- 1Surgical Critical Care Initiative, Department of Surgery, Uniformed Services University of the Health Sciences, Bethesda, MD, United States
- 2Department of Surgery, Uniformed Services University of Health Sciences, Bethesda, MD, United States
- 3Henry M. Jackson Foundation for the Advancement of Military Medicine, Inc., Bethesda, MD, United States
- 4Walter Reed National Military Medical Center, Bethesda, MD, United States
Introduction: Trauma is the leading cause of death in persons under the age of 45. Recovery in patients who survive initial trauma are frequently complicated by sequelae of injury that increases susceptibility to infection and inflammation. Uncontrolled inflammation can advance into life-threatening organ failure, including acute respiratory distress syndrome (ARDS). Similarities exist between biomarkers established in the etiology of acute respiratory distress syndrome and those identified in the acute inflammatory and healing phase of bone fractures. This study investigates the impact of long bone fractures on the development of acute respiratory distress syndrome where it is hypothesized that patients with long bone fractures would have different biomarker profiles and increased development of lung injury compared to patients without long bone fractures.
Methods: This is a retrospective data analysis of patients from an observational data repository from three trauma centers. Trauma patients with and without long bone fractures were matched and analyzed for the presence of known biomarkers of acute respiratory distress syndrome and for the development of acute respiratory distress syndrome.
Results: There were no differences in overall acute respiratory distress syndrome development or hospital outcomes, however long bone fracture patients had a 2.35-fold higher hazard ratio of acute respiratory distress syndrome in the first 10 hospital days. There was a statistically significant increase in the levels of IL-6 in patients with long bone fractures (p = .0007). Structural equations modeling demonstrated that IL-6 was positively influenced by long bone fractures and IL-8.
Conclusion: The presence of long bone fractures did not result in differences in the overall development of acute respiratory distress syndrome or hospital outcomes, though was found to have an increased hazard ratio for acute respiratory distress syndrome development in the first 10 days. Further research is needed to better characterize the relationship between varying cytokine profiles and the development of acute respiratory distress syndrome in a trauma population.
1 Introduction
In the United States, trauma is the leading cause of death in persons under the age of 45 with over 150,000 deaths per year (Centers for Disease Control and PreventionNational Center for Injury Prevention and Control, 2017). Moreover, trauma is the leading cause of nearly all deaths in modern military conflict. In those wounded in combat, more than half sustain a musculoskeletal injury to the extremities (Belmont et al., 2016). Recovery in patients who survive initial trauma is frequently complicated by sequelae of injury that renders patients susceptible to infection and inflammation due to the activation of innate immunity and production of intracellular inflammatory mediators (Lord et al., 2014). Uncontrolled inflammation in the setting of critical injury can advance into life-threatening organ failure, such as acute respiratory distress syndrome (ARDS; Matthay et al., 2012).
ARDS, a form of acute lung injury (ALI), is a progressive inflammatory condition of the lungs that can be defined by acute onset, bilateral lung infiltrates, absence of left atrial hypertension, and hypoxemia (Ranieri et al., 2012). Central to ARDS pathogenesis is dysregulated inflammation and increased alveolar epithelial permeability (Huppert et al., 2019). Prolonged and uncontrolled inflammation following injury causes an accumulation of neutrophils and inflammatory cells within the alveoli and eventual infiltration of proteinaceous fluid and hyaline membranes, resulting in the pulmonary edema and decreased oxygenation characteristic of ARDS (Xu and Song, 2017). Several studies identify specific biomarkers that are associated with trauma and the development of ARDS from systemic inflammation, including mitochondrial DNA (mtDNA), IL-1, IL-6, IL-8, IFN-α, TNF-α, among others (Calfee et al., 2007; Han and Mallampalli, 2015; Xu and Song, 2017).
Similarities exist between these biomarkers established in the etiology of ARDS and those identified in the acute inflammatory response and healing phases of bone fractures. The initial acute phase relies on local and systemic mediators to promote inflammatory reactions primarily through the activation of TNF-α, IL-1b, IL-6, IL-11, and IL-18 (Mountziaris and Mikos, 2008; Maruyama et al., 2020). This is followed by repair and remodeling phases characterized by vascular remodeling and lamellar bone deposition, the latter of which has been shown to express high levels of IL-1, IL-6, and TNF-α (Maruyama et al., 2020).
