- 1Institut für Physik, Carl-von-Ossietzky Universität Oldenburg, Oldenburg, Germany
- 2Research Centre for Neurosensory Sciences, Carl von Ossietzky University of Oldenburg, Oldenburg, Germany
- 3Center for Nanoscale Dynamics (CENAD), Carl von Ossietzky University of Oldenburg, Oldenburg, Germany
- 4Instituto de Física Gleb Wataghin, Universidade Estadual de Campinas, Campinas, São Paulo, Brazil
Quantum biology is a modern field of research that aims to understand how quantum effects can affect the chemistry underlying various biological processes. This paper reviews several examples of biological processes where quantum effects might play a notable role. Initially, the photon capture mechanism present in vision is discussed, where the energy of the photon is used to cause conformational changes to chromophoric proteins. The second example elaborates the highly efficient energy transfer process present in photosynthesis and discusses, in particular, how the random quantum walk process may enhance the performance drastically. Subsequently, the vertebrate magnetoreception, and the possible associated role of the radical pair mechanism in the process is considered. The review concludes with the discussion of some speculative ideas of putative quantum effects arising in neural processes.
1 Introduction
One of the first mentions of the idea of quantum biology can be traced back to the book “What is life?” (Schrödinger, 1944). Quantum biology studies the applications of quantum mechanics and theoretical chemistry to biological systems. The field of quantum biology aims to fundamentally understand how biological processes that rely on quantum effects work. With the development of computational chemistry techniques, the growth of quantum thermodynamics and approaches to study open quantum systems, it is possible to form a more fundamental understanding of the complex systems present in biology. In particular, this review is an introduction for anyone interested in understanding some of the possible ways how quantum mechanics may be relevant in several selected biological processes. Furthermore, a discussion of four biological processes – three well-established and a more speculative one – where quantum mechanisms possibly play a role is performed and guides the reader to a more detailed investigation on each subject. Although this review explores mainly quantum mechanical mechanisms, there are just as many proposals for classical or semiclassical descriptions of the processes discussed here (Cadiou and McNaughton, 2010; Runeson et al., 2022). We do not intend to discuss the existing controversies in detail and leave this discussion to more specialized reviews (McFadden and Al-Khalili, 2018; Marais et al., 2018; Cao et al., 2020) on the topics. Indeed we would like to briefly mention that quantum processes may need to be carefully addressed in the context of multiple biology processes.
Section 2 discusses the quantum effects in vision, by overviewing the photo-absorption process in related molecules. The semi-classical mechanism of light detection is discussed, explaining how vision functions. This mechanism involves a retinal molecule which, when excited by a photon, can undergo different energy decay paths depending on the photon’s energy and its molecular environment. Most organisms use similar mechanisms for photo-detection (Schulten and Hayashi, 2014). Vision requires a quantum or at least semi-classical description in which all or part of the system is described via the quantization of its states. However, the mechanism in vision does not require any degree of coherence or entanglement due to the superposition of the energetic states. On the other hand, there are examples of biological processes that might require a degree of coherence for efficient operation - as is the case with photosynthesis and vertebrate magnetoreception.
Section 3 illustrates how the efficiency of energy transfer in photosynthesis in bacteria may reach up to
Section 4 explores vertebrate magnetoreception, and discribes experimental evidence which suggests that migratory songbirds require light of specific wavelengths to utilize their magnetic compass (Mouritsen and Hore, 2012; Mouritsen, 2018; Wiltschko et al., 2010; Engels et al., 2012). Such behaviour could not be rationalized for a compass sense based on magnetic materials (Solov’yov and Greiner, 2009; Solov’yov and Greiner, 2008; Wiltschko and Wiltschko, 2012). The section discusses a possible molecular mechanism for the Earth’s magnetic field detection, where correlated energy states in a receptor molecule play an essential role. This mechanism, called the radical pair mechanism, aims to explain how migratory songbirds can perceive the direction of the geomagnetic field without the use of magnetic minerals (Hore and Mouritsen, 2016). The radical pair mechanism is rooted upon the blue light-sensitive proteins present in the eyes of some bird species (Cashmore et al., 1999; Liedvogel et al., 2007; Mouritsen et al., 2004).
As a last part of the review, a subject that has been gaining relevance in recent years is presented – the potential role of quantum mechanics in brain function, particularly concerning consciousness (Li et al., 2018; Smith et al., 2021; Cukras and Sadlej, 2021). This section of the review provides a discussion of key theories, including the possible implications of quantum entanglement, coherence, and superposition in neural processes (Hagan et al., 2002; Tegmark, 2000; Froehlich, 1968; Kalra et al., 2023a; Liu et al., 2024; Babcock et al., 2024). The challenges and controversies surrounding the raised hypothesis are addressed, along with future directions for research, highlighting recent advancements.
2 Vision
Vision is a common ability in complex living beings, defined as the ability to detect light and use it to interpret the environment (Starr et al., 2006; Moazed, 2023). A similar mechanism is used by bacteria to guide their locomotion towards or away from a light source; the basic mechanism is similar to photodetection in higher organisms, but does not function as a visual sensor. The basic molecular mechanism of vision relies on the protein rhodopsin (Rh) (Khorana, 1992; Pedram et al., 2022), in the case of animals, or its variant bacteriorhrodopsin (bRh) (Spudich and Jung, 2005), in the case of bacteria. Central to rhodopsin’s structure is the chromophore retinal (Figure 1), which governs the quantum processes involved in photo-detection. A chromophore is a molecule embedded in the protein which has the function of absorbing light of a particular wavelength to start a cascade of chemical reactions (Rüdiger, 1986).Upon capturing a photon, retinal enters an electronically excited state and subsequently undergoes a twist in one of its chemical bonds causing the molecule to change into a new conformation (Figure 1B), the change in conformation is called cis to trans transformation (Joly, 1921; Loulakis et al., 2017; Sen et al., 2022). This conformational change initiates a cascade of chemical reactions related to the visual cycle (Wald, 1968; Mohseni and Plenio, 2014), which signals the photo-detection.
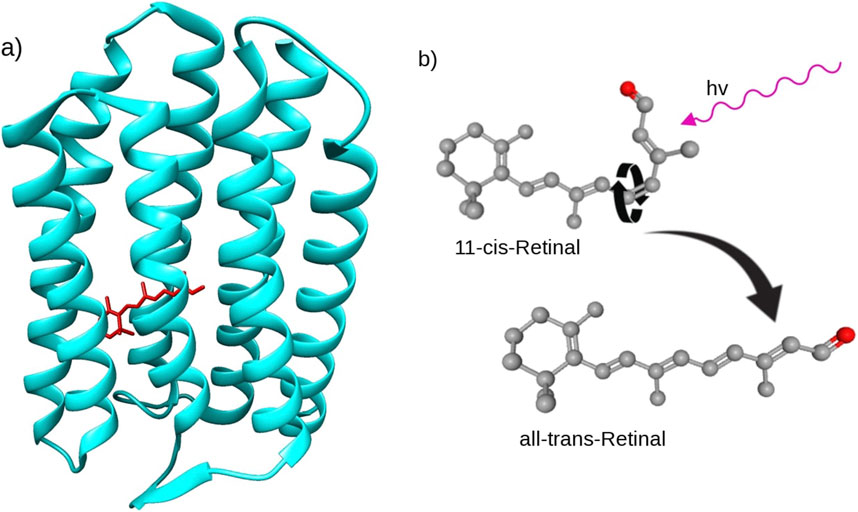
Figure 1. (A) Structure of sensory rhodopsin from Natronomonas pharaonis, the retinal chromophore in all-trans form is highlighted in red inside of the protein (Royant et al., 2001). (B) Upon photo-absorption retinal undergoes a conformational change from 11-cis-Retinal to all-trans-Retinal (National Center for Biotechnology Information, 2024b; National Center for Biotechnology Information, 2024a)). The hydrogen atoms in the molecule are not shown for visualization clarity, grey and red spheres indicate carbon and oxygen atoms, respectively.
The quantum aspect of vision is related to the changes of the energetic states of the molecule, and the mechanism of how those states define the function of the molecule. Only photons of specific wavelengths can be absorbed by the retinal, and those wavelengths are determined by the molecular quantized energy states at the moment of interaction between the retinal and the photon. In an idealized case, the retinal would only be able to absorb energy of one specific wavelength. Still, many different factors affect retinal’s energy spectrum, making it sensitive to a specific range of wavelengths, a phenomena called line broadening in spectroscopy (Haken and Wolf, 1996). Upon photo-absorption the molecule enters an electronically excited state, but eventually decays back to a ground state. One possibility for decaying into a less energetic state, is to use the energy received from the photon to cause conformational changes within the molecule, e.g., to exploit the transition from 11-cis-retinal to all-trans-retinal (Wald, 1968). But there are other multiple possible ways the energy of the absorbed photon can be dissipated (Figure 2), impacting the efficiency of the photodetection mechanism.
