- 1Environmental Science and Management, Portland State University, Portland, OR, United States
- 2Fisheries, Wildlife, and Conservation Sciences, Oregon State University, Corvallis, OR, United States
Microplastics (MPs) and other anthropogenic particles (APs) are pervasive environmental contaminants found throughout marine and aquatic environments. We quantified APs in the edible tissue of black rockfish, lingcod, Chinook salmon, Pacific herring, Pacific lamprey, and pink shrimp, comparing AP burdens across trophic levels and between vessel-retrieved and retail-purchased individuals. Edible tissue was digested and analyzed under a microscope, and a subset of suspected APs was identified using spectroscopy (μFTIR). Anthropogenic particles were found in 180 of 182 individuals. Finfish contained 0.02–1.08 AP/g of muscle tissue. In pink shrimp (Pandalus jordani), the average AP/g was 10.68 for vessel-retrieved and 7.63 for retail-purchased samples; however, APs/g of tissue were higher in retail-purchased lingcod than vessel-retrieved lingcod, signaling possible added contamination during processing from ocean to market. Riverine young adult Pacific lamprey contained higher concentrations of APs (1 AP/g ±0.59) than ocean phase adults (0.60 AP/g ±0.80 and p = 0.08). Particle types identified were 82% fibers, 17% fragments, and 0.66% films. These findings suggest a need for further research into technologies and strategies to reduce microfiber pollution entering the environment.
1 Introduction
Anthropogenic particles (APs), a broad category of materials produced or modified by humans, include microplastics (MPs), plastics less than 5 mm in diameter at their longest dimension (Mattsson et al., 2021; Coffin et al., 2022). Environmental MPs are found in a variety of shapes, including films, foams, pellets, beads, fibers, fragments, and tire wear particles (Brander et al., 2020; Granek et al., 2020; Tamis et al., 2021), and polymer types, including polyester, polyethylene terephthalate (PET), high-density polyethylene (HDPE), and polyvinyl chloride (PVC). Anthropogenically modified substances refer to materials of anthropogenic origin or those that are heavily processed, like dyed cellulose textiles or poly-blends (Athey and Erdle, 2022). Since environmental particles are often a mix of MPs and other anthropogenically modified materials, we refer to APs throughout the paper, a term used by many researchers in the field (Gao et al., 2023; Adams et al., 2021).
To date, APs have been found in a variety of environmental media, including air, fresh and marine waters, sediment, wastewater, and organisms (Crawford and Quinn, 2017; Granek et al., 2022; Thornton Hampton et al., 2022). Pathways include microfibers (MFs) shed from laundering clothing (Galvão et al., 2020), MP beads from personal care products (Sun et al., 2020), and tire wear particles resulting from tire degradation (Goßmann et al., 2021; Siddiqui et al., 2022). APs can be transported aerially by wind (Brahney et al., 2020), into freshwater sources via wastewater treatment plants (WWTPs) and urban runoff (Horton et al., 2017), and into the ocean via rivers, WWTP effluent, and degradation of plastic litter (Cole et al., 2011). APs are abundant in terrestrial, freshwater, and marine environments (Wang et al., 2021) and have been found in the bodies of aquatic organisms across trophic levels, including cetaceans, avifauna, fishes, bivalves, and zooplankton (Currie et al., 2017; Romeo et al., 2015; Tanaka et al., 2019), as well as in human blood, tissue, and organs (Ragussa et al., 2021, 2022).
APs are manufactured with an array of chemicals and can also adsorb substances from the environment (Qi et al., 2021). Chemical additives used in plastic production, such as per and poly-fluorinated compounds (PFAS), phthalates, and colorants, can leach from plastics into water and body tissues over time (Sait et al., 2021; Wang et al., 2020). AP ingestion and adhesion can cause physical damage when internalized by marine organisms (Qiao et al., 2019) and lead to the transfer of constituent or associated chemicals to bodily tissues after ingestion (Tanaka et al., 2020). Gut damage (Qiao et al., 2019), adverse immune response (Sharifinia et al., 2020), protein and enzyme changes (Trestrail et al., 2021), stress response (Lanctôt et al., 2020), oxidative stress (Solomando et al., 2020), and false satiation or food dilution (Mallik et al., 2021) can result from AP exposure.
Despite the array of studies on AP ingestion across diverse species (Barboza et al., 2018; Carbery et al., 2018; Lusher et al., 2017), most studies to date have focused on bivalves in their entirety or the gastrointestinal tract of fish and crustacean species, leaving large gaps in our understanding of AP contamination in the tissue of commercially valuable finfish consumed by humans (Baechler et al., 2019; Dawson et al., 2021; Ferrante et al., 2022; Munno et al., 2021; Qiao et al., 2019; Rochman et al., 2015). However, Akoueson et al. (2020) found 0.50–1 AP/g of tissue in four finfish species collected from Scotland and Argentina, and a study of three Portuguese finfish found an average of 0.054 AP/g in dorsal muscle tissue (Barboza et al., 2020). These represent a limited number of species, geographies, habitats, and trophic levels and generate questions regarding baseline microplastic concentrations in finfish and crustaceans from different regions and trophic levels. Additionally, no known studies to date have examined the consumer source (vessel versus retail) of seafood and its relationship with AP abundance in seafood.
Oregon boasts numerous commercial, recreational, and traditional fisheries, an important part of the state’s coastal economy and fishing culture (Harte et al., 2008; Richerson et al., 2020; Robison, 2022; Sjostrom et al., 2021). There is growing interest in MP regulation and research in Oregon; however, due to the geographic variability in AP distribution and morphology, policymakers have expressed a need for further site-specific APs to guide decision-making (Das et al., 2021). Only two studies in Oregon have identified APs in consumed species (bivalves; Baechler et al., 2020; rockfish, Lasdin et al., 2023), and five studies have examined transport pathways and environmental abundance (Kapp and Yeatman, 2018; Murray et al., 2018; Talbot et al., 2022; Valine et al., 2020; Torres et al., 2023). There are no published studies on AP occurrence in the edible tissues of finfish and crustaceans in Oregon, yet such studies are important to catalyze policy-making. Across many of these studies performed in Oregon, the majority of particles identified were microfibers (Baechler et al., 2020; Lasdin et al., 2023; Torres et al., 2023).
This study aims to inform AP policy decisions by contributing to the research on AP contamination in Oregon finfish and shellfish and understand variation across trophic levels and feeding modes, as well as whether AP contamination differs across points in their pathway to consumers. Black rockfish, lingcod, Chinook salmon, Pacific herring, Pacific lamprey, and pink shrimp were selected based on their economic importance to Oregon’s commercial fisheries, their historical and cultural significance to indigenous cultures and other people in Oregon, and their variability in trophic position and feeding modes (Table 1). We examined AP contamination in individuals harvested from Oregon coastal waters, assessing differences between those obtained directly after being caught on National Oceanic and Atmospheric Administration (NOAA) or Oregon Department of Fish and Wildlife (ODFW) vessels and those caught on commercial vessels but purchased at retail markets to understand AP contamination entry. We hypothesized higher AP concentrations in riverine than oceanic stages of lamprey and in retail-purchased rather than vessel-caught individuals due to their increased exposure to plastic during seafood processing. We also predicted higher concentrations of APs in lower trophic level organisms based on the existing literature (Walkinshaw et al., 2020a).
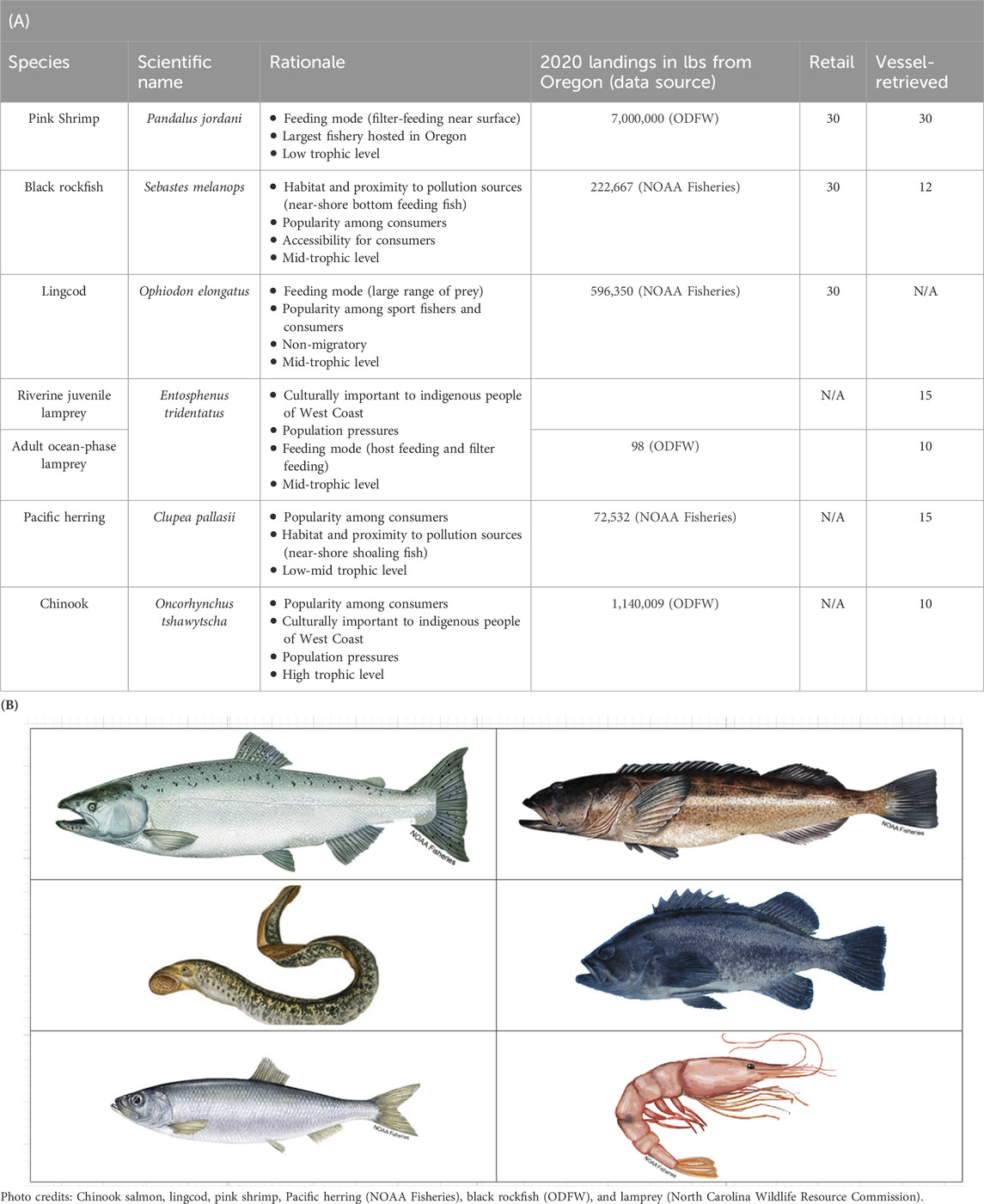
Table 1. (Panel A) Species names (common and scientific), rationale for sampling, 2020 commercial landings in Oregon, and number of individuals collected per species by source. One individual equated to one sample. (Panel B) Study species clockwise from top left: Chinook salmon (Oncorhynchus tshawytscha), lingcod (Ophiodon elongatus), black rockfish (Sebastes melanops), pink shrimp (Pandalus jordani), Pacific lamprey (Entosphenus tridentatus), and Pacific herring (Clupea pallasii).
