- 1Integrative Orthopedic Laboratory, Department of Molecular Medicine and Surgery, Karolinska Institutet, Stockholm, Sweden
- 2Department of Trauma, Acute Surgery and Orthopedics, Karolinska University Hospital, Stockholm, Sweden
Neuromuscular Electrical Stimulation (NMES) uses electrical impulses to induce muscle contractions, providing benefits in rehabilitation, muscle activation, and as an adjunct to exercise, particularly for individuals experiencing immobilization or physical disability. NMES technology has significantly progressed, with advancements in device development and a deeper understanding of treatment parameters, such as frequency, intensity, and pulse duration. These improvements have expanded NMES applications beyond rehabilitation to include enhanced post-exercise recovery, improved blood glucose uptake, and increased lower limb venous return, potentially reducing thrombotic risks. Despite its benefits, NMES faces challenges in user compliance, often due to improper electrode placement and discomfort during treatment. Research highlights the importance of optimizing stimulation parameters, including electrode positioning, to improve both comfort and treatment efficacy. Recent innovations, such as automated processes for locating optimal stimulation points and adaptable electrode sizes, aim to address these issues. When combined with wearable technologies, these innovations could improve NMES treatment adherence and deliver more consistent, long-term therapeutic outcomes for patients with various physical limitations. Together, these developments indicate a promising future for NMES, presenting a valuable tool to enhance the benefits of physical activity across diverse populations, from rehabilitative care to broader health and wellness applications.
Introduction
Neuromuscular electrical stimulation (NMES) is a treatment method used to create muscle contractions through electrical impulses. NMES mimics the body's nervous system during voluntary muscle activation, but instead of the signal originating in the brain, it comes from an electrical stimulator. This is achieved by placing electrodes on the skin over the target muscle.
The use of electricity for medical treatment dates back to ancient Egypt and Greece, where electric eels were used for pain relief. Modern NMES evolves from Galvani’s 18th century discovery that electric current can induce muscle contraction (1). Today, electrical stimulation is applied in various medical contexts, such as transcutaneous electrical nerve stimulation (TENS) for pain management (2) and NMES in rehabilitation settings. NMES is commonly used to strengthen weakened muscles, reduce muscle atrophy during immobilization (e.g., after surgery or injury), and complement exercise to optimize training effects (3, 4).
However, current NMES protocols still suffer from poor compliance and inadequate efficacy, attributed to limited/insufficient user proficiency regarding repeated application of electrodes in the correct placement. Research has shown that electrode placement based on a prior manual search of the optimal points and individual adaption of electrode dimensions significantly improves treatment effectiveness and comfort (5).
Recent innovations have introduced an automated search process that identifies the optimal stimulation points and electrode sizes for each patient, ensuring consistent results and improved compliance with NMES treatment (6). Combined with wearable technologies, such as garment-based applications, these advancements hold the potential to enhance treatment adherence and improve long-term outcomes for patients with physical disabilities.
General considerations of NMES usage
Settings for optimal NMES usage
Studies have shown that the NMES parameters also affect the comfort and effectiveness of the stimulation (3, 7–9). Several parameters can be adjusted during NMES, such as frequency, pulse width, intensity, waveform, plateau time, on:off-time and ramp-up/ramp-down time (Figure 1).
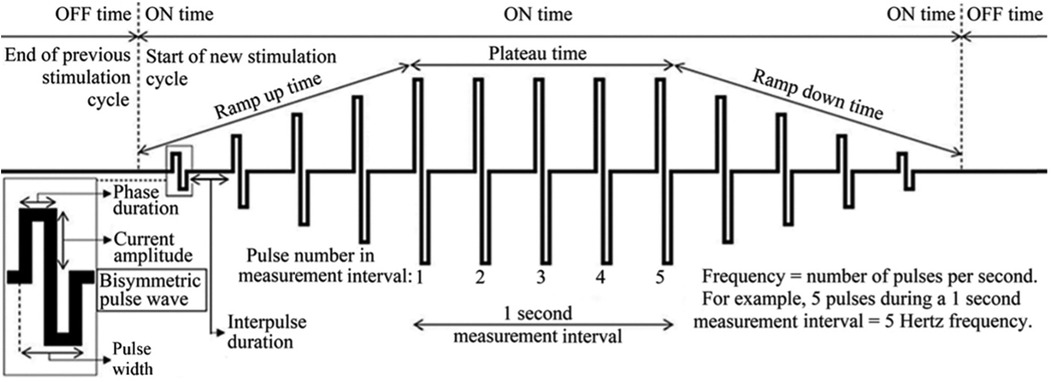
Figure 1. Examples of parameters that may be adjusted for optimal treatment effects and comfort during neuromuscular electrical stimulation (NMES). Image adapted from Juthberg et al. (10).
Pulse width, frequency, and intensity
Overall, research has shown that increasing each of pulse width, frequency, and/or intensity leads to recruitment of more muscle fibers, resulting in higher force production (7–10). However, these parameters also affect the comfort of stimulation. Frequency correlates directly with muscle torque production, even when using textile electrodes (12). Longer pulse widths, such as between 400 and 600 μs, selectively target motor fibers, while shorter pulse widths target sensory fibers to a higher extent. Consequently, longer pulse widths positively influences muscle torque production. Studies on quadriceps NMES suggest that a pulse width of 400–600 µs and frequency between 30 and 50 Hz provide optimal muscle recruitment without inducing excessive muscle fatigue and metabolic demands (3).
On: off-time and ramp-up/ramp-down time
The on: off-time and ramp-up/ramp-down time are believed to mainly affect comfort. The optimal on: off time is not well defined, but if the off-time is too short, the risk of fatigue increases due to insufficient muscle recovery (3). Prior research has demonstrated that an on: off time of 1:5 (e.g., 10 s on and 50 s off) allows the muscle to recover between stimulations (3).
High- and low-intensity NMES
Most research has focused on high-intensity NMES, aiming to mimic the muscle contractions during maximal exercise, which is desirable for muscle strengthening effects (13, 14). However, high intensity NMES can be quite painful. In contrast, low-intensity (LI)-NMES results in minimal pain (15) while still producing muscle contractions, an outcome which significantly increases both venous and arterial blood flow. LI-NMES has therefore been demonstrated as a promising method to prevent venous thromboembolism in both calf and quadriceps muscles (12, 16–18).
