cut in serial sections of 2 μm thickness and placed on microscopic slides. Preparation and pretreatment of the tissue were performed according to the protocol in earlier studies (73, 74). After deparaffinization and rehydration in xylene and ethanol, the antigen was retrieved by boiling it in 0.01 M sodium citrate buffer at pH 6. After the sections were rinsed in distilled water, the tissue was treated with 3% H2O2 to block endogenous peroxidase activity. For PGP9.5, non-specific protein binding was blocked with 3% bovine serum albumin (BSA, VWR Life Science, Solon, OH, USA) and 10% horse serum (S-2000, RRID: AB_2336617, Vector Laboratories), and slides were subsequently incubated with the PGP9.5 monoclonal mouse anti-human antibody (7863-1004, RRID: AB_620256, AbD Serotec, Raleigh, NC, USA, dilution 1 x 10−3 μg/μl) overnight at 4°C. ICC buffer only was used as negative control and an irrelevant mouse IgG (I-2000, RRID: AB_2336354, Mouse IgG, Control Antibody, Vector Laboratories) as isotype control. For FOXO1, 10% goat serum in ICC buffer was used for blocking followed by incubation with the FOXO1 monoclonal rabbit anti-human antibody (C29H4, #2880, RRID: AB_2106495, CST, Danvers, MA, USA, dilution 0, 44 x 10−3 μg/μl) overnight at 4°C. ICC buffer only served as negative control and an irrelevant rabbit IgG (I-1000, RRID: AB_2336355, Rabbit IgG, Control Antibody, Vector Laboratories) as isotype control. Following several washing steps, the respectively treated slides were incubated with SuperVision-2 HRP Enhancer (DCS, Innovative Diagnostik Systeme, Hamburg, Germany) and HRP Polymer (DCS, Innovative Diagnostik Systeme) for 20 min each. Immunopositive signals were visualized with DAB (DCS Chromoline, Innovative Diagnostik Systeme) according to the manufacturer's instructions. Slides were counterstained with hematoxylin after Mayer, dehydrated in increasing alcohol concentrations, and mounted with Roti® Histokitt II (Roth AG, Arlesheim, Switzerland). IHC against DAZL was performed with slight modifications of the protocol mentioned above. For DAZL, non-specific protein binding was blocked with 10% goat serum (S-1000, RRID: AB_2336615, Vector Laboratories, Burlingame, CA, USA) in ICC buffer (1.2 g Na2HPO4, 0.2 g KH2PO4, 0.2 g KCl, 8.0 g NaCl, and 3ml Triton ad 10000 ml), and slides were subsequently incubated with the DAZL polyclonal rabbit anti-mouse antibody (ab34139, RRID:AB_731849, Abcam, Cambridge, UK, dilution 2 x 10−3 μg/μl) overnight at 4°C. On the next day after washing three times with ICC buffer, samples were incubated with a goat anti-rabbit secondary antibody (BA-1000, RRID: AB_2313606, Vector Laboratories). Immunoreactivity was visualized by an immunoperoxidase method according to the manufacturer's instruction (VECTASTAIN PK-6100 Rabbit IgG Elite ABC Kit, RRID: AB_2336819, and Vector Nova-RED Substrate Kit SK-4800, RRID: AB_2336845, Vector Laboratories). ICC buffer only was used as negative control, and an irrelevant rabbit IgG antibody (I-1000, RRID: AB_2336355, Rabbit IgG, Vector Laboratories) in the respective protein concentration was used as isotype control.
For C-Kit, following the abovementioned treatment, the samples were incubated with 10% goat serum (S-1000, Vector Laboratories) in 3% bovine serum albumin to block unspecific antibody binding and then incubated with a polyclonal rabbit anti-human CD117/c-kit antibody (A4502, RRID: AB_2335702, Dako Denmark A/S, Glostrup, Denmark, 0, 208 μg/μl) overnight at 4°C. Following several washing steps, the slides were incubated with SuperVision-2 HRP Enhancer (DCS, Innovative Diagnostik Systeme, Hamburg, Germany) and HRP Polymer (DCS, Innovative Diagnostik Systeme) for 20 min each. Visualization was performed using DAB (DCS Chromoline, Innovative Diagnostik Systeme) according to the manufacturer's instructions. ICC buffer only was used as a negative control and an irrelevant rabbit IgG antibody (I-1000, RRID: AB_2336355, rabbit IgG, Vector Laboratories) in the respective protein concentration was used as isotype control.
All slides for each antibody were performed simultaneously to avoid bias regarding experimental conditions.
For histological evaluation of PGP9.5, DAZL, and FOXO1, immunopositive signals were counted using an Olympus BX41TF Microscope (Olympus®, Shinjuku, Japan) with an Olympus DP72 camera (Olympus Corporation, Tokyo, Japan) and the Olympus cellSense Dimension Software (version 2.1, Olympus Corporation, Tokyo, Japan). In CAO samples, 20 randomly chosen fields of vision of each dog, 10 from the right and the left testis biopsy, respectively, were evaluated at 200-fold magnification. Similarly, 20 randomly selected fields of vision of each dog from CG were counted and served as a comparison.
Due to a diffuse and weak expression pattern in C-Kit staining, adjacent immunopositive cells were difficult to distinguish from each other and other cells, especially in cases of CAO (Figure 12). Therefore, immunopositive staining was evaluated descriptively using an Olympus BX41TF Microscope (Olympus®, Shinjuku, Japan) at 200-fold and 400-fold magnification.
2.6. Protein extraction and Western blot analyses
A Western blot analysis was performed to confirm the specificity and reactivity of primary antibodies in canine tissues as described earlier (73, 75). Frozen canine total testicular tissue (approximately 0.8g) obtained from a healthy adult dog with normal spermatogenesis was dissolved in PBS with a protease inhibitor cocktail (Roche® Diagnostics, Basel, Switzerland), pulverized and homogenized using an Ultraturrax (IKA®, Staufen, Germany). After boiling for 10 min with 0.15 g of dodecyl sulfate sodium salt, the insoluble material was removed by centrifugation at 1200 x g for 10 min at 4°C. The protein concentration of the supernatant was quantified spectrophotometrically using a NanoPhotometer® NP80 (IMPLEN, Munich, Germany). The obtained total protein was aliquoted and stored at −80°C. Tissues of appropriate positive controls were treated appropriately (Table 2). Canine testis protein and corresponding positive control proteins were used following denaturation by heating at 95°C for 10 min in a Laemmli sample buffer in the water bath. Protein separation was achieved by a 4–20% gradient sodium dodecyl sulfate-polyacrylamide gel (Mini-Protean® TGXTM Gels, Bio-Rad Laboratories, Hercules, California, USA) electrophoresis. After blotting the proteins on a nitrocellulose membrane (Trans-Blot® TurboTM Transfer Pack, Bio-Rad Laboratories) using a blotting system (Trans-Blot® Turbo TM Transfer System, Bio-Rad Laboratories), unspecific binding sites were blocked by incubating the membrane for 1 h at room temperature in 5% skimmed milk powder in TBS (Tris-buffered saline). Following several washing steps with TBST (TBS with 0.05% Tween), the membrane was incubated with the primary antibody in 5% skimmed milk powder in TBS for 2 h. After being washed in TBST, the membrane was incubated at room temperature for 1 h with the secondary antibody (biotinylated goat anti-rabbit IgG antibody BA-1000/RRID:AB_2313606 or horse anti-mouse IgG antibody BA-2000/RRID:AB_2313581, Vector Laboratories). Signals were visualized using ClarityTM Western ECL Blotting Substrate (Bio-Rad Laboratories) according to the manufacturer's instructions and a ChemiDoc™ Imaging Systems with Image Lab™ Touch Software (Image Lab 6.0.1, Bio-Rad Laboratories). A mouse IgG nonsense antibody (I-2000, RRID: AB_2336354, Control Antibody, Vector Laboratories) or rabbit IgG nonsense antibody (I-1000, RRID: AB_2336355, Control Antibody, Vector Laboratories) in the same concentration as the primary antibody served as isotype control. Table 2 provides an overview of the use of primary antibodies, secondary antibodies, and positive controls.

Table 2. Overview of reagents and dilutions used in the Western blot; SKU, Stock Keeping Unit; IC, Isotype control; *Abcam, Cambridge, UK; **Bio-Rad Laboratories, Hercules, California, USA; ***Agilent Technologies, Santa Clara, California, USA; ****Cell Signaling Technology, Danvers, Massachusetts, USA.
2.7. Statistical analysis
GraphPad Prism7 software (GraphPad Software, Inc., La Jolla, CA, USA) and Microsoft Excel (Version 16.65, Microsoft, Redmond, WA, USA) were applied for statistical analysis. Values at a level of p≤0.05 were considered to be statistically significant. The aim of our study was to identify significant differences between CAO and CG regarding their PGP9.5, DAZL, FOXO1, and C-Kit mRNA and protein expressions.
As the Shapiro–Wilk test did not confirm normal distribution for the mRNA expression (ratio) of PGP9.5, DAZL, and C-Kit, the Mann–Whitney test was used to test for significant differences between CAO and CG. For FOXO1, normal distribution was verified and therefore an unpaired t-test was applied. CAO samples were furthermore differentiated into early (n = 5) and late arrest of spermatogenesis (n = 9), and the results were compared with CG. Regarding DAZL group comparison (CAO vs. CG), log-transformed data revealed normal distribution. Subsequently, an ANOVA followed by Tukey's multiple comparisons test using log-transformed data was used to test for significant differences between early and late arrest as well as CG. As the Shapiro–Wilk test did not confirm normal distribution for PGP9.5, C-Kit, and FOXO1, a non-parametric ANOVA (Kruskal–Wallis test) was applied for overall group comparison (early CAO vs. late CAO vs. CG) followed by Dunn's multiple comparison test in case of the Kruskal–Wallis test revealing a p-value of ≤0.05. For comparative reasons, all mRNA expressions (ratios) are presented as geometric mean and dispersion factor [xg (DF)].
For histological evaluation of protein expression of PGP9.5, DAZL, and FOXO1, immunopositive signals in 20 randomly chosen fields of vision (F) were counted and compared. Again, CAO samples were further differentiated into early (n = 5) and late arrest of spermatogenesis (n = 7), and the results were compared with CG. In all three groups, immunopositive results of fields of vision (F) were evaluated independently and collected in two numerical data sets (CAO vs. CG; CAO early vs. CAO late vs. CG). In addition, the expression patterns of C-Kit during normal and disturbed spermatogenesis were evaluated and compared descriptively.
In the first step, the Shapiro–Wilk test was applied to test for normal distribution of the respective PGP9.5, DAZL, and FOXO1 protein expression obtained from the left and right testes (CAO). After normal distribution was verified, the paired t-test was applied for PGP9.5, FOXO1, and DAZL (F) data to prove whether the respective data differed depending on the localization (right/left testis CAO). As no significant differences were identified, the results obtained from both the testes of each dog and the parameter were summarized. The obtained results in CAO samples were compared with the results obtained in CG samples. As the applied Shapiro–Wilk test confirmed normal distribution, data are presented as arithmetic mean and standard deviation (SD). An unpaired t-test was used to test for significant differences in PGP9.5, DAZL, and FOXO1 protein expression between CAO and CG.