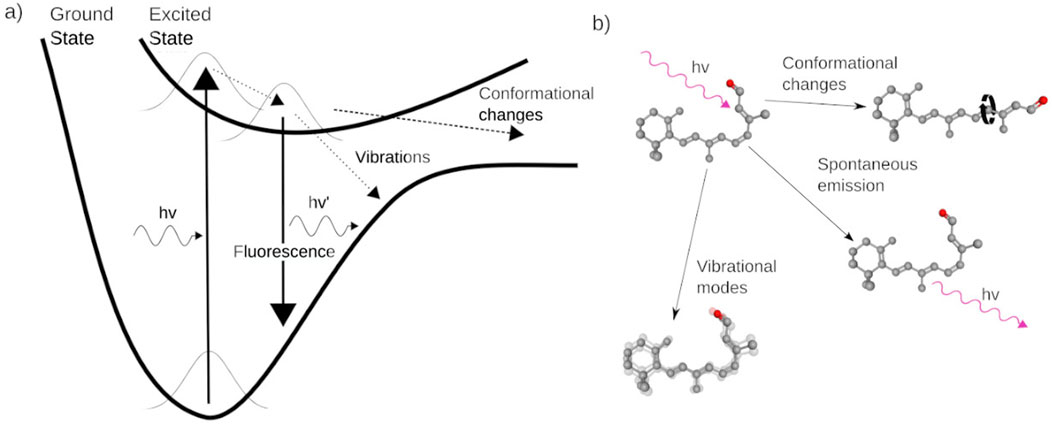
Figure 2. (A) Energy diagram illustrating the possible paths of energy transfer, following photoabsorption by the retinal. (B) Artistic illustration of the possible ways that the energy of the absorbed photon can be dissipated. Vibrational modes in the protein, cause the molecular bonds to vibrate and dissipate the extra energy throughout the whole protein. If no changes have occurred, all the energy of the photon can be spontaneously emitted as another photon of similar energy. The energy of the absorbed photon can also cause a conformational change in the molecule.
The retinal molecule could revert to the original ground state by emitting a photon with a similar energy as the original one via spontaneous emission (Valeur and Berberan-Santos, 2011); the process of dissipating energy through photon emission is called fluorescence. Alternatively the retinal molecule may undergo an adiabatic transition, when the molecule changes its state without radiating photons; the molecule transitions between different states while being on the same adiabatic energy surface (Truhlar, 2003). As the molecule transitions between states, it can dissipate the excess energy to the environment in the form of vibrational modes (Mohseni and Plenio, 2014), transferring the energy to the chemical bonds and, eventually, dissipating the photon’s energy as vibrations throughout the protein. If the energy from the captured photon was not dissipated, and indeed caused a conformational change, then a series of chemical reaction follows (Wald, 1968).
Despite decades of research, questions remain related to the critical understanding of the environmental influence around the retinal. The excitation properties of retinal depend on its molecular environment. Changing the environment also changes the sensitivity of retinal to specific wavelengths, e.g., a chromophore may become affected by red, green or blue light if put in different environments. It is not trivial to describe the effect of the environment onto the absorption spectra of molecules. The surrounding environment, in the case of vision, is complex (see Figure 1) and affects how the energy of the captured photon can be dissipated (Figure 2B). In theoretical calculations, one can include the environmental influence into the retinal by using i.e., polarizable embedding models, where a small region of the system is treated with quantum mechanical methods, and the environment is represented by multipoles and polarizabilities (Steinmann et al., 2019; Frederiksen et al., 2024b; Kretschmer et al., 2024; Di Prima et al., 2024). Solvatochromism (Marini et al., 2010; Mennucci et al., 1998) (Figure 3) is one notable example of how the environment affects a molecule’s spectra. In this case the properties of the solvent affect the dye’s quantum excitations, which changes which photon wavelengths the dye absorbs. While photoabsorption is one of the most prominent examples for the requirement of a quantum mechanical description, there are a plethora of other processes which include quantum effects. For example, the energy transport of the absorbed light in rhodopsin in avian species was investigated previously, where it was illustrated that quantum effects are required for an efficient energy transportation (Zueva et al., 2019). The concept of quantum effect guided energy transportation is also a current research topic within the photosynthetic system of many species (Mohseni et al., 2008; Zhu et al., 2010; Dudhe et al., 2022) as will be described in the next section.
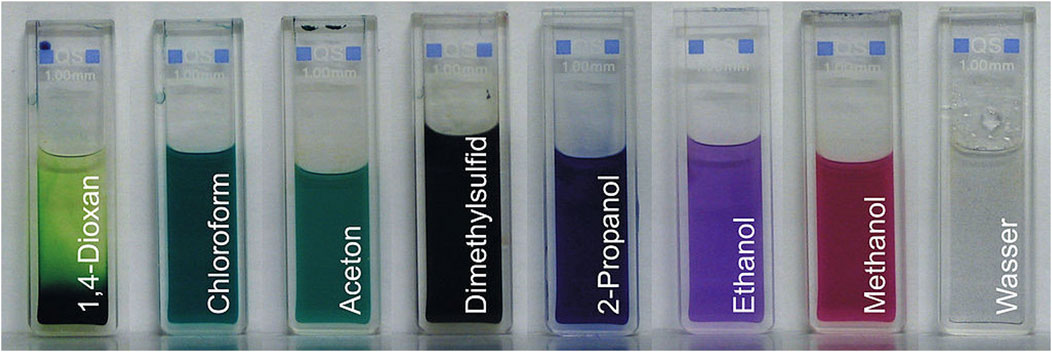
Figure 3. Reichardt’s dye (Osterby and McKelvey, 1996) dissolved in different solutions (labeled). Dielectric constant, hydrogen bonding capacity and other properties of the solution affects the quantum excitations of the dye, changing its absorption and emission spectra.
3 Photosynthesis
Photosynthesis is a well studied biological process (Zhu et al., 2010) that permits plants and some bacteria to store energy harvested from photo-absorption. The complete process of photosynthesis is rather complex, so the focus here is set to the photosynthetic apparatus present in purple bacteria (Chlorobaculum Tepidum) which transports the energy from the antenna, a molecular structure dedicated to capturing light, to the reaction center, where the energy is used to synthesize glucose. The captured photon excites an electron in the antenna, making the electron leave its current energy site. The excitation of the electron forms a region with an absent negative charge, defined as a hole and can be assumed as a virtual particle with a positive charge. The electron-hole pair acts as a quasiparticle called exciton. The energy is transported as an exciton (Fox, 2010; Mohseni et al., 2008; Ghasemi and Shafiee, 2020; Dudhe et al., 2022) through the Fenma-Mattheus-Olson (FMO) protein complex (Figure 4) (Fenna and Matthews, 1975; Dudhe et al., 2022; Tronrud et al., 2009) found in purple photosynthetic bacteria (Ritz et al., 2001; Ritz et al., 1998; Spudich and Jung, 2005). The FMO has embedded multiple Bactoriochlorophyll (BChl) molecules, the BChl serve as sites, where the exciton can diffuse from site to site from the antenna, near sites 1, 2, and 6, to the reaction center near site 3.
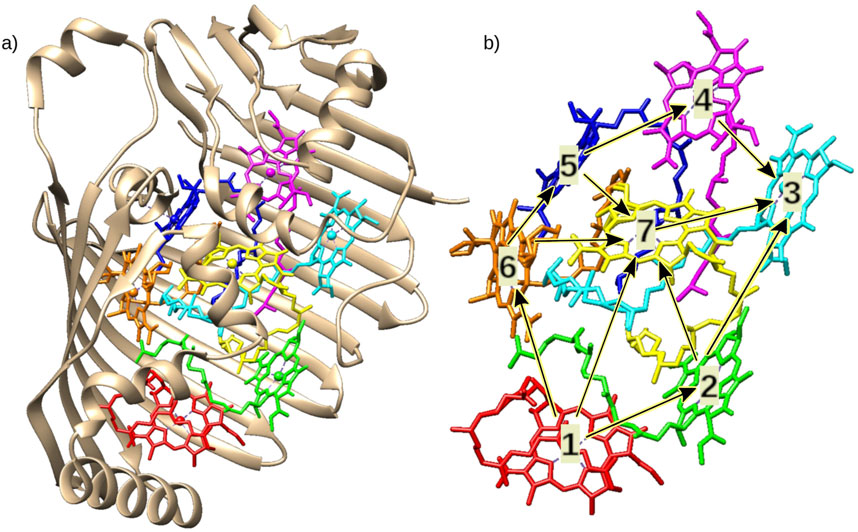
Figure 4. (A) Fenna–Matthews–Olson protein from Chlorobaculum Tepidum (Tronrud et al., 2009) with Bacteriochlorophyll a (BChl a) molecules highlighted inside. Each color represents a chromophore molecule with localized site energy. The sphere in the center of each molecule denotes a magnesium atom. (B) Bacteriochlorophyll numbered following Fenna and Matthews original numbering convention (Fenna and Matthews, 1975). Site 1, 2 and 6 are closer to the antenna (donor), and site 3 is closer to the reaction center (acceptor) (Mohseni et al., 2008). The arrows show some possible pathways for the exciton to diffuse and reach site 3, getting closer to the reaction center.