Differences between NMES and voluntary muscle contractions
While NMES aims to mimic voluntary muscle contraction, it differs from the contractions induced via the central nervous system in a number of ways. NMES activates muscle units simultaneously between the position of the electrodes, often targeting superficial muscles, and recruits them repetitively in a fixed spatial pattern, which leads to quicker fatigue compared to voluntary contractions (3, 7–9). In contrast, voluntary muscle contractions disperse the recruitment of motor units and vary their activation in numbers and across changing locations. NMES also primarily targets fast-twitch muscle fibers, which contribute to quicker fatigue but on the other hand is advantageous for rehabilitation, as these are the fibers predominantly weakened following injury or surgery (3).
Side effects of NMES
In recent years, an increasing number of studies have indicated that improper electrical stimulation can have harmful effects (19). In addition to common muscle soreness lasting one to four days after treatment, over-treatment can result in muscle fiber damage, increased secretion of creatine kinase, and muscle breakdown (rhabdomyolysis). This can potentially lead to acute kidney failure, especially in individuals whose kidney function already is reduced (20). These injuries have been particularly noted with excessive muscle training in suits, i.e., whole-body electromyostimulation, containing many electrodes stimulating several muscle groups simultaneously (20). However, whole-body electromyostimulation has in recent reviews of controlled trials shown significant, moderate to large effect sizes on sarcopenia, muscle mass and strength parameters (21).
Other side effects or drawbacks of current NMES treatments are that many patients experience discomfort or pain during stimulation (3, 4), and difficulties in correctly setting up the NMES device without assistance, leading to low adherence (22, 23). Adherence to treatment is the most important factor in determining whether the treatment in clinical practice can achieve the effects shown in studies. This challenge has prompted researchers at Karolinska Institutet to focus on the development and optimization of NMES, including integrating the treatment into clothing, which has the potential to dramatically improve treatment adherence with NMES (Figure 2) (24).
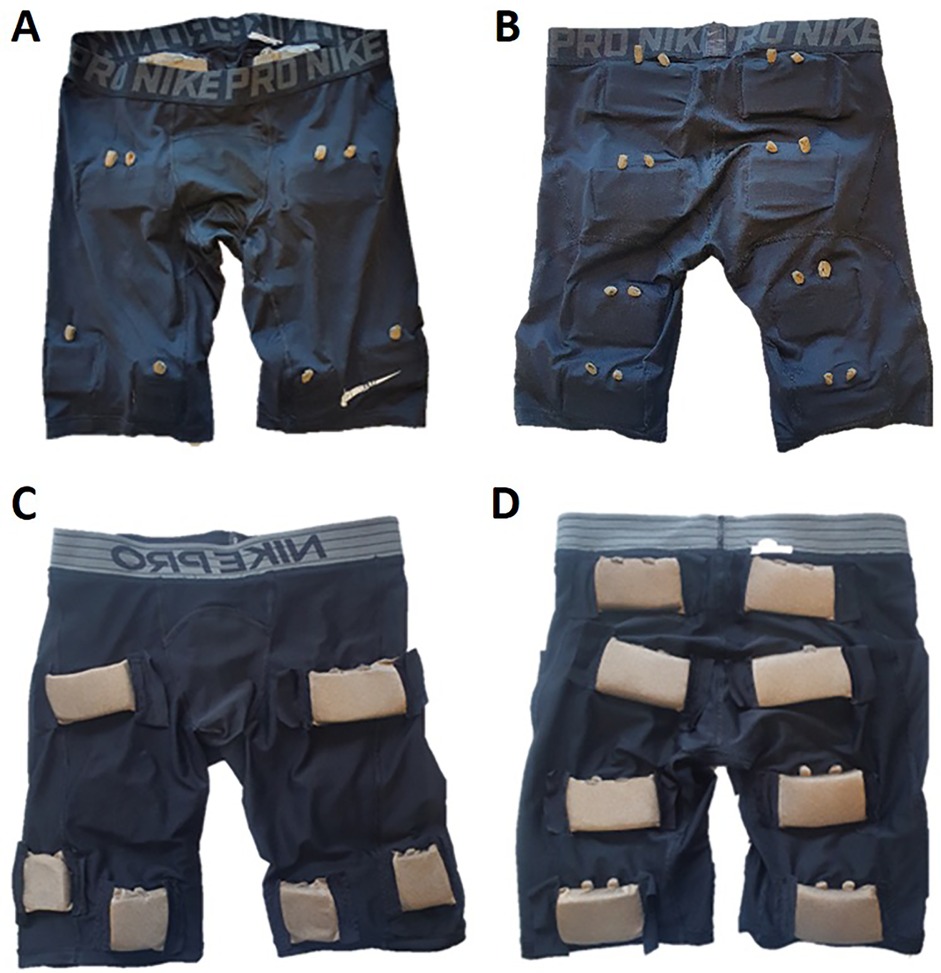
Figure 2. Image of NMES pants seen from the outside (A frontside, B backside) and inside (C frontside, D backside). On (A,B) there are connectors for stimulation. The larger electrodes are sized 5 × 9 cm (upper electrodes in C and all electrodes in D) and the smaller (lower electrodes in C) are sized 5 × 5 cm. The NMES pants are developed together with the Swedish School of Textiles at the University of Borås, Sweden. Image adapted from (12).
NMES electrodes
Optimal NMES usage requires adapting the size, number, and placement of electrodes (Figure 2). Studies have shown that larger electrodes are more comfortable than smaller ones, but excessively large electrodes decrease the effectiveness of the treatment (15, 25). The optimal size also depends on the muscle being stimulated, with larger muscles generally requiring larger electrodes (15, 25). Placement of electrodes on so-called motor points has been shown to provide more comfortable and effective stimulation (3, 5). The electrophysiological definition of a motor point is the location on the skin which requires the lowest intensity of electrical stimulation to cause a muscle contraction (26). However, the time-consuming manual motor point search with a motor point pen requires training, and thus poses a problem in the daily use of NMES. Therefore, anatomical maps have been developed showing where on the body motor points are most likely to be found (5, 27, 28). However, there is significant individual variation in the location of motor points and thus normal users will experience problems in locating motor points, which will lead to a compliance problem with the therapy.
