- School of Integrative Plant Science, Cornell University, Ithaca, NY, United States
The consequences of climate change urgently demand the reduction of atmospheric carbon, including by sequestering carbon in soil. The glomalin-related soil proteins (GRSP) of arbuscular mycorrhizal fungi (AMF) are renowned for their soil aggregation and carbon sequestration properties. With their considerable binding abilities, GRSP can also adsorb various cations and sequester heavy metals in soil, thereby assisting in soil fertilization and remediation efforts. However, despite its benefits for soil health and climate change, the mechanisms underlying these traits in the context of soil chemistry remain unexplored. In this review, we focus on three crucial roles of GRSP—long-term carbon sequestration, soil aggregation, and soil remediation and fertility—in the context of the chemical characteristics elucidated by previous research, namely hydrophobicity, amid group glycosylation (N-glycosylation), and metal adsorption. Based on the proposed chemical mechanisms, the current review also offers insight into soil factors that may influence the persistence of GRSP. We conclude by proposing a working model for GRSP, aiming to establish a conceptual platform for future research to examine GRSP in terms of their known or novel chemical and biochemical reactions, thereby improving our understanding of this important group of soil proteins.
1 Introduction
Global warming is a threat to future food production worldwide. Soil carbon (C) sequestration from the atmosphere has received much attention from both the public and researchers as it has the potential to meet the goal of reducing atmospheric C while enhancing soil fertility (1, 2). Soil C sequestration can also improve the storage of soil organic carbon (SOC), soil physical structure and reduce the risk of soil degradation and erosion while supplying nutrients and energy for soil microorganisms (1).
Arbuscular mycorrhizal fungi (AMF) are important fungi that form symbiosis with more than 80% of terrestrial plants and can effectively aid soil C sequestration and storage in the form of SOC (3). Wright and Upadhyaya (4) first discovered that AMF produced a heat-stable glycoprotein, glomalin, using an enzyme-linked immunosorbent assay (ELISA) and a monoclonal antibody, MAb32B11, raised against crushed AMF spores of Rhizophagus irregularis. Using mass spectrometry analysis, Gadkar and Rillig (5) found that glomalin shared similarities in the protein sequences with a 60 kDa heat shock protein (HSP60) of R. irregularis (> 80%), thus showing that glomalin was a putative gene product of AMF origin. In fact, Magurno et al. (6) successively utilized glomalin gene sequences (HSP 60 homologue) of 15 different AMF isolates as a gene marker to study a diversity of glomalin genes expressed in soils.
The extraction methods of glomalin extracts include: 1) high-temperature citrate extraction (HTCE) through the processes of HTCE, purification and decanting, and quantification using the Bradford assay with a reference of bovine serum albumin (BSA); and 2) the immunoreactivity test to the antibody MAb32B11 using ELISA, western blots/immunoblots, or indirect immunofluorescence assays (7–10). However, HTCE is a nonspecific protein extraction method which co-extracts other heat-stable proteins of non-AMF origin (e.g. plants, bacteria, and other soil autotrophs) along with organic compounds such as lipids, fatty acids, and humic acid (11–14). These co-extracted compounds can interfere with the Bradford assay (e.g. polyphenols) and ELISA (e.g. organic matter), raising questions about precision and accuracy of the quantification with HTCE and ELISA (8, 9).
Due to the co-extracting nature, glomalin is therefore an operationally defined protein of AMF origin, and the term ‘glomalin-related soil proteins’(GRSP) has been widely used to represent a mixture of proteins of both AMF and non-mycorrhizal origin extracted by HTCE (7, 15). The origin of AMF is still controversial due to the presence of the proteins of non-AMF origin in GRSP, especially those of bacteria, although it has been suggested that bacterial proteins contained in GRSP may be a bacterial transformation of AMF exudates and/or necromass, not their product (15). In contrast, many studies have reported positive relationships between the accumulation of GRSP and AMF hyphal growth (16–21), and a higher production of GRSP in soils with plants inoculated by AMF species (22), supporting the causal relationship between AMF and GRSP. Regardless of their origin, GRSP in general contain diverse elements such as C (35-40%), oxygen (33-49%), nitrogen (2-4%), aluminum (1.3-4.8%), and other elements in addition to diverse functional groups, different types of C compounds, and protein-like substances (3).
Glomalin gained popularity owing to increasing interest in their beneficial functions for long-term C sequestration, soil aggregation, and soil remediation and fertility as summarized in Figure 1 (9, 10). Once glomalin is produced within the hyphal wall and released into the surrounding environment, it can form an insoluble protective glue-like layer covering the surface of soil aggregates (19, 23). GRSP is found to contain high concentrations of alkyl C and aromatic C, which contribute to the recalcitrant structure of SOC (11, 24). GRSP are significant components of soil organic matter (SOM), and their hydrophobicity contributes to the chemical stability of SOM leading to slower turnover and thereby holding great importance for long-term soil C sequestration and soil fertility (11). In addition, GRSP consist of N-linked glycoproteins where a variety of carbohydrate residues are covalently linked to the GRSP protein backbone offering reaction sites between GRSP and other soil constituents such as SOM, clays, other soil particles, and nutrients (11). The glycosylated nature of GRSP is assumed to be a key factor for soil micro- and/or macro-aggregation, which nonetheless has not been tested or can be the result of high-temperature citrate extraction (9). Increased aggregation facilitated by GRSP can improve soil hydraulic properties such as water penetration, saturated hydraulic conductivity, infiltrability, porosity, and soil moisture potential as well as water-holding capacity (10, 25). Thus, GRSP can not only contribute to reducing a risk of C loss by soil erosion and water-based degradation such as rain and surface runoff (9, 10, 26) but they also increase drought tolerance in plants (27). In addition, reaction sites present in GRSP can adsorb cations (e.g., heavy metals and nutrients) via its functional groups that provide a negatively charged surface (28). This function allows the GRSP to decrease the bioavailability and toxicity of heavy metals, alongside holding soil cations and increasing soil fertility.