2 Materials and methods
2.1 Sample collection
Five finfish and one shellfish species (Table 1) were collected from one or two sources: vessel-retrieved or/and retail-purchased from Oregon waters (see Supplementary Appendix SA1); the numbers from each source, categorized by species, are provided in Table 1. “Vessel-retrieved individuals” were whole-body fish or crustaceans caught by a fishing vessel in Oregon waters, the majority by the National Oceanic and Atmospheric Administration’s Northwest Fisheries Science Center Observers program during the 2021–2022 collection season, with the exception of riverine and ocean-phase Pacific lamprey that were collected during the 2017–2018 season (due to constraints imposed by the COVID-19 pandemic). Retail-purchased individuals were either fish fillets (finfish) or gutted shrimp purchased from a supermarket or seafood vendor. For one finfish species, lingcod, and the crustacean species, pink shrimp, samples were acquired from both fishing vessels (NOAA) and retail market (seafood counters at grocery stores), the source most accessible to the general public, and analyzed. Pacific lamprey species, listed on Oregon’s threatened and endangered species list, were acquired from ODFW and collected under permit.
Vessel-retrieved individuals were humanely euthanized by placing them in ice water baths, then double-wrapped in aluminum foil, and placed whole in plastic bags (IACUC-37, 2022). These individuals were kept frozen during transport to the Applied Coastal Ecology (ACE) Laboratory at Portland State University. Retail-purchased individuals (fillets and shelled shrimp) were purchased and transported to the ACE Laboratory as packaged by the retail store for a typical consumer. The packaging material included plastic-lined butcher paper, plastic takeout containers, and plastic freezer bags.
2.2 Sample processing
A total of 182 samples (122 finfish and 60 crustaceans) were collected from the Oregon coast and Oregon retail markets in 2021, while Pacific lamprey samples, collected in 2017, were frozen for later analysis.
For vessel-retrieved individuals, biological measurements, including full body length (in) and weight (g), and, if available, muscle tissue length (in) and weight (g) were recorded for all individuals. Whole-body individuals were dissected from behind the pectoral fin to the caudal fin to extract a filet of tissue corresponding to the parts of the animal typically consumed by humans.
For vessel-retrieved lingcod and Chinook salmon, approximately 220 g of muscle tissue (but ranging from 71–702 g) were randomly dissected from the front, middle, and end of the muscle tissue; for retail-purchased black rockfish and lingcod, filets were rinsed and then 32–133 (mean = 88) g of tissue were randomly dissected from the fillet. For shrimp (vessel and retail) and herring, individuals were rinsed and gutted; then all muscle tissue was dissected as all individuals sampled (75) were under 125 g (range 0.1–28 g). On average, vessel pink shrimp were 2 g (0.18–5.4 g), retail pink shrimp were 3.7 g (0.16–28 g), and vessel pacific herring were 10.77 g (3.5–19). For lamprey, riverine juveniles were defined and headed, and a small amount of muscle tissue was digested; for ocean-phase adult lamprey, we received and digested sections of muscle tissue (range 3–21 g) (see Supplementary Appendix Table SA1).
Dissected tissue from each individual was placed in its own 250 mL beaker and covered with a watch glass. Each sample was digested using a 10% potassium hydroxide (KOH) solution heated for 24–48 h at 40 C (except lamprey = 60 C), as outlined by Baechler et al. (2020), although density separation was not needed. A second digestion was needed for all lamprey samples. After digestion, the samples were vacuum-filtered through a 20-micron brass sieve (Hogentogler) to collect APs and remove the remaining liquefied tissue. The samples were then rinsed from the sieve into a vacuum filtration apparatus (Millipore Sigma) using a 47 mm diameter x 10-micron polycarbonate filter (10 μm, Millipore Sigma). Filters were then enclosed in PetriSlides (47 mm, Millipore Sigma).
2.3 Anthropogenic particle enumeration
Filters were examined to enumerate and measure APs using a Leica ICC50 HD with LAS V4.13 software and a ZEISS Primostar 3 with Labscope v3.3 software. Filters were examined under ×40 and ×10 magnification (depending on AP size) and photographed. Suspected APs were counted following the method outlined by Lusher et al. (2020) and classified based on their morphology, color, maximum width, and maximum length. Fiber bundles were separated into individual fibers when possible and classified accordingly. When fiber bundles could not be separated, the ends of fibers were used to count the total number of fibers in the bundle.
2.4 Particle characterization
A subsample of suspected plastics (n = 209, 10% of total suspected APs encountered) was sent to the Ecotox and Environmental Stress Laboratory at Oregon State University to undergo micro-Fourier transform infrared (μFTIR) spectroscopy analysis to identify specific polymers and validate total counts. Six to fifteen suspected APs (depending on the number of individuals per species) were randomly selected from each species group and analyzed via FTIR spectroscopy. OpenSpecy (Cowger et al., 2021) was used to calibrate and confirm sample material types, following methods described by Caldwell et al. (2022), Talbot et al. (2022), and Lasdin et al. (2023).
2.5 QA/QC
Quality control protocols were adapted from Baechler et al. (2020) and Brander et al. (2020). Pink 100% cotton laboratory coats and facemasks were used during all processing steps. Pink material was chosen to easily identify AP input from researchers in samples and controls. In addition, 100% cotton clothing was worn at all times throughout sample processing. All containers, glassware, sieves, and beakers were triple-rinsed with DI water, inverted, covered with aluminum foil, and then air-dried to minimize paper towel fibers on glass surfaces. Three DI water procedural blanks were run in conjunction with each species batch (a total of 18 blanks; 3 per every 30 samples processed) and subjected to the same digestion, sieving, and filtering protocols. Air control blanks (total of 18, three for every species batch regardless of batch size), consisting of a clean polycarbonate filter inside a clean PetriSlide, followed each species batch through the process. A 1:1 sample-to-blank ratio was used to quantify APs entering samples during microscopy. Additionally, a snorkel hood was positioned over the sample on the microscope to minimize airborne contamination.
2.6 Statistical analysis
Using R-studio (version 4.1.2.) statistical software, analysis of variance (ANOVA) (alpha = 0.05) was performed to test for differences in AP load among species. A Tukey honestly significant difference post hoc test was used to confirm significant findings. ANOVA and Welch’s two-sample t-tests were performed to test for differences between source types of the same species (pink shrimp, lingcod, and Pacific lamprey). Spearman correlation coefficients were used to evaluate relationships between the total body weight or total filet weight and overall AP tissue burden. Plots were generated using the ggplot2 and vegan packages in R.
3 Results
3.1 Anthropogenic particle characteristics
In organisms: Through microscope search, 1,806 suspected APs were identified across 180 of 182 individuals (averages varied drastically among species; Table 2). Fibers (1,466; 81.17%) were the most abundant, followed by fragments (332; 18.38%) and films (8; 0.44%) (see Supplementary Appendix Figure SA3B). The most common colors were blue (234; 12.95%), black (234; 12.95%), and clear or white (1,297; 71.81%) (see Supplementary Appendix Figure SA3C). The maximum length of APs ranged from 2.00–3,619 μm (mean = 911.78 μm ± 633.01), and the maximum width ranged from 0.477–1757.5 μm (mean = 26.56 μm ± 71.35) (Table 3; Supplementary Appendix Figure SA1).
