- 1Department of Neuroscience, School of Medicine, West Virginia University Health Science Center, Morgantown, WV, United States
- 2Rockefeller Neuroscience Institute, West Virginia University, Morgantown, WV, United States
- 3Department of Neurosurgery, Rockefeller Neuroscience Institute, West Virginia University, Morgantown, WV, United States
- 4Department of Microbiology, Immunology, and Cell Biology, School of Medicine, West Virginia University Health Science Center, Morgantown, WV, United States
- 5Department of Neurosurgery, Rutgers-Robert Wood Johnson Medical School, New Brunswick, NJ, United States
Subarachnoid hemorrhage (SAH) is a type of hemorrhagic stroke characterized by high morbidity and mortality. Saccular intracranial aneurysms account for most cases of SAH. While the role of hemodynamic stress and inflammation have been extensively studied in SAH, little is known about the role of the microbiome in SAH despite recent studies uncovering new insights on the effects of microbiome alteration in ischemic stroke. This review presents the current knowledge around the role of the microbiome in intracranial aneurysm formation and rupture. We also highlight the influence of diet on intracranial aneurysm formation and provide evidence that corroborates the targeting of inflammatory pathways as a potential strategy to curb SAH-associated neurological dysfunction.
Introduction
Subarachnoid hemorrhage (SAH) is a life-threatening condition that results from the build-up of blood between the arachnoid and pia mater. SAH affects 10 to 14 out of 100,000 persons annually in the United States and is responsible for 10% of hemorrhagic strokes (de Rooij et al., 2007; Ziu, 2022). The etiology of SAH can be non-traumatic or traumatic. Most (85%) non-traumatic SAH arise secondary to intracranial aneurysm rupture (Ikawa et al., 2020). Risk factors such as age (>60), substance abuse (e.g., cocaine), smoking, hypertension, and genetic conditions have been shown to increase the risk of SAH and aneurysm rupture (Vlak et al., 2012).
Hemodynamic stress is a crucial factor responsible for aneurysm formation, especially at arterial junctions and bifurcations. Several studies have proposed that the hemodynamic stress on the arterial wall initiates inflammatory processes that significantly weaken the arterial wall and ultimately lead to rupture (Ziu, 2022). While several cytokines (e.g., interleukin-6) and metalloproteinases (MMPs) have been extensively studied for their role in intracranial aneurysmal formation and rupture (Sathyan et al., 2015; Zhang et al., 2019; Kaminska et al., 2022; Lucke-Wold et al., 2022), the role of the microbiome in aneurysm formation and rupture has only been appreciated in the past 3 years. However, the mechanisms through which the microbiome impact aneurysm formation and rupture remain unclear.
In this mini-review, we explore new insights into the effects of the microbiome on intracranial aneurysm formation and rupture. Since diet has been shown to influence the composition of the microbiota (Leeming et al., 2019), we examined studies demonstrating the influence of diet on intracranial aneurysm formation. Finally, we discuss relevant therapeutic targets of inflammation associated with intracranial aneurysm formation and rupture.
A role for the microbiota-gut-brain axis in cerebrovascular hemorrhage
Over the last decade, our knowledge and understanding of the human microbiome and its link to various systems and pathologies has increased. The microbiota-gut-brain (MGB) axis is comprised of an intricate system of neural and immunological pathways with signaling molecules such as cytokines, hormones, neuropeptides, and bacterial metabolites that are believed to be used as communication between the microbiota, gut, and brain (Arpaia et al., 2013; Benakis et al., 2016; Chen et al., 2017). In particular, the MGB axis is thought to contribute to stroke outcome, as disruption of the gut vascular barrier (GVB) results in increased intestinal permeability and enables gut microbes to enter the bloodstream in animal models (Crapser et al., 2016; Chen et al., 2019; Lee et al., 2020). There is a paucity of literature concerning how the microbiome may influence intracranial aneurysm formation and rupture; thus, in this section, we briefly discuss recent findings demonstrating the role of the microbiome in several brain hemorrhagic conditions.
It has been suggested that commensal gut microbiota protects against ischemic stroke pathology and that their depletion leads to increased mortality and poor outcome, including worse neurological function (Singh et al., 2016; Winek et al., 2016). These findings become relevant given that SAH is a type of hemorrhagic stroke and has also been shown to cause delayed ischemic stroke (Martin and Rymer, 2011; Guresir et al., 2022). Compared to ischemic stroke not secondary to SAH, less is known about the association between SAH and the gut microbiome. A proof-of-concept study showed that antibiotic (vancomycin, metronidazole, ampicillin, and neomycin)-depletion of the gut microbiota in mice reduced the formation of intracranial aneurysms and neuroinflammation (Shikata et al., 2019). However, a significant limitation of the Shikata et al. (2019) study is the lack of clarity on which microbes are beneficial or harmful to aneurysm formation, given that the depletion of the gut microbiota with antibiotics represents a highly artificial scenario. Likewise, non-specific antimicrobial targeting of the gut microbiome may do more harm than good (Tang et al., 2017).
Two recent studies have addressed the contributions of specific microbes with regard to intracranial aneurysm formation and rupture. Notably, a prospective case-control microbiome study comparing the microbiome profile of patients with unruptured intracranial aneurysms (UIA) and ruptured intracranial aneurysms (RIA) demonstrated a significant increase in the relative abundance of Campylobacter at the phylum level in the RIA group compared to the UIA group. Further classification via 16S rRNA polymerase chain reaction at the genus level revealed that Campylobacter and Campylobacter ureolyticus were increased in the RIA group compared to the UIA group (Kawabata et al., 2022). Despite the intriguing findings from the Kawabata et al. study, some limitations exist. First, the composition of the gut microbiome prior to aneurysm rupture was not assessed. Second, the study did not collect patient dietary information, which could influence the gut microbiome. Finally, the study was conducted in a single country.
Microbes such as Hungatella hathewayi have also been implicated in intracranial aneurysm formation and rupture. In a case-control metagenome-wide associated study, Li et al. demonstrated that fecal transplant from UIA and control human patients to mice recipients was sufficient to induce UIAs in mice. The authors revealed that Hungatella hathewayi abundance was decreased in both human and mice UIAs and that the decrease in Hungatella hathewayi correlated to low levels of taurine, an anti-inflammatory metabolite known to be protective in stroke, traumatic brain injury, and SAH. Additionally, mice gavaged with Hungatella hathewayi were protected against intracranial formation and rupture (Menzie et al., 2013; Su et al., 2014; Jin et al., 2018; Li et al., 2020). While the Li et al. (2020) study demonstrated a direct causal effect of microbes on intracranial aneurysm formation and rupture, the study was performed in a single country, and it is unclear how Hungatella hathewayi works in concert with other microbes in decreasing aneurysm formation and rupture via modulation of taurine levels. Table 1 briefly summarizes the findings from the aforementioned SAH studies.