Multiple NMES electrodes in a matrix
To address these challenges and account for the large individual variation in motor point locations, years of development has led to an automatic motor point search procedure performed within a matrix of electrodes, which may improve NMES treatment adherence (6). Within a matrix of electrodes, multiple electrodes can be combined to form individualized electrode sizes, enhancing both compliance and treatment outcomes. Customizing electrode sizes is essential for different treatment indications and muscle groups. Additionally, varying stimulation within the electrode matrix can reduce muscle fatigue and recruit a broader range of muscle fibers. As a result, NMES within an electrode matrix offers the potential for automatic electrode placement over motor points, with individualized electrode sizes and numbers tailored to specific treatments.
Muscle strengthening effects of NMES
NMES is currently used both as a complement to training to optimize the effect in healthy individuals and by physiotherapists as part of rehabilitation, such as after surgery or injuries, to maintain muscle strength and strengthen weakened muscles (3, 4, 27–29). Most earlier studies on the training effects of NMES have focused on its use alongside voluntary muscle activation (30, 31). However, recent studies show that NMES alone can also have beneficial effects on maintaining muscle strength, rehabilitating injuries, and achieving recovery after training (3, 32).
NMES with voluntary muscle contraction
Several studies have demonstrated that combining NMES with voluntary muscle contractions yields superior training effects, both in younger and older individuals (3, 9, 29–31). For example, one study demonstrated that NMES combined with leg and gluteal strength training three times a week during for four weeks in older adults improved walking test times more than exercise alone (33). Another study in young, healthy and physically active individuals showed that a 6-week training program involving vertical jumps with added electrical stimulation increased jump height by 10% more than just jump training alone or no training at all (3). These findings suggest that NMES can stimulate parts of the muscle that regular exercise may not easily reach and/or provide a greater load the than typical training. Moreover, a recent study indicated that NMES and exercise can potentiate each other even when performed on opposite extremities (34). These observations suggest involvement of either systemic mediator mechanisms and/or effects mediated through the central nervous system.
NMES without voluntary muscle contraction
Studies have shown that NMES alone can achieve a relatively high percentage of the maximum force generated during voluntary muscle activation, without any voluntary contraction (3, 9, 35, 36). To measure the force generated during knee extension exercises of the thigh muscles, a dynamometer (Biodex) is used, and the maximum force generated is referred to as the “maximum voluntary contraction” (MVC) (37). The degree of muscle activation during NMES can also be measured using this device (36), and the percentage of MVC achieved is reported as a percentage of MVC.
Research suggests that a stimulation level of at least 20% of MVC is required for muscle-strengthening effects (9). Muscle contractions induced by NMES generally produce lower force output than voluntary contractions, usually less than 50% of MVC at the highest tolerable intensity (38), which is attributed to the difference between how the muscles are activated (3, 8, 9). NMES may also activate more superficial muscle fibers, which could result in poorer training outcomes compared to regular exercise. This has led to further research aimed at improving NMES techniques (38), including optimizing the number, placement, and size of electrodes, NMES parameters, and training protocols (8, 15, 23, 39). Additionally, combining NMES with methods like blood flow restriction has shown potential for producing better training effects than NMES alone (38, 40).
The molecular effects of NMES
To better understand the effects of NMES on muscle, several studies have examined its effects at the gene and muscle fiber levels (13, 41–45). One study comparing a 30-min NMES session at the highest tolerable intensity with regular strength training found that both methods altered the expression of genes activated by exercise in the thigh muscle 24 h post-workout (13). While regular strength exercise regulated gene expression to a greater extent than NMES, NMES remains a good alternative when regular exercise is not possible (13).
A recent study conducted by our research group demonstrated that a single NMES session at 20% MVC, using NMES pants, regulated 4,448 differentially expressed genes (DEGs), with an 80% overlap with the 2,571 DEGs regulated by regular exercise. The genes regulated by NMES included well-known exercise-related genes such as PPARGC1A, ABRA, VEGFA, and GDNF. Only eight genes were regulated in opposite directions by NMES and exercise. The three genes upregulated by NMES and downregulated by exercise included genes involved in neurite outgrowth (MYLIP), cell proliferation and regulation of mTORC1 signaling (ICK) and negative regulation of cell proliferation (JARID2) (34). It was also demonstrated that the NMES-session at 20% of MVC could be applied with an acceptable level of discomfort, e.g., VAS below 4 (34).
In other studies, the effect of multiple NMES treatments (over 5 days to 10 weeks, with 3–6 sessions per week, lasting 18 min to 2 h per session) have been shown to affect gene and muscle fiber composition in both younger (41, 43) and older adults (41, 44). These effects have also been observed orthopedic contexts, aiding recovery of quadriceps strength after knee surgery, anterior cruciate ligament reconstruction and total knee arthroplasty (42, 46–48).
In summary, studies have concluded that NMES can preserve muscle mass, prevent muscle atrophy, and to some extent alter and improve gene expression. When compared to regular exercise, the effects of NMES are less pronounced, but it remains a valuable option for individuals unable to engage in regular exercise and as a complement to standard training for healthy individuals (41, 42).
NMES benefits during physical inactivity
Physical inactivity is a major and growing global health problem, contributing to approximately 3.2 million preventable deaths each year (49, 50). Immobilization and inactivity are closely linked to the development and progression of obesity, type 2 diabetes (50), venous blood clot development in the legs and lungs (51), and reduced muscle strength and balance, which can lead to falls, particularly in older adults (49, 50, 52). While physical activity is an effective way to counteract these negative effects, it is not always possible, especially after surgery or for older patients with underlying illnesses (53). Current treatment methods for these conditions are often insufficient, largely due to low compliance. There is a clear need for improved treatment options to mitigate the adverse effects of physical inactivity. NMES, which uses the body's own energy to create muscle contractions, is an alternative way to activate muscles during periods of immobilization (30, 54–56). This makes NMES especially beneficial for older adults, post-surgical patients, and individuals with cardiovascular risks or other co-morbidities, such as chronic obstructive pulmonary disease, who have difficulty engaging with exercise programs.