Given the substantial number of long bone fractures within military combat and the high incidence of trauma related injuries in the general population, this study investigates the impact of long bone fractures on ARDS development. It is hypothesized that given the similarities between cytokines produced in bone fractures and those that are implicated in the development of ARDS, patients with long bone fractures in this trauma population would have different biomarker profiles and increased risk of lung injury that differ from patients without long bone fractures.
2 Patients and methods
2.1 Study design and population
This was a retrospective data analysis of patients from a large observational data repository of three trauma centers, the Surgical Critical Care Initiative Tissue and Data Acquisition Protocol (TDAP; Vicente et al., 2021; Gelbard et al., 2019). TDAP is a prospective longitudinal evaluation of patients with established injury requiring surgical management or management within a surgical critical care setting. Inclusion criteria were adult patients aged 18–80 who presented to the hospital for trauma with acute or initially stabilized severe injuries. Stable patients included those who have suffered a traumatic injury as the reason for initial visit, but who have been medically treated and stabilized. Acute patients included those with acute traumatic injury recognized upon admission to the emergency department or surgical intensive care setting. Trauma patients were further classified based on the presence or absence of long bone fractures (femur, tibia, fibula, humerus, radius, ulna) and pelvic fracture. Exclusion criteria included pregnancy or any condition that would place the patient at undue risk by participating.
Following written informed consent, biospecimen samples were collected from patients including blood, effluent, cerebral spinal fluid, and/or urine. Blood samples were collected on Day 0 (first 24 h after hospital presentation, injury, or qualifying event), Day 1, Day 3, Day 7, and every 7 days (±24 h). Samples were also collected in the event of any change in a patient’s condition requiring surgical operative intervention or decompensation requiring resuscitative measures. All biospecimen collection data were entered into a Clinical REDCap Event.
2.2 Data collection
Demographic and clinical data collected included age, race, sex, comorbidities, injury type, injury mechanism, extended injury severity score (EISS), acute physiology, age, chronic health evaluation (APACHE) score, sequential organ failure, and fluid and blood transfusion requirements. Biomarkers collected included mtDNA, TNF-a, IFN-α, IFN-γ, IL-1a, IL-1b, and IL-1 receptor antagonist (IL-1RA), IL-2, IL-4, IL-6, IL-8, IL-10, IL-12, and iCAM. Outcomes included the development of ARDS/ALI, intensive care unit (ICU) days, hospital days, and hospital mortality. ARDS diagnosis was based on the accepted Berlin Criteria: hypoxemic respiratory failure; acute onset or new or worsening respiratory symptoms; bilateral opacities not fully explained by effusions, lobar or lung collapse; respiratory failure not fully explained by heart failure or fluid overload (Ranieri et al., 2012).
2.3 Statistical analysis
Patients with and without long bone fractures underwent one-to-one propensity score matching based on demographic information and analyzed for pathogenic biomarkers of ARDS and for the development of ARDS and other hospital outcomes. Chest injuries (rib, clavicle, and sternum fractures) were examined as possible confounding variables. Analyses were performed using structural equations modeling (SEM) and Cox survival models for time to onset of ARDS, both overall and early (10 days) in the hospital course, when ARDS most frequently develops. SEM was used to test and evaluate the relationships between multiple variables. Using this method allowed for greater statistical power to detect pathways between the many correlated variables as compared to conventional multiple regression analyses (Beran and Violato, 2010). Multiple imputations based on random forest were used to fill in missing data. Numerical variables were compared by t-tests, while categorical variables were compared by chi-square tests.