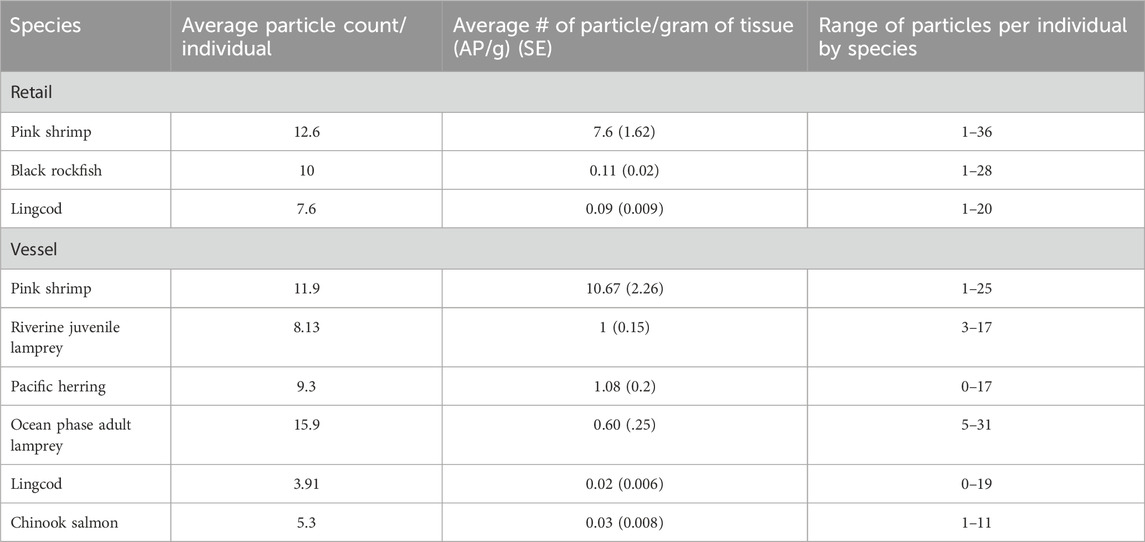
Table 2. Mean particle count and mean number of particles per gram of tissue per individual (with standard error in parentheses) and the range of particles found across individuals by species.
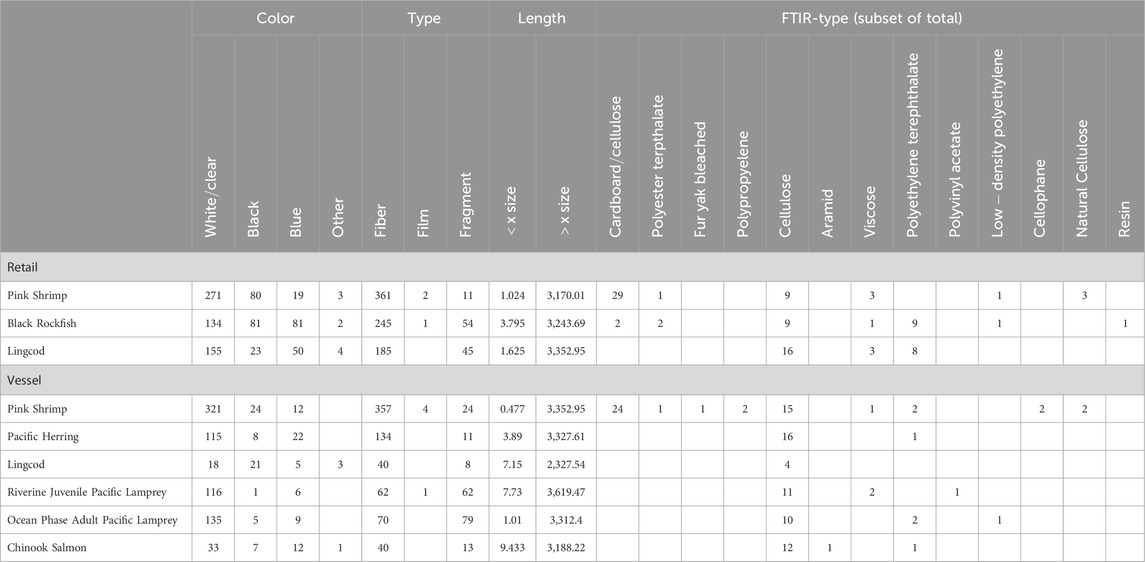
Table 3. Breakdown of material color, shape, length, and material categories identified via FTIR across species and sample collection types.
In controls: Through visual search, a total of 190 suspected APs were identified in procedural, air, and microscopy controls (see Table 4 and Supplementary Appendix Table SA2 for additional details). Fibers (160; 84.21%) were the most abundant shape found, followed by fragments (25, 13.15%) (Supplementary Appendix Figure SA3A). The most common colors were clear (132; 69.74%), blue (26; 13.68%), and black (32; 16.84%). The maximum length of all APs found in controls ranged from 29.75 to 2,969.18 μm (mean of 744.05 μm ± 515.64), and the maximum width ranged from 3.59 to 367.33 μm (mean of 29.91 μm ± 44.12) (Supplementary Appendix Figure SA1).
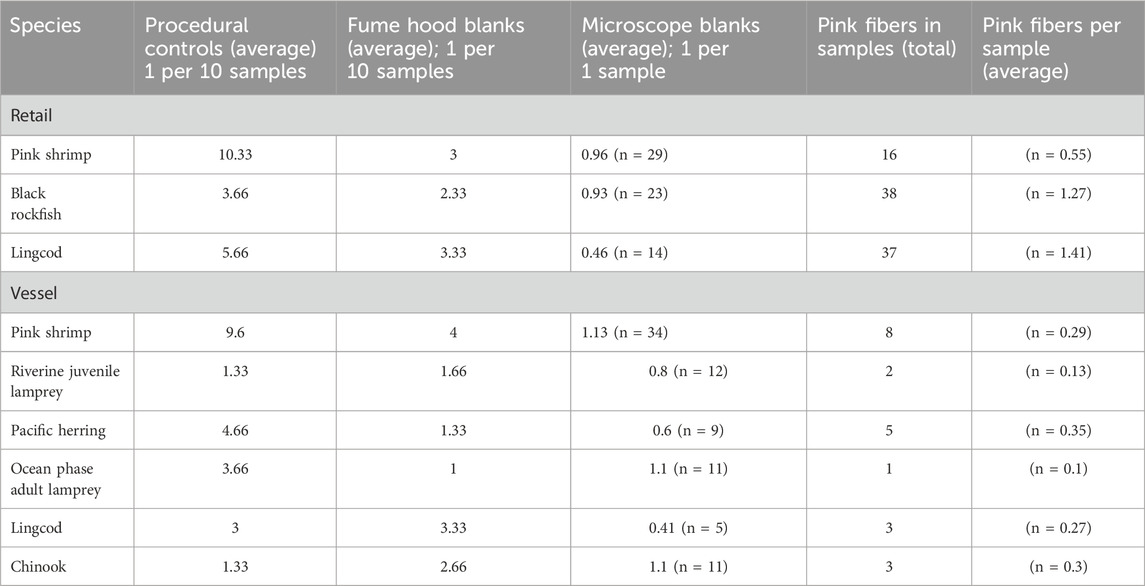
Table 4. APs in procedural controls, fume hood blanks, and microscope blanks, including number of pink fibers found in samples by species. Pink fibers were excluded from AP sample counts as they were presumed contamination from researcher clothing.
3.2 Anthropogenic particles in finfish and shellfish
APs were found in the muscle tissue of all species of finfish and shellfish sampled; of the 182 individuals sampled, only two individuals (1%) had no APs in the section of tissue sampled (one vessel-retrieved lingcod and one vessel-retrieved herring). Among the species sampled, pink shrimp contained the most APs per individual, regardless of source type (retail: 25 (12.6 ± 1.67) per individual; vessel: 36 (11.9 ± 1.22) per individual) (Figure 1), with the most particles (36) found in a single pink shrimp weighing 4.9 g (7.35 AP/g of tissue; Tables 2, 3). Vessel-retrieved Chinook contained the smallest abundance and concentration of APs (1–11 per individual and 0.028 AP/g; Table 3). AP ranges by species and source type varied (Figure 1; Table 3). Muscle tissue weight and AP burden were inversely correlated (Spearman rank = −0.23), indicating that smaller individuals are more likely to contain APs (ANOVA: f = 9.2 and p = 0.0028). Biological measurements were not obtainable for retail-purchased individuals.
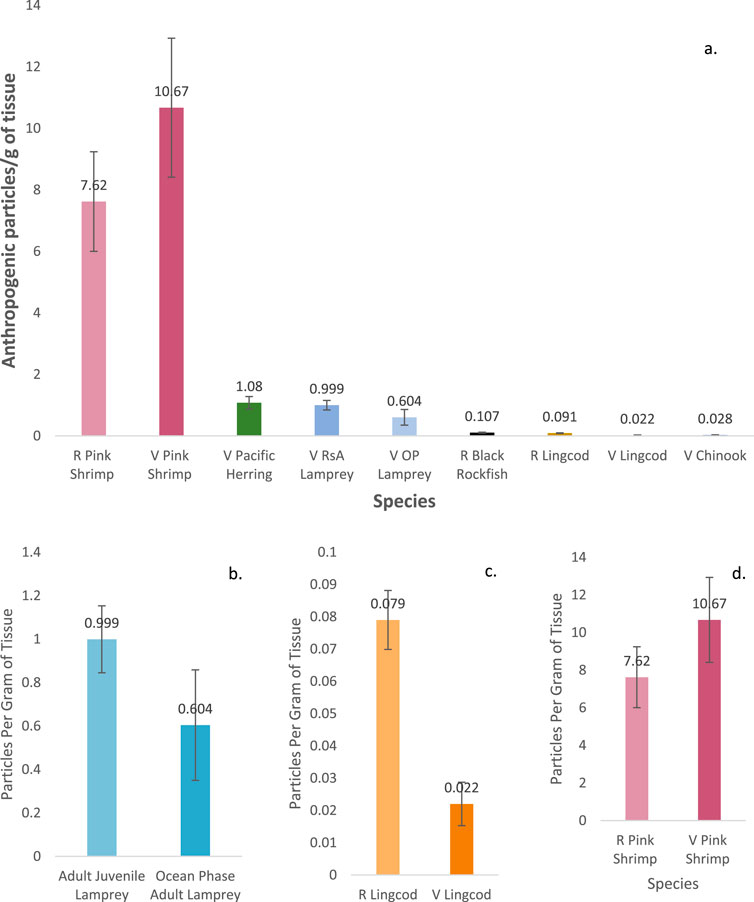
Figure 1. Average number (and standard error) of particles per gram of tissue found (A) across all species, ranked by trophic position and source type; (B) between riverine juvenile and ocean adult lamprey; (C) retail and vessel obtained lingcod; and (D) retail and vessel pink shrimp.