Improved balance
For people over 65, there is a 30% risk of falling each year (57), and for those living in nursing homes, this rate increases to 50% (48). Falls in older adults can result in severe consequences, including fractures, immobilization, and even death (58). While regular physical activity reduces the risk of falls and related fractures (50), many older adults are unable to engage in such activities. NMES has, especially among older and/or untrained individuals, been shown to provide effective muscle activation, resulting in improved muscle strength and function (30, 31, 45, 54, 55, 59). For example, NMES treatment for 30 min, 2–3 times a week for 9 weeks has been shown to improve walking test time by 15%–20% (45).
Improved metabolic control
In addition to the risk of falls, physical inactivity also increases the risk of type 2 diabetes and obesity (50). Globally, one in eleven adults has diabetes, and in 2019 more than four million people died due to diabetes or its complications, equating to one death every 8 s. In addition to those already diagnosed with diabetes, even more people have pre-diabetes with the risk of developing the disease but also with a great opportunity for prevention (60). Physical activity is crucial both for prevention and treatment of type 2 diabetes, but as mentioned above, many are unable to engage in regular exercise. For these individuals, NMES presents as a valuable alternative, offering similar effects as regular physical activity on blood sugar regulation (53, 61–64). One study demonstrated that patients with type 2 diabetes who performed 40-min quadriceps NMES sessions, 5 days per week for 8 weeks, significantly improved fasting glucose levels and reduced body fat (53). A systematic review has confirmed these effects (65). Moreover, patients with type 2 diabetes often suffer from peripheral artery disease, which causes ischemic pain in the lower limbs and impairs walking. NMES has been shown to increase peripheral arterial flow, reduce ischemic pain, and enhance walking distances (66). However, systematic reviews call for more high-quality trials to draw definitive conclusions (67).
Preventing the formation of blood clots
Another significant risk posed by physical inactivity and extended immobilization is the development of blood clots in the legs or lungs (51). Between 1 and 4 out of 100 people will develop a blood clot requiring treatment during their lifetime (68). Anticoagulant treatments, while available, are not always effective (69), and for older adults who are prone to falls, they pose a bleeding risk (70). Mechanical compression therapy, such as intermittent pneumatic compression (IPC), is used in hospitals to increase blood flow, mimicking the muscle pump action that occurs during walking (71). However, IPC machines are too large and noisy for use outside of hospital environments, which is why NMES treatment, where electronics can be minimized, provides a quiet and mobile treatment option outside of hospitals. NMES treatment on the calf and quadriceps has been shown to improve venous blood flow in the leg vessels (12, 17, 18). Adding NMES treatment to drug therapy with anticoagulants during knee replacement surgery (72) and for patients undergoing major surgeries (73) reduces the risk of blood clots in the leg. While NMES alone can lower the risk of clots compared to no treatment during immobilization, it has not yet proven as effective as anticoagulants (18, 74). More research is needed to explore NMES as a sole treatment for preventing blood clots, as studies in this area are limited (18).
Future directions
Future research should clarify the similarities and differences between the effects of NMES and regular physical exercise. One notable area of exploration is the load or impact on the cardiovascular system. Additionally, exercise has demonstrated neurobiological benefits, such as protection against cognitive disorders like dementia. A key factor released during exercise, which is brain protective, is brain-derived neurotrophic factor (BDNF). Interestingly, NMES has also been demonstrated to increased BDNF levels (75). The neurobiological effects of muscle stimulation are likely mediated via the release of myokines, which are peptide modulators of several tissue processes such as brain neuroplasticity, bone mineralization, and tissue repair (76). Notably, NMES has demonstrated the production of several myokines, which can exert beneficial effects on the pathophysiology of several conditions in patients with limited mobility (76). Future research should in more detail delineate the indications, settings and optimal dose-response relationships of NMES to induce beneficial effects.
Limitations
While NMES shows promise, certain patients may be “non-responders” to NMES, particularly those with low contractile responses. Thus, in a study of critically ill patients in an intensive care unit it was demonstrated that patients with higher severity of illness were more likely to be non-responders to NMES (77). However, NMES has shown positive effects to maintain and improve limb strength in other severely ill populations, such as those with acute exacerbation of chronic obstructive pulmonary disease (78), acute heart failure (79), chronic kidney failure on hemodialysis (80) and spinal cord injury (81). Still, the existing studies have reported a wide range of stimulation parameters. Thus, future high-quality randomized trials should focus on standardizing NMES settings for specific indications.
Conclusion
In summary, NMES offers a range of applications with positive effects for both older and younger individuals, including those who are healthy or living with health conditions. It can serve as a complement to physical activity or as an alternative when traditional exercise is not feasible, providing similar benefits. Additionally, NMES can also be considered as a non-invasive tool to address several research questions regarding muscles and muscle function in compromised populations. Although current NMES applications remain suboptimal, recent advancements in automated electrode placement and individualized stimulation settings show promise. These developments may improve treatment adherence and deepen our understanding of how to optimize NMES for various populations.
Author contributions
PA: Conceptualization, Funding acquisition, Resources, Supervision, Writing – original draft, Writing – review & editing. RJ: Conceptualization, Writing – original draft, Writing – review & editing. JF: Conceptualization, Writing – original draft, Writing – review & editing.
Funding
The author(s) declare financial support was received for the research, authorship, and/or publication of this article. The authors would like to thank the Swedish Research Council (2017-00202) and The Swedish Research Council for Sport Science for their financial support. This work was supported by the strategic innovation programs Swelife and Medtech4Health, which are jointly arranged and funded by Swedeńs Innovation Agency (Vinnova), Grant no: 2019-05479 Formas and Energimyndigheten.
Acknowledgments
The authors would like to thank the Department of Trauma, Acute Surgery and Orthopedics at Karolinska University Hospital for providing the clinical research environment.
Conflict of interest
PA and RJ declare a potential conflict of interest. They have a granted patent related to neuromuscular electrical stimulation. UK Patent, publication number GB2601757. A system comprising a controller and an electrical stimulation system.
The remaining author declares that the research was conducted in the absence of any commercial or financial relationships that could be construed as a potential conflict of interest.
Generative AI statement
The author(s) declare that no Gen AI was used in the creation of this manuscript.