3 Results
3.1 Patient demographics
From 2015 to 2020, 857 trauma patients were enrolled in the study. After propensity score matching, 281 patients in each cohort, with and without long bone fractures, were identified and utilized for analysis. The matched groups did not differ substantially in demographic characteristics (Table 1). There were no statistically significant differences in comorbidities between groups. Injuries that resulted in long bone fractures were more likely to be blunt, while injuries that did not sustain long bone fractures were more likely to be penetrating. The primary mechanism of trauma in those with long bone fractures was motor vehicle collisions (MVC), while the primary mechanisms of trauma in those without long bone fractures were penetrating injuries–gunshot or stab wounds. Patients with long bone fractures were more likely to have a higher EISS as compared to patients without long bone fractures (27.5 vs. 23.5, p = .0009).
3.2 Early ARDS is associated with long bone fractures
In total, 9.8% of patients developed ARDS with no statistically significant difference between patients with and without long bone fractures (11.3% vs. 8.2%, p = .26). Time to the onset of ARDS was assessed with Cox survival analysis models. Due to the small number of ARDS cases (N = 55), only long bone fractures were considered as a predictor variable in order to isolate their effect on ARDS development. We determined that 48 out of all 55 ARDS cases (87%) occurred within the first 10 days. Upon analysis of the first 10 days following injury, when the vast majority of ARDS cases occurred, long bone fracture patients had a 2.35-fold higher hazard ratio of ARDS (p = .0053; Figure 1). However, following the initial 10 days, there was no difference in the probability of ARDS development between the two groups. Overall, there was no significant difference in the risk of ARDS when considering the entire time span, where patients stayed up to 216 days in the hospital.
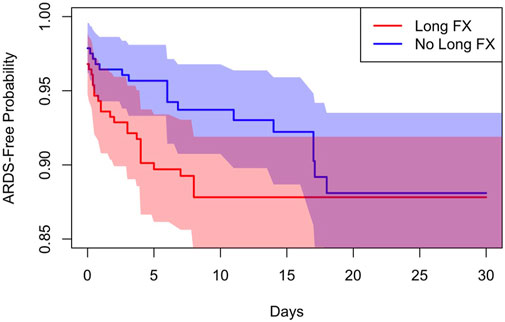
FIGURE 1. Cox survival model demonstrates a 2.35-fold higher hazard ratio of ARDS development in the first 10 days in patients with long bone fractures.
There was a statistically significant increase in the levels of IL-6 in patients with long bone fractures (p = .0007). No significant differences were found among other biomarkers (Table 2). When analyzed by race, Black patients had statistically significant increases in IL-1a,, IL-1RA, IL-08, and IL-10 and a statistically significant decrease in IL-12 when compared to White patients (Table 3). Separate survival models were fit for Black and White patients during the first 10 days and similar effect sizes for long bone fractures were found. Black patients had a hazard ratio of 1.26 (p = .03) while White patients had a hazard ratio of .85 (p = .03).
Patients with long bone fractures had lower arterial blood gas partial pressure of oxygen (PaO2) with an average of 214 mmHg vs. 229 mmHg (p = .04), with no difference identified in oxygen saturation or blood pH regardless of ventilation status. No significant differences were found in hospital outcomes between patients with and without long bone fractures. Mortality was seen in 6% of patients with fractures as compared to 2.5% of patients without long bone fractures (p = .06). Total ICU days were equivalent with an average of 7.8 days vs. 7.9 days (p = .94), and total hospital days of 20.6 days vs. 19.4 days (p = .54) in patients with and without long bone fractures, respectively (Table 4).
3.3 Structural equations modeling demonstrates relationship between ARDS and IL-6
SEM demonstrated that the presence of long bone fractures was not directly related to ARDS development (Figure 2). However, the SEM demonstrated that IL-6, IFN-α and blood transfusions had positive associations with ARDS development (coefficient = .03, .03, .10, respectively). IL-6, a proinflammatory cytokine, was positively influenced by both long bone fractures (coefficient = .33) and IL-8, a key neutrophil recruiter (coefficient = .45). ARDS development was also weakly negatively influenced by IFN-γ, albumin, and IL-8 (coefficient = −.03, −.04, −.04, respectively).