When differentiating CAO into early and late arrest and comparing results to CG, the Shapiro–Wilk test revealed normal distribution for log-transformed data of PGP9.5 and DAZL protein expression, and an ANOVA followed by Tukey's multiple comparisons test was used for overall group comparison. For FOXO1, the Shapiro–Wilk test did not verify normal distribution, and consequently, non-parametric ANOVA (Kruskal–Wallis test) was applied to test for significant differences between the groups (early CAO vs. late CAO vs. CG) followed by Dunn's multiple comparison test in case of the Kruskal–Wallis test revealing a p-value of ≤0.05. Again, for comparative reasons, all results of group comparison are presented as geometric mean and dispersion factor [ (DF)].
3. Results
3.1. PGP9.5 mRNA and protein expression
Ratios for PGP9.5 mRNA expression differed significantly between CAO and CG with a significantly lower PGP9.5 expression in CAO compared with CG (p = 0.0012; Figure 1A). Furthermore, the statistical comparison between mRNA expressions in early arrest, late arrest, and CG revealed a significant difference (p = 0.0012) with early arrest showing significantly lower PGP9.5 expression compared with CG (p = 0.0028, Figure 1B).
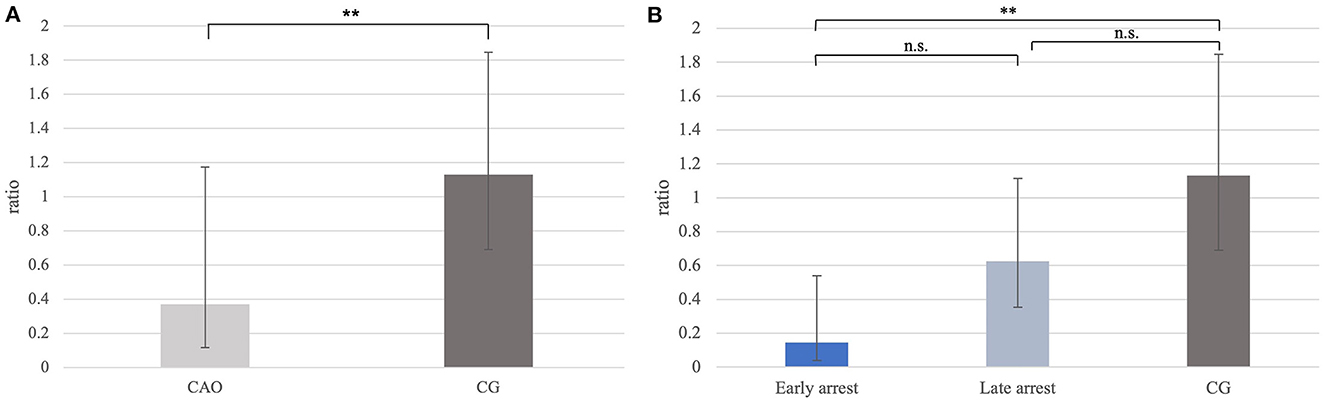
Figure 1. (A) PGP9.5 mRNA expression (ratio) in groups of dogs suffering from immune-mediated orchitis (CAO) and healthy control dogs (CG). The results are presented as geometric mean and dispersion factor [g (DF)]. Datasets with asterisks differ significantly: **p = 0.0012. (B) PGP9.5 mRNA expression (ratio) in groups of early and late arrest (CAO) and control dogs (CG). The results are presented as geometric mean and dispersion factor [g (DF)]. Datasets with asterisks differ significantly: **p = 0.0028.
To confirm the reactivity of the PGP9.5 antibody, a Western blot was performed using testicular tissue and rat brain tissue as positive controls. The molecular weight of the canine band was equally located to the positive control at 25 kD (Figure 2A). No specific immunoreactive band was visible in the negative and isotype controls from the canine testes and positive control.
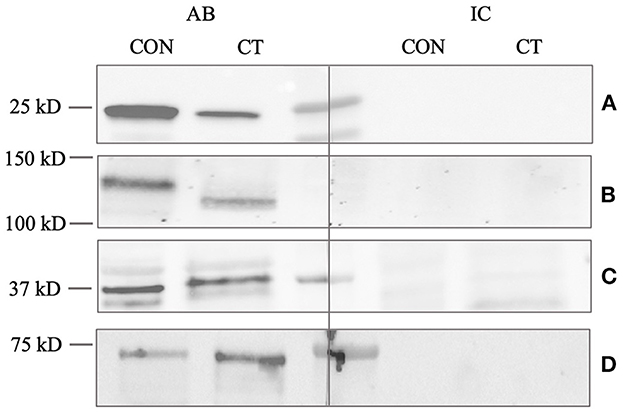
Figure 2. Western blot analysis of (A) PGP9.5, (B) C-Kit, (C) DAZL, and (D) FOXO1. CT (canine testis) indicates adult, healthy canine testis tissue. CON (control) indicates an appropriate positive control, either proposed by the manufacturer or demonstrated by previous studies (45, 76). AB indicates the antibody and IC the isotype control. Protein size in kilodalton (kD) is given on the left.
Immunohistochemistry revealed specific immunopositive staining located in the nuclei and cytoplasm of spermatogonia and early stages of spermatocytes located at the basal membrane of the seminiferous tubules in both groups (Figure 3). However, PGP9.5+ staining was restricted to germ cells attached to the basal membrane within the seminiferous tubules of CG, and some immunopositive cells in CAO were detached (Figure 3E). Additionally, some peritubular cells and Leydig cells in the interstitial compartment were stained with PGP9.5+ in CAO, especially around the tubules with early arrested spermatogenesis (Figure 3F), but not in CG. The total number of PGP9.5+ cells differed significantly between CAO and CG (p = 0.0002), with a significantly lower PGP9.5+ expression in CAO samples (Figure 4A). Differences between early and late arrest as well as CG revealed significance (p = 0.0002). The subsequent Dunn's test identified significant differences between samples with early arrest compared with late arrest (p = 0.0005) and with CG (p < 0.0001), with fewer PGP9.5+ signals in the early arrest group. No significant difference was observed between late arrest and CG (Figure 4B).
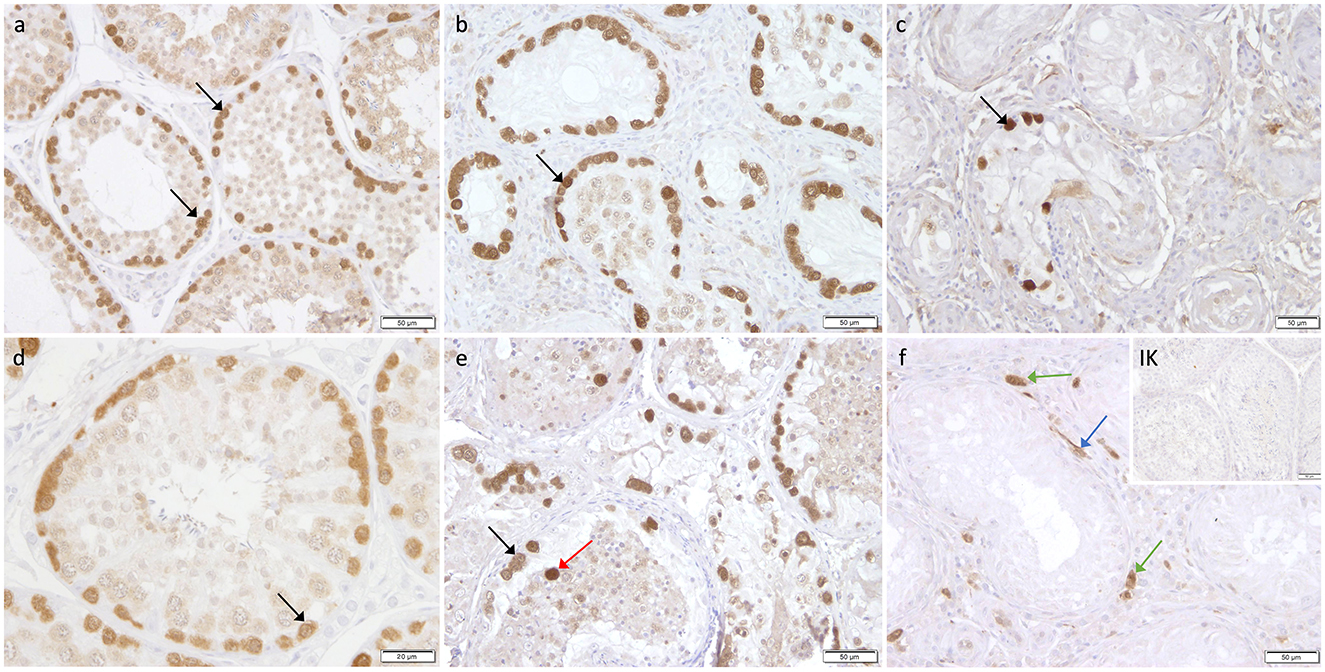
Figure 3. Immunostaining against PGP9.5 (a–f) in CG and CAO dogs (200x magnification, (d) 400x magnification). (a, d) CG, (b, e) late arrest, (c, f) early arrest. Spermatogonia (black arrow); Leydig cell (green arrow); peritubular myoid cell (blue arrow); detached spermatogonia (red arrow).
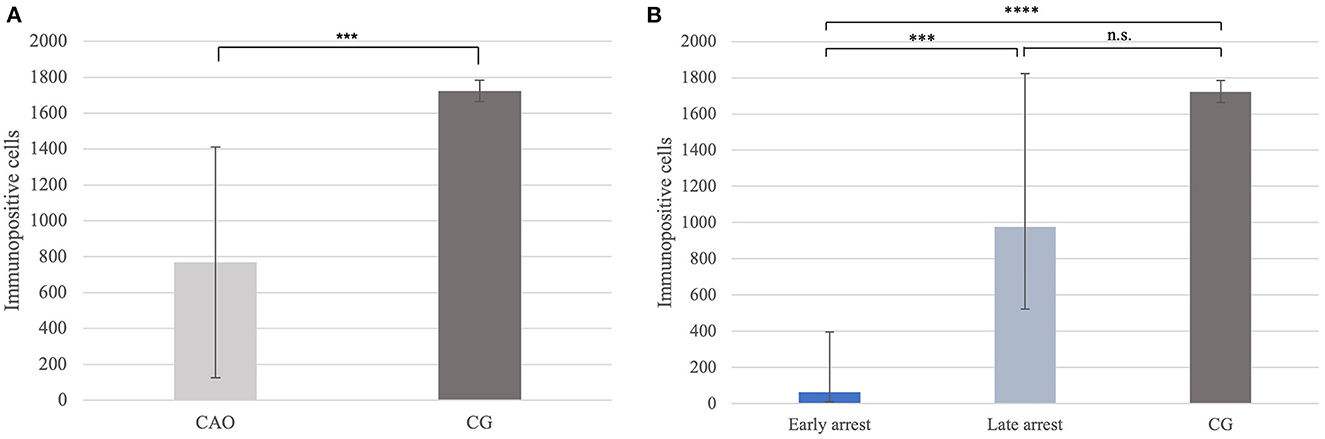
Figure 4. (A) PGP9.5 protein expression evaluated as the number of immunopositive cells in 20 fields of vision in groups of dogs suffering from immune-mediated orchitis (CAO) and healthy control dogs (CG). The results are presented as arithmetic mean and standard deviation (SD). Datasets with asterisks differ significantly: ***p = 0.0002. (B) PGP9.5 protein expression evaluated as the number of immunopositive cells in 20 fields of vision in groups of early and late arrest (CAO) and control dogs (CG). The results are presented as geometric mean and dispersion factor [g (DF)]. Datasets with asterisks differ significantly: ****p = 0.0001; ***p = 0.0005.