Publisher's note
All claims expressed in this article are solely those of the authors and do not necessarily represent those of their affiliated organizations, or those of the publisher, the editors and the reviewers. Any product that may be evaluated in this article, or claim that may be made by its manufacturer, is not guaranteed or endorsed by the publisher.
References
1. Heidland A, Fazeli G, Klassen A, Sebekova K, Hennemann H, Bahner U, et al. Neuromuscular electrostimulation techniques: historical aspects and current possibilities in treatment of pain and muscle waisting. Clin Nephrol. (2013) 79(Suppl 1):S12–23. doi: 10.5414/CNX77S106
2. Sluka KA, Walsh D. Transcutaneous electrical nerve stimulation: basic science mechanisms and clinical effectiveness. J Pain. (2003) 4(3):109–21. doi: 10.1054/jpai.2003.434
3. Glaviano NR, Saliba S. Can the use of neuromuscular electrical stimulation be improved to optimize quadriceps strengthening? Sports Health. (2016) 8(1):79–85. doi: 10.1177/1941738115618174
4. Lyons GM, Leane GE, Clarke-Moloney M, O’Brien JV, Grace PA. An investigation of the effect of electrode size and electrode location on comfort during stimulation of the gastrocnemius muscle. Med Eng Phys. (2004) 26(10):873–8. doi: 10.1016/j.medengphy.2004.08.003
5. Gobbo M, Maffiuletti NA, Orizio C, Minetto MA. Muscle motor point identification is essential for optimizing neuromuscular electrical stimulation use. J NeuroEng Rehab. (2014) 11:17. doi: 10.1186/1743-0003-11-17
6. Ackermann P. Juthberg R, inventors; Matrix Muscle Support AB, assignee. A system comprising a controller and an electrical stimulation system. GB patent G. (2024) B2019319.
7. Baker LL, McNeal DR, Benton LA, Bowman BR, Waters RL. Neuromuscular electrical stimulation—a practical guide, 3rd ed. Downey, CA: Los Amigos Research & Education Institute Inc. (1993).
8. Doucet BM, Lam A, Griffin L. Neuromuscular electrical stimulation for skeletal muscle function. Yale J Biol Med. (2012) 85(2):201–15.22737049
9. Maffiuletti NA. Physiological and methodological considerations for the use of neuromuscular electrical stimulation. Eur J Appl Physiol. (2010) 110(2):223–34. doi: 10.1007/s00421-010-1502-y
10. Juthberg R, Flodin J, Guo L, Rodriguez S, Persson NK, Ackermann PW. Neuromuscular electrical stimulation in garments optimized for compliance. Eur J Appl Physiol. (2023) 123(8):1739–48. doi: 10.1007/s00421-023-05181-9. Erratum in: Eur J Appl Physiol. (2023) 123(8):1749. doi: 10.1007/s00421-023-05211-6
11. Gorgey AS, Mahoney E, Kendall T, Dudley GA. Effects of neuromuscular electrical stimulation parameters on specific tension. Eur J Appl Physiol. (2006) 97(6):737–44. doi: 10.1007/s00421-006-0232-7
12. Flodin J, Wallenius P, Guo L, Persson NK, Ackermann P. Wearable neuromuscular electrical stimulation on quadriceps muscle can increase venous flow. Ann Biomed Eng. 51(12):2873–82. doi: 10.1007/s10439-023-03349-0
13. Latimer LE, Constantin D, Greening NJ, Calvert L, Menon MK, Steiner MC, et al. Impact of transcutaneous neuromuscular electrical stimulation or resistance exercise on skeletal muscle mRNA expression in COPD. Int J Chron Obstruct Pulmon Dis. (2019) 14:1355–64. doi: 10.2147/COPD.S189896
14. Rabello R, Fröhlich M, Maffiuletti NA, Vaz MA. Influence of pulse waveform and frequency on evoked torque, stimulation efficiency, and discomfort in healthy subjects. Am J Phys Med Rehabil. (2021) 100(2):161–7. doi: 10.1097/PHM.0000000000001541
15. Flodin J, Juthberg R, Ackermann PW. Effects of electrode size and placement on comfort and efficiency during low-intensity neuromuscular electrical stimulation of quadriceps, hamstrings and gluteal muscles. BMC Sports Sci Med Rehabil. (2022) 14(1):11. doi: 10.1186/s13102-022-00403-7
16. Sundstrom C, Juthberg R, Flodin J, Guo L, Persson NK, Ackermann PW. Effects on hemodynamic enhancement and discomfort of a new textile electrode integrated in a sock during calf neuromuscular electrical stimulation. Eur J Appl Physiol. (2023) 123(9):2013–22. doi: 10.1007/s00421-023-05212-5
17. Moloney MC, Lyons GM, Breen P, Burke PE, Grace PA. Haemodynamic study examining the response of venous blood flow to electrical stimulation of the gastrocnemius muscle in patients with chronic venous disease. Eur J Vasc Endovasc Surg. (2006) 31(3):300–5. doi: 10.1016/j.ejvs.2005.08.003
18. Hajibandeh S, Antoniou GA, Scurr JR, Torella F. Neuromuscular electrical stimulation for the prevention of venous thromboembolism. Cochrane Database Syst Rev. (2017) 11:CD011764.29161465
19. Fouré A, Gondin J. Skeletal muscle damage produced by electrically evoked muscle contractions. Exerc Sport Sci Rev. (2021) 49(1):59–65. doi: 10.1249/JES.0000000000000239
20. Kemmler W, Teschler M, Bebenek M, von Stengel S. (Very) high creatinkinase concentration after exertional whole-body electromyostimulation application: health risks and longitudinal adaptations. Wien Med Wochenschr. (2015) 165(21–22):427–35. doi: 10.1007/s10354-015-0394-1