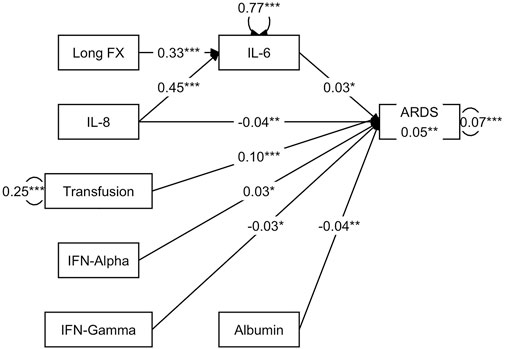
FIGURE 2. SEM demonstrates a direct relationship between IL-6 and ARDS development, where IL-6 is positively influenced by long bone fractures and IL-8.
4 Discussion
ARDS is a serious and potentially fatal consequence of polytrauma, occurring in up to 25% of traumatically injured patients (Watkins et al., 2012; Kasotakis et al., 2021). Though the incidence of ARDS after trauma has been declining in recent years, mortality remains high at rates ranging from 13% to 20% (Watkins et al., 2012; van Wessem and Leenen, 2018; Kasotakis et al., 2021). The use of commonly used trauma injury indices (Injury Severity Score [ISS], Glascow Coma Scale [GCS], APACHE) to predict ARDS development in trauma populations has been well studied (Afshar et al., 2016). However, the isolated effect of fractures in the development of ARDS has not yet been elucidated. With information collected from three trauma centers in the United States in the context of a prospective clinical trial, we found the incidence of ARDS to be 9.8%, slightly lower than incidences observed in other studies which may be a result of modern resuscitation and cardiopulmonary support. In particular, the present study found no difference in overall ARDS development between patients with and without long bone fractures (11.4% vs. 8.2%, p = .26). However, patients with long bone fractures were found to have a 2.35-fold higher hazard ratio of ARDS (p = .0053) within the first 10 days as compared to patients without long bone fractures with no significant difference in the risk of ARDS when considering the entire hospital length of stay (LOS) suggesting the presence of long bone fractures may place patients at increased risk of early ARDS development. This discrepancy makes intuitive sense, as early ARDS is thought to result from the systemic and inflammatory sequelae of injury and resuscitation, while late ARDS most likely reflects downstream complications less directly related to initial injury.
Though time to ARDS resulted in a significant difference, no differences in overall hospital outcomes between the cohorts were observed. Mortality between patients with and without long bone fractures was found to be 6% vs. 2.5% (p = .06). LOS was similar between groups in both ICU days (7.8 days vs. 7.9 days, p = .94) and number of hospital days (20.6 days vs. 19.4 days, p = .54), suggesting that the presence of long bone fractures in a trauma patient does not significantly impact long term hospital outcomes. Recent studies of ARDS in trauma patients report ICU LOS of 12–22 days and hospital LOS of 17–36 days in patients with an ARDS diagnosis, which are significantly greater than patients without ARDS (Salim et al., 2006; Afshar et al., 2016; van Wessem and Leenen, 2018; Kasotakis et al., 2021).
When evaluated by race, Black patients were found to have increased levels of IL-01a, IL-1RA, IL-08, and IL-10 and decreased levels of IL-12 as compared to White patients. However, when adjusted for long bone fracture status, race was not found to significantly affect hazard ratio for ARDS development during the first 10 days. This finding is similar to previous studies that demonstrated no difference in the development of ARDS/ALI between different racial groups (Plurad et al., 2007; Brown et al., 2011).
Recent studies have documented higher average ISS among trauma patients who develop ARDS, ranging on average from 23–30 (Watkins et al., 2012; Afshar et al., 2016; van Wessem and Leenen, 2018; Kasotakis et al., 2021). Compared to other injury severity markers such as APACHE, GCS, revised trauma score, and abbreviated injury score of thorax, ISS has proven to be the best predictor for ARDS development (Afshar et al., 2016). In our current study, patients presenting with long bone fractures were found to have a significantly higher EISS at 27.5 vs. 23.5 (p = .0009), though this difference was not found to be related to the development of ARDS.