3.2. DAZL mRNA and protein expression
Ratios (mRNA expression) for DAZL did not differ significantly between CAO and CG (Figure 5A). However, comparing the groups of early and late arrest to CG, a significant overall difference was found (p = 0.0060) with lower DAZL expression in early arrest samples compared with late arrest (p = 0.0212), but no differences were found between early or late arrest and CG (Figure 5B).
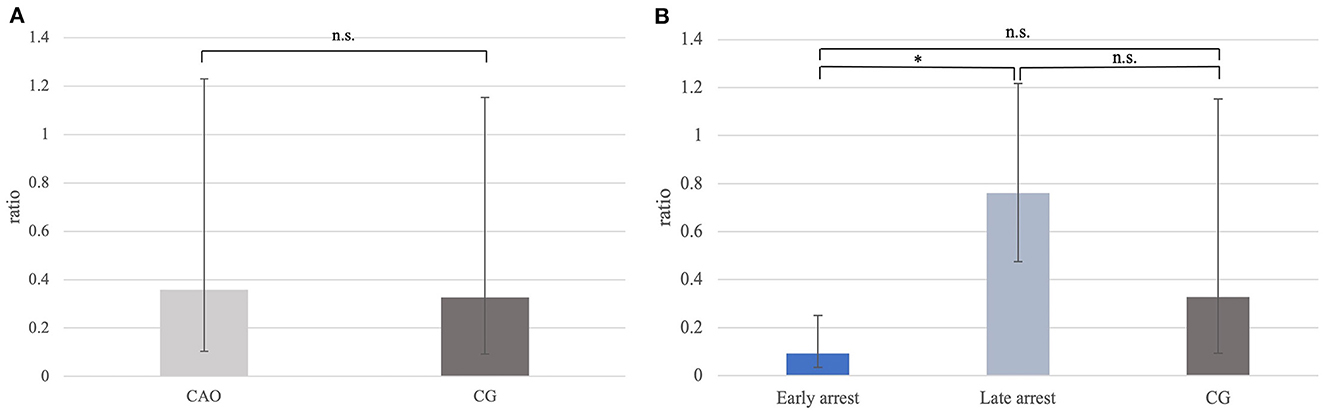
Figure 5. (A) DAZL mRNA expression (ratio) in groups of dogs with immune-mediated orchitis (CAO) and healthy control dogs (CG). The results are presented as geometric mean and dispersion factor [g (DF)]. (B) DAZL mRNA expression (ratio) in dogs with CAO divided into early and late arrest and control dogs (CG). The results are presented as geometric mean and dispersion factor [g (DF)]. Datasets with asterisks differ significantly: *p = 0.0212.
Specificity and reactivity of the DAZL antibody were proven using murine testicular protein as a positive control (Figure 2C). The molecular weight of the specific canine band was equally located to the murine control at 37 kD.
The cytoplasm of spermatogonia in CAO and CG samples, as well as the cytoplasm of primary spermatocytes, was stained immunopositive against DAZL (Figure 6). In the interstitial compartment, DAZL+ staining was identified predominantly in blood vessels and occasionally in the cytoplasm of Leydig cells in both groups. In addition, single DAZL+ signals were detected in the peritubular myoid cells in CAO, with individual differences in staining intensity between the samples (Figure 6F).
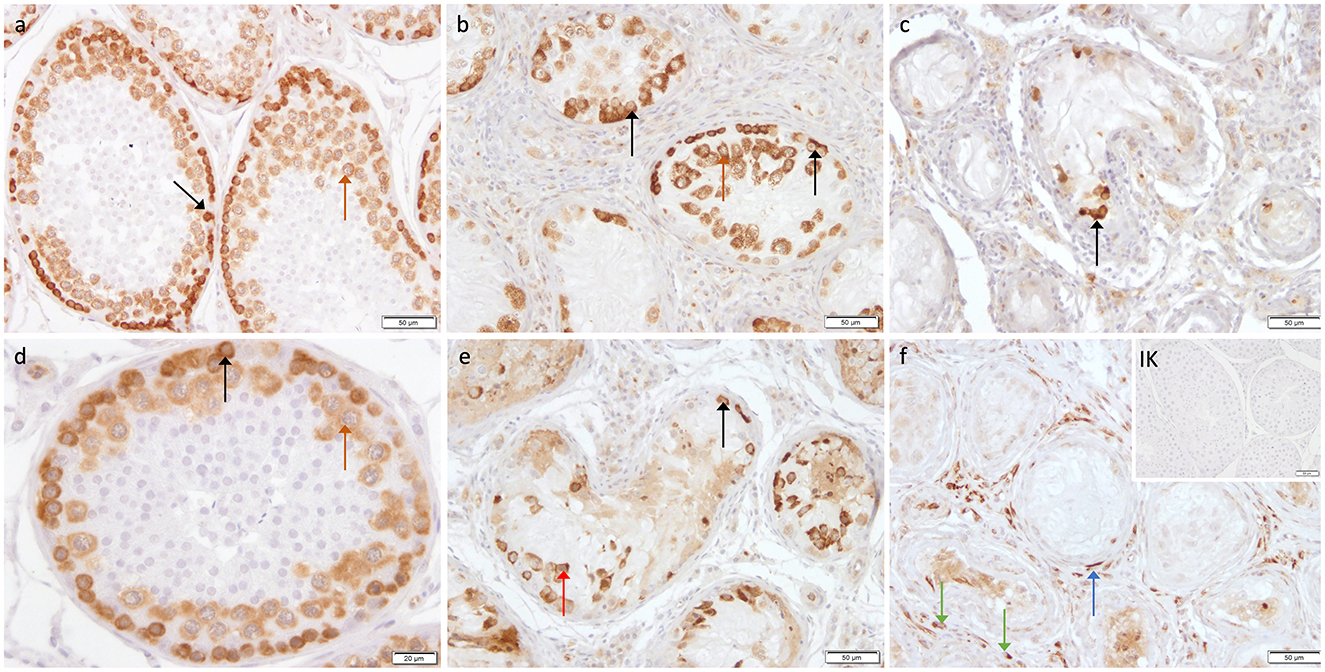
Figure 6. Immunostaining against DAZL (a–f) in CG and CAO dogs (200x magnification, (d) 400x magnification). (a, d) CG, (b, e) late arrest, (c, f) early arrest. early arrest. Spermatogonia (black arrow); spermatocyte (orange arrow); Leydig cell (green arrow); peritubular myoid cell (blue arrow); detached spermatogonia (red arrow).
Significantly fewer DAZL+ signals were counted in CAO compared with CG (p < 0.0001; Figure 7A). Furthermore, differences between early and late arrest and CG were significantly different (p < 0.0001), with the results for early arrest differing significantly different from CG (p < 0.0001) and late arrest (p = 0.0004). No significant difference was observed between late arrest and CG (Figure 7B).
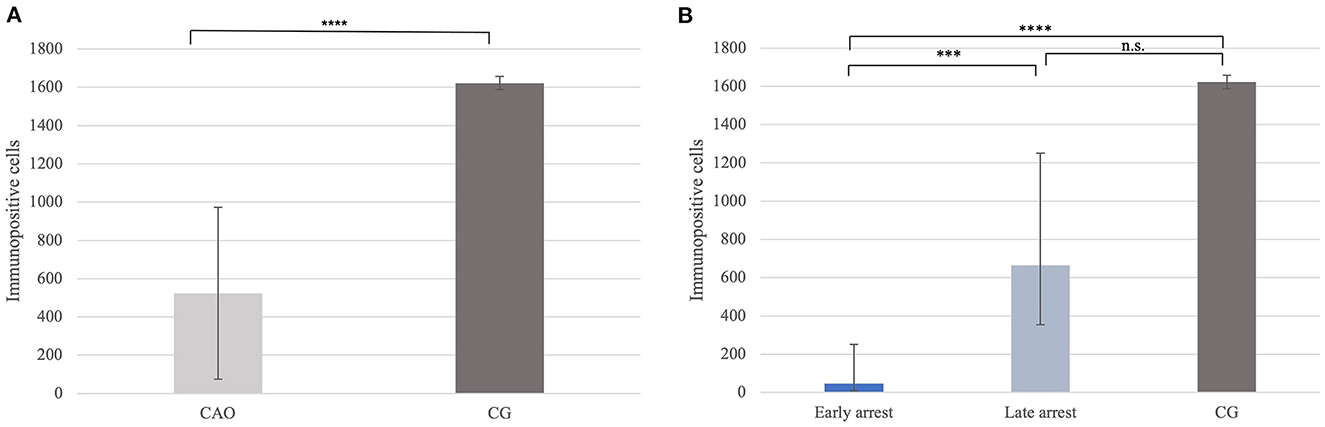
Figure 7. (A) DAZL protein expression evaluated as the number of immunopositive cells in 20 fields of vision in groups of dogs suffering from immune-mediated orchitis (CAO) and healthy control dogs (CG). The results are presented as arithmetic mean and standard deviation (SD). Datasets with asterisks differ significantly: ****p < 0.0001. (B) DAZL protein expression evaluated as the number of immunopositive cells in 20 fields of vision in groups of early and late arrest (CAO) and control dogs (CG). The results are presented as geometric mean and dispersion factor [g (DF)]. Datasets with asterisks differ significantly: ****p < 0.0001; ***p = 0.0004.
3.3. FOXO1 mRNA and protein expression
Ratios for FOXO1 mRNA expression did not differ significantly between CAO and CG (Figure 8A), nor generally between early and late arrest as well as CG (Figure 8B).