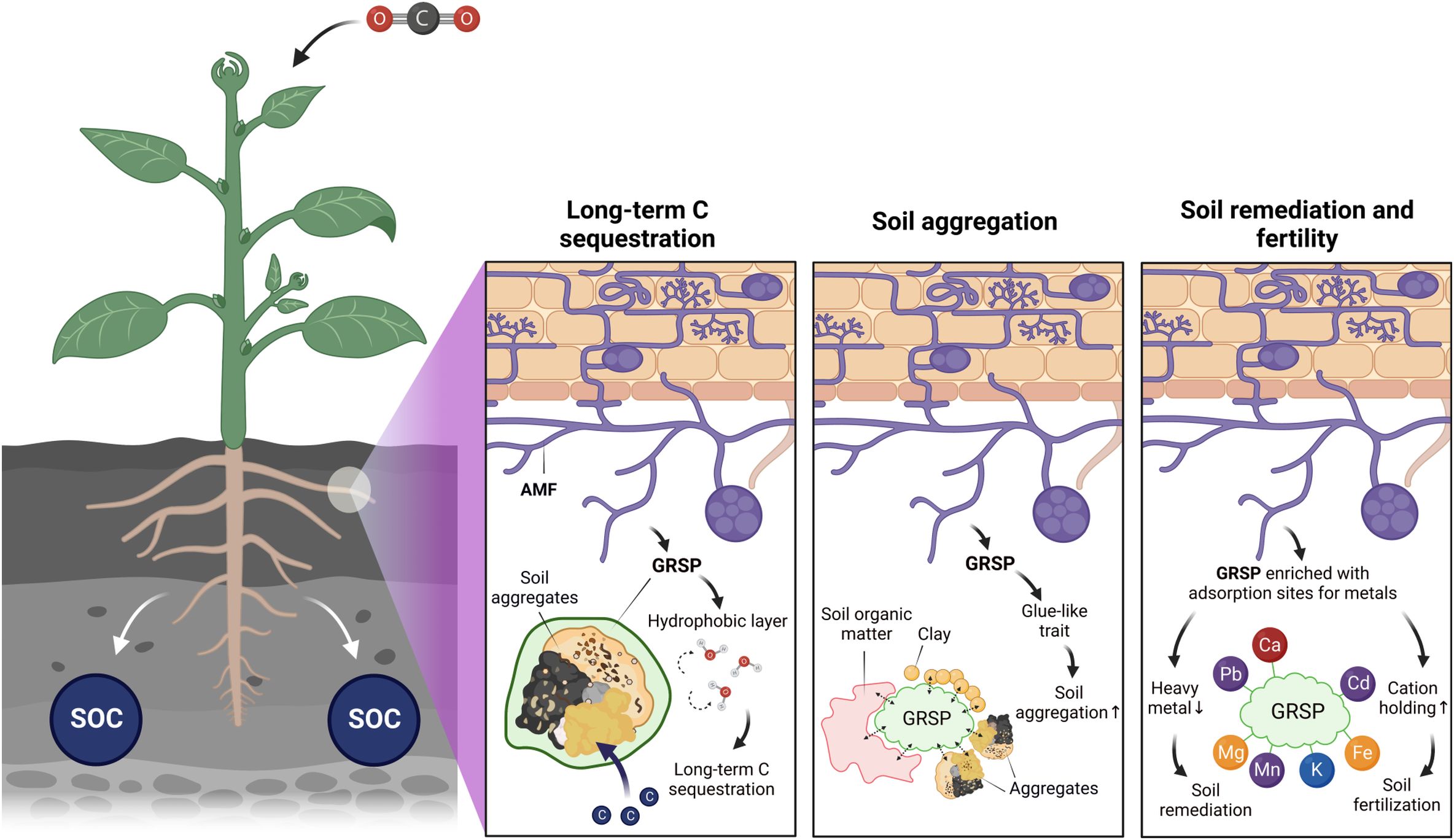
Figure 1. The main benefits of GRSP—long-term carbon (C) sequestration, soil aggregation, and soil remediation and fertilization. Atmospheric CO2 is fixed by plants via photosynthesis, and then the plant C is incorporated into the soil as soil organic carbon (SOC). Arbuscular mycorrhizal fungi (AMF) inoculate the roots of the plant and grow their extraradical hyphal network into the soil, releasing glomalin related soil protein (GRSP) to the soil. GRSP can promote long-term C sequestration by forming a hydrophobic layer around aggregates. GRSP can increase soil aggregation by binding soil organic matter and clays, thus forming microaggregates and macroaggregates using its glue-like trait. GRSP can bind and sequester metals, thereby decreasing their bioavailability and their toxicity in the soil.
Although the importance of GRSP as a key biological player in soil C sequestration and other ecological benefits has been widely recognized, their chemical nature remains unclear due to current methodological challenges and extraction procedures (5, 15). Most chemical studies on GRSP have focused on revealing the individual chemical characteristics of GRSP such as chemical composition (11, 24); soil aggregation mechanism and adsorption behavior (29); heavy metal chelation (30); and organic pollutant removal (31), but less attempts have been made to summarize and interpret these characteristics in the context of soil chemistry. Hence, there is a growing need to collectively review the chemistry and molecular biology of GRSP to understand how its chemical traits relate to the known benefits of long-term C sequestration, soil aggregation, and soil health via enhancing soil remediation and fertility.