The energy transport has a high degree of efficiency in bacteria. About
Random walk is a widely known approach from statistical physics. For the sake of illustration consider a particle that experiences one dimensional (1D) random walk. The particle could, for example, symbolize an exciton that exists in the FMO, although the exciton’s real motion would be much more complex. Assume the particle to be initially placed at the origin. In the 1D random walk it experiences jumps in two possible directions (positive and negative). In the simplest scenario the particle may be displaced by one unit per jump. In the case of a classical random walk, after a finite number of jumps, the probability distribution of the particle’s positions approaches the normal distribution, as illustrated in Figure 5. The width of this distribution grows with the increase of the number of jumps. After a sufficiently large number of jumps, in the classical scenario, the probability of finding the particle at any point becomes similar. On contrary, if the number of jumps is finite, the classical particle has a tendency to localize around its starting position.
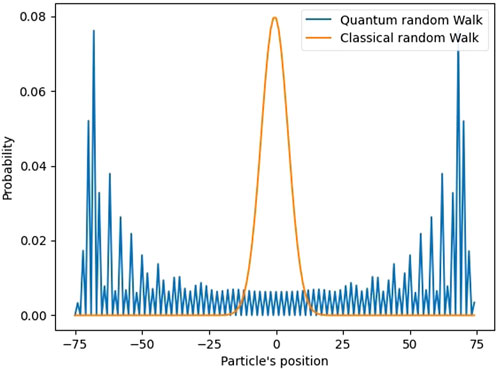
Figure 5. Normalized probability distribution computed for a particle experiencing a 1D classical random walk (orange line) and a quantum random walk (blue line) after 100 steps.
Quantum walk is a variation of the random walk where the movement of the particle is governed by quantum mechanics (Tang et al., 2024). Instead of considering the specific particle, in quantum random walk one considers the particle’s wave-function. To represent the particle starting at the origin, its initial wave-function starts as a spike at the origin with the probability to be observed elsewhere being zero. With each jump the wave-function propagates freely, i.e., analogous to the classical “jump” introduced above. The quantum jump is usually represented by the propagation operator. As the number of jumps increases, the wave-function starts to interfere with itself, causing the probability of the particle being in previous positions to decrease. After a certain number of jumps an interference pattern emerges. The particle has a higher probability of being on the extremities of the 1D space, as opposed to the classical random walk where the particle has higher probability of being around the origin (Nsofini, 2012). The higher probability of the particle being at the periphery of the diffusion limit is the reason why quantum walk is theorized as the source of the FMO’s high efficiency in energy transport (Olaya-Castro et al., 2008; Mohseni et al., 2008; Hoyer et al., 2010). If the exciton diffuses through a quantum walk, it tends to spread around the molecule, instead of remaining around the point of origin. Although the quantum walk illustrated here is a simplified example, studies which explore the quantum walk in photosynthesis use a more refined version of this model, where more transport possibilities are considered, and with the presence of different potentials which affect the wave-function’s dynamics (Mohseni et al., 2008; Hoyer et al., 2010).
Figure 4 introduces a possible excitation pathway in the FMO. Following the numbering scheme in Figure 4, the energy is transferred from sites 1, 2 or 6, which are closer to the antenna, to site 3, which in turn is closer to the reaction center. The existence of quantum walk in the energy transfer is still under discussion (Runeson et al., 2022). The main argument in favor of quantum walk is that it might cause an enhancement in the energy transfer rate, since the particle localization probability experiencing a quantum walk tends to spread out more then in the classical random walk. Some works also suggest environment assisted mechanisms for the quantum walk, where environmental noise could aid transport transfer pathways (Caruso et al., 2009; Plenio and Huelga, 2008; Mohseni et al., 2008). However, other studies point out that the energy transfer rate increase within the quantum random walk is not guaranteed (Dudhe et al., 2022; Hoyer et al., 2010). These studies suggest that the energy transfer is optimized for efficiency instead of speed. Other quantum effects, such as entanglement (Sarovar et al., 2010; Fassioli and Olaya-Castro, 2010; Whaley et al., 2011; Ishizaki and Fleming, 2010) or Grover’s quantum search (Engel et al., 2007), are theorized to be the source of the high efficiency (Karafyllidis, 2017). An argument against quantum effects is the short decoherence time at room temperature, which would greatly limit the lifetime of emerging quantum states. Runeson et al. (2022) presents a counter example to the quantum walk hypothesis, where the authors use trajectory-based simulations to show that a description in terms of quantum electrons and classical nuclei is sufficient to describe the efficiency of the FMO’s energy transfer.
4 Vertebrate magnetoreception
The phenomenon of magnetoreception is observed in various organisms, including bacteria, insects, amphibians, birds, sharks, fish and rays, which use it to orient themselves to the Earth’s magnetic field (Wiltschko, 2012; Grüning et al., 2022; Solov’yov et al., 2014; Maeda et al., 2012; Maeda et al., 2008; Rodgers and Hore, 2009; Hore and Mouritsen, 2016; Xu et al., 2021; Laurien et al., 2024; Frederiksen et al., 2024a). Behavioral experiments with European migratory songbirds suggest the presence of a mechanism that would allow the animals to use the geomagnetic field for navigation or orientation (Engels et al., 2012; Hore and Mouritsen, 2016). The experiments are conducted during the migratory season when the birds are most active and have an impulse to migrate (Wiltschko, 2012; Engels et al., 2012; Mouritsen, 2018). In the experiments, the birds are placed inside an Emlen funnel (Emlen and Emlen, 1966). The funnel has scratch paper inside; as the birds jump and peck, they mark the direction in which they intend to go; it has been observed that the distribution of the scratch marks on the paper changes depending on the controlled illumination of the environment. When the experiment was performed under less energetic colors of light, like red, the direction of the scratches was random, but with more energetic colors, like blue, the scratches were more localized in a specific direction (Wiltschko and Wiltschko, 1972). Since the birds had no other form of orientation or navigation available during the experiment, the conclusion was that they might be using some mechanism to detect the geomagnetic field. This mechanism should be light-dependent, accounting for the different behaviour during light conditions. Similar experiments have shown that birds have an inclination compass (Wiltschko, 2012; Wiltschko and Wiltschko, 1972). Inclination compasses work in a slightly different way than the ordinary compasses. Standard compasses are polarity compasses, they point to one of the poles of the magnetic field. Inclination compasses do not point to the poles; they give information on the inclination of the field lines of a given magnetic field. If the polarity of the field is flipped, but the inclination is the same, then the inclination compass will not change its direction (Mouritsen, 2015).
Following the experimental evidence that the birds use an inclination compass, the animal would not be able to detect magnetic fields solely by employing magnetic materials, since the related mechanisms would naturally imply a polarity compass (Solov’yov and Greiner, 2008). Another magnetoreception mechanism proposed by Schulten et al. (1978) is the so-called radical pair mechanism. Here, a radical pair is formed by a light-activated chemical reaction and works as an inclination compass, providing information about the inclination of the magnetic field and a molecular structure in which the radical pair is embedded. It is theorized to exist inside a protein called cryptochrome in the bird’s retina (Mora et al., 2004; Hanić et al., 2022; Dodson et al., 2013; Engels et al., 2012). Cryptochrome has a chromophore called flavin adenine dinucleotide (FAD), which is sensitive to blue light (Bouly et al., 2007; Hore and Mouritsen, 2016; Liedvogel et al., 2007; Cashmore et al., 1999). Cryptochrome also contains several conserved tryptophan (Trp) residues that bridge the FAD cofactor with the protein surface. Figure 6 shows the structure of a cryptochrome from an european robin (erithacus Rubecula) (Hanić et al., 2022; Timmer et al., 2023) with the embedded FAD and the key Trps. The proposed radical pair mechanism utilizes these molecules to detect the geomagnetic field as illustrated in Figure 7.