Like SAH, recent studies have suggested a role for microbes in intracerebral hemorrhage (ICH). A preclinical study observed that members of Nocardiaceae, Helicobacteraceae, Veillonellaceae, Bacteroidaceae, and Akkermansiaceae significantly increased ICH, while Firmicutes, Barnesiellaceae, Bacteriidales, and Moraxellaceae were reduced (Yu et al., 2021). Another study showed that compared to the sham group, experimental ICH depleted members of Firmicutes, such as Faecalibaculum and Dubosiella. In contrast, the members of Proteobacteria and Campilobacterota, such as Enterobacter and Helicobacter, were enriched (Xiao et al., 2022). Analysis of murine ileum tissue revealed the phylum Proteobacteria, the class Gammaproteobacteria, the order Enterobacteriales, the family Enterobacteriaceae, and the species Escherichia coli were higher in the gut microbiota early (1 day) following ICH, while by day 3 had a greater abundance of the family Peptostreptococcaceae and genus Romboutsia (Zhang et al., 2021). Furthermore, higher levels of gut microbiota metabolite short-chain fatty acids (SCFAs) and equol have been associated with a better outcome. In comparison, higher levels of trimethylamine-N-oxide (TMAO) have been associated with worse outcomes in cardiovascular disease, cerebrovascular disease, and intracranial aneurysm/hemorrhage (Wang et al., 2011; Tonomura and Gyanwali, 2021; Yokosuka et al., 2021; Zhai et al., 2021).
Many simultaneous pathways likely contribute to the interplay between hemorrhagic brain conditions and the MGB axis; these are considerable targets for therapeutic intervention. One such pathway is the NOD-like receptor family, pyrin domain-containing 3 (NLRP3) inflammasome. Administration of the selective NLRP3 inflammasome inhibitor, MCC950, modulated gut microbiota dysbiosis, increased the abundance of Bacteroides, Bifidobacterium, and Paenibacillus, and attenuated corticospinal tract injury and neurological deficits following experimental ICH (Xiao et al., 2022). Additionally, increased intestinal permeability was observed in mice with ICH compared to sham, and ICH mice exhibited worse intestinal motility and pathology (Yu et al., 2021; Zhang et al., 2021). Another potential pathway is the disruption of T-cell homeostasis. Yu et al. demonstrated T-cell accumulation in the brain following ICH, some of which had migrated from the intestine to the peri-hematoma region. Importantly, the recolonization of ICH mice with healthy microbiota through a fecal microbiota transplant ameliorates these deficits (Yu et al., 2021).
Cavernous angiomas (CAs), common vascular anomalies predisposing to brain hemorrhage characterized by dysmorphic dilated vascular capillaries lined by endothelium, have also been shown to affect the gut microbiome. A recent study showed that CA patients' microbiota were significantly enriched in lipopolysaccharide (LPS)-producing Gram-negative bacteria. This study identified five significantly contributive taxa, Bifidobacterium adolescentis, Bacteroides eggerthii, Bacteroides dorei, Dorea, and Escherichia coli, to distinguish aggressive from non-aggressive CA patients. Meanwhile, six taxa, including Faecalibacterium prausnitzii, Oscillobacter, Lactobacillus rhamnosus, Enterobacter cloacae, Odoribacter laneus, and Bacteroides cellulosilyticus were identified to be unique microbiome signatures of CA patients with symptomatic hemorrhage (Polster et al., 2020).
Despite studies providing evidence that microbial by-products modulate vascular inflammation; and thus contribute to the formation or rupture of intracranial aneurysms. It is unclear whether the direct transmigration of gut microbe into cerebral vessels represents a potential mechanism responsible for intracranial aneurysm formation and rupture. Furthermore, future longitudinal human studies are needed to assess the microbiome composition in patients at high risk for intracranial aneurysms, as this could provide insight into understanding the cause-effect relationship between the gut microbiome and intracranial aneurysms.
The influence of diet on aneurysm formation and rupture
A myriad of dietary metabolites influence the risk of SAH formation and rupture. Inadequate intake of dietary antioxidants, hyperhomocysteinemia, hypertension, and alcohol consumption may increase the risk of intracranial aneurysms. At-risk individuals should consider diets that provide sufficient amounts of antioxidant vitamins, B vitamins, flavonoids, and n-3 fatty acids while limiting alcohol and caffeine consumption. Healthy diets such as the Mediterranean diet or Dietary Approach to Stop Hypertension (DASH) diet promote high consumption of vegetables, fruits, legumes, whole grains, nuts, fish, and herbs, which provide those necessary nutrients listed above, and are thus recommended for the prevention of intracranial aneurysms (Czekajlo, 2019; Cao et al., 2021). Interestingly, adherence to a DASH diet was inversely associated with the risk of ischemic stroke and ICH, but not SAH (Larsson et al., 2016).
Vitamin E supplementation in cholesterol-treated rabbits decreased the degree of vasospasm following basilar artery SAH (Sasani et al., 2011). This is contrary to an earlier clinical trial, which showed that vitamin E (dl-alpha-tocopherol) supplementation in hypertensive men decreased the risk of cerebral infarction but increased the risk of SAH; however, this effect was observed in hypertensive subjects (Leppala et al., 2000). Given this disparity in results, future clinical trials are needed to elucidate the role of vitamin E in SAH pathophysiology. Polyphenols, plant secondary metabolites in foods such as fruits, vegetables, beverages including tea and red wine, and extra virgin oil, are beneficial in stroke (Parrella et al., 2020). Cadmium, a toxic metal associated with several adverse health effects, exposure occurs mainly from the diet (whole grain rice and potatoes) and tobacco smoke. Cadmium's excretion is very slow, with a half-life of decades, and this measure has been positively associated with stroke, myocardial infarction, and abdominal aortic aneurysm. Cadmium levels measured in the blood of the Malmo Diet and Cancer cohort (93 SAH cases vs. 276 matched controls) revealed patients with the highest cadmium levels had an increased risk of SAH, which was primarily due to smoking (Soderholm et al., 2020). A major limitation of the Soderholm et al. (2020) study was the low number of SAH cases. Although a few case reports have implicated the use of dietary supplements such as ginkgo biloba and spontaneous cerebral hemorrhage (e.g., ICH, SAH, and SDH), more studies are needed to fully establish this risk (Rosenblatt and Mindel, 1997; Benjamin et al., 2001; Friedman et al., 2007; Stanger et al., 2012).