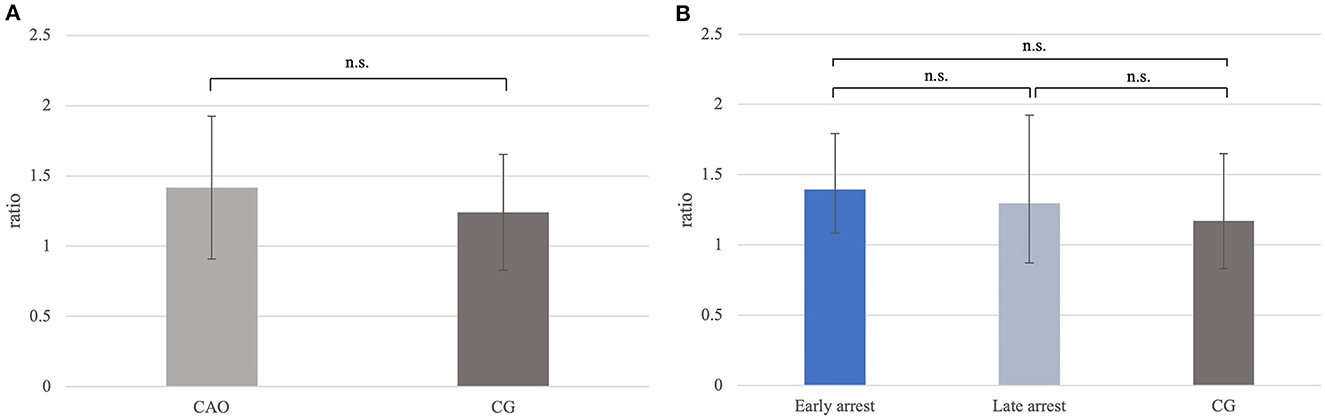
Figure 8. (A) FOXO1 mRNA expression (ratio) in groups of dogs with immune-mediated orchitis (CAO) and healthy control dogs (CG). The results are presented as geometric mean and dispersion factor [g (DF)]. (B) FOXO1 mRNA expression (ratio) in dogs with CAO divided into early and late arrest and control dogs (CG). The results are presented as geometric mean and dispersion factor [g (DF)].
The specificity and reactivity of the FOXO1 antibody were demonstrated by the Western blot using the testicular tissue and an appropriate positive control (Figure 2D). The canine band was located at the same molecular weight (72–75 kD) as the positive control.
Immunopositive staining against FOXO1 was specifically found in the cytoplasm of spermatogonia in CAO and CG (Figure 9) with the staining intensity differing considerably between the samples, but independent of the group (CAO/CG). FOXO1+ expression was limited to a specific subgroup within the spermatogonial population with FOXO1+ spermatogonia characterized by a prominent round nucleus and extensive cytoplasm (Figure 9).
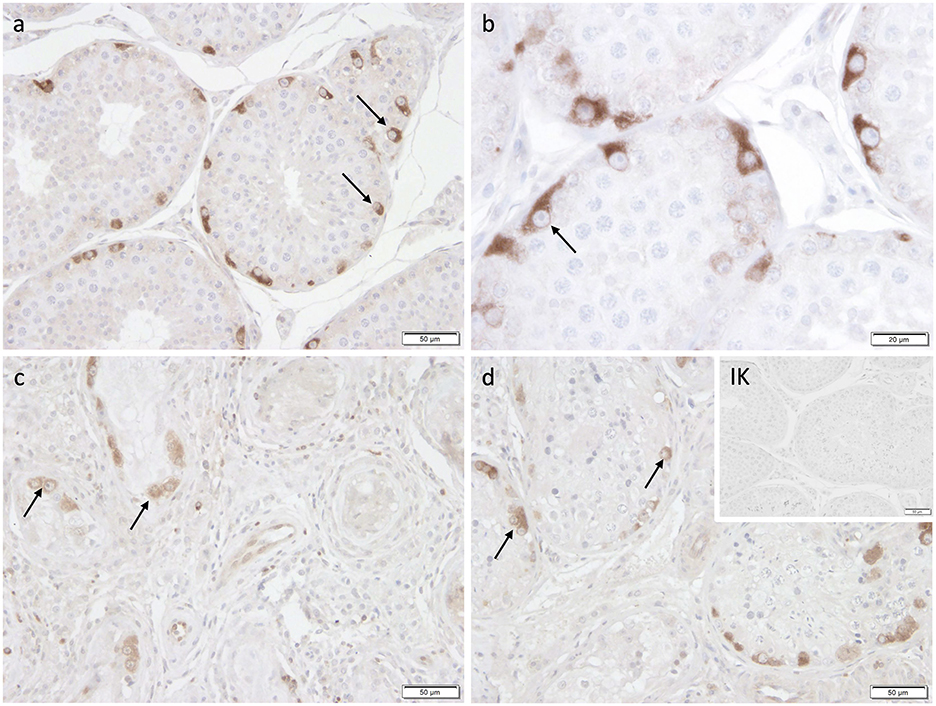
Figure 9. Immunostaining against FOXO1 (a-d) in CG and CAO dogs (200x magnification, (d) 400x magnification). (a, b) CG, (c, d) CAO [(c) early arrest; (d) late arrest]. Spermatogonia (black arrow).
Significantly fewer FOXO1+ signals were identified in CAO compared with CG (p < 0.0001, Figure 10A). In addition, the Kruskal–Wallis test showed a significant difference between all three groups (p < 0.0001), with the results obtained from early and late arrest samples differing significantly from CG (p = 0.0007; late: p = 0.009), but no results from early and late arrest differed significantly (Figure 10B).
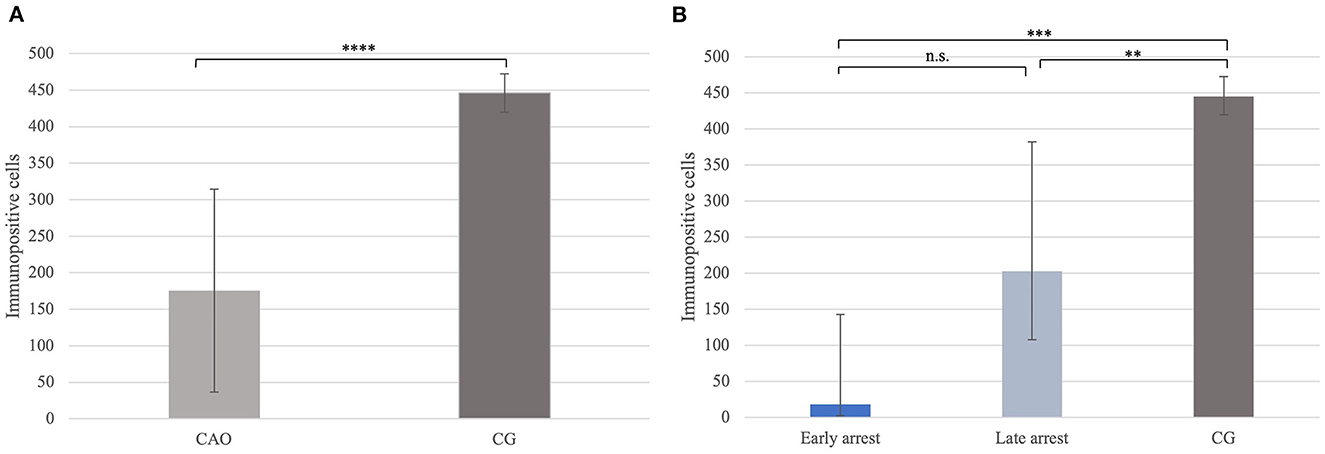
Figure 10. (A) FOXO1 protein expression evaluated as the number of immunopositive cells in 20 fields of vision in groups of dogs suffering from immune-mediated orchitis (CAO) and healthy control dogs (CG). The results are presented as arithmetic mean and standard deviation (SD). Datasets with asterisks differ significantly: ****p < 0.0001. (B) FOXO1 protein expression evaluated as the number of immunopositive cells in 20 fields of vision in groups of early and late arrest (CAO) and control dogs (CG). The results are presented as geometric mean and dispersion factor [g (DF)]. Datasets with asterisks differ significantly: ***p = 0.0007; **p = 0.009.
3.4. C-Kit mRNA and protein expression
The ratio for C-Kit mRNA expression did not differ significantly between CAO and CG (Figure 11A), nor overall between early and late arrest as well as CG (Figure 11B).
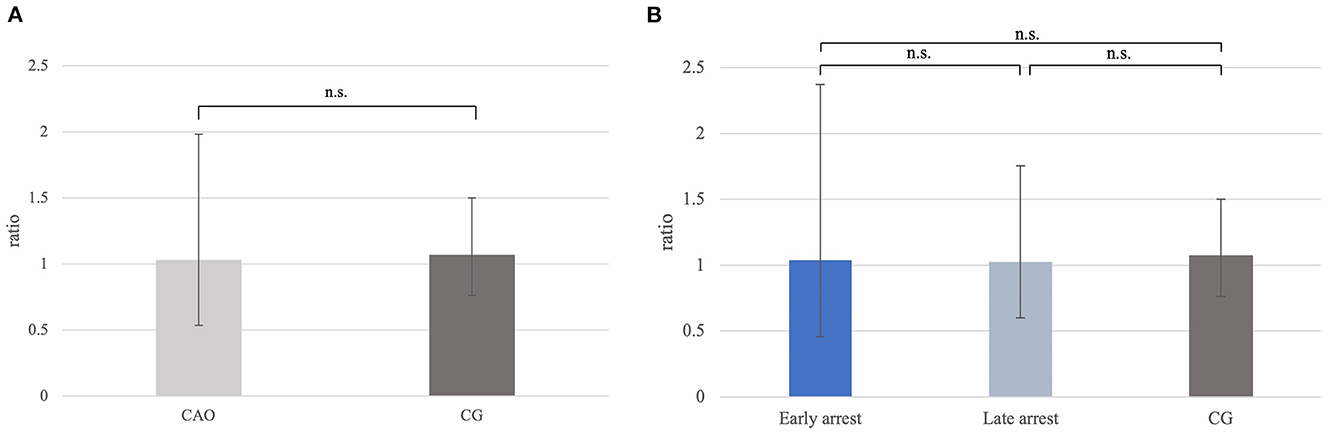
Figure 11. (A) C-Kit mRNA expression (ratio) in groups of dogs with immune-mediated orchitis (CAO) and healthy control dogs (CG). The results are presented as geometric mean and dispersion factor [g (DF)]. (B) C-Kit mRNA expression (ratio) in dogs with CAO divided into early and late arrest and control dogs (CG). Results are presented as geometric mean and dispersion factor [g (DF)].
The Western blot for C-Kit revealed a specific immunoreactive band using protein homogenate from an adult dog testis and canine mast cell tumor tissue as positive control; however, the molecular weight of the canine testicular band was slightly higher (approximately 150 kD) than that of the positive control (140 kD) (Figure 2B). Nevertheless, both bands are located within the predicted range of 140-155 kD indicating specificity.
Specific cytoplasmatic staining against C-Kit was identified in spermatogonia and Leydig cells in CAO and CG. Interestingly, not all spermatogonia stained C-Kit+; sporadic cells with prominent round nuclei and extensive cytoplasm, also considered as spermatogonia, did not show any immunopositive signal (Figure 12).