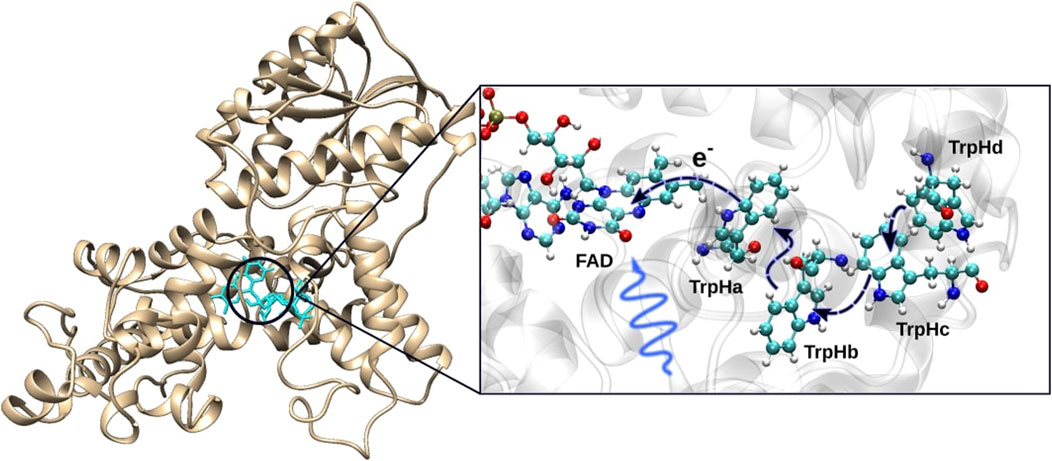
Figure 6. Structure of the cryptochrome protein with indication of the location of the flavin adenine dinucleotide (FAD) cofactor and the surrounding tryptophan residues. After photo-absorption of blue light by FAD, an electron is transferred from TrpHa. The initial transfer initiates a chain of electron transfers from TrpHb to TrpHa, then from TrpHc to TrpHb and finally from TrpHd to TrpHc. With each transfer, the state of the correlated electrons is moved to the next residue, where the FAD has a probability of being in the radical pair state with TrpHd or TrpHc (Wong et al., 2021).
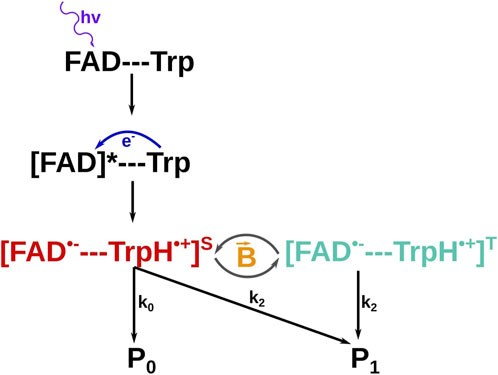
Figure 7. Proposed reaction path for a FAD-Trp radical pair. The FAD first absorbs a photon and gets into an excited state. The excitation allows an electron from the tryptophan (Trp) to be transferred to the nearby FAD; putting both molecules into the radical pair state. In this state the electrons are sensitive to weak external magnetic fields and flip between the singlet(S) and the triplet(T) spin states with a rate modulated by the external field. After some time the molecules decay into products
The reaction cascade starts with the FAD absorbing a photon of an appropriate energy. The photon excites an electron in the FAD to a higher energy state and allows the excited FAD to receive another electron from the Trp residue nearby (Timmer et al., 2023; Schuhmann et al., 2023; Solov’yov et al., 2024; Matysik et al., 2023; Xu et al., 2021). After the electron transfer, the FAD and Trp have unpaired electrons, i.e., form a radical pair. The transferred electron was originally paired with another electron with a similar energy, therefore, both electrons in the radical pair initially form a singlet spin state, where the spins of the electrons appear anti-parallel, and the spins of the electrons are correlated. Each unpaired electron of the radical pair is located at a different site; therefore, the electrons experience different magnetic environments, which include the interactions between the electron spins and the nuclear spins, called hyperfine interactions, and the interaction between the electron spins and the external magnetic field, called the Zeeman interaction (Grüning et al., 2022; Grüning et al., 2024; Kattnig et al., 2016a). The radical pair is sensitive to weak magnetic fields, such as the geomagnetic field of about
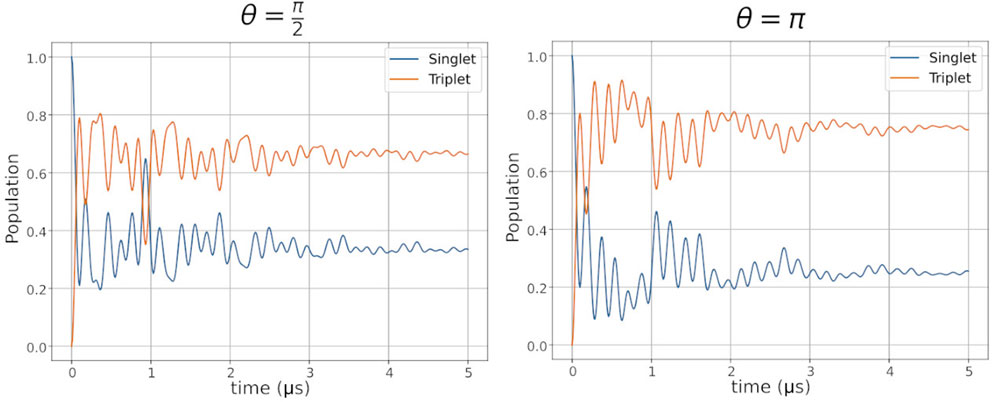
Figure 8. Dynamics of the singlet (blue) and triplet (orange) populations of a hypothetic radical pair (see Figure 7) during the radical pair reaction consisting of 2 electrons and one proton with anisotropic hyperfine interaction coupled to one of the electrons, and recombination rates of
A relevant discussion on the radical pair mechanism is whether it requires a quantum description. There are multiple descriptions for the dynamics of the radical pair mechanism (Fay et al., 2020; Jones and Hore, 2010; Wong et al., 2021), both purely quantum and semi-classical. The primary quantum aspect of radical pairs is the correlation of spin states. Spin is a quantized property only able to assume specific discrete values, and cannot be described by a purely classical approach. The quantized nature of spin defines the description of the radical pair as either semi-classical or purely quantum. While the radical pair mechanism is a promising hypothesis for the underlying phenomenon of magnetoreception, the concept of coherence challenges the theory. Due to the thermal motion of the molecular structure of proteins in which the radical pair is embedded, constant perturbations through adjacent magnetic momenta of the nuclear spins are leading to rapid decoherence of the radical pair emerging in spin relaxation (Gerhards et al., 2023; Grüning et al., 2024; Kattnig et al., 2016b; Worster et al., 2016). It was demonstrated in several studies that these decoherence processes drastically decrease the efficiency of the magnetic radical pair compass (Grüning et al., 2024; Kattnig et al., 2016a; Worster et al., 2016). Emerging new theories address the spin relaxation problem. For example, Smith et al. showed that complex time-dependencies of inter-radical pair magnetic interactions might drastically increase magnetic sensitivity (Smith et al., 2022). Non-Markovian approaches were also suggested to explore the impact of thermal motion in spin relaxation (Grüning et al., 2024; Li and Shen, 2024; Breuer et al., 2009; Vacchini and Breuer, 2010; Xin et al., 2022; Breuer et al., 2016; Shen et al., 2018). Another theory is the involvement of a third scavenger radical participating in the radical pair mechanism proposed by Kattnig and co-workers (Babcock and Kattnig, 2021; Deviers et al., 2024). This new hypothesis still requires the consideration of the quantum mechanical nature of spin, which sets the topic of magnetoreception as one of the major research areas of quantum biology.
5 Quantum effects in neural processes
The hypothesis that quantum mechanics may play a role in brain function, especially in the context of consciousness, has recently sparked considerable interest and debate (Jedlicka, 2017; Hameroff, 2022; Hagan et al., 2002). Quantum mechanics, which governs the behaviour of physical systems at the smallest scales, may offer insights into the complex phenomena observed in the brain. This section briefly overviews the current research on quantum effects in the brain, focusing on some of the key findings and theories and presents the major challenges and controversies involved.
One of the most prominent theories on quantum effects in the brain is the “quantum consciousness” hypothesis proposed by Hameroff and Penrose (Hameroff and Penrose, 2014). Although the unfortunate naming might attract unexpected ideas, the quantum consciousness theory posits that quantum processes such as entanglement, coherence, and superposition might have a role in brain function. Consciousness here does not relate to any metaphysical ideal, but to the brain’s ability to process and to react to external or internal stimuli and how anesthetics affect it, e.g., a rat following the smell of food, or a reaction to pain. While quantum consciousness is highly controversial, it is physically possible that quantum phenomena might be involved in critical points in neural processes. For instance, anesthetic gases can selectively block consciousness while sparing non-conscious brain activities, i.e., make someone unconscious (Li et al., 2018; Miller, 1961; Clar and Patel, 2023). Notably, it has been shown that, in mice, xenon isotopes with nuclear spin 1/2 are significantly less potent as anesthetics compared to isotopes with spin 0, suggesting a potential link between nuclear spin and consciousness (Fisher, 2015; Li et al., 2018; Cukras and Sadlej, 2021; Smith et al., 2021). The lesser effect of spin 1/2 isotopes supports the idea that consciousness might involve quantum processes, possibly through mechanisms like nuclear spin interactions and electron spin dipole oscillations in proteins present in neurons (Li et al., 2018).