High-fat diet in rats promotes increased serum cholesterol and facilitates enlargement and degenerative changes in the media of intracranial aneurysms, presumably through foam cell transformation (Shimizu et al., 2019). The population-based case-control Australasian Cooperative Research on Subarachnoid Hemorrhage Study (ACROSS), consisting of 432 first-ever cases of SAH vs. 473 controls, found frequent fat intake was associated with SAH risk. In contrast, frequent skim or reduced-fat milk and fruit intake were protective against SAH (Shiue et al., 2011). In comparison, the Japan Public Health Center-based prospective (JPHC) Study found that dietary saturated fatty acids (SFAs) were inversely associated with deep intraparenchymal hemorrhage and lacunar infarction but had no association with SAH (Yamagishi et al., 2013). Interestingly, pretreatment with omega-3 fatty acids protected against SAH in rats via the G protein-coupled receptor 120/β-arrestin2/TGF-β activated kinase-1 binding protein-1 anti-inflammatory signaling pathway, suggesting that fish oil supplementation as part of a daily diet may contribute to improved clinical outcomes in SAH patients (Yin et al., 2016).
The European Prospective Investigation into Cancer and Nutrition (EPIC)-Oxford cohort showed that while fish eaters and vegetarians had lower rates of ischemic heart disease, vegetarians had higher rates of hemorrhagic and total stroke (i.e., ischemic and hemorrhagic stroke) (Tong et al., 2019). Data from two prospective population-based cohorts of Swedish women and men showed that while total fruit and vegetable consumption was significantly inversely associated with total stroke, SAH alone was not (Larsson et al., 2013). Another study from the Danish Diet, Cancer, and Health cohort found that adherence to the EAT-Lancet diet, a sustainable, and primarily plant-based diet, was associated with a lower risk of SAH, while the Alternate Healthy Eating Index-2010 (AHEI) was associated with a lower risk of ischemic stroke (Ibsen et al., 2022).
While several population studies have demonstrated the role of diet in the pathogenesis of intracranial aneurysms, it remains unclear how diet indirectly alters the gut microbiome composition in a way that could promote or mitigate intracranial aneurysm formation. Likewise, future studies examining mechanistic pathways on how specific diet components promote or mitigate intracranial aneurysm formation are needed.
Neuroinflammation as a therapeutic target in subarachnoid hemorrhage
The neuroinflammatory response to acute central nervous system (CNS) insult involves a multimodal activation of peripheral and central immune cells and mediators. This inflammatory cascade is a critical pathologic force in the development and progression of intracranial aneurysms, in addition to acute and chronic consequences of SAH. Furthermore, recent studies suggest that gut microbes promote intracranial aneurysm formation and rupture via modulation of vascular inflammation; thus, targeting vascular inflammation may be therapeutically beneficial in SAH management (Shikata et al., 2019; Li et al., 2020; Kawabata et al., 2022).
Inflammatory factors in the development, growth, and rupture of intracranial aneurysms
Endothelial injury, a core process in aneurysm pathogenesis, invokes a coordinated inflammatory response, first through increased proinflammatory (i.e., COX2-PGE2-EP2-NFkB) signaling and subsequently through the infiltration of inflammatory cells (Aoki et al., 2011; Chalouhi et al., 2012). The resultant “inflammatory zone,” consisting of macrophages, T-cells, mast cells, and cytokines, causes vascular smooth muscle cells (vSMCs) within the media to adopt a proinflammatory matrix remodeling phenotype. TNF-alpha contributes to the reprogramming of normal contractile vSMCs to a pathologic phenotype resulting in myointimal hyperplasia (Kosierkiewicz et al., 1994; Ali et al., 2013). These vSMCs exhibit reduced expression of contractile proteins and production of inflammatory factors and matrix metalloproteases (MMPs) (Starke et al., 2014). MMPs produced by vSMCs and macrophages incite damage against the vessel wall, disrupting the internal elastic lamina (IEL) and extracellular matrix (Texakalidis et al., 2019). Disruption of the IEL by this inflammatory cascade appears to be a critical event in aneurysm formation (Kim et al., 1993). The aneurysm's continued growth depends on a complex, coordinated degenerative and remodeling process (Frosen et al., 2019). Rupture results from the continued weakening of the wall, specifically the replacement of collagen and muscular layers with a hyaline-like substance (Kataoka et al., 1999). Furthermore, it appears that a shift in vSMCs from the proinflammatory phenotype to apoptosis and subsequent thinning of the media is a late event preceding aneurysm rupture (Guo et al., 2007).
Acute and sub-acute neuroinflammatory response to subarachnoid hemorrhage
Inflammation, edema, ischemia, and cell death, are the primary pathologic processes underlying early brain injury (0–72 h) following subarachnoid hemorrhage. The initial rapid accumulation of blood in the subarachnoid space—most often resulting from aneurysmal rupture—results in an immediate rise in intracranial pressure and a corresponding reduction in cerebral blood flow (Conzen et al., 2019). The consequent parenchymal metabolic dysfunction and neuronal apoptosis/necrosis incite microglia, the resident immune cells of the CNS, to become activated in regions closest to the bleeding (Provencio et al., 2011). The binding of pathogen- or damage-associated molecular patterns (PAMPs/DAMPs), such as heme from lysed erythrocytes to Toll-like receptors (TLRs), including TLR2 and TLR4, is implicated as the cellular mechanism underlying microglial activation (Hanafy, 2013; Xu et al., 2021; Islam et al., 2022). During the acute period, microglia are thought to adopt an M1 polarization characterized by neurotoxicity (Li et al., 2018). This drives further neuronal death, blood-brain barrier permeability, and recruitment of peripheral inflammatory cells (Schneider et al., 2015). Infiltration of neutrophils, the most abundant of the circulating leukocytes, is considered a hallmark of post-SAH inflammation which drives early tissue damage and neuronal death. Indeed, neutrophil accumulation within the cerebral vasculature and parenchyma has been identified as early as 10 min following hemorrhage and peaks between 3–4 days following (Friedrich et al., 2011; Atangana et al., 2017). Experimental preclinical studies utilizing the depletion of neutrophils have determined that early infiltration is responsible for microvascular injury and resultant local ischemia, as well as aggravation of the inflammatory response and neuronal death (Friedrich et al., 2011; Atangana et al., 2017; Neulen et al., 2019).