2 The hydrophobicity of GRSP
2.1 Hydrophobicity of GRSP explained by disulfide bonds
The GRSP is a homolog of heat shock protein 60 (HSP60) in terms of its secondary and tertiary structures (5). Using tandem liquid chromatography-mass spectrometry, Gadkar and Rillig (5) identified that the partial amino acid sequence of GRSP were 80% identical to the full-length open-reading frame encoding the 590 amino acids of the HSP60 gene in the cDNA library of R. irregularis. Brocchieri and Karlin (32) created the consensus sequences using diverse HSP60 sequences of 43 prokaryotes, called GroEL. They reported that the conserved regions of HSP60 contained ATP/ADP and magnesium binding sites, hydrophobic residues, and charged residues in the center of HSP60, facilitating substrate interaction and hydrophobic core formation. Later, other studies including both prokaryotic and eukaryotic HSP60 sequences revealed that unlike GroEL, eukaryotic HSP60 contained cysteine residues in the conserved region which could contribute to the stability of tertiary structure by forming disulfide bonds (33, 34). Disulfide bridges are known to increase thermodynamic and mechanical stability in a protein, energetically favoring protein folding and increasing the packing of a local cluster of hydrophobic residues and a hydrophobic core (35, 36). Many filamentous fungi such as Ascomycetes and Basidiomycetes produce fungal hydrophobins consisting of eight conserved cysteine residues that form disulfide bridges and self-assemble into various kinds of aggregate as an amphiphile (37, 38). Rillig (23) proposed that GRSP was a homolog of fungal hydrophobins based on its ability to form a hydrophobic coating on soil aggregates with slow turnover and chemical recalcitrance. Compared to the hydroxyl (-OH) group, the sulfhydryl (-SH) group of cysteine prefers to form weak hydrogen bonds with water while establishing covalent bonds between two cysteine residues, and thus creating a hydrophobic layer over the surface of GRSP (Figure 2) (39). Disulfide bonds can limit the exposure of solvent-accessible surfaces such as H-bond donors and acceptors in the unfolded protein due to the hydrophobic effect and a higher entropy of the solvent surrounding the disulfide-containing protein, imposing structural elements that stabilize protein conformation (35). By forming longer covalent bonds of disulfides, the disulfide bonds can increase the intra- and inter-molecular cross-links and thermostability of a protein (40), which may explain the heat-resistance of GRSP.
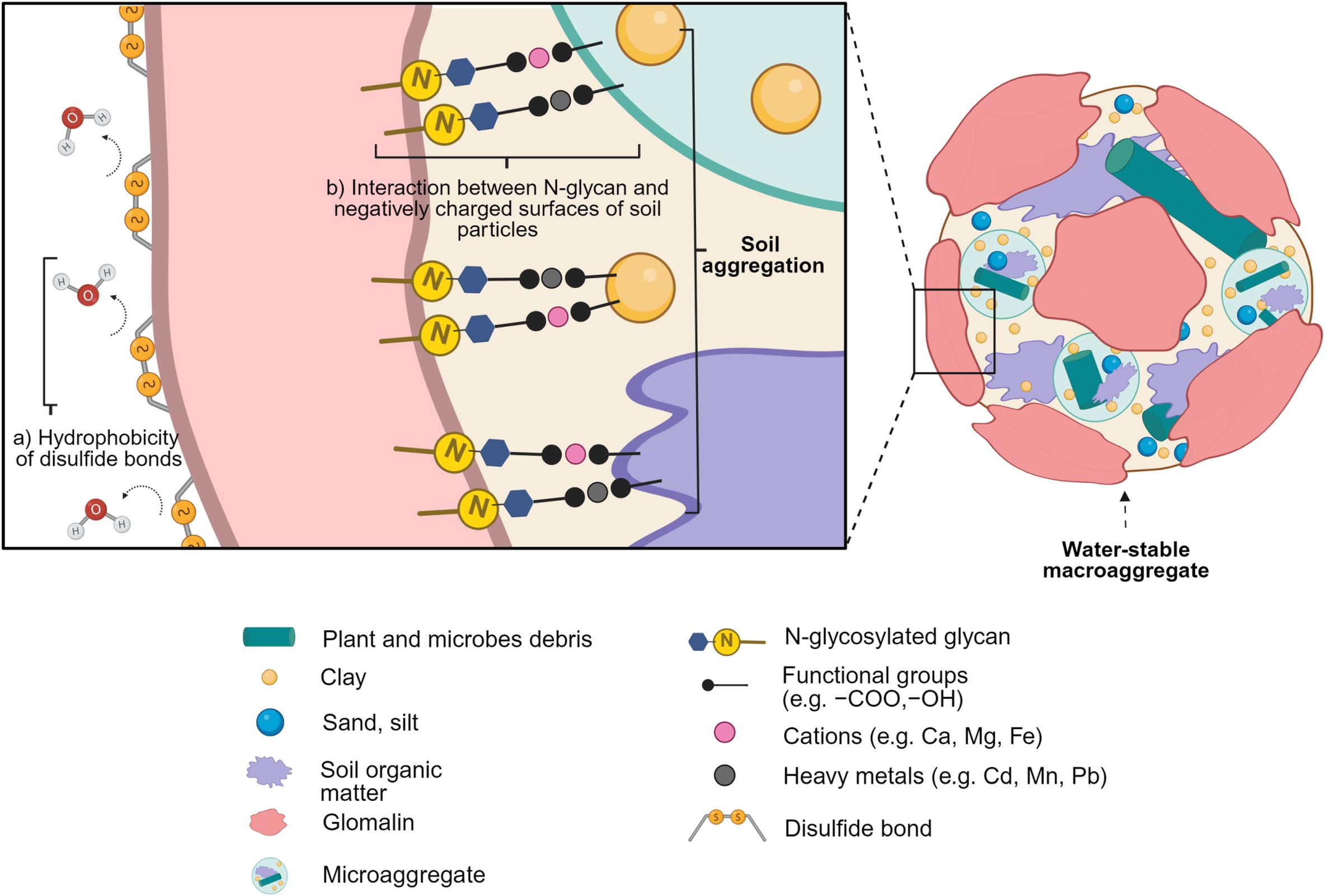
Figure 2. The outline of the formation of a water-stable macroaggregate facilitated by GRSP. Mechanisms a and b are as follows: (A) The disulfide bonds within glomalin can help form a sticky, insoluble, hydrophobic layer around aggregates; (B) the N-glycosylation within glomalin not only expands the reaction sites for cation and heavy metal adsorption but also helps bind soil organic matter, microaggregates, and soil particles, producing a water-stable aggregate.
2.2 The hydrophobicity of GRSP in relation with soil metal composition
The functional disulfide bonds include catalytic and allosteric sites which regulate the functions of a protein, providing a ligand-exchange site, for example, polarizable and anionic thiols (-R-S-) (41). The negative charge on the ligands can be stabilized by a high amount of metal cations in GRSP; when they are coordinated with chalcophile elements such as Fe2+, Zn2+, and Cu2+ (42) and form Fe-S clusters (43). These metals can react with the beta thiol group of cysteine residues, thereby producing a thiyl radical (−S•) (44). Sulfur radicals initiate a free-radical polymerization and a ring-opening polymerization of disulfide moieties both of which convert monomeric GRSP units into a multimeric complex, enhancing the hydrophobicity of GRSP units (43, 45). In addition, GRSP contains other multivalent cations (e.g., Al3+, Mg2+, and Ca2+) which can bind to non-S containing functional groups and induce intramolecular rearrangement by neutralizing the negative charge on the surface of GRSP (46). These processes create a stable GRSP structure, allowing it to increase in size and hydrophobicity.