The hallmark of injury central to ARDS is dysregulated inflammation and increased alveolar epithelial permeability (Huppert et al., 2019). Previous studies have identified specific biomarkers involved in the acute immune activation and inflammatory cascade that leads to ARDS (Calfee et al., 2007; Han and Mallampalli, 2015; Xu and Song, 2017). In particular, cytokines such as IL-1β, TNF-α, IL-6, and IL-8 have been elevated in both plasma and bronchoalveolar lavage fluid in patients with ARDS (Meduri et al., 2009). While the majority of cytokines displayed no significant difference between patients with and without long bone fractures, the production of IL-6 was significantly increased in patients with long bone fractures (p < .0007).
There is strong evidence that supports the role of IL-6 in the context of the acute inflammatory response. Secreted by T cells, macrophages and endothelial cells, IL-6 is a critical pro-inflammatory factor that activates a cascade of inflammatory mediators (Qin and Qiu, 2019). IL-6 promotes the differentiation of B cells, activation of additional T cells, as well as the synthesis of various acute phase reactants including C-reactive protein and fibrinogen (Maggio et al., 2006). IL-6 additionally plays a particular role in mediating the cytokine excess in lung tissue that precipitates microvascular injury resulting in diffuse alveolar damage, the hallmark of acute lung injury (Severgnini et al., 2004). One possible mechanism for this reaction is through IL-6 activation of the Janus tyrosine kinase (JAK), which leads to further activation via phosphorylation of signal transducer and activator of transcription (STAT) family of transcription factors (Čokić et al., 2015). JAK/STAT signaling induces alveolar macrophage polarization to a pro-inflammatory state which ultimately contributes to tissue destruction (Chen and Hua, 2020; Wang et al., 2020). In various animal models and human tissue models, STAT transcription factors were found to be activated prior to evidence of acute lung injury suggesting that STAT plays a role in the initial pulmonary inflammatory response (Severgnini et al., 2004). Type I interferons, including IFN-α, have also been implicated in the initiation of lung inflammation through its recruitment and activation of immune cells and cytokines. While typically seen in response to viral infections, this proinflammatory function of Type I IFNs may further contribute to the initiation and maintenance of lung inflammation (Makris et al., 2017).
While IL-6 is a pro-inflammatory mediator involved in the pathogenesis of diffuse alveolar damage, it also plays a role in inflammation suppression through its activation of IL-1RA, a key anti-inflammatory mediator (Steensberg et al., 2003). IL-1RA is a potent suppressor of IL-1, an additional pro-inflammatory cytokine primarily produced by monocytes and activated macrophages (Dinarello, 2018). The role of IL-6 in both inducing and suppressing pulmonary inflammation illustrates the delicate interplay between pro-inflammatory reactions responsible for responding to acute injury and anti-inflammatory processes necessary to mitigate prolonged tissue catabolism.
Although the present study did not show any relationship between overall ARDS development and long bone fractures, the process of bone repair and the pathogenesis of ARDS share a similar cytokine profile. The ultimate healing of fractured bones relies on acute inflammation affected by both local and systemic mediators. During this acute phase, which typically occurs 24–48 h from injury, activated macrophages both directly contribute to host defense and promote further inflammatory reactions through the activation of TNF-α, IL-1b, IL-6, IL-11, and IL-18 (Maruyama et al., 2020). The acute inflammatory phase is followed by a repair phase, characterized by vascular remodeling and collagen deposition, and a remodeling phase, characterized by lamellar bone deposition resulting from an interplay of osteoblasts and osteoclasts. In particular, IL-1, IL-6, and TNF-α have been found to be highly expressed during the final remodeling phase (Maruyama et al., 2020). Patients with long bone fractures were found to have both an increased level of IL-6 as well as an increased probability of ARDS in the first 10 days, which suggests a possible association between varying cytokine profiles and onset of ARDS.