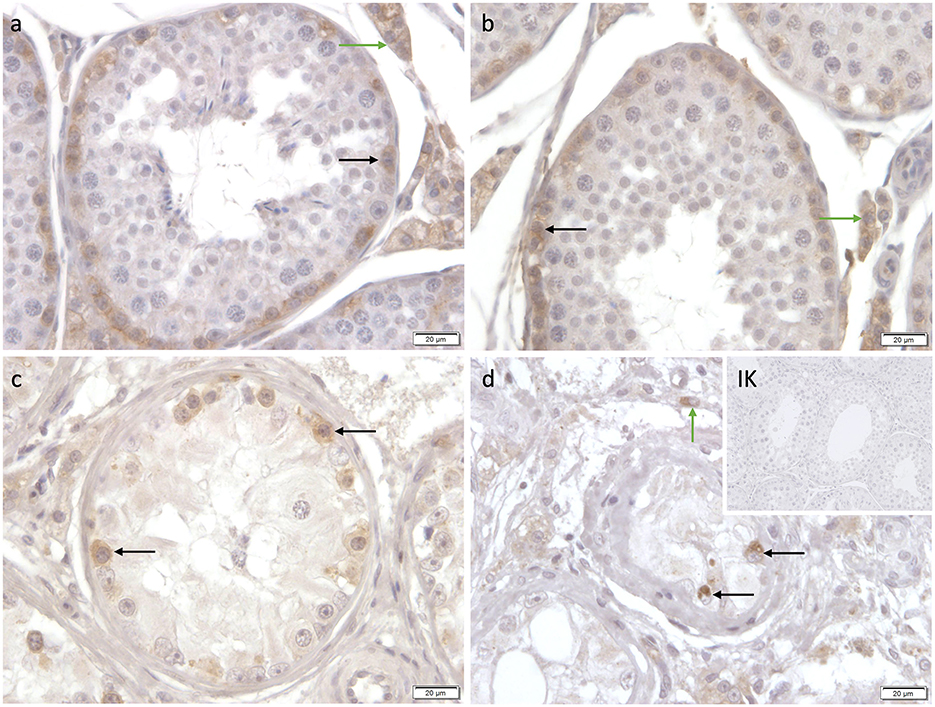
Figure 12. Immunostaining against C-Kit (a-d) in CG and CAO dogs (400x magnification). (a, b) CG, (c, d) CAO [(c) early arrest; (d) late arrest]. Spermatogonia (black arrow); Leydig cells (green arrow).
4. Discussion
Testicular NOA is the most common cause of infertility in breeding male dogs (5), but also in men (9, 10, 77). Based on our recent findings, idiopathic CAO is an important, but neglected cause of infertility in dogs (8) and men (78). Due to the similarity of findings, such as the lack of clinical signs, lack of the underlying etiology causing immune cell infiltration, and disruption of spermatogenesis, we postulate that CAO in dogs is suitable to gain further insights into human infertility due to CAO. This might be helpful to identify effective treatment options that are still not available for both, dogs (23, 79–84) and men (24, 26, 85–88). Although significantly upregulated PTGS2 expression points to the importance of inflammation as a key process in canine CAO (89), it seems unlikely that selective Cox-2 inhibitors (alone) are suitable to protect fertility indicating the need for other therapeutic options such as assisted reproductive techniques (24, 90, 91). Stem cell-based therapeutic options (36, 42, 92) to (re-) initialize spermatogenesis (37, 38, 40, 47, 93–98) might be a future treatment for CAO-affected dogs, too.
Considering the option of a stem-cell-based therapeutic approach in CAO-affected dogs, knowledge about the presence and possible dysregulation of putative SSCs in canine CAO testes is crucial. Despite the fact that we did not study the final ability of the cells to engraft (47), our results significantly improved the understanding of canine CAO. Confirming the presence of all investigated biomarkers at mRNA and protein levels in CAO-affected canine testes supports our hypothesis that among the detected germ cells resilient SSCs are present—with the potential to replenish the germ cell pool and (re-) initiate spermatogenesis. Further studies should include transplantation assays to finally confirm the true stem cell potential of the surviving cells in canine CAO testes.
In detail, the PGP9.5 and DAZL mRNA ratio of total testicular homogenates and the number of PGP9.5+, DAZL+, and FOXO1+ spermatogonia were significantly decreased in CAO samples compared with normal, healthy testis indicating a severe disruption of spermatogenesis (8). As undifferentiated and differentiating spermatogonia were PGP9.5+ in the CAO-affected testis as described in healthy testicular tissues (43, 53, 99), the observed reduction in immunopositive cells supports that all spermatogonial populations are affected by inflammatory and immune-cell mediated changes related to CAO. Similar to previous observations in gracile axonal dystrophic (gad) mice with a deletion within the gene encoding PGP9.5 (100), apoptosis was significantly increased (66) and proliferation of germ cells reduced (unpublished) in canine CAO testis supporting our hypothesis that the accurate regulation of PGP9.5 mRNA expression is crucial for maintaining canine spermatogenesis, too. Inflammatory changes in canine CAO testis altered PGP9.5 expression; it remains to be clarified whether inflammation is causative for the alteration or a consequence. In addition to the PGP9.5+ staining in the early stages of spermatocytes attached to the basal membrane (45), Leydig cells stained positive as previously described for donkeys (99), stallions (101), and men (102), but not for dogs (43). Interestingly, Leydig cells were predominantly stained in CAO samples and especially around the tubules with early arrested spermatogenesis. Surprisingly, another unanticipated PGP9.5+ signal was detected in spermatogonia, and preleptotene spermatocytes detached from the basal membrane in the CAO testis. This indicates apoptosis within the early germ cell population, supporting our recent data (66) as well as the postulated PGP9.5 role as a crucial modulator for germ cell apoptosis and sperm quality control during spermatogenesis to maintain testicular homeostasis (99, 103, 104).
The reduced DAZL mRNA level in early arrest samples compared with the late arrest further supports that not only the loss (105) but also already a reduction of DAZL might disrupt spermatogonial development and might lead to meiotic arrest at early pachytene. Nevertheless, so far, we cannot determine if the reduced DAZL mRNA expression in CAO contributes to the arrest of spermatogenesis or if the reduced number of spermatocytes leads to lower DAZL mRNA expression in cases of early arrest, although the latter possibility seems more likely. However, the reduction of DAZL mRNA and protein expression during canine CAO has crucial impacts on its role in translational regulation and affects numerous target mRNAs during SSC maintenance and differentiation. Different from the published findings in men and mice (58) indicating a transit of DAZ family protein from the nucleus to cytoplasm during meiosis, we identified DAZL+ expression in the cytoplasm of undifferentiated and differentiating spermatogonia and of spermatocytes as observed by Pieri et al. (49) in the dog.
However, FOXO1 mRNA expression in CAO and CG did not differ, significantly fewer FOXO1+ cells were identified in CAO supporting the need for analysis of this target at the protein level (50) due to posttranslational regulation through phosphorylation, acetylation, mono- and polyubiquitination (106). To what extent the posttranslational regulation of FOXO1 is altered during canine CAO has not yet been characterized. FOXO1 protein was localized in undifferentiated spermatogonia in CAO and CG samples reflecting the results of Tarnawa et al. (50) in 11 species studied including the dog. As FOXO1 plays an essential role in SSC maintenance and differentiation in mice, inactivation or loss of FOXO1 causes defects in SSC and therefore spermatogenesis itself (61). Consequently, detecting FOXO1+ undifferentiated spermatogonia in the testes of CAO patients has important implications for the prognosis and development of treatment options, as residual FOXO1+ cells provide great potential for reinitializing spermatogenesis. Whether the variable staining intensity is biologically relevant and due to defects in SSCs or due to a transition to differentiating spermatogonia, with a decrease and extinction of FOXO1 expression, remains unclear.
Similar to FOXO1, the mRNA level of C-Kit did not differ significantly between CAO and CG or between the early or late arrest against CG as shown also in the case of different forms of maturation arrests (107). A possible explanation for this observation could be the relatively high C-Kit gene activity in the interstitial cell compartment compared with the intratubular testicular compartment in men (107). As our previous study verified an “enrichment” of the interstitial compartment in CAO testis (8), the missing significance might be attributable to the altered compartment composition supporting the need for laser-assisted cell-picked tissue for mRNA analysis (tubular vs. interstitial compartment). Although C-Kit protein expression subjectively appears to be reduced in the cases of CAO, we did not determine the number of C-Kit+ spermatogonia quantitatively. Nevertheless, it seems likely that C-Kit mRNA transcription and translation are regulated independently by distant systems, as earlier studies found evidence that undifferentiated spermatogonia can express C-Kit mRNA, but not protein (108). C-Kit protein expression and localization seems to differ between species. Whereas, staining of spermatogonia (membranous and cytoplasm), round spermatids (acrosomal), and peritubular Leydig cells was observed in human adult testis (109), germ cells within the stage of type A2 spermatogonia to preleptotene spermatocytes and Leydig cells (110) were C-Kit+ in mice and staining in stallion were restricted to differentiating spermatogonia (65). Our results in canine CG and CAO testis with C-Kit+ expression in differentiating spermatogonia and Leydig cells were controversial to the observations by Lee et al. (45) detecting C-Kit in spermatocytes differentiating to spermatids and Sertoli cells in adult canine testes, but are in agreement with Grieco et al. (111). However, as the tyrosin-kinase C-Kit and its ligand the stem cell factor (SCF) were postulated to be important modulators of spermatogonial proliferation (112) and differentiation (113) and due to the agreement of observations in human and canine testes, our results confirm C-Kit+ expression in a subpopulation of spermatogonia undergoing or committed to differentiation.
Taken together, these findings contribute to our understanding of CAO as accompanied by or possibly even attributable to a significant loss of SSCs. Further research is required to provide deeper insights into the effects of lymphoplasmacytic inflammation and seminiferous tubular degeneration on the maintenance and viability of SSCs. Notwithstanding, our data confirm also for the first time the survival of putative stem cell populations in the damaged testicular tissues probably providing the opportunity for stem cell-based therapeutic options, such as testicular grafting. Further studies are, however, required to investigate this approach in CAO-affected dogs focusing also on possible limitations of testicular grafting (38, 114) in adult dogs with a likely severely disrupted SSC niche due to immune cell infiltration as a cause or consequence of CAO.
5. Conclusion
The present study not only enhances our understanding of the pathophysiology of canine CAO but is also the first study revealing the presence of putative SSC markers PGP9.5, DAZL, FOXO1, and C-Kit in CAO-affected canine testicular tissues. Overall, expression of investigated biomarkers was significantly lower in CAO, mostly at the protein level, but also regarding mRNA expressions which supports the hypothesis that immunological and inflammatory changes lead to a disturbance in the maintenance and differentiation of SSCs and consequently to a collapse of testicular homeostasis in CAO-affected dogs. As previously postulated, the CAO tissue is most severely affected in cases with early arrest possibly pointing to the fact that this might be the more “chronic stage” of CAO compared with late arrest. Despite the limitations, these results lay the groundwork for further research into stem cell-based therapeutic options such as testicular xenografting in CAO-affected dogs. Due to the fact that different spermatogonial populations including putative SSCs survive but are unable to provide a continuous output of spermatozoa, further research is required to focus on the surrounding microenvironment, the so-called SSC Niche, to fully understand the implications of chronic CAO on canine spermatogonial stem cells.