Delayed neuroinflammatory response to subarachnoid hemorrhage
Persistent neuroinflammatory changes following subarachnoid hemorrhage have been identified; however, little is known about their pathophysiologic implications. Notably, microglial polarization appears to shift from the proinflammatory, neurotoxic (M1) to anti-inflammatory, neuroprotective (M2) phenotype. TNF-stimulated gene-6 (TSG-6), a glycoprotein that comprises the negative feedback loop which tempers inflammatory progression, has emerged as one molecular mediator of this transition (Li et al., 2018). Vasospasm and cerebral ischemia are clinical hallmarks of the delayed period following subarachnoid hemorrhage and represent the primary cause of morbidity and mortality. Irritation of the cerebral vessels by blood breakdown products like hemoglobin has been the classical hypothesis of delayed cerebral ischemia. However, the failure of agents targeting vasospasm to mitigate neurological deterioration suggests that microvascular injury, thrombosis, and inflammation may be more pervasive processes (Dodd et al., 2021). Indeed, monoclonal antibody-mediated neutralization of secreted HMGB1 was shown to attenuate microglial activity, ameliorate the vasocontractile response to thrombin, and improve locomotor recovery (Haruma et al., 2016). Consistent with this, elevated HMGB1 in cerebrospinal fluid correlated with levels of inflammatory cytokines and was associated with unfavorable neurological outcomes (Nakahara et al., 2009). These specific examples support the generally well-appreciated relationship between heightened systemic immune response, delayed ischemia, and poor neurological outcome (Al-Mufti et al., 2019; Ma et al., 2021).
Considerations for therapeutic targeting of neuroinflammation in subarachnoid hemorrhage
The pervasiveness of inflammatory processes in multiple phases of subarachnoid hemorrhage pathophysiology renders them clear targets for future therapeutics. However, successful targeting will depend on coordinating the cellular target and appropriate timing of intervention. For example, the administration of pharmacological agents that favor the anti-inflammatory (M2) phenotype early after hemorrhage may moderate microglial activation and polarization (Schneider et al., 2015). Additionally, the unintended effects of manipulating the inflammatory response must be considered. While induction of neutropenia mitigates peripheral inflammatory infiltration and subsequent microvascular injury, agents used to induce the neutropenic state can predispose to systemic infection or impair coagulative responses (Friedrich et al., 2011). This is borne out in clinical literature where utilization of corticosteroids to modulate inflammation has demonstrated no benefit at best and potential risk for harm (i.e., infection, hyperglycemia) at worst (Feigin et al., 2005; Czorlich et al., 2017).
Conclusion
While we broadly understand the role of inflammatory cytokines and other risk factors (e.g., hypertension, smoking, etc.) on intracranial aneurysm formation and rupture, further insights on the role of the microbiome and its interplay in the pathways as mentioned above with regards to intracranial aneurysm formation and rupture remains to be elucidated. The recent microbiome studies discussed in this review and the findings summarized in Figure 1 emphasize the importance of the gut microbiome intracranial formation and rupture. Further studies are needed to delineate the effects of specific microbes on intracranial aneurysm formation and rupture. Likewise, a functional understanding of how microbes work in concert to modulate intracranial aneurysm formation and rupture is needed. These studies will rely heavily on developing new preclinical models and tools that accurately resemble the human clinical progression of intracranial aneurysm rupture and allow for the detection of aneurysms prior to euthanasia.
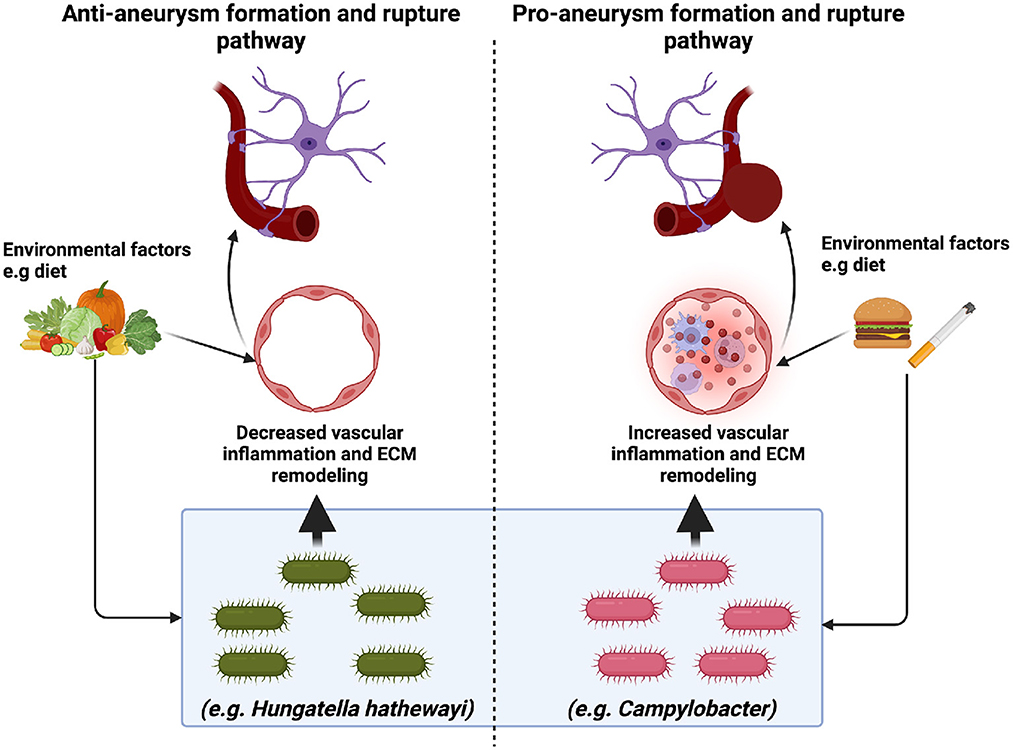
Figure 1. The implications of the microbiome in intracranial aneurysm formation and rupture. Recent studies suggest that specific microbes may promote (Campylobacter) or mitigate (H. hathewayi) intracranial aneurysm formation and rupture. Pro-aneurysm microbes are thought to induce vascular inflammation (immune cell infiltration and extracellular matrix (ECM) remodeling). In contrast, anti-aneurysm microbes are thought to decrease vascular inflammation via anti-inflammatory metabolites such as taurine. While it is clear that environmental factors such as diet may alter the gut microbiome, the mechanism through which this occurs is unclear. Future studies are needed to elucidate further the role of diet and the gut microbiome in intracranial aneurysm formation and rupture.