3.3 Retail-purchased and vessel-retrieved comparison
Differences in average AP/g of tissue were inconsistent between retail and vessel-caught individuals across species (lingcod and pink shrimp). Retail lingcod contained more AP/individual and more APs/g of tissue (7.33 AP/individual, 0.091 AP/g) than vessel-retrieved lingcod (3.91 AP/individual, 0.022 AP/g; Welch’s t-test for AP/g tissue: t = −5.1, p = 8.79–5) (Figure 1). However, retail pink shrimp contained slightly more APs/individual but fewer APs/g of tissue (12.6 AP/individual, 7.62 AP/g) than vessel-retrieved pink shrimp (11.9 AP/individual, 10.67 AP/g; Welch’s t-test for AP/g tissue: t = −1.2, p = 0.227) although the difference was not significant. An individual retail pink shrimp contained the most particles across all species and source types in a single individual (36 particles) (Figures 1C, D).
Comparing late-stage riverine juveniles and ocean phase-adult Pacific lamprey, the adults had marginally higher AP loads (15.9 versus 8.13 particles per individual) but marginally lower concentrations (0.6 AP/g versus 0.99 AP/g; Welch’s t-test for AP/g tissue: t = −1.32, p = 0.08) than juveniles (Figure 1B).
3.4 FTIR results
Of the 270 (∼10%) suspected APs tested using FTIR, 230 suspected APs were from individuals and 40 were from controls (detailed FTIR results in Supplementary Appendix Table SA3). OpenSpecy (Cowger et al., 2021) was used to calibrate and confirm sample material types according to methods described by Caldwell et al. (2022), Talbot et al. (2022), and Lasdin et al. (2023). In addition, 17.06% of suspected APs were fully synthetic materials, 9.47% were semi-synthetic, 8.05% were natural materials, and the overwhelming majority, 65.40%, were identified as anthropogenically modified (Supplementary Appendix Figures SA3A, SA3C). Synthetic and semi-synthetic material types included polyethylene terephthalate (PET; n = 33), polypropylene (PP; n = 3), high-density polyethene (HDPE; n = 1), low-density polyethylene (LDPE; n = 4), polyethylene vinyl acetate (PEVA; n = 1), fiberglass (n = 16), and semi-synthetic cardboard (n = 22). There was a single aramid fiber, a common material used in marine rope, flame-retardant fabrics, and military applications (Gong and Chen, 2016). Cellulose (n = 52), cotton fiber (n = 41), and cellulose acetate filter (n = 55) were the most common anthropogenically modified particles found.
3.5 Quality control
AP contamination in procedural controls (average: 4.80 particles), fume hood blanks (average: 2.51 particles), and microscope blanks (0.82 particles) (Table 4) was averaged for each species batch, as per previous studies (Li et al., 2015; Rochman et al., 2015; Abbasi et al., 2018), and reported in Table 4 to provide an estimate of total contamination at each sample processing step. Pink MF contamination from laboratory clothing ranged from 0.41 to 1.25 particles per sample and was excluded from all counts. Supplementary Appendix Table SA1 details AP contamination across individuals.
4 Discussion
4.1 Anthropogenic contamination characteristics
The array of AP types and colors found across the six taxa highlights the complexity of identifying AP pollution sources in aquatic environments. Li et al. (2021) described using “microplastic communities”—APs of various colors, shapes, and polymer types that accumulate together in the environment—to elicit a potential number of AP pollution sources (Li et al., 2021). Of the published studies on APs in muscle tissue that share size categories, translocation into the muscle tissue is facilitated by shape (most frequently fibers) and size although this study found slightly larger fibers and fragments than those in fish muscle tissue of other studies (Barboza et al., 2020; McIlwraith et al., 2021). This may be due to differences in protocols, AP presence in the environment at each study area, or other factors not measured in these studies. While some organisms have a tendency to ingest APs of certain colors, shapes, and sizes (Q. Chen et al., 2020; Okamoto et al., 2022), further research is needed to understand how these variables affect translocation into the muscle tissue and toxicity to organisms (Mehito et al., 2022). A recent synthesis indicated that particles <80 μm could translocate in aquatic organisms (Mehinto et al., 2022), and given that the diameter of the typical microfiber is 10–15 μm, indications are that these particles could translocate if in the proper orientation.
Of the 182 individuals sampled, only two (one vessel-retrieved Pacific herring and one vessel-retrieved lingcod) had no detected APs in their tissue although these were smaller samples 0.18–19 g of muscle (Lingcod) or full tissue (herring) sampled. The study results mirror those of Akoueson et al. (2020) and Barboza et al. (2020) and provide evidence of the widespread presence of APs in the edible tissues of Oregon’s marine and freshwater species across trophic levels and feeding modes. Pink shrimp, which filter-feed in the upper water column which contains 8–9200 AP particles/m3, had the highest concentrations of APs (ODFW, 2019; Curren et al., 2020; Dawson et al., 2021; Yang et al., 2021). On the other hand, Chinook salmon had the lowest concentrations, followed by black rockfish and lingcod.
Across different life stages of lamprey, we hypothesized riverine-phase juvenile lamprey, which follow the filter-feeding phase, would contain more APs than the parasitic-feeding ocean-phase adult lamprey; on an AP/g of fish basis, this hypothesis proved true, with ocean-phase adults containing 0.604 AP/g compared to riverine juveniles with 0.999 AP/g; however, since adult lamprey are larger than juveniles, they had a higher total number of APs per individual. As adults attach to a host with their mouthparts and feed primarily on the bodily fluids of host organisms (Goodman and Reid, 2017), they may inherit a portion of their APs from the bloodstream of the host organisms they feed on.
This study aligns with other studies in that species across various trophic levels are exposed to AP pollution (Talley et al., 2020; F; Wang et al., 2021; Yıldız et al., 2022) and uptake and translocate particles into their tissue (e.g., Akhbarizadeh et al., 2019; Bagheri et al., 2020; Hossain et al., 2023; Nan et al., 2020; Figure 2, Supplementary Appendix Table SA2 and references therein). Furthermore, as evident from comparisons with other studies (Figure 2), we found evidence of an inverse relationship between muscle tissue AP concentration and trophic level, indicating a potential relationship between AP presence and habitat or feeding mode (see Figure 1A). Similar conclusions have been drawn by others studying trophic transfer and habitat depth (Aiguo et al., 2022; Carbery et al., 2018) and in comparisons of shellfish muscle tissue to that of higher trophic level finfish (Walkinshaw et al., 2020a).
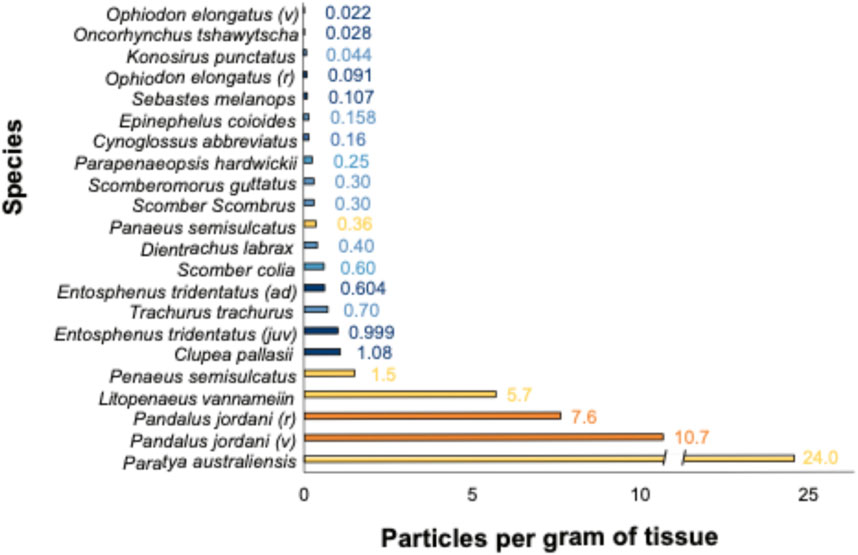
Figure 2. Comparison of microplastics per gram of edible shrimp (dark orange) and fish (dark blue) tissue from this study (see Table 1 for common names) compared with shrimp (orange) and fish (blue) from other studies around the world. v, vessel caught; r, retail; juv, juvenile; ad, adult. See Supplementary Appendix Table SA4 for sample collection locations and data sources.
Our study bolsters existing work on the AP muscle tissue presence, but further studies are needed to understand the mechanisms by which APs translocate into muscle tissue. Barboza et al. (2020) hypothesized that APs may transit through the bloodstream and into the muscle tissue (Barboza et al., 2020). Others hypothesize that macrophages may scavenge particles, leading to immune response and inflammation, which may facilitate translocation into the muscle tissue via cells (Leslie et al., 2022; Beijer et al., 2022).