Data availability statement
The raw data supporting the conclusions of this article will be made available by the authors, without undue reservation.
Ethics statement
Collection of CAO samples for diagnostic purposes was approved by the Dyreforsøgstilsynet Fødevarestyrelsen and by the animal Ethics Committee of the University of Veterinary Medicine Hannover, Foundation. Testis samples from control animals were taken from dogs that were presented for routine castration for other than health issues. Written informed consent was obtained from the owners for the participation of their animals in this study.
Author contributions
SG-P and LR: conceptualization, sample collection, funding acquisition, and writing–original draft preparation. E-MP, HK, LR, and SG-P: methodology. LR, HK, E-MP, and SG-P: formal analysis, investigation, and writing—reviewing and editing. LR, HK, and SG-P: visualization. SG-P: supervision. All authors contributed to the article and approved the submitted version.
Funding
This study was generously supported by a scholarship from the Akademie für Tiergesundheit e.V. (Bonn-Bad Godesberg, Germany). This Open Access publication was funded by the Deutsche Forschungsgemeinschaft (DFG, German Research Foundation)—491094227-Open Access Publication Funding and the University of Veterinary Medicine Hannover, Foundation.
Acknowledgments
The authors thank Prof. Dr. Andreas Beineke (University of Veterinary Medicine Hannover, Foundation, Department of Pathology) for kindly providing the C-Kit test antibody and appropriate positive control.
Conflict of interest
The authors declare that the research was conducted in the absence of any commercial or financial relationships that could be construed as a potential conflict of interest.
Publisher's note
All claims expressed in this article are solely those of the authors and do not necessarily represent those of their affiliated organizations, or those of the publisher, the editors and the reviewers. Any product that may be evaluated in this article, or claim that may be made by its manufacturer, is not guaranteed or endorsed by the publisher.
References
1. Johnston SD, Root Kustritz M V, Olsen PNS. Clinical Approach to Infertility in the Male Dog. In:Kersey R, LeMelledo D, , editors. Canine and Feline Theriogenology. Philadelphia, PA, USA: Saunders, Philadelphia, PA, USA (2001). p. 370–88.
2. Memon MA. Common causes of male dog infertility. Theriogenology. (2007) 68:322–8. doi: 10.1016/j.theriogenology.2007.04.025
3. Olsen PN. Clinical and laboratory findings associated with actual or suspected azoospermia in dogs: 18 cases (1979-1990). J Am Vet Med Assoc. (1992) 201:478–82.
4. Dubiel A. Evaluation of semen properties and ejaculation reflex in dogs with reference to fertility. In: VIIIth International Congress on Animal Reproduction and Artificial Insemination, Krakow, July 12-16, 1976 Proceedings Volume I Communication Abstracts. Institute of Pathology and Therapy of Animals, Agricultural Academy, Wroclaw, Poland (1976), p. 75.
5. Romagnoli S. Two common causes of infertility in the male dog. In:Svoboda M, , editor. 2006 World Congress Proceedings 31st World Small Animal Association Congress, 12th European Congress FECAVA, & 14th Czech Small Animal Veterinary Association Congress, Prague, Czech Republic. European College of Animal Reproduction, Department of Veterinary Clinical Sciences, University of Padova Agripolis, Legnaro 35020 (PD), Italy.: Czech Small Animal Veterinary Association (2006) p. 686–90.
6. Olsen PNS. Clinical approach for evaluation dogs with azoospermia or aspermia. Vet Clin North Am Small Anim Pract. (1991) 21:591–608. doi: 10.1016/S0195-5616(91)50062-0
7. Goericke-Pesch S, Wehrend A. Bestimmung der alkalischen Phosphatase im Seminalplasma zu Differenzierung von Azoospermie und unvollständiger Ejakulation. Tierärztliche Prax. (2008) 36:A24. doi: 10.1055/s-0038-1622694
8. Goericke-Pesch S, Reifarth L, Behrens Mathiesen C, Schuler G, Umbach AK, Körber H. Chronic Immune-mediated orchitis is the major cause of acquired non-obstructive azoospermia in dogs. Front Vet Sci. (2022) 9:1–14. doi: 10.3389/fvets.2022.865967
9. Cerván-Martín M, Castilla JA. Palomino-Morales F, Rogelio J. Genetic Landscape of Nonobstructive Azoospermia and New Perspectives for the Clinic. J Clin Med. (2020) 9:300. doi: 10.3390/jcm9020300
10. Wosnitzer M, Goldstein M, Hardy MP. Review of azoospermia. Spermatogenesis. (2014) 4:1–7. doi: 10.4161/spmg.28218
11. Tüttelmann F, Nieschlag E. Nosologie andrologischer Krankheitsbilder. In: Andrologie - Grundlagen und Klinik der reproduktiven Gesundheit des Mannes. 3. Auflage. Berlin Heidelberg New York: Nieschlag E, Behre H, Nieschlag S (2009). p. 90–6.
12. Lustig L, Guazzone VA, Theas MS, Pleuger C, Jacobo P, Pérez CV, et al. Pathomechanisms of Autoimmune Based TesticularInflammation. Front Immunol. (2020) 11:1–8. doi: 10.3389/fimmu.2020.583135
13. Fijak M, Pilatz A, Hedger MP, Nicolas N, Bhushan S, Michel V, et al. Infectious, inflammatory and “autoimmune” male factor infertility: How do rodent models inform clinical practice? Hum Reprod Update. (2018) 24:416–41. doi: 10.1093/humupd/dmy009
14. Schuppe HC, Pilatz A, Hossain H, Diemer T, Wagenlehner F, Weidner W. Urogenital infection as a risk factor for male infertility. Dtsch Arztebl Int. (2017) 114:339–46. doi: 10.3238/arztebl.2017.0339
15. Fritz TE, Lombard LS, Tyler SA, Norris WP. Pathology and familial incidence of orchitis and its relation to thyroiditis in a closed beagle colony. Exp Mol Pathol. (1976) 24:142–58. doi: 10.1016/0014-4800(76)90002-2
16. Allen WE, Longstaffe JA. Spermatogenic arrest associated with focal degenerative orchitis in related dogs. J Small Anim Pract. (1982) 23:337–43. doi: 10.1111/j.1748-5827.1982.tb01675.x
17. Allen WE, Patel JR. Autoimmune orchitis in two related dogs. J Small Anim Pract. (1982) 23:713. doi: 10.1111/j.1748-5827.1982.tb02064.x
18. Davidson AP, von Dehn BJ, Schlafer DH. Adult-Onset Lymphoplasmacytic Orchitis in a Labrador Retriever Stud Dog. Top Companion Anim Med. (2015) 30:31–4. doi: 10.1053/j.tcam.2015.03.003
19. Matschurat C, Rode K, Hollenbach J, Wolf K, Urhausen C, Beineke A, et al. Impaired spermatogenesis, tubular wall disruption, altered blood-testis barrier composition and intratubular lymphocytes in an infertile Beagle dog — a putative case of autoimmune orchitis. Histol Histopathol. (2019) 34:525–35. doi: 10.14670/HH-18-058
20. Metcalfe SS, Gunn IM, Champness KA. Azoospermia in two Labrador Retrievers. Aust Vet J. (1999) 77:570–3. doi: 10.1111/j.1751-0813.1999.tb13191.x
21. Pröbstl C, Umbach A, Beineke A, Körber H, Goericke-Pesch S. Immune cell characterization in spontaneous autoimmune orchitis in dogs. Theriogenology. (2022) 187:219–26. doi: 10.1016/j.theriogenology.2022.05.010
22. Bruun Jensen H, Engelhard Holm J, Körber H, Goericke-Pesch S. Expression of connexin 43 and androgen receptor in testes of azoospermic dogs. In: Proceedings of the 21st EVSSAR congress Venice, 22nd-23rd of June 2018. (2018), p. 114.