Author contributions
All authors listed have made a substantial, direct, and intellectual contribution to the work and approved it for publication.
Conflict of interest
The authors declare that the research was conducted in the absence of any commercial or financial relationships that could be construed as a potential conflict of interest.
Publisher's note
All claims expressed in this article are solely those of the authors and do not necessarily represent those of their affiliated organizations, or those of the publisher, the editors and the reviewers. Any product that may be evaluated in this article, or claim that may be made by its manufacturer, is not guaranteed or endorsed by the publisher.
References
Ali, M.S., Starke, R.M., Jabbour, P.M., Tjoumakaris, S.I., Gonzalez, L.F., Rosenwasser, R.H., et al. (2013). TNF-alpha induces phenotypic modulation in cerebral vascular smooth muscle cells: implications for cerebral aneurysm pathology. J. Cereb. Blood Flow Metab. 33, 1564–1573. doi: 10.1038/jcbfm.2013.109
Al-Mufti, F., Misiolek, K.A., Roh, D., Alawi, A., Bauerschmidt, A., Park, S., et al. (2019). White blood cell count improves prediction of delayed cerebral ischemia following aneurysmal subarachnoid hemorrhage. Neurosurgery 84, 397–403. doi: 10.1093/neuros/nyy045
Aoki, T., Nishimura, M., Matsuoka, T., Yamamoto, K., Furuyashiki, T., Kataoka, H., et al. (2011). PGE(2) -EP(2) signalling in endothelium is activated by haemodynamic stress and induces cerebral aneurysm through an amplifying loop via NF-kappaB. Br. J. Pharmacol. 163, 1237–1249. doi: 10.1111/j.1476-5381.2011.01358.x
Arpaia, N., Campbell, C., Fan, X., Dikiy, S., van der Veeken, J., deRoos, P., et al. (2013). Metabolites produced by commensal bacteria promote peripheral regulatory T-cell generation. Nature 504, 451–455. doi: 10.1038/nature12726
Atangana, E., Schneider, U.C., Blecharz, K., Magrini, S., Wagner, J., Nieminen-Kelha, M., et al. (2017). Intravascular inflammation triggers intracerebral activated microglia and contributes to secondary brain injury after experimental subarachnoid hemorrhage (eSAH). Transl. Stroke Res 8, 144–156. doi: 10.1007/s12975-016-0485-3
Benakis, C., Brea, D., Caballero, S., Faraco, G., Moore, J., Murphy, M., et al. (2016). Commensal microbiota affects ischemic stroke outcome by regulating intestinal gammadelta T cells. Nat. Med. 22, 516–523. doi: 10.1038/nm.4068
Benjamin, J., Muir, T., Briggs, K., and Pentland, B. (2001). A case of cerebral haemorrhage-can Ginkgo biloba be implicated? Postgrad. Med. J. 77, 112–113. doi: 10.1136/pmj.77.904.112
Cao, Z., Li, S., Yang, H., Xu, C., Zhang, Y., Yang, X., et al. (2021). Associations of behaviors, biological phenotypes and cardiovascular health with risks of stroke and stroke subtypes: A prospective cohort study. EClinicalMedicine 33, 100791. doi: 10.1016/j.eclinm.2021.100791
Chalouhi, N., Ali, M.S., Jabbour, P.M., Tjoumakaris, S.I., Gonzalez, L.F., Rosenwasser, R.H., et al. (2012). Biology of intracranial aneurysms: role of inflammation. J. Cereb. Blood Flow Metab. 32, 1659–1676. doi: 10.1038/jcbfm.2012.84
Chen, R., Wu, P., Cai, Z., Fang, Y., Zhou, H., Lasanajak, Y., et al. (2019). Puerariae Lobatae Radix with chuanxiong Rhizoma for treatment of cerebral ischemic stroke by remodeling gut microbiota to regulate the brain-gut barriers. J. Nutr. Biochem. 65, 101–114. doi: 10.1016/j.jnutbio.2018.12.004
Chen, Z., Venkat, P., Seyfried, D., Chopp, M., Yan, T., and Chen, J. (2017). Brain-heart interaction: cardiac complications after stroke. Circ. Res. 121, 451–468. doi: 10.1161/CIRCRESAHA.117.311170
Conzen, C., Becker, K., Albanna, W., Weiss, M., Bach, A., Lushina, N., et al. (2019). The acute phase of experimental subarachnoid hemorrhage: intracranial pressure dynamics and their effect on cerebral blood flow and autoregulation. Transl. Stroke Res. 10, 566–582. doi: 10.1007/s12975-018-0674-3
Crapser, J., Ritzel, R., Verma, R., Venna, V.R., Liu, F., Chauhan, A., et al. (2016). Ischemic stroke induces gut permeability and enhances bacterial translocation leading to sepsis in aged mice. Aging (Albany NY) 8, 1049–1063. doi: 10.18632/aging.100952
Czekajlo, A. (2019). Role of diet-related factors in cerebral aneurysm formation and rupture. Rocz. Panstw. Zakl. Hig. 70, 119–126. doi: 10.32394/rpzh.2019.0061
Czorlich, P., Sauvigny, T., Ricklefs, F., Abboud, T., Nierhaus, A., Vettorazzi, E., et al. (2017). Impact of dexamethasone in patients with aneurysmal subarachnoid haemorrhage. Eur. J. Neurol. 24, 645–651. doi: 10.1111/ene.13265
de Rooij, N.K., Linn, F.H., van der Plas, J.A., Algra, A., and Rinkel, G.J. (2007). Incidence of subarachnoid haemorrhage: a systematic review with emphasis on region, age, gender and time trends. J. Neurol. Neurosurg. Psychiatry 78, 1365–1372. doi: 10.1136/jnnp.2007.117655
Dodd, W.S., Laurent, D., Dumont, A.S., Hasan, D.M., Jabbour, P.M., Starke, R.M., et al. (2021). Pathophysiology of delayed cerebral ischemia after subarachnoid hemorrhage: a review. J. Am. Heart Assoc. 10, e021845. doi: 10.1161/JAHA.121.021845
Feigin, V.L., Anderson, N., Rinkel, G.J., Algra, A., van Gijn, J., and Bennett, D.A. (2005). Corticosteroids for aneurysmal subarachnoid haemorrhage and primary intracerebral haemorrhage. Cochrane Datab. Syst. Rev. 2005, CD004583. doi: 10.1002/14651858.CD004583.pub2
Friedman, J.A., Taylor, S.A., McDermott, W., and Alikhani, P. (2007). Multifocal and recurrent subarachnoid hemorrhage due to an herbal supplement containing natural coumarins. Neurocrit. Care 7, 76–80. doi: 10.1007/s12028-007-0075-z
Friedrich, V., Flores, R., Muller, A., Bi, W., Peerschke, E.I., and Sehba, F.A. (2011). Reduction of neutrophil activity decreases early microvascular injury after subarachnoid haemorrhage. J. Neuroinflammation 8, 103. doi: 10.1186/1742-2094-8-103