Quantum effects might be particularly relevant in addressing the “binding problem” in cognitive neuroscience—the question of how the brain integrates disparate sensory information into a unified conscious experience (Feldman, 2012; Singer, 1999; Varela et al., 2001). In visual processing, for example, information about the shape, color, motion, and meaning of an object is processed in different areas of the visual cortex at different times (Bullier, 2001). These disparate elements are then correlated to bring forward a coherent perceptual experience. Quantum entanglement, where particles remain connected over distance and time, could provide a mechanism for this integration (Hameroff, 2018).
In another direction, microtubules, cytoskeletal components within neurons, have been speculated as potential sites for quantum computing in the brain. Microtubules are theorized to exhibit quantum resonance oscillations and might host quantum processes that regulate neuronal activity and behavior (Hameroff, 2022). Anesthetic gases are believed to dampen these oscillations, correlating with their ability to induce unconsciousness (Craddock et al., 2017).
While the many ideas of quantum effects playing possible roles in brain functioning may be interesting, there are essential problematics drastically damping the hypotheses. For quantum effects be involved in any neural process, the decoherence time for quantum states in a biological environment is critical. Decoherence times
where
where
In a recent article (Deviers et al., 2022), explore the surprising light-harvesting properties of microtubules. Their experimental investigation demonstrates that microtubules can facilitate long-range electronic energy migration. Using tryptophan autofluorescence lifetimes, the study shows that energy can diffuse over distances of 6.6 nm within microtubules, a length significantly longer than predicted by conventional Förster theory, Forster (1946). The study also highlights that this energy migration is sensitive to the polymerization state of tubulin and can be dampened by anesthetics like etomidate and isoflurane. These findings suggest that microtubules may have unique biophysical properties that could be relevant in both biological processes and the development of biohybrid devices, opening new avenues for exploring the non-classical roles of microtubules beyond their well-known functions in cellular architecture and transport, and possibly influencing future research in quantum biology and neurobiology.
To advance our understanding of potential quantum effects in the brain, interdisciplinary research combining neuroscience, quantum physics, and computational biology is essential. Future studies should focus on identifying specific quantum processes in neurons and developing experimental techniques to observe these processes in vivo. Additionally, exploring how quantum mechanics might contribute to other cognitive functions could provide valuable insights into the nature of consciousness (Schlosshauer, 2007; Varela et al., 2001; Hameroff, 1998).
Following this line, a recent study demonstrates the possibility of generating entangled biphotons in the myelin sheath using cavity quantum electrodynamics (cQED) (Liu et al., 2024). This study demonstrates that the vibrational modes of C-H bonds within lipid molecules’ tails can generate a significant number of entangled photon pairs.
The abundance of C-H bond vibration units in neurons can, therefore, serve as a source of quantum entanglement resources for the nervous system, thereby elucidating a potential source for the synchronized activity of neurons (Liu et al., 2024; Froehlich, 1968; Schroedinger, 1944). By demonstrating the feasibility of entangled biphoton generation in the brain’s myelin sheath, Liu et al. opens up new avenues for exploring how quantum entanglement could contribute to higher-order cognitive functions, such as decision-making, problem-solving, and consciousness (Fisher, 2015).
Despite the intriguing hypotheses mentioned before, the idea of quantum processes in the brain remains largely speculative and controversial. One major challenge is the issue of decoherence. Quantum states are susceptible to environmental disturbances, and the “warm, wet, and noisy” environment of the brain is expected to cause rapid decoherence, disrupting the possible quantum effects (Tegmark, 2000). However, certain conditions, such as the hydrophobic environments within proteins, might be more conducive to maintaining quantum coherence (Hagan et al., 2002). Moreover, some researchers argue that the brain’s complexity and functionality can be explained without invoking quantum mechanics. They point out that many proposed quantum brain processes remain speculative and lack direct experimental evidence (Eger et al., 2008; Koch, 2016; Reimers et al., 2009; McKemmish et al., 2009). Tegmark (2000) argued that the warm temperature of the brain would cause quantum coherence to break down too rapid to have any functional role. Despite this, proponents of quantum consciousness theories suggest that certain brain structures, such as microtubules, could protect against decoherence through as-yet-unknown mechanisms (Hameroff and Penrose, 2014; Fisher, 2015).
Additionally, the feasibility of quantum computing within neurons is questioned. Critics highlight the lack of empirical data supporting the quantum brain hypothesis and emphasize the success of classical computational models in explaining brain functions (Koch, 2016; Freeman, 2003). The authors also argue that many cognitive phenomena can be adequately explained without invoking quantum processes, pointing to advances in neuroscience and computational modeling that provide a detailed understanding of brain mechanisms using classical physics (Reimers et al., 2009; Tegmark, 2000). Nevertheless, recent experimental and theoretical developments continue to keep the debate alive, such as the peculiar effects of anesthetics on consciousness (Li et al., 2018; Hameroff, 2018), suggest that quantum effects could play a role in brain function, even if the exact mechanisms remain elusive.
6 Conclusion
Quantum biology is an emerging interdisciplinary field that elucidates how quantum effects can influence biological processes. This review discusses some examples that demonstrate the potential impact of quantum mechanics on biological systems, ranging from vision and photosynthesis to magnetoreception and neural processes. Starting the discussion with more well known processes and moving towards more elusive and speculative ones.
In vision, the quantum photo-absorption mechanism in retinal underscores the necessity of quantum descriptions for understanding light detection and subsequent chemical reactions. The discussed energy dynamics in the retinal after photo-absorption also illustrates how many effects, both classical and quantum, have to be taken into account when describing a biological process.
Section 3 illustrates the role of the quasi-particle exciton in a molecular mechanism, and how energy can be transported during a biological process. The efficiency of photosynthetic energy transfer through the Fenna-Matthews-Olson complex highlights the potential role of quantum random walks in biological systems. But there is still much to be studied on the precise description of the photosynthesic energy transfer mechanism, and what impact quantum random walk could have on it.
Magnetoreception in migratory songbirds, possibly mediated by the radical pair mechanism in the cryptochrome protein, illustrates how quantum effects can influence animal behavior and navigation. Avian magnetoreception is one of the more elusive examples in this review. Although there is extensive research on the subject (Hore and Mouritsen, 2016; Mouritsen, 2015; Fay et al., 2020; Wiltschko, 2012), it still needs rigorous experimental and theoretical investigation for the complete mechanism to be well understood.
The final section, discusses possible quantum effects present in neuronal activities. Specifically the activities connected with an animal’s active behaviour, or consciousness. As mentioned in the section, “consciousness” here refers to the term when used in anesthetic research. The ability for the animal to process and react to sensory data, or an awareness of internal and external stimuli. This review presents experimental results that show how isotopes with different spin can cause different effects on neuronal activities in mice (Li et al., 2018), and discussed the impact of low decoherence times on the presence of quantum effects in neurons.
Overall, this review emphasizes the importance of continued interdisciplinary research in quantum biology. As experimental techniques advance and theoretical models become more refined, the understanding of quantum effects in biological systems will likely deepen, offering novel insights into the fundamental mechanisms of life.
Author contributions
PA: Conceptualization, Investigation, Writing–original draft, Writing–review and editing. LG: Writing–review and editing. IS: Supervision, Writing–review and editing. MO: Conceptualization, Supervision, Writing–original draft, Writing–review and editing.
Funding
The author(s) declare that financial support was received for the research, authorship, and/or publication of this article. The authors would like to declare funding from the Volkswagen Foundation (Lichtenberg professorship awarded to I.A.S.), the Deutsche Forschungsgemeinschaft (SFB 1372 Magnetoreception and Navigation in Vertebrates, no. 395940726 to I.A.S.; TRR386/1-2023 HYP∗MOL, no 514664767 to I.A.S.), and the Ministry for Science and Culture of Lower Saxony Simulations Meet Experiments on the Nanoscale: Opening up the Quantum World to Artificial Intelligence (SMART) and Dynamik auf der Nanoskala: Von koharenten Elementarprozessen zur Funktionalitaet (DyNano). PA is partially supported the Pioneer Science Initiative (Iniciativa Ciência Pioneira) and the D’Or Institute of Research & Education. MO is partially supported by CNPq.
Conflict of interest
The authors declare that the research was conducted in the absence of any commercial or financial relationships that could be construed as a potential conflict of interest.