We recognize several limitations to our present study. Primarily, this study resulted in a relatively small sample size (n = 281) within each cohort after propensity score matching. Of note, we report a nearly 40% increased risk (11.4% vs. 8.2%) of ARDS throughout the entire hospital stay and a 240% increased risk of death (6% vs. 2.5%) in patients with long bone fractures, but neither of these findings was statistically significant. Additionally, the total incidence of ARDS remained low (n = 55), which likely reflects the decreasing ARDS rates with modern resuscitation and cardiopulmonary support, but this may have contributed to limited statistical power. Included in the study were patients who had underlying respiratory conditions which may have independently contributed to further lung injury, although we did propensity score matching including co-morbidities. Finally, we did not account for the clinical diagnosis of fat embolism, specifically, within the population. Though uncommon, fat emboli arising from long bone fractures may also play an independent role in lung injury and the development of ARDS (Kao et al., 2007).
In conclusion, although similarities exist between the biomarkers involved in the pathogenesis of ARDS and those involved in the inflammatory response of bone tissue repair, the presence of long bone fractures did not result in a difference in the overall development of ARDS or hospital outcomes. However, long bone fractures were associated with increased IL-6. Additionally, patients with long bone fractures were found to have a higher hazard ratio for ARDS development within the first 10 days without differences in overall ARDS development or hospital outcome. Further research is needed to better characterize the relationship between varying cytokine profiles and the development of ARDS in a trauma population.
Data availability statement
The raw data supporting the conclusion of this article will be made available by the authors, without undue reservation.
Ethics statement
Ethical review and approval was not required for the study on human participants in accordance with the local legislation and institutional requirements. The patients/participants provided their written informed consent to participate in this study.
Author contributions
JL conceived the presented idea and wrote the manuscript with support from BP and EE. HR and SG performed and verified the analytic methods. HR, SG, SS, BP, and EE helped supervise the project. All authors provided feedback and commented on the manuscript.
Acknowledgments
We thank Walter Reed National Military Medical Center, Duke University, Emory University School of Medicine and Grady Medical Center for their participation in the TDAP protocol.
Conflict of interest
Authors HR, SG, and SS were employed by Henry M. Jackson Foundation for the Advancement of Military Medicine, Inc.
The remaining authors declare that the research was conducted in the absence of any commercial or financial relationships that could be construed as a potential conflict of interest.
Publisher’s note
All claims expressed in this article are solely those of the authors and do not necessarily represent those of their affiliated organizations, or those of the publisher, the editors and the reviewers. Any product that may be evaluated in this article, or claim that may be made by its manufacturer, is not guaranteed or endorsed by the publisher.
Supplementary material
The Supplementary Material for this article can be found online at: https://www.frontiersin.org/articles/10.3389/fsysb.2022.1058603/full#supplementary-material
References
Afshar, M., Smith, G. S., Cooper, R. S., Murthi, S., and Netzer, G. (2016). Trauma indices for prediction of acute respiratory distress syndrome. J. Surg. Res. 201 (2), 394–401. doi:10.1016/j.jss.2015.11.050
Belmont, P. J., Owens, B. D., and Schoenfeld, A. J. (2016). Musculoskeletal injuries in Iraq and Afghanistan: Epidemiology and outcomes following a decade of war. J. Am. Acad. Orthop. Surg. 24 (6), 341–348. doi:10.5435/JAAOS-D-15-00123
Beran, T. N., and Violato, C. (2010). Structural equation modeling in medical research: A primer. BMC Res. Notes 3, 267. doi:10.1186/1756-0500-3-267
Brown, L. M., Kallet, R. H., Matthay, M. A., and Dicker, R. A. (2011). The influence of race on the development of acute lung injury in trauma patients. Am. J. Surg. 201 (4), 486–491. doi:10.1016/j.amjsurg.2010.02.003
Calfee, C. S., Eisner, M. D., Ware, L. B., Thompson, B. T., Parsons, P. E., Wheeler, A. P., et al. (2007). Trauma-associated lung injury differs clinically and biologically from acute lung injury due to other clinical disorders. Crit. Care Med. 35 (10), 2243–2250. doi:10.1097/01.ccm.0000280434.33451.87
Centers for Disease Control and Prevention, National Center for Injury Prevention and Control (2017). Web-based injury statistics query and reporting system (WISQARS) fatal injury data.
Chen, Z., and Hua, S. (2020). Transcription factor-mediated signaling pathways' contribution to the pathology of acute lung injury and acute respiratory distress syndrome. Am. J. Transl. Res. 12 (9), 5608–5618.