4.2 Retail-purchased versus vessel-retrieved
Although other studies have found APs (MPs in the literature referenced) in retail market seafood products (Ferrante et al., 2022; Nalbone et al., 2021; Thiele et al., 2021), our results raise questions about the extent to which the retail process is a source. Our source type comparisons indicate ambiguity in retail processing as a source: AP concentrations were greater for retail than vessel-retrieved lingcod (after rinsing the surface flesh of fillets) but lower for retail than vessel-retrieved pink shrimp (after rinsing). Our results suggest that, in some cases, retail market individuals may be exposed to additional APs through processing, resulting in the incorporation of additional particles into the edible portions of seafood items. These post-mortem APs could be introduced by plastic packaging meant to preserve seafood (Dawson et al., 2021; Habib et al., 2022; Jadhav et al., 2021; Kedzierski et al., 2020). It is unclear why the retail process did not add microplastics to lingcod, as was observed for pink shrimp, indicating a need for further investigation to understand where and when AP contamination occurs post-catch.
4.3 Study limitations
Our sample size for the larger finfish was small, limiting the generalizability of the findings for these species. Additionally, since the study focused on species that span the US West Coast but only collected organisms from the Oregon coast, the concentrations may not represent coast-wide microplastic concentrations. However, a comparison with other studies on muscle tissue microplastic concentrations demonstrates that our results fall within the range of microplastics per gram of edible fish tissue found globally (Figure 2; Supplementary Appendix Table SA4). Future research should consider collecting the same species at various points along the West Coast to determine whether there is spatial variability in microplastic contamination from northern Washington to Southern California, particularly for the species that span the entire coast.
5 Implications for Oregon’s seafood producers, consumers, and threatened species
For producers and handlers of seafood, we recommend shifting to alternative packaging methods such as natural materials made from beeswax, starches, or sugars that will limit the introduction of APs into retail seafood (Chen et al., 2022; Herrmann et al., 2022; Rangaraj et al., 2021). Research and development may be needed to provide economically viable alternative products that perform similarly to plastic (Hurst-Mayr et al., in prep). For consumers, we recommend buying whole, local fish whenever possible to minimize APs introduced via plastic packaging. Regardless of the source of seafood products, individuals containing APs were found to have at least 0.3 AP/10 g of edible tissue, signaling the need for policy and other interventions to regulate APs.
Since the species we sampled are consumed by both humans and marine predators, there is a potential for biomagnification (see Torres et al., 2023). The presence of APs in edible tissues and the possibility that they are translocated from the gut or gills highlight the need for further research into the health effects of AP consumption for both aquatic organisms and humans. To date, the majority of studies identifying health effects of AP consumption by aquatic organisms, e.g., adverse cellular responses, inflammation, oxidative stress, negative impacts on growth and development, physical damage to organs, behavioral changes, adverse reproductive responses, and decreased survivorship (Lanctôt et al., 2020; Mallik et al., 2021; Qiao et al., 2019; Sharifinia et al., 2020; Solomando et al., 2020; Trestrail et al., 2021; Siddiqui et al., 2022), have focused on model species, which may under-represent the effects on wild species or did not use environmentally relevant AP concentrations, which may mis/over-represent effects.
In Oregon and the Western U.S., Pacific lamprey are listed as endangered at the state level, affected by artificial barriers to migration, poor water quality, loss of habitat, and changing ocean conditions. Moreover, Pacific lamprey are a culturally important food source for indigenous peoples of the Pacific Northwest, so consumption of Pacific lamprey is a source of AP exposure for these communities specifically (Monroe, 2013). Although APs have been found in a variety of food items across trophic levels, people who depend upon subsistence fisheries are likely ingesting fish-borne contaminants, highlighting the environmental justice issue perpetuated by AP pollution in Pacific lamprey (National Environmental Justice Advisory & Council Fish Consumption and Environmental Justice, 2002). Further research is needed to understand the baseline exposure of indigenous communities from lamprey consumption, how lamprey internalize APs relative to other seafood species, how APs may impact this already endangered species in Oregon, and how to reduce lamprey exposure. Due to the historical and systemic oppression of indigenous tribes in the U.S., the state of Oregon has a responsibility to employ larger-scale AP regulation methods to minimize exposure to AP pollution through Pacific lamprey consumption (Duffield et al., 2021). While Walkinshaw et al. (2020a) highlighted that seafood most likely does not contribute more APs than consuming other foods or drinking water, the high consumption of seafood by low income and indigenous peoples and the continued prevalence of APs detected in humans (Ibrahim et al., 2021; Jenner et al., 2022; Leslie et al., 2022; Ragusa et al., 2021) necessitates further study of the long-term exposure effects on human health (Lusher et al., 2017; Walkinshaw et al., 2020b; Coffin et al., 2022).
6 Implications and next steps for policymakers and researchers
Although the percentage of validated APs is only 10%, the detection of APs across all six taxa spanning multiple trophic levels and two sources confirms the need for actions to address aquatic exposures. Four potential pathways for policymakers and researchers to address APs include mitigation technology, monitoring, long-term research, and legislation.
Current AP pollution loads in the environment have reached a level irreversible by current technologies (Uzun et al., 2022). Therefore, addressing AP pollution requires mitigative approaches to reduce the flow of AP pollution into the environment or “turning off the tap” on virgin plastic production (Bergmann et al., 2022). A newer mitigative technology is laundry machine MF catchment filters. Widespread implementation of this mitigative technology or, at the very least, selective use at high-emission sites, would reduce MF pollution entering waterways (Erdle et al., 2021).
Government agencies should follow California’s lead and pursue ambient monitoring of AP in drinking water, air, and waterways (California S.B. 1,422, 2018) to inform large-scale regional policy to effectively control AP pollution. Long-term and multi-generational research studies on organismal health following exposure to AP pollution are also needed. Both anthropogenically modified and synthetic/semi-synthetic materials should be tested as the abundance of both continues to grow in the environment (Siddiqui et al., 2022; Walkinshaw et al., 2023). Ambient monitoring along the West Coast would inform concentrations for such long-term studies. Finally, the Western states should work collaboratively to create a standardized monitoring approach and, ultimately, coordinate regulatory policy for AP pollution. However, since AP environmental pollution is no longer the problem of one country or government, global policies such as the ongoing global plastics treaty negotiations and enforcement of an eventual treaty are ultimately needed to address the problem of AP pollution at its current magnitude. Unless we change our relationship with plastic and significantly reduce plastic production, we will continue to witness its negative impacts.
Data availability statement
The original contributions presented in the study are included in the article/Supplementary Material; further inquiries can be directed to the corresponding author.
Ethics statement
Ethical approval was not required for the study involving animals in accordance with the local legislation and institutional requirements because the animals were already deceased prior to our acquisition of the animals.
Author contributions
ST: formal analysis, investigation, and writing–original draft. EG: conceptualization, funding acquisition, methodology, project administration, supervision, and writing–review and editing. MD: investigation and writing–review and editing. SB: resources, validation, and writing–review and editing.
Funding
The author(s) declare that financial support was received for the research, authorship, and/or publication of this article. Funding for this research was provided by Oregon Sea Grant through a SEED grant (to EG) and an Edward D. and Olive C. Bushby Scholarship (to ST).
Acknowledgments
The authors thank Dr. Garth Covington for assistance with scope protocol modifications and equipment troubleshooting, Emily Pederson at Oregon State University, Steve Rumrill for expertise on Oregon fisheries, and Britta Baechler and Sarah Carvill for assistance in project design; they also thank the Oregon Sea Grant SEED Science Advisory Panel for input and guidance on the project; NOAA Northwest Fisheries Science Center and the Ocean Phase Lamprey Technical Working Group of Oregon Department of Fish and Wildlife for assistance with procuring samples. The authors also thank numerous undergraduate students, Joshua Fackrell, Kylee Brevick, and Micheal Noonan for assistance with this project.
Conflict of interest
The authors declare that the research was conducted in the absence of any commercial or financial relationships that could be construed as a potential conflict of interest.
Publisher’s note
All claims expressed in this article are solely those of the authors and do not necessarily represent those of their affiliated organizations, or those of the publisher, the editors, and the reviewers. Any product that may be evaluated in this article, or claim that may be made by its manufacturer, is not guaranteed or endorsed by the publisher.