Publisher’s note
All claims expressed in this article are solely those of the authors and do not necessarily represent those of their affiliated organizations, or those of the publisher, the editors and the reviewers. Any product that may be evaluated in this article, or claim that may be made by its manufacturer, is not guaranteed or endorsed by the publisher.
References
Babcock, N. S., and Kattnig, D. R. (2021). Radical scavenging could answer the challenge posed by electron–electron dipolar interactions in the cryptochrome compass model. JACS Au 1 (11), 2033–2046. doi:10.1021/jacsau.1c00332
Babcock, N. S., Montes-Cabrera, G., Oberhofer, K. E., Chergui, M., Celardo, G. L., and Kurian, P. (2024). Ultraviolet superradiance from mega-networks of tryptophan in biological architectures. J. Phys. Chem. B 128 (17), 4035–4046. doi:10.1021/acs.jpcb.3c07936
Blankenship, R. E., Tiede, D. M., Barber, J., Brudvig, G. W., Fleming, G., Ghirardi, M., et al. (2011). Comparing photosynthetic and photovoltaic efficiencies and recognizing the potential for improvement. Science 332 (6031), 805–809. doi:10.1126/science.1200165
Bouly, J.-P., Schleicher, E., Dionisio-Sese, M., Vandenbussche, F., Van Der Straeten, D., Bakrim, N., et al. (2007). Cryptochrome blue light photoreceptors are activated through interconversion of flavin redox states. J. Biol. Chem. 282 (13), 9383–9391. doi:10.1074/jbc.m609842200
Boyer, M. M., Poulsen, G. L., and Nork, T. M. (2000). Relative contributions of the neurosensory retina and retinal pigment epithelium to macular hypofluorescence. Archives Ophthalmol. 118 (1), 27–31. doi:10.1001/archopht.118.1.27
Breuer, H.-P., Laine, E.-M., and Piilo, J. (2009). Measure for the degree of non-markovian behavior of quantum processes in open systems. Phys. Rev. Lett. 103, 210401. doi:10.1103/physrevlett.103.210401
Breuer, H.-P., Laine, E.-M., Piilo, J., and Vacchini, B. (2016). Colloquium: non-markovian dynamics in open quantum systems. Rev. Mod. Phys. 88, 021002. doi:10.1103/revmodphys.88.021002
Breuer, H.-P., and Petruccione, F. (2007). The theory of open quantum systems. Oxford: Oxford University Press.
Bullier, J. (2001). Integrated model of visual processing. Brain Res. Rev. 36 (2), 96–107. The Brain in Health and Disease - from Molecules to Man. Swiss National Foundation Symposium NRP 38. doi:10.1016/s0165-0173(01)00085-6
Cadiou, H., and McNaughton, P. A. (2010). Avian magnetite-based magnetoreception: a physiologist’s perspective. J. R. Soc. Interface 7, S193–S205. doi:10.1098/rsif.2009.0423.focus
Cao, J., Cogdell, R. J., Coker, D. F., Duan, H.-G., Hauer, J., Kleinekathöfer, U., et al. (2020). Quantum biology revisited. Sci. Adv. 6 (14), eaaz4888. doi:10.1126/sciadv.aaz4888
Carrillo, A., Cornelio, M. F., and de Oliveira, M. C. (2015). Environment-induced anisotropy and sensitivity of the radical pair mechanism in the avian compass. Phys. Rev. E 92, 012720. doi:10.1103/physreve.92.012720
Caruso, F., Chin, A. W., Datta, A., Huelga, S. F., and Plenio, M. B. (2009). Highly efficient energy excitation transfer in light-harvesting complexes: the fundamental role of noise-assisted transport. J. Chem. Phys. 131 (10), 105106. doi:10.1063/1.3223548
Cashmore, A. R., Jarillo, J. A., Wu, Y.-J., and Liu, D. (1999). Cryptochromes: blue light receptors for plants and animals. Science 284 (5415), 760–765. doi:10.1126/science.284.5415.760
Cintolesi, F., Ritz, T., Kay, C., Timmel, C., and Hore, P. (2003). Anisotropic recombination of an immobilized photoinduced radical pair in a 50-μt magnetic field: a model avian photomagnetoreceptor. Chem. Phys. 294 (3), 385–399. doi:10.1016/s0301-0104(03)00320-3
Clar, H., and Patel, R. (2023). Anesthetic Gases — ncbi.nlm.nih.gov. Available at: https://www.ncbi.nlm.nih.gov/books/NBK537013/ (Accessed August 18, 2024).
Craddock, T. J., Kurian, P., Preto, J., Sahu, K., Hameroff, S. R., Klobukowski, M., et al. (2017). Anesthetic alterations of collective terahertz oscillations in tubulin correlate with clinical potency: implications for anesthetic action and post-operative cognitive dysfunction. Sci. Rep. 7, 9877. doi:10.1038/s41598-017-09992-7
Cukras, J., and Sadlej, J. (2021). Towards quantum-chemical modeling of the activity of anesthetic compounds. Int. J. Mol. Sci. 22 (17), 9272. doi:10.3390/ijms22179272
Deviers, J., Cailliez, F., de la Lande, A., and Kattnig, D. R. (2024). Avian cryptochrome 4 binds superoxide. Comput. Struct. Biotechnol. J. 26, 11–21. doi:10.1016/j.csbj.2023.12.009
Deviers, J., Cailliez, F., Gutiérrez, B. Z., Kattnig, D. R., and de la Lande, A. (2022). Ab initio derivation of flavin hyperfine interactions for the protein magnetosensor cryptochrome. Phys. Chem. Chem. Phys. 24 (27), 16784–16798. doi:10.1039/d1cp05804e
Di Prima, D., Reinholdt, P., and Kongsted, J. (2024). Color tuning in bovine rhodopsin through polarizable embedding. J. Phys. Chem. B 128 (12), 2864–2873. PMID: 38489248. doi:10.1021/acs.jpcb.3c07891
Dodson, C. A., Hore, P., and Wallace, M. I. (2013). A radical sense of direction: signalling and mechanism in cryptochrome magnetoreception. Trends Biochem. Sci. 38 (9), 435–446. doi:10.1016/j.tibs.2013.07.002
Dudhe, N., Sahoo, P. K., and Benjamin, C. (2022). Testing quantum speedups in exciton transport through a photosynthetic complex using quantum stochastic walks. Phys. Chem. Chem. Phys. 24, 2601–2613. doi:10.1039/d1cp02727a
Eger, E. I., Raines, D. E., Shafer, S. L., Hemmings, H. C., and Sonner, J. M. (2008). Is a new paradigm needed to explain how inhaled anesthetics produce immobility? Anesth. and Analgesia 107 (3), 832–848. doi:10.1213/ane.0b013e318182aedb
Emlen, S. T., and Emlen, J. T. (1966). A technique for recording migratory orientation of captive birds. Auk 83 (3), 361–367. doi:10.2307/4083048
Engel, G. S., Calhoun, T. R., Read, E. L., Ahn, T.-K., Mančal, T., Cheng, Y.-C., et al. (2007). Evidence for wavelike energy transfer through quantum coherence in photosynthetic systems. Nature 446 (7137), 782–786. doi:10.1038/nature05678
Engels, S., Hein, C. M., Lefeldt, N., Prior, H., and Mouritsen, H. (2012). Night-migratory songbirds possess a magnetic compass in both eyes. PLoS ONE 7 (9), e43271. doi:10.1371/journal.pone.0043271
Fassioli, F., and Olaya-Castro, A. (2010). Distribution of entanglement in light-harvesting complexes and their quantum efficiency. New J. Phys. 12 (8), 085006. doi:10.1088/1367-2630/12/8/085006
Fay, T. P., Lindoy, L. P., Manolopoulos, D. E., and Hore, P. J. (2020). How quantum is radical pair magnetoreception? Faraday Discuss. 221, 77–91. doi:10.1039/c9fd00049f
Feldman, J. (2012). The neural binding problem(s). Cogn. Neurodynamics 7 (1), 1–11. doi:10.1007/s11571-012-9219-8
Fenna, R. E., and Matthews, B. W. (1975). Chlorophyll arrangement in a bacteriochlorophyll protein from chlorobium limicola. Nature 258 (5536), 573–577. doi:10.1038/258573a0
Fisher, M. P. A. (2015). Quantum cognition: the possibility of processing with nuclear spins in the brain. Ann. Phys. 362, 593–602. doi:10.1016/j.aop.2015.08.020
Forster, T. (1946). Energiewanderung und fluoreszenz. Naturwissenschaften 33, 166–175. doi:10.1007/bf00585226
Frederiksen, A., Aldag, M., Solov’yov, I. A., and Gerhards, L. (2024a). Activation of cryptochrome 4 from atlantic herring. Biology 13 (4), 262. doi:10.3390/biology13040262
Frederiksen, A., Gerhards, L., Reinholdt, P., Kongsted, J., and Solov’yov, I. A. (2024b). Importance of polarizable embedding for absorption spectrum calculations of arabidopsis thaliana cryptochrome 1. J. Phys. Chem. B 0 (0), 6283–6290. PMID: 38913544. doi:10.1021/acs.jpcb.4c02168
Freeman, W. J. (2003). Evidence from human scalp electroencephalograms of global chaotic itinerancy. Chaos 13 (3), 1067–1077. doi:10.1063/1.1596553
Froehlich, H. (1968). Long-range coherence and energy storage in biological systems. Int. J. Quantum Chem. 2, 641–649. doi:10.1002/qua.560020505
Gerhards, L., Nielsen, C., Kattnig, D. R., Hore, P., and Solov’yov, I. A. (2023). Modeling spin relaxation in complex radical systems using MolSpin. J. Comput. Chem. 44, 1704–1714. doi:10.1002/jcc.27120
Ghasemi, F., and Shafiee, A. (2020). An investigation into the energy transfer efficiency of a two-pigment photosynthetic system using a macroscopic quantum model. Biosystems 197, 104209. doi:10.1016/j.biosystems.2020.104209
Grüning, G., Gerhards, L., Wong, S. Y., Kattnig, D., and Solov’yov, I. (2024). The effect of spin relaxation on magnetic compass sensitivity in ercry4a. Chemphyschem a Eur. J. Chem. Phys. Phys. Chem. 25, e202400129. e202400129–e202400129. doi:10.1002/cphc.202400129
Grüning, G., Wong, S. Y., Gerhards, L., Schuhmann, F., Kattnig, D. R., Hore, P., et al. (2022). Effects of dynamical degrees of freedom on magnetic compass sensitivity: a comparison of plant and avian cryptochromes. J. Am. Chem. Soc. 144 (50), 22902–22914. doi:10.1021/jacs.2c06233
Haas, T., Krause, R., Weber, R., Demler, M., and Schmid, G. (2018). Technical photosynthesis involving co2 electrolysis and fermentation. Nat. Catal. 1 (1), 32–39. doi:10.1038/s41929-017-0005-1
Hagan, S., Hameroff, S. R., and Tuszynski, J. A. (2002). Quantum computation in brain microtubules? decoherence and biological feasibility. Phys. Rev. E 65 (6), 061901. doi:10.1103/physreve.65.061901
Haken, H., and Wolf, H. C. (1996). General laws of optical transitions. Berlin, Heidelberg: Springer Berlin Heidelberg, 281–296. chapter 16.