24. Esteves SC. Clinical management of infertile men with nonobstructive azoospermia. Asian J Androl. (2015) 17:459–70. doi: 10.4103/1008-682X.148719
25. Silva CA, Cocuzza M, Borba EF, Bonfá E. Cutting-edge issues in autoimmune orchitis. Clin Rev Allergy Immunol. (2012) 42:256–63. doi: 10.1007/s12016-011-8281-z
26. Silva CA, Cocuzza M, Carvalho JF, Bonfá E. Diagnosis and classification of autoimmune orchitis. Autoimmun Rev. (2014) 13:431–4. doi: 10.1016/j.autrev.2014.01.024
27. Neuhaus N, Schlatt S. Stem cell-based options to preserve male fertility. Reproduction. (2019) 363:8–10. doi: 10.1126/science.aaw6927
28. De Rooij DG. The nature and dynamics of spermatogonial stem cells. Development. (2017) 144:3022–30. doi: 10.1242/dev.146571
29. Lord T, Oatley JM. A revised Asingle model to explain stem cell dynamics in the mouse male germline. Reproduction. (2017) 154:R55–64. doi: 10.1530/REP-17-0034
30. González R, Dobrinski I. Beyond the mouse monopoly: Studying the male germ line in domestic animal models. ILAR J. (2015) 56:83–98. doi: 10.1093/ilar/ilv004
31. Griswold MD. Spermatogenesis: the commitment to meiosis. Physiol Rev. (2016) 96:1–17. doi: 10.1152/physrev.00013.2015
32. Klein AM, Nakagawa T, Ichikawa R, Yoshida S, Simons BD. Mouse germ line stem cells undergo rapid and stochastic turnover. Cell Stem Cell. (2010) 7:214–24. doi: 10.1016/j.stem.2010.05.017
33. Hara K, Nakagawa T, Enomoto H, Suzuki M, Yamamoto M, Simons BD, et al. Mouse spermatogenic stem cells continually interconvert between equipotent singly isolated and syncytial states. Cell Stem Cell. (2014) 14:658–72. doi: 10.1016/j.stem.2014.01.019
34. Nakagawa T, Sharma M, Nabeshima YI, Braun RE, Yoshida S. Functional hierarchy and reversibility within the murine spermatogenic stem cell compartment. Science. (2010) 328:62–7. doi: 10.1126/science.1182868
35. Phillips BT, Gassei K, Orwig KE. Spermatogonial stem cell regulation and spermatogenesis. Philos Trans R Soc B Biol Sci. (2010) 365:1663–78. doi: 10.1098/rstb.2010.0026
36. Pieri NCG, de Souza AF, Botigelli RC, Machado LS, Ambrosio CE, dos Santos Martins D, et al. Stem cells on regenerative and reproductive science in domestic animals. Vet Res Commun. (2019) 43:7–16. doi: 10.1007/s11259-019-9744-6
37. Kim Y, Turner D, Nelson J, Dobrinski I, McEntee M, Travis AJ. Production of donor-derived sperm after spermatogonial stem cell transplantation in the dog. Reproduction. (2008) 136:823–31. doi: 10.1530/REP-08-0226
38. Abrishami M, Abbasi S, Honaramooz A. The effect of donor age on progression of spermatogenesis in canine testicular tissue after xenografting into immunodeficient mice. Theriogenology. (2010) 73:512–22. doi: 10.1016/j.theriogenology.2009.09.035
39. Lee WY, Lee R, Song H, Hur T-Y, Lee S, Ahn J, et al. Establishment of a surgically induced cryptorchidism canine recipient model for spermatogonial stem cell transplantation. Lab Anim Res. (2016) 32:257. doi: 10.5625/lar.2016.32.4.257
40. Pieri NCG, Mançanares ACF, De Souza AF, Fernandes H, Diaza AMG, Bressan FF, et al. Xenotransplantation of canine spermatogonial stem cells (cSSCs) regulated by FSH promotes spermatogenesis in infertile mice. Stem Cell Res Ther. (2019) 10:1–11. doi: 10.1186/s13287-019-1250-9
41. Ibtisham F, Honaramooz A. Spermatogonial stem cells for in vitro spermatogenesis and in vivo restoration of fertility. Cells. (2020) 9:745. doi: 10.3390/cells9030745
42. Ibtisham F, Awang-Junaidi AH, Honaramooz A. The study and manipulation of spermatogonial stem cells using animal models. Cell Tissue Res. (2020) 380:393–414. doi: 10.1007/s00441-020-03212-x
43. Lee KH, Lee R, Lee WY, Kim DH, Chung HJ, Kim JH, et al. Identification and in vitro derivation of spermatogonia in beagle testis. PLoS One. (2014) 9:1–9. doi: 10.1371/journal.pone.0109963
44. Lee KH, Lee WY, Kim DH, Lee SH, Do JT, Park C, et al. Vitrified canine testicular cells allow the formation of spermatogonial stem cells and seminiferous tubules following their xenotransplantation into nude mice. Sci Rep. (2016) 6:1–11. doi: 10.1038/srep21919
45. Lee WY, Lee R, Park H-J, Do JT, Park C, Kim J-H, et al. Characterization of male germ cell markers in canine testis. Anim Reprod Sci. (2017) 182:1–8. doi: 10.1016/j.anireprosci.2017.01.002
46. Lee WY, Park HJ, Lee R, Lee JH, Jhun H, Hur TY, et al. Analysis of putative biomarkers of undifferentiated spermatogonia in dog testis. Anim Reprod Sci. (2017) 185:174–80. doi: 10.1016/j.anireprosci.2017.08.017
47. Harkey MA, Asano A, Zoulas ME, Torok-Storb B, Nagashima J, Travis A. Isolation, genetic manipulation, and transplantation of canine spermatogonial stem cells: Progress toward transgenesis through the male germ-line. Reproduction. (2013) 146:75–90. doi: 10.1530/REP-13-0086
48. Chen YC, Hsu WL, Chiu CY, Liao JW, Chang CC, Chang SC. Expression of MAGE-A restricted to testis and ovary or to various cancers in dogs. Vet Immunol Immunopathol. (2013) 153:26–34. doi: 10.1016/j.vetimm.2013.01.012
49. Pieri NCG, Souza AF, Mançanares ACF, Roballo KCS, Casals JB, Ambrosio CE, et al. Immunolocalization of proteins in the spermatogenesis process of canine. Reprod Domest Anim. (2017) 52:170–6. doi: 10.1111/rda.12848
50. Tarnawa ED, Baker MD, Aloisio GM, Carr BR, Castrillon DH. Gonadal expression of Foxo1, but not Foxo3, is conserved in diverse Mammalian species. Biol Reprod. (2013) 88:1–11. doi: 10.1095/biolreprod.112.105791
51. Kwon J, Wang Y-L, Setsuie R, Sekiguchi S, Sakurai M, Sato Y, et al. Developmental regulation of ubiquitin c-terminal hydrolase isozyme expression during spermatogenesis in mice. Biol Reprod. (2004) 71:515–21. doi: 10.1095/biolreprod.104.027565
52. Sutovsky P. Ubiquitin-dependent proteolysis in mammalian spermatogenesis, fertilization, and sperm quality control: Killing three birds with one stone. Microsc Res Tech. (2003) 61:88–102. doi: 10.1002/jemt.10319
53. Luo J, Meege S, Dobrinski I. Asymmetric Distribution of UCH-L1 in Spermatogonia Is Associated With Maintenance and Differentiation of Spermatogonial Stem Cells. J Cell Physiol. (2009) 220:460–8. doi: 10.1002/jcp.21789
54. Luo J, Megee S, Rathi R, Dobrinski I. Protein Gene Product 9. 5 Is a Spermatogonia-Specific Marker in the Pig Testis: Application to Enrichment and Culture of Porcine Spermatogonia Mol Reprod Dev. (2006) 73:1531–40. doi: 10.1002/mrd.20529
55. Wrobel KH. Prespermatogenesis and spermatogoniogenesis in the bovine testis. Anat Embryol (Berl). (2000) 202:209–22. doi: 10.1007/s004290000111
56. Eberhart CG, Maines JZ, Wasserman SA. Meiotic cell cycle requirement for a fly homologue of human deleted in Azoospermia. Nature. (1996) 381:783–5. doi: 10.1038/381783a0
57. Collier B, Gorgoni B, Loveridge C, Cooke HJ, Gray NK. The DAZL family proteins are PABP-binding proteins that regulate translation in germ cells. EMBO J. (2005) 24:2656–66. doi: 10.1038/sj.emboj.7600738
58. Reijo RA, Dorfman DM, Slee R, Renshaw AA, Loughlin KR, Cooke H, et al. DAZ family proteins exist throughout male germ cell development and transit from the nucleus to cytoplasm at meiosis in humans and mice. Biol Reprod. (2000) 63:1490–6. doi: 10.1095/biolreprod63.5.1490
59. Luo J, Rodriguez-Sosa JR, Tang L, Bondareva A, Megee S, Dobrinski I. Expression pattern of acetylated α-tubulin in porcine spermatogonia. Mol Reprod Dev. (2010) 77:348–52. doi: 10.1002/mrd.21153
60. Ruggiu M, Speed R, Taggart M, McKay SJ, Kilanowski F, Saunders P, et al. The mouse Dazla gene encodes a cytoplasmic protein essential for gametogenesis. Nature. (1997) 389:73–7. doi: 10.1038/37987
61. Goertz MJ, Wu Z, Gallardo TD, Hamra FK, Castrillon DH. Foxo1 is required in mouse spermatogonial stem cells for their maintenance and the initiation of spermatogenesis. J Clin Invest. (2011) 121:3456–66. doi: 10.1172/JCI57984
62. Schrans-Stassen BHGJ, Van De Kant HJG, De Rooij DG, Van Pelt AMM. Differential expression of c-kit in mouse undifferentiated and differentiating type A spermatogonia. Endocrinology. (1999) 140:5894–900. doi: 10.1210/endo.140.12.7172
63. Sandlow JI, Feng HL, Sandra A. Localization and expression of the c-kit receptor protein in human and rodent testis and sperm. Urology. (1997) 49:494–500. doi: 10.1016/S0090-4295(96)00494-3
64. Heidari B, Rahmati-Ahmadabadi M, Akhondi MM, Zarnani AH, Jeddi-Tehrani M, Shirazi A, et al. Isolation, identification, and culture of goat spermatogonial stem cells using c-kit and PGP9. 5 markers J Assist Reprod Genet. (2012) 29:1029–38. doi: 10.1007/s10815-012-9828-5
65. Jung H, Song H, Yoon M. The KIT is a putative marker for differentiating spermatogonia in stallions. Anim Reprod Sci [Internet]. (2015) 152:39–46. doi: 10.1016/j.anireprosci.2014.11.004
66. Morawietz J, Packeiser E-M, Körber H, Beinecke A, Goericke-Pesch S. Insights into canine infertility: Apoptosis in chronic asymptomatic immune-mediated orchitis. Int J Mol Sci. (2023) 24:1–22. doi: 10.3390/ijms24076083
67. Riesenbeck A, Völker D, Hoffmann B. Praxisnahe Bestimmung von Vitalitätsparameter zur Beurteilung von Rüdensperma. Tierärztliche Prax. (2001) 29:116–20.
68. Günzel-Apel A, Terhaer P, Waberski D. Hodendimensionen und Ejakulatbeschaffenheit fertiler Rüden unterschiedlicher Körpergewichte. Kleintierpraxis. (1994) 39:483–6.