Čokić, V. P., Mitrović-Ajtić, O., Beleslin-Čokić, B. B., Marković, D., Buač, M., Diklić, M., et al. (2015). Proinflammatory cytokine IL-6 and JAK-STAT signaling pathway in myeloproliferative neoplasms. Mediat. Inflamm. 2015, 453020. doi:10.1155/2015/453020
Dinarello, C. A. (2018). Overview of the IL-1 family in innate inflammation and acquired immunity. Immunol Rev. 281 (1), 8–27. doi:10.1111/imr.12621
Gelbard, R. B., Hensman, H., Schobel, S., Khatri, V., Tracy, B. M., Dente, C. J., et al. (2019). Random forest modeling can predict infectious complications following trauma laparotomy. J. Trauma Acute Care Surg. 87 (5), 1125–1132. doi:10.1097/TA.0000000000002486
Han, S., and Mallampalli, R. K. (2015). The acute respiratory distress syndrome: From mechanism to translation. J. Immunol. 194 (3), 855–860. doi:10.4049/jimmunol.1402513
Huppert, L. A., Matthay, M. A., and Ware, L. B. (2019). Pathogenesis of acute respiratory distress syndrome. Semin. Respir. Crit. Care Med. 40 (1), 31–39. doi:10.1055/s-0039-1683996
Kao, S. J., Yeh, D. Y., and Chen, H. I. (2007). Clinical and pathological features of fat embolism with acute respiratory distress syndrome. Clin. Sci. (Lond). 113 (6), 279–285. doi:10.1042/CS20070011
Kasotakis, G., Stanfield, B., Haines, K., Vatsaas, C., Alger, A., Vaslef, S. N., et al. (2021). Acute respiratory distress syndrome (ARDS) after trauma: Improving incidence, but increasing mortality. J. Crit. Care 64, 213–218. doi:10.1016/j.jcrc.2021.05.003
Lord, J. M., Midwinter, M. J., Chen, Y. F., Belli, A., Brohi, K., Kovacs, E. J., et al. (2014). The systemic immune response to trauma: An overview of pathophysiology and treatment. Lancet 384 (9952), 1455–1465. doi:10.1016/S0140-6736(14)60687-5
Maggio, M., Guralnik, J. M., Longo, D. L., and Ferrucci, L. (2006). Interleukin-6 in aging and chronic disease: A magnificent pathway. J. Gerontol. A Biol. Sci. Med. Sci. 61 (6), 575–584. doi:10.1093/gerona/61.6.575
Makris, S., Paulsen, M., and Johansson, C. (2017). Type I interferons as regulators of lung inflammation. Front. Immunol. 8, 259. doi:10.3389/fimmu.2017.00259
Maruyama, M., Rhee, C., Utsunomiya, T., Zhang, N., Ueno, M., Yao, Z., et al. (2020). Modulation of the inflammatory response and bone healing. Front. Endocrinol. (Lausanne). 11, 386. doi:10.3389/fendo.2020.00386
Matthay, M. A., Ware, L. B., and Zimmerman, G. A. (2012). The acute respiratory distress syndrome. J. Clin. Invest. 122 (8), 2731–2740. doi:10.1172/JCI60331
Meduri, G. U., Annane, D., Chrousos, G. P., Marik, P. E., and Sinclair, S. E. (2009). Activation and regulation of systemic inflammation in ARDS: Rationale for prolonged glucocorticoid therapy. Chest 136 (6), 1631–1643. doi:10.1378/chest.08-2408
Mountziaris, P. M., and Mikos, A. G. (2008). Modulation of the inflammatory response for enhanced bone tissue regeneration. Tissue Eng. Part B Rev. 14 (2), 179–186. doi:10.1089/ten.teb.2008.0038
Plurad, D., Martin, M., Green, D., Salim, A., Inaba, K., Belzberg, H., et al. (2007). The decreasing incidence of late posttraumatic acute respiratory distress syndrome: The potential role of lung protective ventilation and conservative transfusion practice. J. Trauma 63 (1), 1–7. doi:10.1097/TA.0b013e318068b1ed
Qin, M., and Qiu, Z. (2019). Changes in TNF-α, IL-6, IL-10 and VEGF in rats with ARDS and the effects of dexamethasone. Exp. Ther. Med. 17 (1), 383–387. doi:10.3892/etm.2018.6926