Hameroff, S. (2022). Consciousness, cognition and the neuronal cytoskeleton – a new paradigm needed in neuroscience. Front. Mol. Neurosci. 15, 869935. doi:10.3389/fnmol.2022.869935
Hameroff, S. R. (1998). Quantum computation in brain microtubules? the penrose-hameroff ’orch or’ model of consciousness. Philosophical Trans. R. Soc. Lond. Ser. A Math. Phys. Eng. Sci. 356 (1743), 1869–1896. doi:10.1098/rsta.1998.0254
Hameroff, S. R. (2018). Anesthetic action and ’quantum consciousness’: a match made in olive oil. Anesthesiology 129 (2), 228–231. doi:10.1097/aln.0000000000002273
Hameroff, S. R., and Penrose, R. (2014). Consciousness in the universe: a review of the ’orch or’ theory. Phys. Life Rev. 11 (1), 39–78. doi:10.1016/j.plrev.2013.08.002
Hanić, M., Schuhmann, F., Frederiksen, A., Langebrake, C., Manthey, G., Liedvogel, M., et al. (2022). Computational reconstruction and analysis of structural models of avian cryptochrome 4. J. Phys. Chem. B 126 (25), 4623–4635. PMID: 35704801. doi:10.1021/acs.jpcb.2c00878
Hore, P. J., and Mouritsen, H. (2016). The radical-pair mechanism of magnetoreception. Annu. Rev. Biophysics 45 (1), 299–344. PMID: 27216936. doi:10.1146/annurev-biophys-032116-094545
Hoyer, S., Sarovar, M., and Birgitta Whaley, K. (2010). Limits of quantum speedup in photosynthetic light harvesting. New J. Phys. 12 (6), 065041. doi:10.1088/1367-2630/12/6/065041
Imahashi, M., Watanabe, M., Jha, S., and Hayashi, K. (2014). Olfaction-inspired sensing using a sensor system with molecular recognition and optimal classification ability for comprehensive detection of gases. Sensors 14 (3), 5221–5238. doi:10.3390/s140305221
Ishizaki, A., and Fleming, G. R. (2010). Quantum superpositions in photosynthetic light harvesting: delocalization and entanglement. New J. Phys. 12 (5), 055004. doi:10.1088/1367-2630/12/5/055004
Jedlicka, P. (2017). Revisiting the quantum brain hypothesis: toward quantum (neuro)biology? Front. Mol. Neurosci. 10, 366. doi:10.3389/fnmol.2017.00366
Joly, J. (1921). A quantum theory of colour vision. Proc. R. Soc. Lond. Ser. B, Contain. Pap. a Biol. Character 92 (646), 219–232. doi:10.1098/rspb.1921.0020
Jones, J., and Hore, P. (2010). Spin-selective reactions of radical pairs act as quantum measurements. Chem. Phys. Lett. 488 (1), 90–93. doi:10.1016/j.cplett.2010.01.063
Kalra, A. P., Benny, A., Travis, S. M., Zizzi, E. A., Morales-Sanchez, A., Oblinsky, D. G., et al. (2023a). Electronic energy migration in microtubules. ACS Central Sci. 9 (3), 352–361. doi:10.1021/acscentsci.2c01114
Karafyllidis, I. G. (2017). Quantum transport in the fmo photosynthetic light-harvesting complex. J. Biol. Phys. 43, 239–245. doi:10.1007/s10867-017-9449-4
Kattnig, D. R., Solov’yov, I. A., and Hore, P. (2016a). Electron spin relaxation in cryptochrome-based magnetoreception. Phys. Chem. Chem. Phys. 18 (18), 12443–12456. doi:10.1039/c5cp06731f
Kattnig, D. R., Sowa, J. K., Solov’yov, I. A., and Hore, P. (2016b). Electron spin relaxation can enhance the performance of a cryptochrome-based magnetic compass sensor. New J. Phys. 18 (6), 063007. doi:10.1088/1367-2630/18/6/063007
Khorana, H. G. (1992). Rhodopsin, photoreceptor of the rod cell. an emerging pattern for structure and function. J. Biol. Chem. 267 (1), 1–4. doi:10.1016/s0021-9258(18)48444-x
Koch, C. (2016). How to build a conscious machine. Sci. Am. 317 (5), 28. doi:10.1038/scientificamerican1117-28
Kretschmer, K., Frederiksen, A., Reinholdt, P., Kongsted, J., and Solov’yov, I. A. (2024). Understanding the red shift in the absorption spectrum of the fad cofactor in clcry4 protein. J. Phys. Chem. B 128 (22), 5320–5326. PMID: 38805723. doi:10.1021/acs.jpcb.4c00710
Landau, L. D., and Lifshitz, E. M. (1981). Quantum mechanics. 3 edition. Oxford: Butterworth-Heinemann.