21. de Oliveira TMD, Felicio DC, Filho JE, Fonseca DS, Durigan JLQ, Malaguti C. Effects of whole-body electromyostimulation on health indicators of older people: systematic review and meta-analysis of randomized trials. J Bodyw Mov Ther. (2022) 31:134–45. doi: 10.1016/j.jbmt.2022.03.010
22. Burgess LC, Taylor P, Wainwright TW, Bahadori S, Swain ID. Adherence to neuromuscular electrical stimulation interventions for muscle impairment in hip and knee osteoarthritis: a systematic review. Clin Med Insights Arthritis Musculoskelet Disord. (2021) 14:11795441211028746. doi: 10.1177/11795441211028746
23. Maffiuletti NA, Gondin J, Place N, Stevens-Lapsley J, Vivodtzev I, Minetto MA. Clinical use of neuromuscular electrical stimulation for neuromuscular rehabilitation: what are we overlooking? Arch Phys Med Rehabil. (2018) 99(4):806–12. doi: 10.1016/j.apmr.2017.10.028
24. Innovation K. Smarta Kläder som kan Upptäcka Långa Perioder av Muskelinaktivitet. Stockholm: Karolinska Institutet Innovations AB (2020). Available online at: https://karolinskainnovations.ki.se/case/smart-clothing-that-can-detect-prolonged-periods-of-muscle-inactivity/
25. Brooks ME, Smith EM, Currier D. Effect of longitudinal versus transverse electrode placement on torque production by the quadriceps femoris muscle during neuromuscular electrical stimulation. J Orthop Sports Phys Ther. (1990) 11(11):530–4. doi: 10.2519/jospt.1990.11.11.530
26. Moon JY, Hwang TS, Sim SJ, Chun SI, Kim M. Surface mapping of motor points in biceps brachii muscle. Ann Rehabil Med. (2012) 36(2):187–96. doi: 10.5535/arm.2012.36.2.187
27. Flodin J, Juthberg R, Ackermann PW. Motor point heatmap guide for neuromuscular electrical stimulation of the quadriceps muscle. J Electromyogr Kinesiol. (2023) 70:102771. doi: 10.1016/j.jelekin.2023.102771
28. Schriwer E, Juthberg R, Flodin J, Ackermann PW. Motor point heatmap of the calf. J Neuroeng Rehabil. (2023) 20(1):28. doi: 10.1186/s12984-023-01152-5
29. Dabadghav R, Potdar A, Patil V, Sancheti P, Shyam A. Additional effect of neuromuscular electrical stimulation on knee extension lag, pain and knee range of motion in immediate postsurgical phase (0–2 weeks) in primary total knee arthroplasty patient. Ann Transl Med. (2019) 7(Suppl 7):S253. doi: 10.21037/atm.2019.09.79
30. Langeard A, Bigot L, Chastan N, Gauthier A. Does neuromuscular electrical stimulation training of the lower limb have functional effects on the elderly?: A systematic review. Exp Gerontol. (2017) 91:88–98. doi: 10.1016/j.exger.2017.02.070
31. Benavent-Caballer V, Rosado-Calatayud P, Segura-Orti E, Amer-Cuenca JJ, Lison JF. Effects of three different low-intensity exercise interventions on physical performance, muscle CSA and activities of daily living: a randomized controlled trial. Exp Gerontol. (2014) 58:159–65. doi: 10.1016/j.exger.2014.08.004
32. Malone JK, Coughlan GF, Crowe L, Gissane GC, Caulfield B. The physiological effects of low-intensity neuromuscular electrical stimulation (NMES) on short-term recovery from supra-maximal exercise bouts in male triathletes. Eur J Appl Physiol. (2012) 112(7):2421–32. doi: 10.1007/s00421-011-2212-9
33. Jang EM, Park SH. Effects of neuromuscular electrical stimulation combined with exercises versus an exercise program on the physical characteristics and functions of the elderly: a randomized controlled trial. Int J Environ Res Public Health. (2021) 18(5):2463. doi: 10.3390/ijerph18052463
34. Flodin J, Reitzner SM, Emanuelsson EB, Sundberg CJ, Ackermann P. The effect of neuromuscular electrical stimulation on the human skeletal muscle transcriptome. Acta Physiol. (2024) 240(5):e14129. doi: 10.1111/apha.14129
35. Maffiuletti NA, Morelli A, Martin A, Duclay J, Billot M, Jubeau M, et al. Effect of gender and obesity on electrical current thresholds. Muscle Nerve. (2011) 44(2):202–7. doi: 10.1002/mus.22050
36. Flodin J, Mikkelsen C, Ackermann PW. Knee extensor force production and discomfort during neuromuscular electrical stimulation of quadriceps with and without gluteal muscle co-stimulation. Eur J Appl Physiol. (2022) 122(6):1521–30. doi: 10.1007/s00421-022-04949-9
37. Drouin JM, Valovich-mcLeod TC, Shultz SJ, Gansneder BM, Perrin DH. Reliability and validity of the biodex system 3 pro isokinetic dynamometer velocity, torque and position measurements. Eur J Appl Physiol. (2004) 91(1):22–9. doi: 10.1007/s00421-003-0933-0
38. Blazevich AJ, Collins DF, Millet GY, Vaz MA, Maffiuletti NA. Enhancing adaptations to neuromuscular electrical stimulation training interventions. Exerc Sport Sci Rev. (2021) 49(4):244–52. doi: 10.1249/JES.0000000000000264
39. Maffiuletti NA, Vivodtzev I, Minetto MA, Place N. A new paradigm of neuromuscular electrical stimulation for the quadriceps femoris muscle. Eur J Appl Physiol. (2014) 114(6):1197–205. doi: 10.1007/s00421-014-2849-2