3 Soil aggregating ability of GRSP
3.1 N-glycosylation of GRSP facilitates soil aggregation
The GRSP is known to promote soil aggregation which is important to soil C sequestration (7). The N-terminal carbohydrates provide glue-like traits that are important for the sorption of proteins to mineral and soil particles (47). Understanding the roles of N-terminal interactions of GRSP with soil particles can unravel the mechanisms underlying soil aggregation. Gadkar and Rillig (5) discovered the presence of N-linked oligosaccharides at its N-terminus, showing its glycoproteinaceous nature. Schindler et al. (11) reported that GRSP was composed of 30–40% protein, with 4–11% aliphatic, 42–49% aromatic, 24–30% carboxyl, and 4–16% carbohydrate-type C content. Due to its glycosylation sites, GRSP can be stabilized by covalent bonding with soil carbohydrates at the side-chain amino groups of lysine and arginine at the N-terminus (48). Mothay and Ramesh (49) visualized the 3D structure of glomalin of R. irregularis based on HSP60 amino acid sequences to understand the molecular interactions and kinetic energy of glomalin with the ligands of SOM. They reported that binding of glomalin to SOM involved hydrogen bonds, electrostatic interactions, and Van der Waals forces. However, hydrophilic interactions were predominant for ligand-protein docking while hydrophobic interactions were more involved in stabilizing protein structure and activity. In fact, a wide range of glycans such as sugars, monosaccharides, oligosaccharides, or polysaccharides can attach to multiple side chains of a protein backbone, determining a diversity of GRSP (3, 50). Furthermore, N-linked sugar moieties can be diversified by phosphorylation, sulfation, methylation, or fatty acylation which can generate more binding sites for a variety of soil particles (51). According to Smith et al. (52), prions with 10 basic amino acid residues at their N-terminus interacted strongly with the negatively charged surface of clays such as montmorillonite due to the positive charges developed on the N-terminal ends. The adsorption of proteins can be greater when oligosaccharides are added to the N-terminus because they expand their reaction sites for adsorption to the exterior of aggregates.
The adsorption process can vary depending on the constituents of the polysaccharides and their different sizes and charges. Polar or charged polysaccharides, such as polysaccharide-N moieties mainly present in proteins, can adsorb to the surface of montmorillonite and kaolinite via electrostatic interaction and H-bonding between carbonyl group in the protein and the Al-OH of montmorillonite and kaolinite edge-sites (53). In contrast, uncharged sugar moieties can increase sorption through hydrophobic interaction by increasing conformational entropy (52). Likewise, N-linked glycans that attach to GRSP can act as extracellular polymeric substances (EPS), forming a sticky and insoluble biofilm that glues surrounding SOM, minerals (i.e., oxides, layer silicates), and microbes (Figure 2). Numerous studies have reported a significant contribution of GRSP to the formation of water-stable macroaggregates, leading to improved soil quality and health by improving the resistance to soil erosion, soil C storage, and nutrient availability (4, 10, 20, 54, 55).
3.2 The adsorption of cations to GRSP can facilitate soil aggregation but depends on soil pH
N-glycan attachment in GRSP provides multiple binding sites for soil cations, such as K, Ca, Mg, Fe, Al, Mn, Zn, Cu, Fe, and Na, to form metal complexes with GRSP (31, 56). Soil microorganisms can exudate biopolymers composed of extracellular proteins and polysaccharides, which might attach to GRSP via N-glycosylation (57). The polysaccharides provide negatively charged active sites, such as carboxyl and hydroxyl groups, that can form complexes with cations (58). The cations within the cation-GRSP complex can also help GRSP make bridges with clays and SOM by increasing the electrostatic flocculation process. Wang et al. (56) reported that the absolute value of the zeta potential declined when GRSP was added to a kaolin suspension, which indicates that the negative charges on the kaolin were balanced and neutralized by the adsorption of the cation-GRSP complex. Hence, this adsorption offers strong binding effects that cause soil particles to stick together and increases soil aggregation, contributing to SOC sequestration (59).
However, adsorption of GRSP onto soil minerals can be dependent on soil pH. According to Wang et al. (60), glycosylated proteins are less stable compared to unglycosylated proteins at a low pH of 4 because sugar chains interact with one another and weakly interact with the protein structure through weak interactions such as H-bonding or van der Waals forces rather than strong ion-bonding. Also, the negative charges of polysaccharides can produce repulsive electrostatic forces at higher pH, because the clay surface is mostly negatively charged and produces a larger amount of endothermic heat during the adsorption at a high pH (53). As the point of zero charge (PZC) of soils varies depending on the mineral constituents, it is a challenge to describe consistent behavior in the adsorption of GRSP. Layer silicate clays have mostly negatively charged surfaces, due to isomorphous substitution at most soil pH values (53). The researchers observed decreasing adsorption with increasing pH because of the progressive deprotonation of functional groups and negative charge development on EPS molecules causing repulsion between EPS and clay surfaces. However, the decreasing patterns of adsorption differed from the type of clay; for montmorillonite and kaolinite, the adsorption of EPS-derived C, N, and P greatly decreased when the pH was higher than their PZC values (3.0 and 3.3, respectively), while with goethite, the adsorption was still high when pH was below 8.3, owing to its high PZC (≈8.3) and the positive charge on EPS at relatively high pH (53). If N-linked glycans of GRSP behave similarly as EPS, the adsorption behavior of GRSP can vary depending on the type of mineral with which they interact. In fact, Tian et al. (61) reported that soil pH was the greatest variable, among other soil parameters such as moisture, depth and salinity, in determining the deposition of GRSP in mangrove wetland soils. Similarly, Wang et al. (29) discovered that in deep and shallow soils, GRSP strongly influenced SOC accumulation, an effect that was largely determined by the soil pH in deep soil. The chemistry of soil aggregation facilitated by GRSP can, therefore, be reliant on soil pH, mineral type, and the composition of related polysaccharides at the N-terminus.