Frosen, J., Cebral, J., Robertson, A.M., and Aoki, T. (2019). Flow-induced, inflammation-mediated arterial wall remodeling in the formation and progression of intracranial aneurysms. Neurosurg. Focus 47, E21. doi: 10.3171/2019.5.FOCUS19234
Guo, F., Li, Z., Song, L., Han, T., Feng, Q., Guo, Y., et al. (2007). Increased apoptosis and cysteinyl aspartate specific protease-3 gene expression in human intracranial aneurysm. J. Clin. Neurosci. 14, 550–555. doi: 10.1016/j.jocn.2005.11.018
Guresir, E., Welchowski, T., Lampmann, T., Brandecker, S., Guresir, A., Wach, J., et al. (2022). Delayed cerebral ischemia after aneurysmal subarachnoid hemorrhage: the results of induced hypertension only after the IMCVS trial-a prospective cohort study. J. Clin. Med. 11, 5850. doi: 10.3390/jcm11195850
Hanafy, K.A. (2013). The role of microglia and the TLR4 pathway in neuronal apoptosis and vasospasm after subarachnoid hemorrhage. J. Neuroinflammation 10, 83. doi: 10.1186/1742-2094-10-83
Haruma, J., Teshigawara, K., Hishikawa, T., Wang, D., Liu, K., Wake, H., et al. (2016). Anti-high mobility group box-1 (HMGB1) antibody attenuates delayed cerebral vasospasm and brain injury after subarachnoid hemorrhage in rats. Sci. Rep. 6, 37755. doi: 10.1038/srep37755
Ibsen, D.B., Christiansen, A.H., Olsen, A., Tjonneland, A., Overvad, K., Wolk, A., et al. (2022). Adherence to the EAT-lancet diet and risk of stroke and stroke subtypes: a cohort study. Stroke 53, 154–163. doi: 10.1161/STROKEAHA.121.036738
Ikawa, F., Michihata, N., Matsushige, T., Abiko, M., Ishii, D., Oshita, J., et al. (2020). In-hospital mortality and poor outcome after surgical clipping and endovascular coiling for aneurysmal subarachnoid hemorrhage using nationwide databases: a systematic review and meta-analysis. Neurosurg. Rev. 43, 655–667. doi: 10.1007/s10143-019-01096-2
Islam, R., Vrionis, F., and Hanafy, K.A. (2022). Microglial TLR4 is critical for neuronal injury and cognitive dysfunction in subarachnoid hemorrhage. Neurocrit. Care. 37, 761–769. doi: 10.1007/s12028-022-01552-w
Jin, R., Xiao, A.Y., Liu, S., Wang, M., and Li, G. (2018). Taurine reduces tPA (Tissue-Type Plasminogen Activator)-induced hemorrhage and microvascular thrombosis after embolic stroke in rat. Stroke 49, 1708–1718. doi: 10.1161/STROKEAHA.118.020747
Kaminska, J., Maciejczyk, M., Cwiklinska, A., Matowicka-Karna, J., and Koper-Lenkiewicz, O.M. (2022). Pro-inflammatory and anti-inflammatory cytokines levels are significantly altered in cerebrospinal fluid of unruptured intracranial aneurysm (UIA) patients. J. Inflamm. Res. 15, 6245–6261. doi: 10.2147/JIR.S380524
Kataoka, K., Taneda, M., Asai, T., Kinoshita, A., Ito, M., and Kuroda, R. (1999). Structural fragility and inflammatory response of ruptured cerebral aneurysms. A comparative study between ruptured and unruptured cerebral aneurysms. Stroke 30, 1396–1401. doi: 10.1161/01.STR.30.7.1396
Kawabata, S., Takagaki, M., Nakamura, H., Oki, H., Motooka, D., Nakamura, S., et al. (2022). Dysbiosis of gut microbiome is associated with rupture of cerebral aneurysms. Stroke 53, 895–903. doi: 10.1161/STROKEAHA.121.034792
Kim, C., Cervos-Navarro, J., Kikuchi, H., Hashimoto, N., and Hazama, F. (1993). Degenerative changes in the internal elastic lamina relating to the development of saccular cerebral aneurysms in rats. Acta Neurochir. (Wien) 121, 76–81. doi: 10.1007/BF01405187
Kosierkiewicz, T.A., Factor, S.M., and Dickson, D.W. (1994). Immunocytochemical studies of atherosclerotic lesions of cerebral berry aneurysms. J. Neuropathol. Exp. Neurol. 53, 399–406. doi: 10.1097/00005072-199407000-00012
Larsson, S.C., Virtamo, J., and Wolk, A. (2013). Total and specific fruit and vegetable consumption and risk of stroke: a prospective study. Atherosclerosis 227, 147–152. doi: 10.1016/j.atherosclerosis.2012.12.022
Larsson, S.C., Wallin, A., and Wolk, A. (2016). Dietary approaches to stop hypertension diet and incidence of stroke: results from 2 prospective cohorts. Stroke 47, 986–990. doi: 10.1161/STROKEAHA.116.012675
Lee, J., d'Aigle, J., Atadja, L., Quaicoe, V., Honarpisheh, P., Ganesh, B.P., et al. (2020). Gut microbiota-derived short-chain fatty acids promote poststroke recovery in aged mice. Circ. Res. 127, 453–465. doi: 10.1161/CIRCRESAHA.119.316448
Leeming, E.R., Johnson, A.J., Spector, T.D., and Le Roy, C.I. (2019). Effect of diet on the gut microbiota: rethinking intervention duration. Nutrients 11, 2862. doi: 10.3390/nu11122862
Leppala, J.M., Virtamo, J., Fogelholm, R., Albanes, D., Taylor, P.R., and Heinonen, O.P. (2000). Vitamin E and beta carotene supplementation in high risk for stroke: a subgroup analysis of the Alpha-Tocopherol, Beta-Carotene Cancer Prevention Study. Arch. Neurol. 57, 1503–1509. doi: 10.1001/archneur.57.10.1503
Li, H., Xu, H., Li, Y., Jiang, Y., Hu, Y., Liu, T., et al. (2020). Alterations of gut microbiota contribute to the progression of unruptured intracranial aneurysms. Nat. Commun. 11, 3218. doi: 10.1038/s41467-020-16990-3
Li, R., Liu, W., Yin, J., Chen, Y., Guo, S., Fan, H., et al. (2018). TSG-6 attenuates inflammation-induced brain injury via modulation of microglial polarization in SAH rats through the SOCS3/STAT3 pathway. J. Neuroinflammation 15, 231. doi: 10.1186/s12974-018-1279-1
Lucke-Wold, B., Dodd, W., Motwani, K., Hosaka, K., Laurent, D., Martinez, M., et al. (2022). Investigation and modulation of interleukin-6 following subarachnoid hemorrhage: targeting inflammatory activation for cerebral vasospasm. J. Neuroinflammation 19, 228. doi: 10.1186/s12974-022-02592-x
Ma, X., Lan, F., and Zhang, Y. (2021). Associations between C-reactive protein and white blood cell count, occurrence of delayed cerebral ischemia and poor outcome following aneurysmal subarachnoid hemorrhage: a systematic review and meta-analysis. Acta Neurol. Belg. 121, 1311–1324. doi: 10.1007/s13760-020-01496-y
Martin, C.O., and Rymer, M.M. (2011). Hemorrhagic stroke: aneurysmal subarachnoid hemorrhage. Mo. Med. 108, 124–127.