40. Natsume T, Ozaki H, Saito AI, Abe T, Naito H. Effects of electrostimulation with blood flow restriction on muscle size and strength. Med Sci Sports Exerc. (2015) 47(12):2621–7. doi: 10.1249/MSS.0000000000000722
41. Sillen MJ, Franssen FM, Gosker HR, Wouters EF, Spruit MA. Metabolic and structural changes in lower-limb skeletal muscle following neuromuscular electrical stimulation: a systematic review. PLoS One. (2013) 8(9):e69391. doi: 10.1371/journal.pone.0069391
42. Karlsen A, Cullum CK, Norheim KL, Scheel FU, Zinglersen AH, Vahlgren J, et al. Neuromuscular electrical stimulation preserves leg lean mass in geriatric patients. Med Sci Sports Exerc. (2020) 52(4):773–84. doi: 10.1249/MSS.0000000000002191
43. Dirks ML, Wall BT, Snijders T, Ottenbros CL, Verdijk LB, van Loon LJ. Neuromuscular electrical stimulation prevents muscle disuse atrophy during leg immobilization in humans. Acta Physiol (Oxf). (2014) 210(3):628–41. doi: 10.1111/apha.12200
44. Mancinelli R, Toniolo L, Di Filippo ES, Doria C, Marrone M, Maroni CR, et al. Neuromuscular electrical stimulation induces skeletal muscle fiber remodeling and specific gene expression profile in healthy elderly. Front Physiol. (2019) 10:1459. doi: 10.3389/fphys.2019.01459
45. Kern H, Barberi L, Löfler S, Sbardella S, Burggraf S, Fruhmann H, et al. Electrical stimulation counteracts muscle decline in seniors. Front Aging Neurosci. (2014) 6:189. doi: 10.3389/fnagi.2014.00189
46. Conley CEW, Mattacola CG, Jochimsen KN, Dressler EV, Lattermann C, Howard JS. A comparison of neuromuscular electrical stimulation parameters for postoperative quadriceps strength in patients after knee surgery: a systematic review. Sports Health. (2021) 13(2):116–27. doi: 10.1177/1941738120964817
47. Hauger AV, Reiman MP, Bjordal JM, Sheets C, Ledbetter L, Goode AP. Neuromuscular electrical stimulation is effective in strengthening the quadriceps muscle after anterior cruciate ligament surgery. Knee Surg Sports Traumatol Arthrosc. (2018) 26(2):399–410. doi: 10.1007/s00167-017-4669-5
48. Labanca L, Bonsanto F, Raffa D, Orlandi Magli A, Benedetti MG. Does adding neuromuscular electrical stimulation to rehabilitation following total knee arthroplasty lead to a better quadriceps muscle strength recovery? A systematic review. Int J Rehabil Res. (2022) 45(2):118–25. doi: 10.1097/MRR.0000000000000525
49. World Health Organization. Falls. Geneva: WHO (2021). Available online at: https://www.who.int/news-room/fact-sheets/detail/falls
50. World Health Organization. Physical Activity. Geneva: WHO (2024). Available online at: https://www.who.int/news-room/fact-sheets/detail/physical-activity.
51. Pandor A, Horner D, Davis S, Goodacre S, Stevens JW, Clowes M, et al. Different strategies for pharmacological thromboprophylaxis for lower-limb immobilisation after injury: systematic review and economic evaluation. Health technology assessment (Winchester). England. (2019) 23(63):1–190. doi: 10.3310/hta23630
52. Rubenstein LZ. Falls in older people: epidemiology, risk factors and strategies for prevention. Age Ageing. (2006) 35(Suppl 2):ii37–41. doi: 10.1093/ageing/afl084
53. Miyamoto T, Iwakura T, Matsuoka N, Iwamoto M, Takenaka M, Akamatsu Y, et al. Impact of prolonged neuromuscular electrical stimulation on metabolic profile and cognition-related blood parameters in type 2 diabetes: a randomized controlled cross-over trial. Diabetes Res Clin Pract. (2018) 142:37–45. doi: 10.1016/j.diabres.2018.05.032
54. Caulfield B, Prendergast A, Rainsford G, Minogue C. Self directed home based electrical muscle stimulation training improves exercise tolerance and strength in healthy elderly. Conference Proceedings: Annual International Conference of the IEEE Engineering in Medicine and Biology Society IEEE Engineering in Medicine and Biology Society Annual Conference, 2013 (2013). p. 7036–9
55. Miyamoto T, Kamada H, Tamaki A, Moritani T. Low-intensity electrical muscle stimulation induces significant increases in muscle strength and cardiorespiratory fitness. Eur J Sport Sci. (2016) 16(8):1104–10. doi: 10.1080/17461391.2016.1151944
56. Snyder-Mackler L, Delitto A, Stralka SW, Bailey SL. Use of electrical stimulation to enhance recovery of quadriceps femoris muscle force production in patients following anterior cruciate ligament reconstruction. Phys Ther. (1994) 74(10):901–7. doi: 10.1093/ptj/74.10.901
57. Appeadu MK, Bordoni B. Falls and Fall Prevention in Older Adults. Treasure Island, FL: StatPearls (2024).
58. Ambrose AF, Paul G, Hausdorff JM. Risk factors for falls among older adults: a review of the literature. Maturitas. (2013) 75(1):51–61. doi: 10.1016/j.maturitas.2013.02.009
59. Quittan M, Wiesinger GF, Sturm B, Puig S, Mayr W, Sochor A, et al. Improvement of thigh muscles by neuromuscular electrical stimulation in patients with refractory heart failure. Am J Phys Med Rehabil. (2001) 80(3):206–14.; quiz 15–6, 24. doi: 10.1097/00002060-200103000-00011