4 Improving soil health: Soil remediation and fertilization by GRSP
4.1 The remediation ability of GRSP by sequestering heavy metals and organic pollutants in the soil
AMF are known for their ability to accumulate heavy metals within the mycorrhizal structures (62). The GRSP have a high affinity for toxic heavy metals such as Pb and Cd, while the immobilization of Al was also recently discovered (63). The GRSP can adsorb toxic metals on their functional groups, including the carboxyl, hydroxyl, and thiol groups that provide a negatively charged surface for heavy metals to bind to, which alleviates heavy metal toxicity (28). In fact, Cornejo et al. (30) discovered that GRSP reduced the bioavailability of total Cu and Zn by 26% and 5.8%, respectively. Similarly, González-Chávez et al. (64) extracted 1.6–4.3 mg Cu, 0.02–0.08 mg Cd, and 0.62–1.12 mg Pb per g glomalin, which were the immobilized forms of the heavy metals, whereby the maximum immobilization rate for Cu was 35%. Organic pollutants were also found adsorbed to GRSP. For example, Chen et al. (31) reported the enhanced sorption of a carcinogenic organic pollutant, phenanthrene, in cation-modified montmorillonites (Ca2+ and Fe3+) when treated with GRSP. They discovered that the sorption process of phenanthrene started with the hydroxyl and carboxyl functional groups of GRSP, which then formed stable complexes with metal cations in the montmorillonites via cation-π interaction. The phenyl rings of phenanthrene then bound with aromatic hydrocarbons and hydroxyl-benzenes in GRSP via π-π electron donor-accepter interaction. Likewise, GRSP, enriched with multiple functional groups, are involved in the retention of diverse heavy metals and organic molecules, thus holding significance for the bioremediation of contaminated soils.
4.2 Soil fertilization and plant growth promotion by GRSP
The GRSP contains numerous cations, such as K, Ca, Mg, Fe, and Al, within their glycoprotein-derived extensive networks of organic polymers (3). As GRSP possess numerous complex ligands, they can retain cations present in the soil solution (65). The cation binding sites are mostly oxidized functional groups such as carboxyl, phenolic, and hydroxyl, all of which are abundant in GRSP (31, 66). The GRSP-bound cations could be used as a nutrient source for soil microbes and plants. In fact, GRSP were found to increase soil microbial activities such as the production of extracellular polysaccharides, glucosidase, urease, catalase, peroxidase, and phosphatase by improving soil quality and providing a food substrate for the microbes all of which benefit plant growth and development (10, 28). Recently, many studies have examined the application of exogenous GRSP on plant growth and nutritional quality (67–70). Xu et al. (70) discovered that peanuts treated with GRSP fertilized with K, Fe, Mg, Zn, and Cu showed improved stomatal opening, photosynthesis, release of photosynthates to the rhizosphere, dry biomass, and pod length and yield as well as higher activity of antioxidative enzymes under drought conditions compared with GRSP alone. This suggests that GRSP-bound nutrients can play important roles in determining plant growth and biology. Liu et al. (67) also found that exogenous GRSP application enhanced the growth of trifoliate orange seedlings, the uptake of N, P, and K, and auxin expression in the roots. Similarly, fruit nutritional quality improves after a foliar application of cation-enriched GRSP for citrus, accompanied by better soil nutrient availability for nitrate, ammonium, P, and K (68). Meng et al. (69) discovered that sweet oranges accumulated more N, P, K, Fe, and Si in their sarcocarp after the application of exogenous GRSP to their foliar parts. The findings may explain the high capacity of GRSP to adsorb nutrients onto its functional groups, which can be subsequently used as a nutrient source for both soil microbes and plants.
5 Conclusion
There are still mysteries surrounding GRSP, including their origin, chemical structure and composition, full genome and amino acid sequences, and phylogenetic history. Nonetheless, the importance of studying GRSP is growing due to the growing threat of climate change alongside soil degradation and pollution. Increasing soil GRSP is crucial for long-term soil C storage, agricultural soil aggregation, and the remediation of polluted lands, all of which promote the environmental sustainability of soils. The GRSP can contribute to forming a water-repellent macroaggregate, by proposing a working model where glomalin-related functional groups interact with N-glycans, cations, heavy metals, and SOM. Although the full structure of glomalin is unknown, this soil protein conglomerate warrants significant attention to facilitate C sequestration in the soil as an important part of mitigating climate change.
Author contributions
YS: Conceptualization, Formal analysis, Investigation, Visualization, Writing – original draft, Writing – review & editing. CM: Supervision, Validation, Writing – original draft, Writing – review & editing. JK-K: Conceptualization, Supervision, Validation, Writing – original draft, Writing – review & editing.
Funding
The author(s) declare financial support was received for the research, authorship, and/or publication of this article. This work was financially supported by the Cornell University Atkinson Center for Sustainability (2021-SGP-Son-ys978) and the Fulbright Scholarship program (PS00298891) to YS. This research was supported in part by the intramural research program of the U.S. Department of Agriculture, National Institute of Food and Agriculture, Hatch program (2019-67013-29364).
Conflict of interest
The authors declare that the research was conducted in the absence of any commercial or financial relationships that could be construed as a potential conflict of interest.
Publisher’s note
All claims expressed in this article are solely those of the authors and do not necessarily represent those of their affiliated organizations, or those of the publisher, the editors and the reviewers. Any product that may be evaluated in this article, or claim that may be made by its manufacturer, is not guaranteed or endorsed by the publisher.