Ranieri, V. M., Rubenfeld, G. D., Thompson, B. T., Ferguson, N. D., Caldwell, E., Fan, E., et al. (2012). Acute respiratory distress syndrome: The Berlin definition. JAMA 307 (23), 2526–2533. doi:10.1001/jama.2012.5669
Salim, A., Martin, M., Constantinou, C., Sangthong, B., Brown, C., Kasotakis, G., et al. (2006). Acute respiratory distress syndrome in the trauma intensive care unit: Morbid but not mortal. Arch. Surg. 141 (7), 655–658. doi:10.1001/archsurg.141.7.655
Severgnini, M., Takahashi, S., Rozo, L. M., Homer, R. J., Kuhn, C., Jhung, J. W., et al. (2004). Activation of the STAT pathway in acute lung injury. Am. J. Physiol. Lung Cell Mol. Physiol. 286 (6), L1282–L1292. doi:10.1152/ajplung.00349.2003
Steensberg, A., Fischer, C. P., Keller, C., Møller, K., and Pedersen, B. K. (2003). IL-6 enhances plasma IL-1ra, IL-10, and cortisol in humans. Am. J. Physiol. Endocrinol. Metab. 285 (2), E433–7. doi:10.1152/ajpendo.00074.2003
van Wessem, K. J. P., and Leenen, L. P. H. (2018). Incidence of acute respiratory distress syndrome and associated mortality in a polytrauma population. Trauma Surg. Acute Care Open 3 (1), e000232. doi:10.1136/tsaco-2018-000232
Vicente, D., Schobel, S. A., Anfossi, S., Hensman, H., Lisboa, F., Robertson, H., et al. (2021). Viral micro- RNAs are detected in the early systemic response to injury and are associated with outcomes in polytrauma patients. Crit. Care Med. 50, 296–306. doi:10.1097/CCM.0000000000005181
Wang, L., Zhang, H., Sun, L., Gao, W., Xiong, Y., Ma, A., et al. (2020). Manipulation of macrophage polarization by peptide-coated gold nanoparticles and its protective effects on acute lung injury. J. Nanobiotechnology 18 (1), 38. doi:10.1186/s12951-020-00593-7
Watkins, T. R., Nathens, A. B., Cooke, C. R., Psaty, B. M., Maier, R. V., Cuschieri, J., et al. (2012). Acute respiratory distress syndrome after trauma: Development and validation of a predictive model. Crit. Care Med. 40 (8), 2295–2303. doi:10.1097/CCM.0b013e3182544f6a
Keywords: acute lung injury, ARDS, trauma, critical care, long bone fracture
Citation: Larson JL, Robertson HT, Grey SF, Schobel SA, Potter BK and Elster EA (2023) Acute respiratory distress syndrome and acute lung injury in a trauma population with and without long bone fractures. Front. Syst. Biol. 2:1058603. doi: 10.3389/fsysb.2022.1058603
Received: 30 September 2022; Accepted: 25 November 2022;
Published: 05 January 2023.
Edited by:
Naveena Yanamala, Rutgers, The State University of New Jersey, United StatesReviewed by:
Nathan Weinstein, National Autonomous University of Mexico, MexicoLuis Diambra, National University of La Plata, Argentina
Copyright © 2023 Larson, Robertson, Grey, Schobel, Potter and Elster. This is an open-access article distributed under the terms of the Creative Commons Attribution License (CC BY). The use, distribution or reproduction in other forums is permitted, provided the original author(s) and the copyright owner(s) are credited and that the original publication in this journal is cited, in accordance with accepted academic practice. No use, distribution or reproduction is permitted which does not comply with these terms.
*Correspondence: Julia L. Larson, anVsaWEubC5sYXJzb24ubWlsQGhlYWx0aC5taWw=