Menzie, J., Prentice, H., and Wu, J.Y. (2013). Neuroprotective mechanisms of taurine against ischemic stroke. Brain Sci. 3, 877–907. doi: 10.3390/brainsci3020877
Nakahara, T., Tsuruta, R., Kaneko, T., Yamashita, S., Fujita, M., Kasaoka, S., et al. (2009). High-mobility group box 1 protein in CSF of patients with subarachnoid hemorrhage. Neurocrit. Care 11, 362–368. doi: 10.1007/s12028-009-9276-y
Neulen, A., Pantel, T., Kosterhon, M., Kramer, A., Kunath, S., Petermeyer, M., et al. (2019). Neutrophils mediate early cerebral cortical hypoperfusion in a murine model of subarachnoid haemorrhage. Sci. Rep. 9, 8460. doi: 10.1038/s41598-019-44906-9
Parrella, E., Gussago, C., Porrini, V., Benarese, M., and Pizzi, M. (2020). From preclinical stroke models to humans: polyphenols in the prevention and treatment of stroke. Nutrients 13, 85. doi: 10.3390/nu13010085
Polster, S.P., Sharma, A., Tanes, C., Tang, A.T., Mericko, P., Cao, Y., et al. (2020). Permissive microbiome characterizes human subjects with a neurovascular disease cavernous angioma. Nat. Commun. 11, 2659. doi: 10.1038/s41467-020-16436-w
Provencio, J.J., Altay, T., Smithason, S., Moore, S.K., and Ransohoff, R.M. (2011). Depletion of Ly6G/C(+) cells ameliorates delayed cerebral vasospasm in subarachnoid hemorrhage. J. Neuroimmunol. 232, 94–100. doi: 10.1016/j.jneuroim.2010.10.016
Rosenblatt, M., and Mindel, J. (1997). Spontaneous hyphema associated with ingestion of Ginkgo biloba extract. N. Engl. J. Med. 336, 1108. doi: 10.1056/NEJM199704103361518
Sasani, M., Yazgan, B., Celebi, I., Aytan, N., Catalgol, B., Oktenoglu, T., et al. (2011). Hypercholesterolemia increases vasospasm resulting from basilar artery subarachnoid hemorrhage in rabbits which is attenuated by Vitamin E. Surg. Neurol. Int. 2, 29. doi: 10.4103/2152-7806.77600
Sathyan, S., Koshy, L.V., Srinivas, L., Easwer, H.V., Premkumar, S., Nair, S., et al. (2015). Pathogenesis of intracranial aneurysm is mediated by proinflammatory cytokine TNFA and IFNG and through stochastic regulation of IL10 and TGFB1 by comorbid factors. J. Neuroinflammation 12, 135. doi: 10.1186/s12974-015-0383-8
Schneider, U.C., Davids, A.M., Brandenburg, S., Muller, A., Elke, A., Magrini, S., et al. (2015). Microglia inflict delayed brain injury after subarachnoid hemorrhage. Acta Neuropathol. 130, 215–231. doi: 10.1007/s00401-015-1440-1
Shikata, F., Shimada, K., Sato, H., Ikedo, T., Kuwabara, A., Furukawa, H., et al. (2019). Potential influences of gut microbiota on the formation of intracranial aneurysm. Hypertension 73, 491–496. doi: 10.1161/HYPERTENSIONAHA.118.11804
Shimizu, K., Miyata, H., Abekura, Y., Oka, M., Kushamae, M., Kawamata, T., et al. (2019). High-fat diet intake promotes the enlargement and degenerative changes in the media of intracranial aneurysms in rats. J. Neuropathol. Exp. Neurol. 78, 798–807. doi: 10.1093/jnen/nlz057
Shiue, I., Arima, H., Hankey, G.J., and Anderson, C.S. (2011). Dietary intake of key nutrients and subarachnoid hemorrhage: a population-based case-control study in Australasia. Cerebrovasc Dis. 31, 464–470. doi: 10.1159/000324386
Singh, V., Roth, S., Llovera, G., Sadler, R., Garzetti, D., Stecher, B., et al. (2016). Microbiota dysbiosis controls the neuroinflammatory response after stroke. J. Neurosci. 36, 7428–7440. doi: 10.1523/JNEUROSCI.1114-16.2016
Soderholm, M., Borne, Y., Hedblad, B., Persson, M., Barregard, L., and Engstrom, G. (2020). Blood cadmium concentration and risk of subarachnoid haemorrhage. Environ. Res. 180, 108826. doi: 10.1016/j.envres.2019.108826
Stanger, M.J., Thompson, L.A., Young, A.J., and Lieberman, H.R. (2012). Anticoagulant activity of select dietary supplements. Nutr. Rev. 70, 107–117. doi: 10.1111/j.1753-4887.2011.00444.x
Starke, R.M., Chalouhi, N., Ding, D., Raper, D.M., McKisic, M.S., Owens, G.K., et al. (2014). Vascular smooth muscle cells in cerebral aneurysm pathogenesis. Transl. Stroke Res. 5, 338–346. doi: 10.1007/s12975-013-0290-1
Su, Y., Fan, W., Ma, Z., Wen, X., Wang, W., Wu, Q., et al. (2014). Taurine improves functional and histological outcomes and reduces inflammation in traumatic brain injury. Neuroscience 266, 56–65. doi: 10.1016/j.neuroscience.2014.02.006
Tang, W.H., Kitai, T., and Hazen, S.L. (2017). Gut microbiota in cardiovascular health and disease. Circ. Res. 120, 1183–1196. doi: 10.1161/CIRCRESAHA.117.309715
Texakalidis, P., Sweid, A., Mouchtouris, N., Peterson, E.C., Sioka, C., Rangel-Castilla, L., et al. (2019). Aneurysm formation, growth, and rupture: The biology and physics of cerebral aneurysms. World Neurosurg. 130, 277–284. doi: 10.1016/j.wneu.2019.07.093