References
1. Lal R. Soil carbon sequestration impacts on global climate change and food security. Science (2004) 304:1623–7. doi: 10.1126/science.1097396
2. Lal R. Carbon sequestration. Philos Trans R Soc B: Biol Sci. (2007) 363:815–30. doi: 10.1098/rstb.2007.2185
3. Agnihotri R, Sharma MP, Prakash A, Ramesh A, Bhattacharjya S, Patra AK, et al. Glycoproteins of arbuscular mycorrhiza for soil carbon sequestration: Review of mechanisms and controls. Sci Total Environ. (2022) 806:150571. doi: 10.1016/j.scitotenv.2021.150571
4. Wright SF, Upadhyaya A. Extraction of an abundant and unusual protein from soil and comparison with hyphal protein of arbuscular mycorrhizal fungi. Soil Sci. (1996) 161:575–86. doi: 10.1097/00010694-199609000-00003
5. Gadkar V, Rillig MC. The arbuscular mycorrhizal fungal protein glomalin is a putative homolog of heat shock protein 60. FEMS Microbiol Lett. (2006) 263:93–101. doi: 10.1111/j.1574-6968.2006.00412.x
6. Magurno F, Malicka M, Posta K, Wozniak G, Lumini E, Piotrowska-Seget Z. Glomalin gene as molecular marker for functional diversity of arbuscular mycorrhizal fungi in soil. Biol Fertil Soils. (2019) 55:411–7. doi: 10.1007/s00374-019-01354-x
7. Rillig MC. Arbuscular mycorrhizae, glomalin, and soil aggregation. Can J Soil. Sci. (2004) 84:355–63. doi: 10.4141/S04-003
8. Rosier CL, Hoye AT, Rillig MC. Glomalin-related soil protein: Assessment of current detection and quantification tools. Soil Biol Biochem. (2006) 38:2205–11. doi: 10.1016/j.soilbio.2006.01.021
9. Irving TB, Alptekin B, Kleven B, Ané J-M. A critical review of 25 years of glomalin research: a better mechanical understanding and robust quantification techniques are required. New Phytol. (2021) 232:1572–81. doi: 10.1111/nph.17713
10. Singh AK, Zhu X, Chen C, Wu J, Yang B, Zakari S, et al. The role of glomalin in mitigation of multiple soil degradation problems. Crit Rev Environ Sci Technol. (2022) 52:1604–38. doi: 10.1080/10643389.2020.1862561
11. Schindler FV, Mercer EJ, Rice JA. Chemical characteristics of glomalin-related soil protein (GRSP) extracted from soils of varying organic matter content. Soil Biol Biochem. (2007) 39:320–9. doi: 10.1016/j.soilbio.2006.08.017
12. Bolliger A, Nalla A, Magid J, de Neergaard A, Dole Nalla A, Bøg-hansen T.C. Re-examining the glomalin-purity of glomalin-related soil protein fractions through immunochemical, lectin-affinity and soil labelling experiments. Soil Biol Biochem. (2008) 40:887–93. doi: 10.1016/j.soilbio.2007.10.019
13. Gillespie AW, Farrell RE, Walley FL, Ross ARS, Leinweber P, Eckhardt K-U, et al. Glomalin-related soil protein contains non-mycorrhizal-related heat-stable proteins, lipids and humic materials. Soil Biol Biochem. (2011) 43:766–77. doi: 10.1016/j.soilbio.2010.12.010
14. Walley FL, Gillespie AW, Adetona AB, Germida JJ, Farrell RE. Manipulation of rhizosphere organisms to enhance glomalin production and C sequestration: Pitfalls and promises. Can J Plant Sci. (2014) 94:1025–32. doi: 10.4141/cjps2013-146
15. Holátko J, Brtnický M, Kučerík J, Kotianová M, Elbl J, Kintl A, et al. Glomalin – Truths, myths, and the future of this elusive soil glycoprotein. Soil Biol Biochem. (2021) 153:108116. doi: 10.1016/j.soilbio.2020.108116
16. Steinberg PD, Rillig MC. Differential decomposition of arbuscular mycorrhizal fungal hyphae and glomalin. Soil Biol Biochem. (2003) 35:191–4. doi: 10.1016/S0038-0717(02)00249-3
17. Lovelock CE, Wright SF, Clark DA, Ruess RW. Soil stocks of glomalin produced by arbuscular mycorrhizal fungi across a tropical rain forest landscape. J Ecol. (2004) 92:278–87. doi: 10.1111/j.0022-0477.2004.00855.x
18. Nichols K, Wright S. Contributions of fungi to soil organic matter in agroecosystems. (Elsevier) (2004). doi: 10.1201/9780203496374.ch6
19. Driver JD, Holben WE, Rillig MC. Characterization of glomalin as a hyphal wall component of arbuscular mycorrhizal fungi. Soil Biol Biochem. (2005) 37:101–6. doi: 10.1016/j.soilbio.2004.06.011
20. Wu Q-S, Cao M-Q, Zou Y-N, He X. Direct and indirect effects of glomalin, mycorrhizal hyphae and roots on aggregate stability in rhizosphere of trifoliate orange. Sci Rep. (2014) 4:5823. doi: 10.1038/srep05823
21. Wang Y-J, He X-H, Meng L-L, Zou Y-N, Wu Q-S. Extraradical mycorrhizal hyphae promote soil carbon sequestration through difficultly extractable glomalin-related soil protein in response to soil water stress. Microb Ecol. (2023) 86:1023–34. doi: 10.1007/s00248-022-02153-y
22. Selvakumar G, Yi PH, Lee SE, Shagol CC, Han SG, Sa T, et al. Effects of long-term subcultured arbuscular mycorrhizal fungi on red pepper plant growth and soil glomalin content. Mycobiology. (2018) 46:122–8. doi: 10.1080/12298093.2018.1461315
23. Rillig MC. A connection between fungal hydrophobins and soil water repellency? Pedobiologia. (2005) 49:395–9. doi: 10.1016/j.pedobi.2005.04.004
24. Zhang J, Tang X, Zhong S, Yin G, Gao Y, He X. Recalcitrant carbon components in glomalin-related soil protein facilitate soil organic carbon preservation in tropical forests. Sci Rep. (2017) 7:2391. doi: 10.1038/s41598-017-02486-6
25. Moebius-Clune BN, van Es HM, Idowu OJ, Schindelbeck RR, Moebius-Clune DJ, Wolfe DW, et al. Long-term effects of harvesting maize stover and tillage on soil quality. Soil Sci Soc America J. (2008) 72:960–9. doi: 10.2136/sssaj2007.0248