60. WHO. Diabetes. Geneva: WHO (2024). Available online at: https://www.who.int/news-room/fact-sheets/detail/diabetes.
61. Guzmán-González B, Llanos P, Calatayud J, Maffiuletti NA, Cruz-Montecinos C. Effect of neuromuscular electrical stimulation frequency on postprandial glycemia, current-related discomfort, and muscle soreness. A crossover study. Appl Physiol Nutr Metab. (2019) 44(8):834–9. doi: 10.1139/apnm-2018-0801
62. Jabbour G, Belliveau L, Probizanski D, Newhouse I, McAuliffe J, Jakobi J, et al. Effect of low frequency neuromuscular electrical stimulation on glucose profile of persons with type 2 diabetes: a pilot study. Diabetes Metab J. (2015) 39(3):264–7. doi: 10.4093/dmj.2015.39.3.264
63. Miyamoto T, Fukuda K, Kimura T, Matsubara Y, Tsuda K, Moritani T. Effect of percutaneous electrical muscle stimulation on postprandial hyperglycemia in type 2 diabetes. Diabetes Res Clin Pract. (2012) 96(3):306–12. doi: 10.1016/j.diabres.2012.01.006
64. Miyamoto T, Fukuda K, Watanabe K, Hidaka M, Moritani T. Gender difference in metabolic responses to surface electrical muscle stimulation in type 2 diabetes. J Electromyogr Kinesiol. (2015) 25(1):136–42. doi: 10.1016/j.jelekin.2014.06.013
65. Sanchez MJ, Mossayebi A, Sigaroodi S, Apaflo JN, Galvan MJ, Min K, et al. Effects of neuromuscular electrical stimulation on glycemic control: a systematic review and meta-analysis. Front Endocrinol (Lausanne). (2023) 14:1222532. doi: 10.3389/fendo.2023.1222532
66. Babber A, Ravikumar R, Onida S, Lane TRA, Davies AH. Effect of footplate neuromuscular electrical stimulation on functional and quality-of-life parameters in patients with peripheral artery disease: pilot, and subsequent randomized clinical trial. Br J Surg. (2020) 107(4):355–63. doi: 10.1002/bjs.11398
67. Jehannin P, Craughwell M, Omarjee L, Donnelly A, Jaquinandi V, Mahe G, et al. A systematic review of lower extremity electrical stimulation for treatment of walking impairment in peripheral artery disease. Vasc Med. (2020) 25(4):354–63. doi: 10.1177/1358863X20902272
68. Cohen AT, Agnelli G, Anderson FA, Arcelus JI, Bergqvist D, Brecht JG, et al. Venous thromboembolism (VTE) in Europe. The number of VTE events and associated morbidity and mortality. Thromb Haemostasis. (2007) 98(4):756–64. doi: 10.1160/TH07-03-0212
69. Falck-Ytter Y, Francis CW, Johanson NA, Curley C, Dahl OE, Schulman S, et al. Prevention of VTE in orthopedic surgery patients: antithrombotic therapy and prevention of thrombosis, 9th ed: American college of chest physicians evidence-based clinical practice guidelines. Chest. (2012) 141(2 Suppl):e278S–325. doi: 10.1378/chest.11-2404
70. Gómez-Outes A, Terleira-Fernández AI, Suárez-Gea ML, Vargas-Castrillón E. Dabigatran, rivaroxaban, or apixaban versus enoxaparin for thromboprophylaxis after total hip or knee replacement: systematic review, meta-analysis, and indirect treatment comparisons. Br Med J. (2012) 344:e3675. doi: 10.1136/bmj.e3675
71. Tsuda K, Takahira N, Ejiri M, Sakai K, Sakamoto M, Akamine A. Effect of resistance of the exercise band on the peak femoral vein velocity during active ankle flexion. Phlebology. (2020) 35(3):176–83. doi: 10.1177/0268355519865164
72. Izumi M, Ikeuchi M, Aso K, Sugimura N, Kamimoto Y, Mitani T, et al. Less deep vein thrombosis due to transcutaneous fibular nerve stimulation in total knee arthroplasty: a randomized controlled trial. Knee Sur Sports Traumatol Arthrosc. (2015) 23(11):3317–23. doi: 10.1007/s00167-014-3141-z
73. Lobastov K, Barinov V, Laberko L, Obolensky V, Boyarintsev V, Rodoman G. Electrical calf muscle stimulation in patients with post-thrombotic syndrome and residual venous obstruction after anticoagulation therapy. Int Angiol. (2018) 37(5):400–10. doi: 10.23736/S0392-9590.18.03997-4
74. Hajibandeh S, Antoniou GA, Scurr JR, Torella F. Neuromuscular electrical stimulation for thromboprophylaxis: a systematic review. Phlebology. (2015) 30(9):589–602. doi: 10.1177/0268355514567731
75. Kimura T, Kaneko F, Iwamoto E, Saitoh S, Yamada T. Neuromuscular electrical stimulation increases serum brain-derived neurotrophic factor in humans. Exp Brain Res. (2019) 237(1):47–56. doi: 10.1007/s00221-018-5396-y
76. Sanchis-Gomar F, Lopez-Lopez S, Romero-Morales C, Maffulli N, Lippi G, Pareja-Galeano H. Neuromuscular electrical stimulation: a new therapeutic option for chronic diseases based on contraction-induced myokine secretion. Front Physiol. (2019) 10:1463. doi: 10.3389/fphys.2019.01463
77. Grunow JJ, Goll M, Carbon NM, Liebl ME, Weber-Carstens S, Wollersheim T. Differential contractile response of critically ill patients to neuromuscular electrical stimulation. Crit Care. (2019) 23(1):308. doi: 10.1186/s13054-019-2540-4
78. Okura K, Nonoyama T, Shibuya M, Yamamoto S, Kawachi S, Nishie K, et al. Effectiveness of neuromuscular electrical stimulation in patients with acute exacerbation of chronic obstructive pulmonary disease: A systematic review and meta-analysis. Physiother Res Int. (2024) 29(2):e2076. doi: 10.1002/pri.2076
79. Kondo T, Yamada S, Tanimura D, Kazama S, Ishihara T, Shimojo M, et al. Neuromuscular electrical stimulation is feasible in patients with acute heart failure. ESC Heart Fail. (2019) 6(5):975–82. doi: 10.1002/ehf2.12504
80. Schardong J, Stein C, Della Mea Plentz R. Neuromuscular electrical stimulation in chronic kidney failure: a systematic review and meta-analysis. Arch Phys Med Rehabil. (2020) 101(4):700–11. doi: 10.1016/j.apmr.2019.11.008
Keywords: neuromuscular electrical stimulation, immobilization, muscle strengthening, exercise, blood flow, venous thromboembolism, motor points
Citation: Ackermann PW, Juthberg R and Flodin J (2024) Unlocking the potential of neuromuscular electrical stimulation: achieving physical activity benefits for all abilities. Front. Sports Act. Living 6:1507402. doi: 10.3389/fspor.2024.1507402
Received: 7 October 2024; Accepted: 15 November 2024;
Published: 29 November 2024.
Edited by:
Ronald F. Zernicke, University of Michigan, United StatesReviewed by:
Akira Tamaki, Hyogo Medical University, JapanMassimiliano Gobbo, University of Brescia, Italy
Copyright: © 2024 Ackermann, Juthberg and Flodin. This is an open-access article distributed under the terms of the Creative Commons Attribution License (CC BY). The use, distribution or reproduction in other forums is permitted, provided the original author(s) and the copyright owner(s) are credited and that the original publication in this journal is cited, in accordance with accepted academic practice. No use, distribution or reproduction is permitted which does not comply with these terms.
*Correspondence: Paul W. Ackermann, cGF1bC5hY2tlcm1hbm5Aa2kuc2U=