Tong, T.Y.N., Appleby, P.N., Bradbury, K.E., Perez-Cornago, A., Travis, R.C., Clarke, R., et al. (2019). Risks of ischaemic heart disease and stroke in meat eaters, fish eaters, and vegetarians over 18 years of follow-up: results from the prospective EPIC-Oxford study. BMJ 366, l4897. doi: 10.1136/bmj.l4897
Tonomura, S., and Gyanwali, B. (2021). Cerebral microbleeds in vascular dementia from clinical aspects to host-microbial interaction. Neurochem. Int. 148, 105073. doi: 10.1016/j.neuint.2021.105073
Vlak, M.H., Rinkel, G.J., Greebe, P., van der Bom, J.G., and Algra, A. (2012). Trigger factors for rupture of intracranial aneurysms in relation to patient and aneurysm characteristics. J. Neurol. 259, 1298–1302. doi: 10.1007/s00415-011-6341-1
Wang, Z., Klipfell, E., Bennett, B.J., Koeth, R., Levison, B.S., Dugar, B., et al. (2011). Gut flora metabolism of phosphatidylcholine promotes cardiovascular disease. Nature 472, 57–63. doi: 10.1038/nature09922
Winek, K., Engel, O., Koduah, P., Heimesaat, M.M., Fischer, A., Bereswill, S., et al. (2016). Depletion of cultivatable gut microbiota by broad-spectrum antibiotic pretreatment worsens outcome after murine stroke. Stroke 47, 1354–1363. doi: 10.1161/STROKEAHA.115.011800
Xiao, L., Zheng, H., Li, J., Zeng, M., He, D., Liang, J., et al. (2022). Targeting NLRP3 inflammasome modulates gut microbiota, attenuates corticospinal tract injury and ameliorates neurobehavioral deficits after intracerebral hemorrhage in mice. Biomed. Pharmacother. 149, 112797. doi: 10.1016/j.biopha.2022.112797
Xu, S., Mei, S., Lu, J., Wu, H., Dong, X., Shi, L., et al. (2021). Transcriptome analysis of microglia reveals that the tlr2/irf7 signaling axis mediates neuroinflammation after subarachnoid hemorrhage. Front. Aging Neurosci. 13, 645649. doi: 10.3389/fnagi.2021.645649
Yamagishi, K., Iso, H., Kokubo, Y., Saito, I., Yatsuya, H., Ishihara, J., et al. (2013). Dietary intake of saturated fatty acids and incident stroke and coronary heart disease in Japanese communities: the JPHC Study. Eur. Heart J. 34, 1225–1232. doi: 10.1093/eurheartj/eht043
Yin, J., Li, H., Meng, C., Chen, D., Chen, Z., Wang, Y., et al. (2016). Inhibitory effects of omega-3 fatty acids on early brain injury after subarachnoid hemorrhage in rats: Possible involvement of G protein-coupled receptor 120/beta-arrestin2/TGF-beta activated kinase-1 binding protein-1 signaling pathway. Int. J. Biochem. Cell Biol. 75, 11–22. doi: 10.1016/j.biocel.2016.03.008
Yokosuka, K., Rutledge, C., Kamio, Y., Kuwabara, A., Sato, H., Rahmani, R., et al. (2021). Roles of phytoestrogen in the pathophysiology of intracranial aneurysm. Stroke 52, 2661–2670. doi: 10.1161/STROKEAHA.120.032042
Yu, X., Zhou, G., Shao, B., Zhou, H., Xu, C., Yan, F., et al. (2021). Gut microbiota dysbiosis induced by intracerebral hemorrhage aggravates neuroinflammation in mice. Front. Microbiol. 12, 647304. doi: 10.3389/fmicb.2021.647304
Zhai, Q., Sun, T., Sun, C., Yan, L., Wang, X., Wang, Y., et al. (2021). High plasma levels of trimethylamine N-oxide are associated with poor outcome in intracerebral hemorrhage patients. Neurol. Sci. 42, 1009–1016. doi: 10.1007/s10072-020-04618-9
Zhang, H., Huang, Y., Li, X., Han, X., Hu, J., Wang, B., et al. (2021). Dynamic process of secondary pulmonary infection in mice with intracerebral hemorrhage. Front. Immunol. 12, 767155. doi: 10.3389/fimmu.2021.767155
Zhang, X., Ares, W.J., Taussky, P., Ducruet, A.F., and Grandhi, R. (2019). Role of matrix metalloproteinases in the pathogenesis of intracranial aneurysms. Neurosurg. Focus 47, E4. doi: 10.3171/2019.4.FOCUS19214
Keywords: subarachnoid hemorrhage, microbiome, neuroinflammation, aneurysm, diet, intracranial hemorrhage
Citation: Nwafor DC, Brichacek AL, Rallo MS, Bidwai N and Marsh RA (2023) Subarachnoid hemorrhage: New insights on pathogenesis. Front. Stroke 2:1110506. doi: 10.3389/fstro.2023.1110506
Received: 28 November 2022; Accepted: 23 January 2023;
Published: 06 February 2023.
Edited by:
Ryan C. Turner, West Virginia University, United StatesReviewed by:
Daniel J. Beard, The University of Newcastle, AustraliaCopyright © 2023 Nwafor, Brichacek, Rallo, Bidwai and Marsh. This is an open-access article distributed under the terms of the Creative Commons Attribution License (CC BY). The use, distribution or reproduction in other forums is permitted, provided the original author(s) and the copyright owner(s) are credited and that the original publication in this journal is cited, in accordance with accepted academic practice. No use, distribution or reproduction is permitted which does not comply with these terms.
*Correspondence: Robert A. Marsh, cm9iZXJ0Lm1hcnNoQGhzYy53dnUuZWR1