Supplementary material
The Supplementary Material for this article can be found online at: https://www.frontiersin.org/articles/10.3389/ftox.2024.1469995/full#supplementary-material
References
Abbasi, S., Soltani, N., Keshavarzi, B., Moore, F., Turner, A., and Hassanaghaei, M. (2018). Microplastics in different tissues of fish and prawn from the Musa Estuary, Persian Gulf. Chemosphere 205, 80–87. doi:10.1016/j.chemosphere.2018.04.076
Adams, J. K., Dean, B. Y., Athey, S. N., Jantunen, L. M., Bernstein, S., Stern, G., et al. (2021). Anthropogenic particles (including microfibers and microplastics) in marine sediments of the Canadian Arctic. Sci. Total Environ. 784, 147155. doi:10.1016/j.scitotenv.2021.147155
Aiguo, Z., Di, S., Chong, W., Yuliang, C., Shaolin, X., Peiqin, L., et al. (2022). Characteristics and differences of microplastics ingestion for farmed fish with different water depths, feeding habits and diets. J. Environ. Chem. Eng. 10 (2), 107189. doi:10.1016/j.jece.2022.107189
Akhbarizadeh, R., Moore, F., and Keshavarzi, B. (2019). Investigating microplastics bioaccumulation and biomagnification in seafood from the Persian Gulf: a threat to human health? Food Addit. and Contam. Part A 36 (11), 1696–1708. doi:10.1080/19440049.2019.1649473
Akoueson, F., Sheldon, L. M., Danopoulos, E., Morris, S., Hotten, J., Chapman, E., et al. (2020). A preliminary analysis of microplastics in edible versus non-edible tissues from seafood samples. Environ. Pollut. 263, 114452. doi:10.1016/j.envpol.2020.114452
Athey, S. N., and Erdle, L. M. (2022). Are we underestimating anthropogenic microfiber pollution? A critical review of occurrence, methods, and reporting, 2024 pollution? A critical review of occurrence, methods, and reporting. Environ. Toxicol. Chem. 41 (4), 822–837. doi:10.1002/etc.5173
Baechler, B. R., Granek, E. F., Hunter, M. V., and Conn, K. E. (2020). Microplastic concentrations in two Oregon bivalve species: spatial, temporal, and species variability. Limnol. Oceanogr. Lett. 5 (1), 54–65. doi:10.1002/lol2.10124
Baechler, B. R., Stienbarger, C. D., Horn, D. A., Joseph, J., Taylor, A. R., Granek, E. F., et al. (2019). Microplastic occurrence and effects in commercially harvested North American finfish and shellfish: current knowledge and future directions. Limnology and Oceanography Letters. Limnol. Oceanogr. Lett. 5, 113–136. doi:10.1002/lol2.10122
Bagheri, T., Gholizadeh, M., Abarghouei, S., Zakeri, M., Hedayati, A., Rabaniha, M., et al. (2020). Microplastics distribution, abundance and composition in sediment, fishes and benthic organisms of the Gorgan Bay, Caspian sea. Chemosphere 257, 127201. doi:10.1016/j.chemosphere.2020.127201
Barboza, L. G. A., Dick Vethaak, A., Lavorante, B. R. B. O., Lundebye, A.-K., and Guilhermino, L. (2018). Marine microplastic debris: an emerging issue for food security, food safety and human health. Mar. Pollut. Bull. 133, 336–348. doi:10.1016/j.marpolbul.2018.05.047
Barboza, L. G. A., Lopes, C., Oliveira, P., Bessa, F., Otero, V., Henriques, B., et al. (2020). Microplastics in wild fish from North East Atlantic Ocean and its potential for causing neurotoxic effects, lipid oxidative damage, and human health risks associated with ingestion exposure East Atlantic Ocean and its potential for causing neurotoxic effects, lipid oxidative damage, and human health risks associated with ingestion exposure. Sci. Total Environ., 717, 134625. doi:10.1016/j.scitotenv.2019.134625
Beijer, N. R. M., Dehaut, A., Carlier, M. P., Wolter, H., Versteegen, R. M., Pennings, J. L. A., et al. (2022). Relationship between particle properties and immunotoxicological effects of environmentally-sourced microplastics. Front. Water 4, 866732. doi:10.3389/frwa.2022.866732
Bergmann, M., Carney Almroth, B., Brander, S. M., Dey, T., Green, D. S., Gundogdu, S., et al. (2022). A global plastic treaty must cap production. Science 376 (6592), 469–470. doi:10.1126/science.abq0082
Brahney, J., Hallerud, M., Heim, E., Hahnenberger, M., and Sukumaran, S. (2020). Plastic rain in protected areas of the United States. Science 368 (6496), 1257–1260. doi:10.1126/science.aaz5819
Brander, S. M., Renick, V. C., Foley, M. M., Steele, C., Woo, M., Lusher, A., et al. (2020). Sampling and quality assurance and quality control: a guide for scientists investigating the occurrence of microplastics across matrices. Appl. Spectrosc. 74 (9 (September 1), 1099–1125. doi:10.1177/0003702820945713
Caldwell, A., Brander, S., Wiedenmann, J., Clucas, G., and Craig, E. (2022). Incidence of microplastic fiber ingestion by common terns (Sterna hirundo) and roseate terns (S. Dougallii) breeding in the northwestern atlantic. Mar. Pollut. Bull. 177, 113560. doi:10.1016/j.marpolbul.2022.113560
California S.B. 1422 (n.d.). California safe drinking water act: microplastics. Available at: https://leginfo.legislature.ca.gov/faces/billTextClient.xhtml?bill_id=201720180SB1422 (Accessed July 5, 2021)
Carbery, M., O’Connor, W., and Palanisami, T. (2018). Trophic transfer of microplastics and mixed contaminants in the marine food web and implications for human health. Environ. Int. 115, 400–409. doi:10.1016/j.envint.2018.03.007
Chen, J., Zheng, M., Tan, K. B., Lin, J., Chen, M., and Zhu, Y. (2022). Polyvinyl alcohol/xanthan gum composite film with excellent food packaging, storage and biodegradation capability as potential environmentally-friendly alternative to commercial plastic bag. Int. J. Biol. Macromol. 212, 402–411. doi:10.1016/j.ijbiomac.2022.05.119
Chen, Q., Li, Y., and Li, B. (2020). Is color a matter of concern during microplastic exposure to Scenedesmus obliquus and Daphnia magna? J. Hazard. Mater. 383, 121224. doi:10.1016/j.jhazmat.2019.121224
Coffin, S., Bouwmeester, H., Brander, S., Damdimopoulou, P., Gouin, T., Hermabessiere, L., et al. (2022). Development and application of a health-based framework for informing regulatory action in relation to exposure of microplastic particles in California drinking water. Microplastics Nanoplastics 2, 12. doi:10.1186/s43591-022-00030-6
Cole, M., Lindeque, P., Halsband, C., and Galloway, T. S. (2011). Microplastics as contaminants in the marine environment: a review. Mar. Pollut. Bull. 62 (12), 2588–2597. doi:10.1016/j.marpolbul.2011.09.025
Cowger, W., Steinmetz, Z., Gray, A., Munno, K., Lynch, J., Hapich, H., et al. (2021). Microplastic spectral classification needs an open source community: open specy to the rescue! Open source community: open specy to the rescue!. Anal. Chem. 93 (21), 7543–7548. doi:10.1021/acs.analchem.1c00123
Crawford, C. B., and Quinn, B. (2017). “3—plastic production, waste and legislation,” in Microplastic pollutants. Editors C. B. Crawford,, and B. Quinn (Elsevier), 39–56. doi:10.1016/B978-0-12-809406-8.00003-7
Curren, E., Leaw, C. P., Lim, P. T., and Leong, S. C. Y. (2020). Evidence of marine microplastics in commercially harvested seafood. Front. Bioeng. Biotechnol. 8, 562760. doi:10.3389/fbioe.2020.562760
Currie, J. J., Stack, S. H., McCordic, J. A., and Kaufman, G. D. (2017). Quantifying the risk that marine debris poses to cetaceans in coastal waters of the 4-island region of Maui. Mar. Pollut. Bull. 121 (1), 69–77. doi:10.1016/j.marpolbul.2017.05.031
Das, R. K., Sanyal, D., Kumar, P., Pulicharla, R., and Brar, S. K. (2021). Science-society-policy interface for microplastic and nanoplastic: environmental and biomedical aspects. Environ. Pollut. 290, 117985. doi:10.1016/j.envpol.2021.117985
Dawson, A. L., Santana, M. F. M., Miller, M. E., and Kroon, F. J. (2021). Relevance and reliability of evidence for microplastic contamination in seafood: a critical review using Australian consumption patterns as a case study Australian consumption patterns as a case study. Environ. Pollut. 276, 116684. doi:10.1016/j.envpol.2021.116684
Duffield, J., Neher, C., and Patterson, D. (2021). Estimating compensation ratios for tribal resources within a habitat equivalency framework. Ecol. Econ. 179, 106862. doi:10.1016/j.ecolecon.2020.106862
Erdle, L. M., Nouri Parto, D., Sweetnam, D., and Rochman, C. M. (2021). Washing machine filters reduce microfiber emissions: evidence from a community-scale pilot in Parry Sound, Ontario. Front. Mar. Sci. 1703. doi:10.3389/fmars.2021.777865
Ferrante, M., Pietro, Z., Allegui, C., Maria, F., Antonio, C., Pulvirenti, E., et al. (2022). Microplastics in fillets of Mediterranean seafood. A risk assessment study. Environ. Res. 204, 112247. doi:10.1016/j.envres.2021.112247
Galvão, A., Aleixo, M., De Pablo, H., Lopes, C., and Raimundo, J. (2020). Microplastics in wastewater: microfiber emissions from common household laundry. Environ. Sci. Pollut. Res. 27 (21), 26643–26649. doi:10.1007/s11356020-08765-6
Gao, P., Noor, N. Q. I. M., Razali, U. H. M., Yusop, M. H. M., and Shaarani, S. M. (2023). Anthropogenic particles in the muscle, gill, and gastrointestinal tract of marine fish sold for human consumption. Heliyon 9 (10), e20835. doi:10.1016/j.heliyon.2023.e20835
Gong, R. H., and Chen, X. (2016). “3—Technical yarns,” in Handbook of technical textiles. Editors A. R. Horrocks,, and S. C. Anand Second Edition (Cambridge, United Kingdom: Woodhead Publishing), 43–62. doi:10.1016/B978-1-78242-458-1.00003-0
Goodman, D. H., and Reid, S. B. (2017). Climbing above the competition: innovative approaches and recommendations for improving Pacific Lamprey passage at fishways. Ecol. Eng. 107, 224–232. doi:10.1016/j.ecoleng.2017.07.041
Goßmann, I., Halbach, M., and Scholz-Böttcher, B. M. (2021). Car and truck tire wear particles in complex environmental samples – a quantitative comparison with “traditional” microplastic polymer mass loads. Sci. Total Environ. 773, 145667. doi:10.1016/j.scitotenv.2021.145667
Granek, E. F., Brander, S. M., and Holland, E. B. (2020). Microplastics in aquatic organisms: improving understanding and identifying research directions for the next decade. Limnol. Oceanogr. Lett. 5 (1), 1–4. doi:10.1002/lol2.10145
Granek, E. F., Traylor, S. D., Tissot, A. G., Hurst, P. T., Wood, R. S., and Brander, S. M. (2022). “Clothes encounters of the microfibre kind: the effects of natural and synthetic textiles on organisms,” in Polluting Textiles. Editor J. Weis (London, England: Francis and Taylor).