26. Le Bissonnais Y. Aggregate stability and assessment of soil crustability and erodibility: I. Theory and methodology. Eur J Soil Sci. (1996) 47:425–37. doi: 10.1111/j.1365-2389.1996.tb01843.x
27. Chi G-G, Srivastava AK, Wu Q-S. Exogenous easily extractable glomalin-related soil protein improves drought tolerance of trifoliate orange. Arch Agron Soil Sci. (2018) 64:1341–50. doi: 10.1080/03650340.2018.1432854
28. Gujre N, Agnihotri R, Rangan L, Sharma MP, Mitra S. Deciphering the dynamics of glomalin and heavy metals in soils contaminated with hazardous municipal solid wastes. J Hazardous Materials. (2021) 416:125869. doi: 10.1016/j.jhazmat.2021.125869
29. Wang W, Zhong Z, Wang Q, Wang H, Fu Y, He X. Glomalin contributed more to carbon, nutrients in deeper soils, and differently associated with climates and soil properties in vertical profiles. Sci Rep. (2017) 7:13003. doi: 10.1038/s41598-017-12731-7
30. Cornejo P, Meier S, Borie G, Rillig MC, Borie F. Glomalin-related soil protein in a Mediterranean ecosystem affected by a copper smelter and its contribution to Cu and Zn sequestration. Sci Total Environ. (2008) 406:154–60. doi: 10.1016/j.scitotenv.2008.07.045
31. Chen S, Sheng X, Qin C, Waigi MG, Gao Y. Glomalin-related soil protein enhances the sorption of polycyclic aromatic hydrocarbons on cation-modified montmorillonite. Environ Int. (2019) 132:105093. doi: 10.1016/j.envint.2019.105093
32. Brocchieri L, Karlin S. Conservation among HSP60 sequences in relation to structure, function, and evolution. Protein Sci. (2000) 9:476–86. doi: 10.1110/ps.9.3.476
33. Cappello F, Marino Gammazza A, Palumbo Piccionello A, Campanella C, Pace A, Conway de Macario E, et al. Hsp60 chaperonopathies and chaperonotherapy: targets and agents. Expert Opin Ther Targets. (2014) 18:185–208. doi: 10.1517/14728222.2014.856417
34. Tikhomirova TS, Matyunin MA, Lobanov MY, Galzitskaya OV. In-depth analysis of amino acid and nucleotide sequences of Hsp60: How conserved is this protein? Proteins: Structure Function Bioinf. (2022) 90:1119–41. doi: 10.1002/prot.26294
35. Feige MJ, Braakman I, Hendershot LM. CHAPTER 1.1 disulfide bonds in protein folding and stability. (Springer Berlin Heidelberg) (2018). pp. 1–33. doi: 10.1039/9781788013253-00001
36. Wedemeyer WJ, Welker E, Narayan M, Scheraga HA. Disulfide bonds and protein folding. Biochemistry. (2000) 39:4207–16. doi: 10.1021/bi992922o
37. Linder MB, Szilvay GR, Nakari-Setälä T, Penttilä ME. Hydrophobins: the protein-amphiphiles of filamentous fungi. FEMS Microbiol Rev. (2005) 29:877–96. doi: 10.1016/j.femsre.2005.01.004
38. Li X, Wang F, Xu Y, Liu G, Dong C. Cysteine-rich hydrophobin gene family: genome wide analysis, phylogeny and transcript profiling in cordyceps militaris. Int J Mol Sci. (2021) 22:643. doi: 10.3390/ijms22020643
39. Iyer BR, Mahalakshmi R. Hydrophobic characteristic is energetically preferred for cysteine in a model membrane protein. Biophys J. (2019) 117:25–35. doi: 10.1016/j.bpj.2019.05.024
40. Liu T, Wang Y, Luo X, Li J, Reed SA, Xiao H, et al. Enhancing protein stability with extended disulfide bonds. Proc Natl Acad Sci. (2016) 113:5910–5. doi: 10.1073/pnas.1605363113
41. Cook KM. Determining the redox potential of a protein disulphide bond. In: Hogg P, editor. Functional disulphide bonds: methods and protocols. Springer, New York, NY (2019). p. 65–86. doi: 10.1007/978-1-4939-9187-7_5
42. Linsdell P. Metal bridges to probe membrane ion channel structure and function. Biomolecular Concepts. (2015) 6:191–203. doi: 10.1515/bmc-2015-0013
43. Nagano N, Ota M, Nishikawa K. Strong hydrophobic nature of cysteine residues in proteins. FEBS Lett. (1999) 458:69–71. doi: 10.1016/S0014-5793(99)01122-9
44. Góngora-Benítez M, Tulla-Puche J, Albericio F. Multifaceted roles of disulfide bonds. Peptides as therapeutics. Chem Rev. (2014) 114:901–26. doi: 10.1021/cr400031z
45. Pięta M, Purohit VB, Pietrasik J, M. Plummer C. Disulfide-containing monomers in chain-growth polymerization. Polymer Chem. (2023) 14:7–31. doi: 10.1039/D2PY01291J
46. Polubesova T, Sherman-Nakache M, Chefetz B. Binding of pyrene to hydrophobic fractions of dissolved organic matter: effect of polyvalent metal complexation. Environ Sci Technol. (2007) 41:5389–94. doi: 10.1021/es070722r
47. Gunina A, Kuzyakov Y. Sugars in soil and sweets for microorganisms: Review of origin, content, composition and fate. Soil Biol Biochem. (2015) 90:87–100. doi: 10.1016/j.soilbio.2015.07.021
48. Rillig MC, Caldwell BA, Wösten HAB, Sollins P. Role of proteins in soil carbon and nitrogen storage: controls on persistence. Biogeochemistry. (2007) 85:25–44. doi: 10.1007/s10533-007-9102-6
49. Mothay D, Ramesh KV. Computational studies of mycorrhizal protein: GiHsp60 and its interaction with soil organic matter. Curr Sci. (2021) 120:389–97. doi: 10.18520/cs/v120/i2/389-397
50. Ma B, Guan X, Li Y, Shang S, Li J, Tan Z. Protein glycoengineering: an approach for improving protein properties. Front Chem. (2020) 8. Available online at: https://www.frontiersin.org/articles/10.3389/fchem.2020.00622.
51. Berninsone PM. Carbohydrates and glycosylation, in: WormBook (2018). Available online at: https://www.ncbi.nlm.nih.gov/books/NBK116083/ (Accessed November 13, 2022).