69. Goericke-Pesch S, Spang A, Schulz M, Özalp G, Bergmann M, Ludwig C, et al. Recrudescence of spermatogenesis in the dog following downregulation using a slow release GnRH agonist implant. Reprod Domest Anim. (2009) 44:302–8. doi: 10.1111/j.1439-0531.2009.01378.x
70. Jungmann C, Houghton CG, Nielsen FG, Packeiser EM, Körber H, Reichler IM, et al. Involvement of oxytocin and progesterone receptor expression in the etiology of canine uterine inertia. Int J Mol Sci. (2022) 23:3601. doi: 10.3390/ijms232113601
71. Pfaffl MW, A. new mathematical model for relative quantification in real-time RT–PCR. Nucleic Acids Res. (2001) 29:E45. doi: 10.1093/nar/29.9.e45
72. Hellemans J, Mortier G, De Paepe A, Speleman F, Vandesompele J. qBase relative quantification framework and software for management and automated analysis of real-time quantitative PCR data. Genome Biol. (2008) 8:r19. doi: 10.1186/gb-2007-8-2-r19
73. Körber H, Goericke-Pesch S. Expression of PTGS2, PGFS and PTGFR during downregulation and restart of spermatogenesis following GnRH agonist treatment in the dog. Cell Tissue Res. (2019) 375:531–41. doi: 10.1007/s00441-018-2915-x
74. Körber H, Goericke-Pesch S. Expression of prostaglandin (PG) D synthase lipocalin and hematopoietic type and PG D receptor during restart of spermatogenesis following downregulation using a slow release GnRH agonist implant in the dog. Cell Tissue Res. (2019) 378:359–70. doi: 10.1007/s00441-019-03059-x
75. Rempel LM, Körber H, Reichler IM, Balogh O, Goericke-Pesch S. Investigations on the potential role of prostaglandin E2 in canine uterine inertia. Theriogenology. (2021) 175:134–47. doi: 10.1016/j.theriogenology.2021.09.003
76. Kajimoto Y, Hashimoto T, Shirai Y, Nishino N, Kuno T, Tanaka C. cDNA cloning and tissue distribution of a rat ubiquitin carboxyl-terminal hydrolase PGP9. J Biochem. (1992) 112:28–32. doi: 10.1093/oxfordjournals.jbchem.a123860
77. Kasak L, Laan M. Monogenic causes of non-obstructive azoospermia: challenges, established knowledge, limitations and perspectives. Hum Genet. (2021) 140:135–54. doi: 10.1007/s00439-020-02112-y
78. Schuppe HC, Pilatz A, Hossain H, Meinhardt A, Bergmann M, Haidl G, et al. Orchitis und Infertilität. Urologe. (2010) 49:629–35. doi: 10.1007/s00120-010-2256-1
79. Kawakami E, Hori T, Tsutsui T. Changes in Plasma LH and Testosterone levels and semen quality after a single injection of hcg in two dogs with spermatogenic dysfunction. J Vet Med Sci. (1998) 60:765–7. doi: 10.1292/jvms.60.765
80. Kawakami E, Taguchi N, Hirano T, Hori T, Tsutsui T. Therapeutic effect of aromatase inhibitor in two azoospermic dogs with high plasma estradiol-17β levels. J Vet Med Sci. (2003) 65:1343–5. doi: 10.1292/jvms.65.1343
81. Kawakami E, Hori T, Tsutsui T. Changes in plasma testosterone level and semen quality after frequent injections of GnRH analogue in a beagle dog with azoospermia. J Vet Med Sci. (2009) 71:1373–5. doi: 10.1292/jvms.001373
82. Kawakami E, Hirano T, Hori T, Tsutsui T. Improvement in spermatogenic function after subcutaneous implantation of a capsule containing an aromatase inhibitor in four oligozoospermic dogs and one azoospermic dog with high plasma estradiol-17β concentrations. Theriogenology. (2004) 62:165–78. doi: 10.1016/j.theriogenology.2003.09.021
83. Kawakami E, Kuroda D, Hori T, Tsutsui T. Changes in the plasma testosterone level and testicular superoxide dismutase activity of 5 azoospermic beagles after GnRH analogue injections. J Vet Med Sci. (2007) 69:561–2. doi: 10.1292/jvms.69.561
84. Kawakami E, Hori T, Tsutsui T. Changes in plasma luteinizing hormone, testosterone and estradiol-17β levels and semen quality after injections of gonadotropin releasing hormone agonist and human chorionic gonadotropin in three dogs with oligozoospermia and two dogs with azoospermia. Anim Reprod Sci. (1997) 47:157–67. doi: 10.1016/S0378-4320(97)00001-8
85. Selman H, De Santo M, Sterzik K, Cipollone G, Aragona C, El-Danasouri I. Rescue of spermatogenesis arrest in azoospermic men after long-term gonadotropin treatment. Fertil Steril. (2006) 86:466–8. doi: 10.1016/j.fertnstert.2005.12.055
86. Reifsnyder JE, Ramasamy R, Husseini J, Schlegel PN. Role of optimizing testosterone before microdissection testicular sperm extraction in men with nonobstructive azoospermia. J Urol. (2012) 188:532–6. doi: 10.1016/j.juro.2012.04.002
87. Hussein A, Ozgok Y, Ross L, Niederberger C. Clomiphene administration for cases of nonobstructive azoospermia: a multicenter study. J Androl. (2005) 26:787–91. doi: 10.2164/jandrol.04180
88. Hussein A, Ozgok Y, Ross L, Rao P, Niederberger C. Optimization of spermatogenesis-regulating hormones in patients with non-obstructive azoospermia and its impact on sperm retrieval: a multicentre study. BJU Int. (2013) 111:1–5. doi: 10.1111/j.1464-410X.2012.11485.x
89. Morawitz J, Körber H, Goericke-Pesch S. Expression of PTGS2 in testicular tissue of dogs with non-obstructive azoospermia. Reprod Domest Anim. (2021) 56:23–4. doi: 10.1111/rda.13908
90. Palermo GD, Neri Q V, Schlegel PN, Rosenwaks Z. Intracytoplasmic Sperm Injection (ICSI) in extreme cases of male infertility. PLoS ONE. (2014) 9:1–20. doi: 10.1371/journal.pone.0113671
91. Esteves SC. Microdissection testicular sperm extraction (micro-TESE) as a sperm acquisition method for men with nonobstructive azoospermia seeking fertility: operative and laboratory aspects. Int Brazilian J Urol. (2013) 39:440–1. doi: 10.1590/S1677-5538.IBJU.2013.03.21
92. Dobrinski I. Germ cell transplantation and testis tissue xenografting in domestic animals. Anim Reprod Sci. (2005) 89:137–45. doi: 10.1016/j.anireprosci.2005.06.020
93. Brinster RL, Avarbock MR. Germline transmission of donor haplotype following spermatogonial transplantation. Proc Natl Acad Sci U S A. (1994) 91:11303–7. doi: 10.1073/pnas.91.24.11303
94. Izadyar F, Den Ouden K, Stout TAE, Stout J, Coret J, Lankveld DPK, et al. Autologous and homologous transplantation of bovine spermatogonial stem cells. Reproduction. (2003) 126:765–74. doi: 10.1530/rep.0.1260765
95. Mikkola M, Sironen A, Kopp C, Taponen J, Sukura A, Vilkki J, et al. Transplantation of normal boar testicular cells resulted in complete focal spermatogenesis in a boar affected by the immotile short-tail sperm defect. Reprod Domest Anim. (2006) 41:124–8. doi: 10.1111/j.1439-0531.2006.00651.x
96. Honaramooz A, Behboodi E, Megee SO, Overton SA, Galantino-Homer H, Echelard Y, et al. Fertility and germline transmission of donor haplotype following germ cell transplantation in immunocompetent goats. Biol Reprod. (2003) 69:1260–4. doi: 10.1095/biolreprod.103.018788
97. Silva R, Costa GMJ, Lacerda SMSN, Batlouni SR, Soares JM, Avelar GF, et al. Germ cell transplantation in felids: a potential approach to preserving endangered species. J Androl. (2012) 33:264–76. doi: 10.2164/jandrol.110.012898
98. Nagano M, McCarrey JR, Brinster RL. Primate spermatogonial stem cells colonize mouse testes. Biol Reprod. (2001) 64:1409–16. doi: 10.1095/biolreprod64.5.1409
99. Choi Y, Jung Y, Kim S, Kim J, Jung H, Yoon M. Stage-dependent expression of protein gene product 9. 5 in donkey testes. Animals. (2020) 10:1–10. doi: 10.3390/ani10112169
100. Kwon J, Kikuchi T, Setsuie R, Ishii Y, Kyuwa S, Yoshikawa Y. Characterization of the testis in congenitally ubiquitin carboxy-terminal hydrolase-1 (Uch-L1) defective (gad) mice. Exp Anim. (2003) 52:1–9. doi: 10.1538/expanim.52.1
101. Jung H, Yoon M. Protein gene product 9. 5 Expression in Stallion Testes. J Equine Vet Sci. (2016) 45:1–7. doi: 10.1016/j.jevs.2016.02.227
102. Wilson POG, Barber PC, Hamidt QA, Powert BF, Dhillon AP, Rode J, et al. The immunolocalization of protein gene product 9. 5 using rabbit polyclonal and mouse monoclonal antibodies. Br J Exp Pathol. (1988) 69:91–104.
103. Kwon J, Mochida K, Wang YL, Sekiguchi S, Sankai T, Aoki S, et al. Ubiquitin C-terminal hydrolase L-1 is essential for the early apoptotic wave of germinal cells and for sperm quality control during spermatogenesis. Biol Reprod. (2005) 73:29–35. doi: 10.1095/biolreprod.104.037077
104. Kwon J. The new function of two ubiquitin C-terminal hydrolase isozymes as reciprocal modulators of germ cell apoptosis. Exp Anim. (2007) 56:71–7. doi: 10.1538/expanim.56.71
105. Li H, Liang Z, Yang J, Wang D, Wang H, Zhu M, et al. DAZL is a master translational regulator of murine spermatogenesis. Natl Sci Rev. (2019) 6:455–68. doi: 10.1093/nsr/nwy163
107. Bialas M, Borczynska A, Rozwadowska N, Fiszer D, Kosicki W, Jedrzejczak P, et al. SCF and c-kit expression profiles in male individuals with normal and impaired spermatogenesis. Andrologia. (2010) 42:83–91. doi: 10.1111/j.1439-0272.2009.00960.x
108. Prabhu SM, Meistrich ML, McLaughlin EA, Roman SD, Warne S, Mendis S, et al. Expression of c-Kit receptor mRNA and protein in the developing, adult and irradiated rodent testis. Reproduction. (2006) 131:489–99. doi: 10.1530/rep.1.00968
109. Unni SK, Modi DN, Pathak SG, Dhabalia J V, Bhartiya D. Stage-specific localization and expression of c-kit in the adult human testis. J Histochem Cytochem. (2009) 57:861–9. doi: 10.1369/jhc.2009.953737
110. Manova K, Nocka K, Besmer P, Bachvarova RF. Gonadal expression of c-kit encoded at the W locus of the mouse. Development. (1990) 110:1057–69. doi: 10.1242/dev.110.4.1057
111. Grieco V, Banco B, Giudice C, Mosca F, Finazzi M. Immunohistochemical expression of the kit protein (cd117) in normal and neoplastic canine testes. J Comp Pathol. (2010) 142:213–7. doi: 10.1016/j.jcpa.2009.07.002
112. Mauduit C, Hamamah S, Benahmed M. Stem cell factor/c-kit system in spermatogenesis. Hum Reprod Update. (1999) 5:535–45. doi: 10.1093/humupd/5.5.535
113. Rossi P, Sette C, Dolci S, Geremia R, Pubblica S, Anatomia S, et al. Role of c-kit in mammalian spermatogenesis. Endocrinology. (2000) 23:609–15. doi: 10.1007/BF03343784
Keywords: DAZL, FOXO1, PGP9.5, C-Kit, chronic asymptomatic orchitis, immune orchitis, Infertility, dog
Citation: Reifarth L, Körber H, Packeiser E-M and Goericke-Pesch S (2023) Detection of spermatogonial stem cells in testicular tissue of dogs with chronic asymptomatic orchitis. Front. Vet. Sci. 10:1205064. doi: 10.3389/fvets.2023.1205064
Received: 13 April 2023; Accepted: 25 May 2023;
Published: 15 June 2023.
Edited by:
Regiane R. Santos, Schothorst Feed Research, NetherlandsReviewed by:
Shanti Choudhary, Guru Angad Dev Veterinary and Animal Sciences University, IndiaAlexandre Rodrigues Silva, Federal University Rural Semi-Arid, Brazil
Copyright © 2023 Reifarth, Körber, Packeiser and Goericke-Pesch. This is an open-access article distributed under the terms of the Creative Commons Attribution License (CC BY). The use, distribution or reproduction in other forums is permitted, provided the original author(s) and the copyright owner(s) are credited and that the original publication in this journal is cited, in accordance with accepted academic practice. No use, distribution or reproduction is permitted which does not comply with these terms.
*Correspondence: Sandra Goericke-Pesch, Sandra.Goericke-Pesch@tiho-hannover.de