Habib, R. Z., Poulose, V., Alsaidi, R., al Kendi, R., Iftikhar, S. H., Mourad, A.-H. I., et al. (2022). Plastic cutting boards as a source of microplastics in meat. Food Addit. and Contam. Part A 0 (0), 609–619. doi:10.1080/19440049.2021.2017002
Harte, M., Endreny, P., Sylvia, G., and Mann, H. M. (2008). Developing underutilized fisheries: Oregon’s developmental fisheries program. Mar. Policy 32 (4), 643–652. doi:10.1016/j.marpol.2007.11.005
Herrmann, C., Rhein, S., and Sträter, K. F. (2022). Consumers’ sustainability-related perception of and willingness-to-pay for food packaging alternatives. Resour. Conservation Recycl. 181, 106219. doi:10.1016/j.resconrec.2022.106219
Horton, A. A., Walton, A., Spurgeon, D. J., Lahive, E., and Svendsen, C. (2017). Microplastics in freshwater and terrestrial environments: evaluating the current understanding to identify the knowledge gaps and future research priorities. Sci. Total Environ. 586, 127–141. doi:10.1016/j.scitotenv.2017.01.190
Hossain, M. B., Pingki, F. H., Azad, M. A. S., Nur, A. A. U., Banik, P., Paray, B. A., et al. (2023). Microplastics in different tissues of a commonly consumed fish, Scomberomorus guttatus, from a large subtropical estuary: accumulation, characterization, and contamination assessment. Biology 12 (11), 1422. doi:10.3390/biology12111422
IACUC-37 (2022). Guidelines for euthanasia of research and teaching animals. Available at: https://research.ucdavis.edu/wp-content/uploads/IACUC-37.pdf.
Ibrahim, Y. S., Anuar, S. T., Azmi, A. A., Khalik, W. M. A. W. M., Lehata, S., Hamzah, S. R., et al. (2021). Detection of microplastics in human colectomy specimens. JGH Open 5 (1), 116–121. doi:10.1002/jgh3.12457
Jadhav, E. B., Sankhla, M. S., Bhat, R. A., and Bhagat, D. S. (2021). Microplastics from food packaging: an overview of human consumption, health threats, and alternative solutions. Environ. Nanotechnol. Monit. and Manag. 16, 100608. doi:10.1016/j.enmm.2021.100608
Jenner, L. C., Rotchell, J. M., Bennett, R. T., Cowen, M., Tentzeris, V., and Sadofsky, L. R. (2022). Detection of microplastics in human lung tissue using μFTIR spectroscopy. Sci. Total Environ. 831, 154907. doi:10.1016/j.scitotenv.2022.154907
Kapp, K. J., and Yeatman, E. (2018). Microplastic hotspots in the snake and lower columbia rivers: a journey from the greater yellowstone ecosystem to the pacific ocean. Environ. Pollut. 241, 1082–1090. doi:10.1016/j.envpol.2018.06.033
Kedzierski, M., Lechat, B., Sire, O., Le Maguer, G., Le Tilly, V., and Bruzaud, S. (2020). Microplastic contamination of packaged meat: occurrence and associated risks. Food Packag. Shelf Life 24, 100489. doi:10.1016/j.fpsl.2020.100489
Lanctôt, C. M., Bednarz, V. N., Melvin, S., Jacob, H., Oberhaensli, F., Swarzenski, P. W., et al. (2020). Physiological stress response of the scleractinian coral Stylophora pistillata exposed to polyethylene microplastics. Environ. Pollut. 263, 114559. doi:10.1016/j.envpol.2020.114559
Lasdin, K. S., Arnold, M., Agrawal, A., Fennie, H. W., Grorud-Colvert, K., Sponaugle, S., et al. (2023). Presence of microplastics and microparticles in Oregon Black Rockfish sampled near marine reserve areas. PeerJ 11, e14564.
Leslie, H. A. J. M., van Velzen, M., Brandsma, S. H., Vethaak, D., Garcia-Vallejo, J. J., and Lamoree, M. H. (2022). Discovery and quantification of plastic particle pollution in human blood. Environ. Int. 163, 107199. doi:10.1016/j.envint.2022.107199
Li, C., Gan, Y., Zhang, C., He, H., Fang, J., Wang, L., et al. (2021). “Microplastic communities” in different environments: differences, links, and role of diversity index in source analysis. Water Res. 188, 116574. doi:10.1016/j.watres.2020.116574
Li, J., Yang, D., Li, L., Jabeen, K., and Shi, H. (2015). Microplastics in commercial bivalves from China. Environmental pollution 207, 190–195.
Lusher, A., Hollman, P. C. H., and Mendoza-Hill, J. (2017). Microplastics in fisheries and aquaculture: status of knowledge on their occurrence and implications for aquatic organisms and food safety. Food Agric. Organ. U. N.
Lusher, A. L., Bråte, I. L. N., Munno, K., Hurley, R. R., and Welden, N. A. (2020). Is it or isn’t it: the importance of visual classification in microplastic characterization. Appl. Spectrosc. 74 (9), 1139–1153. doi:10.1177/0003702820930733
Mallik, A., Xavier, K. A. M., Naidu, B. C., and Nayak, B. B. (2021). Ecotoxicological and physiological risks of microplastics on fish and their possible mitigation measures. Sci. Total Environ. 779, 146433. doi:10.1016/j.scitotenv.2021.146433
Mattsson, K., da Silva, V. H., Deonarine, A., Louie, S. M., and Gondikas, A. (2021). Monitoring anthropogenic particles in the environment: recent developments and remaining challenges at the forefront of analytical methods. Curr. Opin. Colloid and Interface Sci. 56, 101513. doi:10.1016/j.cocis.2021.101513
McIlwraith, H. K., Kim, J., Helm, P., Bhavsar, S. P., Metzger, J. S., and Rochman, C. M. (2021). Evidence of microplastic translocation in wild-caught fish and implications for microplastic accumulation dynamics in food webs. Environ. Sci. and Technol. 55 (18), 12372–12382. doi:10.1021/acs.est.1c02922
Mehinto, A. C., Coffin, S., Koelmans, A., Brander, S. M., Wagner, M., Hampton, L., et al. (2022). Risk-based management framework for microplastics in aquatic ecosystems. Microplastics Nanoplastics 2 (1), 17. doi:10.1186/s43591-022-00033-3
Monroe, J. (2013). The lost fish film. Columbia River Inter-Tribal Fish. Commision. Available at: https://critfc.org/fish-and-watersheds/columbia-river-fish-species/lost-fish-film/.
Munno, K., Helm, P. A., Rochman, C., George, T., and Jackson, D. A. (2021). Microplastic contamination in great lakes fish. Conserv. Biol. 36 (n/a), e13794. n/a. doi:10.1111/cobi.13794
Murray, C. C., Maximenko, N., and Lippiatt, S. (2018). The influx of marine debris from the Great Japan Tsunami of 2011 to North American shorelines. Mar. Pollut. Bull. 132, 26–32. doi:10.1016/j.marpolbul.2018.01.004
Nalbone, L., Cincotta, F., Giarratana, F., Ziino, G., and Panebianco, A. (2021). Microplastics in fresh and processed mussels sampled from fish shops and large retail chains in Italy. Food control. 125, 108003. doi:10.1016/j.foodcont.2021.108003
Nan, B., Su, L., Kellar, C., Craig, N. J., Keough, M. J., and Pettigrove, V. (2020). Identification of microplastics in surface water and Australian freshwater shrimp Paratya australiensis in Victoria, Australia. Environ. Pollut. 259, 113865. doi:10.1016/j.envpol.2019.113865
National Environmental Justice Advisory and Council (2002). “Fish consumption and environmental justice,” in Fish consumption and environmental justice, 1–185. Available at: https://www.epa.gov/sites/default/files/2015-02/documents/fish-consumpreport_1102.pdf.
Okamoto, K., Nomura, M., Horie, Y., and Okamura, H. (2022). Color preferences and gastrointestinal-tract retention times of microplastics by freshwater and marine fishes. Environ. Pollut. 304, 119253. doi:10.1016/j.envpol.2022.119253
Qi, K., Lu, N., Zhang, S., Wang, W., Wang, Z., and Guan, J. (2021). Uptake of Pb(II) onto microplastic-associated biofilms in freshwater: adsorption and combined toxicity in comparison to natural solid substrates. J. Hazard. Mater. 411, 125115. doi:10.1016/j.jhazmat.2021.125115
Qiao, R., Deng, Y., Zhang, S., Wolosker, M. B., Zhu, Q., Ren, H., et al. (2019). Accumulation of different shapes of microplastics initiates intestinal injury and gut microbiota dysbiosis in the gut of zebrafish. Chemosphere 236, 124334. doi:10.1016/j.chemosphere.2019.07.065
Ragusa, A., Svelato, A., Santacroce, C., Catalano, P., Notarstefano, V., Carnevali, O., et al. (2021). Plasticenta: first evidence of microplastics in human placenta. Environ. Int. 146, 106274. doi:10.1016/j.envint.2020.106274
Ragusa, A., Notarstefano, V., Svelato, A., Belloni, A., Gioacchini, G., Blondeel, C., et al. (2022). Raman microspectroscopy detection and characterisation of microplastics in human breastmilk. Polymers 14 (13), 2700.