52. Smith CB, Booth CJ, Pedersen JA. Fate of prions in soil: A review. J Environ Qual. (2011) 40:449–61. doi: 10.2134/jeq2010.0412
53. Lin D, Ma W, Jin Z, Wang Y, Huang Q, Cai P. Interactions of EPS with soil minerals: A combination study by ITC and CLSM. Colloids Surfaces B: Biointerfaces. (2016) 138:10–6. doi: 10.1016/j.colsurfb.2015.11.026
54. Franzluebbers A, Wright SF, Stuedemann J. Soil aggregation and glomalin under pastures in the southern piedmont USA. Soil Sci Soc America J. (2000) 64:1018–26. doi: 10.2136/sssaj2000.6431018x
55. Rillig MC, Wright SF, Eviner VT. The role of arbuscular mycorrhizal fungi and glomalin in soil aggregation: comparing effects of five plant species. Plant Soil. (2002) 238:325–33. doi: 10.1023/A:1014483303813
56. Wang Q, Hong H, Liao R, Yuan B, Li H, Lu H, et al. Glomalin-related soil protein: The particle aggregation mechanism and its insight into coastal environment improvement. Ecotoxicology Environ Saf. (2021) 227:112940. doi: 10.1016/j.ecoenv.2021.112940
57. Redmile-Gordon M, Gregory AS, White RP, Watts CW. Soil organic carbon, extracellular polymeric substances (EPS), and soil structural stability as affected by previous and current land-use. Geoderma. (2020) 363:114143. doi: 10.1016/j.geoderma.2019.114143
58. Potts M. Desiccation tolerance of prokaryotes. Microbiol Rev. (1994) 58:755–805. doi: 10.1128/mr.58.4.755-805.1994
59. Rowley MC, Grand S, Verrecchia É.P. Calcium-mediated stabilisation of soil organic carbon. Biogeochemistry. (2018) 137:27–49. doi: 10.1007/s10533-017-0410-1
60. Wang W, Antonsen K, Wang YJ, Wang DQ. pH dependent effect of glycosylation on protein stability. Eur J Pharm Sci. (2008) 33:120–7. doi: 10.1016/j.ejps.2007.10.008
61. Tian Y, Yan C, Wang Q, Ma W, Yang D, Liu J, et al. Glomalin-related soil protein enriched in δ13C and δ15N excels at storing blue carbon in mangrove wetlands. Sci Total Environ. (2020) 732:138327. doi: 10.1016/j.scitotenv.2020.138327
62. Gaur A, Adholeya A. Prospects of arbuscular mycorrhizal fungi in phytoremediation of heavy metal contaminated soils. Curr Sci. (2004) 86:528–34.
63. Vlček V, Pohanka M. Glomalin – an interesting protein part of the soil organic matter. Soil Water Res. (2020) 15:67–74. doi: 10.17221/29/2019-SWR
64. González-Chávez MC, Carrillo-González R, Wright SF, Nichols KA. The role of glomalin, a protein produced by arbuscular mycorrhizal fungi, in sequestering potentially toxic elements. Environ Pollut. (2004) 130:317–23. doi: 10.1016/j.envpol.2004.01.004
65. Zhang Z, Wang Q, Wang H, Nie S, Liang Z. Effects of soil salinity on the content, composition, and ion binding capacity of glomalin-related soil protein (GRSP). Sci Total Environ. (2017) 581–582:657–65. doi: 10.1016/j.scitotenv.2016.12.176
66. Wang Z, Yao Y, Yang Y. Fulvic acid-like substance-Ca(II) complexes improved the utilization of calcium in rice: Chelating and absorption mechanism. Ecotoxicology Environ Saf. (2022) 237:113502. doi: 10.1016/j.ecoenv.2022.113502
67. Liu R-C, Gao W-Q, Srivastava AK, Zou Y-N, Kuča K, Hashem A, et al. Differential effects of exogenous glomalin-related soil proteins on plant growth of trifoliate orange through regulating auxin changes. Front Plant Sci. (2021) 12:745402. doi: 10.3389/fpls.2021.745402
68. Liu X-Q, Xie Y-C, Li Y, Zheng L, Srivastava AK, Hashem A, et al. Biostimulatory response of easily extractable glomalin-related soil protein on soil fertility mediated changes in fruit quality of citrus. Agriculture. (2022) 12:1076. doi: 10.3390/agriculture12081076
69. Meng L-L, Liang S-M, Srivastava AK, Li Y, Liu C-Y, Zou Y-N, et al. Easily extractable glomalin-related soil protein as foliar spray improves nutritional qualities of late ripening sweet oranges. Horticulturae. (2021) 7:228. doi: 10.3390/horticulturae7080228
70. Xu F-J, Zhang A-Y, Yu Y-Y, Sun K, Tang M-J, Zhang W, et al. Soil legacy of arbuscular mycorrhizal fungus Gigaspora margarita: The potassium-sequestering glomalin improves peanut (Arachis hypogaea) drought resistance and pod yield. Microbiological Res. (2021) 249:126774. doi: 10.1016/j.micres.2021.126774
Keywords: glomalin-related soil protein, soil aggregation, climate change, arbuscular mycorrhizal fungi, carbon sequestration, soil health, soil remediation, microbial protein
Citation: Son Y, Martínez CE and Kao-Kniffin J (2024) Three important roles and chemical properties of glomalin-related soil protein. Front. Soil Sci. 4:1418072. doi: 10.3389/fsoil.2024.1418072
Received: 16 April 2024; Accepted: 09 September 2024;
Published: 27 September 2024.
Edited by:
Muhammad Shaukat, Allama Iqbal Open University, PakistanReviewed by:
Sadam Hussain, Northwest A&F University, ChinaCopyright © 2024 Son, Martínez and Kao-Kniffin. This is an open-access article distributed under the terms of the Creative Commons Attribution License (CC BY). The use, distribution or reproduction in other forums is permitted, provided the original author(s) and the copyright owner(s) are credited and that the original publication in this journal is cited, in accordance with accepted academic practice. No use, distribution or reproduction is permitted which does not comply with these terms.
*Correspondence: Jenny Kao-Kniffin, anRrNTdAY29ybmVsbC5lZHU=