Rangaraj, M. V., Rambabu, K., Banat, F., and Mittal, V. (2021). Natural antioxidants-based edible active food packaging: an overview of current advancements. Food Biosci. 43, 101251. doi:10.1016/j.fbio.2021.101251
Richerson, K., Punt, A. E., and Holland, D. S. (2020). Nearly a half century of high but sustainable exploitation in the Dungeness crab (Cancer magister) fishery. Fish. Res. 226, 105528. doi:10.1016/j.fishres.2020.105528
Robison, F. V. (2022). Net values: social and economic impacts of the Oregon marine reserves on the fishing communities of garibaldi, depoe bay, and port orford. Corvallis, OR: Oregon State University.
Rochman, C. M., Tahir, A., Williams, S. L., Baxa, D. V., Lam, R., Miller, J. T., et al. (2015). Anthropogenic debris in seafood: plastic debris and fibers from textiles in fish and bivalves sold for human consumption fibers from textiles in fish and bivalves sold for human consumption. Sci. Rep., 5(1), 14340. doi:10.1038/srep14340
Romeo, T., Pietro, B., Pedà, C., Consoli, P., Andaloro, F., and Fossi, M. C. (2015). First evidence of presence of plastic debris in stomach of large pelagic fish in the Mediterranean Sea. Mar. Pollut. Bull. 95 (1), 358–361. doi:10.1016/j.marpolbul.2015.04.048
Sait, S. T. L., Sørensen, L., Kubowicz, S., Vike-Jonas, K., Gonzalez, S. V., Asimakopoulos, A. G., et al. (2021). Microplastic fibres from synthetic textiles: environmental degradation and additive chemical content. Environ. Pollut. 268, 115745. doi:10.1016/j.envpol.2020.115745
Sharifinia, M., Bahmanbeigloo, Z. A., Keshavarzifard, M., Khanjani, M. H., and Lyons, B. P. (2020). Microplastic pollution as a grand challenge in marine research: a closer look at their adverse impacts on the immune and reproductive systems. Ecotoxicol. Environ. Saf. 204, 111109. doi:10.1016/j.ecoenv.2020.111109
Siddiqui, S., Dickens, J. M., Cunningham, B. E., Hutton, S. J., Pedersen, E. I., Harper, B., et al. (2022). Internalization, reduced growth, and behavioral effects following exposure to micro and nano tire particles in two estuarine indicator species. Chemosphere 296, 133934. doi:10.1016/j.chemosphere.2022.133934
Sjostrom, A. J. C., Ciannelli, L., Conway, F., and Wakefield, W. W. (2021). Gathering local ecological knowledge to augment scientific and management understanding of a living coastal resource: the case of Oregon’s nearshore groundfish trawl fishery. Mar. Policy 131, 104617. doi:10.1016/j.marpol.2021.104617
Solomando, A., Capó, X., Alomar, C., Álvarez, E., Compa, M., Valencia, J. M., et al. (2020). Long-term exposure to microplastics induces oxidative stress and a pro-inflammatory response in the gut of Sparus aurata Linnaeus, 1758. Environ. Pollut. 266, 115295. doi:10.1016/j.envpol.2020.115295
Sun, Q., Ren, S.-Y., and Ni, H.-G. (2020). Incidence of microplastics in personal care products: an appreciable part of plastic pollution. Sci. Total Environ. 742, 140218. doi:10.1016/j.scitotenv.2020.140218
Talbot, R., Granek, E., Chang, H., Wood, R., and Brander, S. (2022). Spatial and temporal variations of microplastic concentrations in Portland’s freshwater ecosystems. Sci. Total Environ. 833, 155143. doi:10.1016/j.scitotenv.2022.155143
Talley, T. S., Venuti, N., and Whelan, R. (2020). Natural history matters: plastics in estuarine fish and sediments at the mouth of an urban watershed. PLOS ONE 15 (3), e0229777. doi:10.1371/journal.pone.0229777
Tamis, J. E., Koelmans, A. A., Dröge, R., Kaag, N. H. B. M., Keur, M. C., Tromp, P. C., et al. (2021). Environmental risks of car tire microplastic particles and other road runoff pollutants. Microplastics Nanoplastics 1 (1), 10. doi:10.1186/s43591-021-00008-w
Tanaka, K., van Franeker, J. A., Deguchi, T., and Takada, H. (2019). Piece-by-piece analysis of additives and manufacturing byproducts in plastics ingested by seabirds: implication for risk of exposure to seabirds. Mar. Pollut. Bull. 145, 36–41. doi:10.1016/j.marpolbul.2019.05.028
Tanaka, K., Watanuki, Y., Takada, H., Ishizuka, M., Yamashita, R., Kazama, M., et al. (2020). In Vivo accumulation of plastic-derived chemicals into seabird tissues seabird tissues. Curr. Biol. 30 (4), 723–728.e3. doi:10.1016/j.cub.2019.12.037
Thiele, C. J., Hudson, M. D., Russell, A. E., Saluveer, M., and Sidaoui-Haddad, G. (2021). Microplastics in fish and fishmeal: an emerging environmental challenge? Sci. Rep. 11 (1), 2045. doi:10.1038/s41598-021-81499-8
Thornton Hampton, L. M., Bouwmeester, H., Brander, S. M., Coffin, S., Cole, M., Hermabessiere, L., et al. (2022). Research recommendations to better understand the potential health impacts of microplastics to humans and aquatic ecosystems. Microplastics Nanoplastics 2, 18. doi:10.1186/s43591-022-00038-y
Torres, L. G., Brander, S. M., Parker, J. I., Bloom, E. M., Norman, R., Van Brocklin, J. E., et al. (2023). Zoop to poop: assessment of microparticle loads in gray whale zooplankton prey and fecal matter reveal high daily consumption rates. Front. Mar. Sci. 26. doi:10.3389/fmars.2023.1201078
Trestrail, C., Walpitagama, M., Miranda, A., Nugegoda, D., and Shimeta, J. (2021). Microplastics alter digestive enzyme activities in the marine bivalve, Mytilus galloprovincialis. Sci. Total Environ. 779, 146418. doi:10.1016/j.scitotenv.2021.146418
Uzun, P., Farazande, S., and Guven, B. (2022). Mathematical modeling of microplastic abundance, distribution, and transport in water environments: a review. Chemosphere 288, 132517. doi:10.1016/j.chemosphere.2021.132517
Valine, A. E., Peterson, A. E., Horn, D. A., Scully-Engelmeyer, K. M., and Granek, E. F. (2020). Microplastic prevalence in 4 Oregon rivers along a rural to urban gradient applying a cost-effective validation technique. Environ. Toxicol. Chem. 39 (8), 1590–1598. doi:10.1002/etc.4755
Walkinshaw, C., Lindeque, P. K., Thompson, R., Tolhurst, T., and Cole, M. (2020a). Microplastics and seafood: lower trophic organisms at highest risk of contamination. Ecotoxicol. Environ. Saf. 190, 110066. doi:10.1016/j.ecoenv.2019.110066
Walkinshaw, C., Lindeque, P. K., Thompson, R., Tolhurst, T., and Cole, M. (2020b). Microplastics and seafood: lower trophic organisms at highest risk of contamination. Ecotoxicol. Environ. Saf. 190, 110066. doi:10.1016/j.ecoenv.2019.110066
Walkinshaw, C., Tolhurst, T. J., Lindeque, P. K., Thompson, R. C., and Cole, M. (2023). Impact of polyester and cotton microfibers on growth and sublethal biomarkers in juvenile mussels. Microplastics and Nanoplastics 3 (1), 5. doi:10.1186/s43591-023-00052-8
Wang, C., Zhao, J., and Xing, B. (2021). Environmental source, fate, and toxicity of microplastics microplastics. J. Hazard. Mater. 407, 124357. doi:10.1016/j.jhazmat.2020.124357
Wang, F., Wu, H., Wu, W., Wang, L., Xia, B., Zhang, J., et al. (2020). Polystyrene microplastics increase uptake, elimination and cytotoxicity of decabromodiphenyl ether (BDE-209) in the marine scallop Chlamys farreri. Environ. Pollut. 258, 113657. doi:10.1016/j.envpol.2019.113657
Yang, H., Chen, G., and Wang, J. (2021). Microplastics in the marine environment: sources, fates, impacts and microbial degradation fates, impacts and microbial degradation. Toxics, 9(2), 41. doi:10.3390/toxics9020041
Keywords: contamination, lingcod, microplastics, Oregon, Pacific herring, Pacific lamprey, pink shrimp
Citation: Traylor SD, Granek EF, Duncan M and Brander SM (2024) From the ocean to our kitchen table: anthropogenic particles in the edible tissue of U.S. West Coast seafood species. Front. Toxicol. 6:1469995. doi: 10.3389/ftox.2024.1469995
Received: 24 July 2024; Accepted: 19 November 2024;
Published: 24 December 2024.
Edited by:
Marisa Passos, RWTH Aachen University, GermanyReviewed by:
Sedat Gundogdu, Çukurova University, TürkiyeWenke Yuan, Chinese Academy of Sciences (CAS), China
Copyright © 2024 Traylor, Granek, Duncan and Brander. This is an open-access article distributed under the terms of the Creative Commons Attribution License (CC BY). The use, distribution or reproduction in other forums is permitted, provided the original author(s) and the copyright owner(s) are credited and that the original publication in this journal is cited, in accordance with accepted academic practice. No use, distribution or reproduction is permitted which does not comply with these terms.
*Correspondence: Elise F. Granek, Z3JhbmVrZUBwZHguZWR1