Laurien, M., Mende, L., Luhrmann, L., Frederiksen, A., Aldag, M., Spiecker, L., et al. (2024). Magnetic orientation in juvenile atlantic herring (clupea harengus) could involve cryptochrome 4 as a potential magnetoreceptor. J. R. Soc. Interface 21 (215), 20240035. doi:10.1098/rsif.2024.0035
Li, N., Lu, D., Yang, L., Tao, H., Xu, Y., Wang, C., et al. (2018). Nuclear spin attenuates the anesthetic potency of xenon isotopes in mice: implications for the mechanisms of anesthesia and consciousness. Anesthesiology 129 (2), 271–277. doi:10.1097/aln.0000000000002226
Li, Z. Y., and Shen, H. Z. (2024). Non-markovian dynamics with a giant atom coupled to a semi-infinite photonic waveguide. Phys. Rev. A 109, 023712. doi:10.1103/physreva.109.023712
Liedvogel, M., Maeda, K., Henbest, K., Schleicher, E., Simon, T., Timmel, C. R., et al. (2007). Chemical magnetoreception: bird cryptochrome 1a is excited by blue light and forms long-lived radical-pairs. PLoS ONE 2 (10), e1106. doi:10.1371/journal.pone.0001106
Liu, Z., Chen, Y.-C., and Ao, P. (2024). Entangled biphoton generation in the myelin sheath. Phys. Rev. E 110, 024402. doi:10.1103/physreve.110.024402
Loulakis, M., Blatsios, G., Vrettou, C., and Kominis, I. (2017). Quantum biometrics with retinal photon counting. Phys. Rev. Appl. 8 (4), 044012. doi:10.1103/physrevapplied.8.044012
Maeda, K., Henbest, K. B., Cintolesi, F., Kuprov, I., Rodgers, C. T., Liddell, P. A., et al. (2008). Chemical compass model of avian magnetoreception. Nature 453 (7193), 387–390. doi:10.1038/nature06834
Maeda, K., Robinson, A. J., Henbest, K. B., Hogben, H. J., Biskup, T., Ahmad, M., et al. (2012). Magnetically sensitive light-induced reactions in cryptochrome are consistent with its proposed role as a magnetoreceptor. Proc. Natl. Acad. Sci. 109 (13), 4774–4779. doi:10.1073/pnas.1118959109
Marais, A., Adams, B., Ringsmuth, A. K., Ferretti, M., Gruber, J. M., Hendrikx, R., et al. (2018). The future of quantum biology. J. R. Soc. Interface 15 (148), 20180640. doi:10.1098/rsif.2018.0640
Marini, A., Muñoz-Losa, A., Biancardi, A., and Mennucci, B. (2010). What is solvatochromism? J. Phys. Chem. B 114 (51), 17128–17135. PMID: 21128657. doi:10.1021/jp1097487
Matysik, J., Gerhards, L., Theiss, T., Timmermann, L., Kurle-Tucholski, P., Musabirova, G., et al. (2023). Spin dynamics of flavoproteins. Int. J. Mol. Sci. 24 (9), 8218. doi:10.3390/ijms24098218
McFadden, J., and Al-Khalili, J. (2018). The origins of quantum biology. Proc. R. Soc. A Math. Phys. Eng. Sci. 474 (2220), 20180674. doi:10.1098/rspa.2018.0674
McKemmish, L. K., Reimers, J. R., McKenzie, R. H., Mark, A. E., and Hush, N. S. (2009). Quantum chemistry: simulation of the geometry and vibrational spectra of biomolecules relevant to the orch or model. Phys. Rev. E 80 (1), 021912. doi:10.1103/physreve.80.021912
Mennucci, B., Cammi, R., and Tomasi, J. (1998). Excited states and solvatochromic shifts within a nonequilibrium solvation approach: a new formulation of the integral equation formalism method at the self-consistent field, configuration interaction, and multiconfiguration self-consistent field level. J. Chem. Phys. 109 (7), 2798–2807. doi:10.1063/1.476878
Miller, S. L. (1961). A theory of gaseous anesthetics*</sup>. Proc. Natl. Acad. Sci. 47 (9), 1515–1524. doi:10.1073/pnas.47.9.1515
Moazed, K. T. (2023). “Quantum retina,” in Quantum biology of the eye: understanding the essentials (Germany: Springer), 67–86.
Mohseni, M., Rebentrost, P., Lloyd, S., and Aspuru-Guzik, A. (2008). Environment-assisted quantum walks in photosynthetic energy transfer. J. Chem. Phys. 129 (17), 174106. doi:10.1063/1.3002335
Mohseni, O. E., and Plenio, H. (2014). Quantum effects in biology. Cambridge: Cambridge University Press.
Mora, C. V., Davison, M., Wild, J. M., and Walker, M. M. (2004). Magnetoreception and its trigeminal mediation in the homing pigeon. Nature 432 (7016), 508–511. doi:10.1038/nature03077
Mouritsen, H. (2015). “Chapter 8 - magnetoreception in birds and its use for long-distance migration,” in Sturkie’s avian physiology. sixth edition edition (San Diego: Academic Press), 113–133.
Mouritsen, H. (2018). Long-distance navigation and magnetoreception in migratory animals. Nature 558 (7708), 50–59. doi:10.1038/s41586-018-0176-1
Mouritsen, H., and Hore, P. (2012). The magnetic retina: light-dependent and trigeminal magnetoreception in migratory birds. Curr. Opin. Neurobiol. 22 (2), 343–352. Neuroethology. doi:10.1016/j.conb.2012.01.005
Mouritsen, H., Janssen-Bienhold, U., Liedvogel, M., Feenders, G., Stalleicken, J., Dirks, P., et al. (2004). Cryptochromes and neuronal-activity markers colocalize in the retina of migratory birds during magnetic orientation. Proc. Natl. Acad. Sci. 101 (39), 14294–14299. doi:10.1073/pnas.0405968101
National Center for Biotechnology Information (2024a). PubChem compound summary for CID 5280490, 11-cis-Retinal. Available at: https://pubchem.ncbi.nlm.nih.gov/compound/11-cis-Retinal (Accessed June 3, 2024).
National Center for Biotechnology Information (2024b). PubChem compound summary for CID 638015, retinal. Available at: https://pubchem.ncbi.nlm.nih.gov/compound/Retinal (Accessed May 26, 2024).
Olaya-Castro, A., Lee, C. F., Olsen, F. F., and Johnson, N. F. (2008). Efficiency of energy transfer in a light-harvesting system under quantum coherence. Phys. Rev. B 78, 085115. doi:10.1103/physrevb.78.085115
Osterby, B. R., and McKelvey, R. D. (1996). Convergent synthesis of betaine-30, a solvatochromic dye: an advanced undergraduate project and demonstration. J. Chem. Educ. 73 (3), 260. doi:10.1021/ed073p260
Pedram, A., Müstecaplıoğlu, Ö. E., and Kominis, I. K. (2022). Using quantum states of light to probe the retinal network. Phys. Rev. Res. 4 (3), 033060. doi:10.1103/physrevresearch.4.033060
Plenio, M. B., and Huelga, S. F. (2008). Dephasing-assisted transport: quantum networks and biomolecules. New J. Phys. 10 (11), 113019. doi:10.1088/1367-2630/10/11/113019
Reimers, J. R., McKemmish, L. K., McKenzie, R. H., Mark, A. E., and Hush, N. S. (2009). Penrose-Hameroff orchestrated objective-reduction proposal for human consciousness is not biologically feasible. Phys. Rev. E 80 (2), 021912. doi:10.1103/PhysRevE.80.021912
Ritz, T., Hu, X., Damjanović, A., and Schulten, K. (1998). Excitons and excitation transfer in the photosynthetic unit of purple bacteria. J. Luminescence 76-77, 310–321. doi:10.1016/s0022-2313(97)00286-x
Ritz, T., Park, S., and Schulten, K. (2001). Kinetics of excitation migration and trapping in the photosynthetic unit of purple bacteria. J. Phys. Chem. B 105 (34), 8259–8267. doi:10.1021/jp011032r
Rodgers, C. T., and Hore, P. J. (2009). Chemical magnetoreception in birds: the radical pair mechanism. Proc. Natl. Acad. Sci. 106 (2), 353–360. doi:10.1073/pnas.0711968106
Royant, A., Nollert, P., Edman, K., Neutze, R., Landau, E. M., Pebay-Peyroula, E., et al. (2001). X-ray structure of sensory rhodopsin II at 2.1-Å resolution. Proc. Natl. Acad. Sci. 98 (18), 10131–10136. doi:10.1073/pnas.181203898
Runeson, J. E., Lawrence, J. E., Mannouch, J. R., and Richardson, J. O. (2022). Explaining the efficiency of photosynthesis: quantum uncertainty or classical vibrations? J. Phys. Chem. Lett. 13 (15), 3392–3399. PMID: 35404611. doi:10.1021/acs.jpclett.2c00538
Sahu, S., Ghosh, S. K., Fujita, D., and Bandyopadhyay, A. (2014). Live visualizations of single isolated tubulin protein self-assembly via tunneling current: effect of electromagnetic pumping during spontaneous growth of microtubule. Sci. Rep. 4, 7303. doi:10.1038/srep07303
Sarovar, M., Ishizaki, A., Fleming, G. R., and Whaley, K. B. (2010). Quantum entanglement in photosynthetic light-harvesting complexes. Nat. Phys. 6 (6), 462–467. doi:10.1038/nphys1652
Schrödinger, E. (1944). What is life? The physical aspect of the living cell. Based on lectures delivered under the auspices of the Institute at trinity college, Dublin, in february 1943. Cambridge: Cambridge University Press.
Schrödinger, E., and Penrose, R. (2012). What is life? with Mind and Matter and autobiographical sketches. Canto classics. Cambridge: Cambridge University Press.
Schroedinger, E. (1944). What is life? The physical aspect of the living cell. Cambridge: Cambridge University Press.
Schuhmann, F., Ryvkin, L., McLaren, J. D., Gerhards, L., and Solov’yov, I. A. (2023). Across atoms to crossing continents: application of similarity measures to biological location data. Plos one 18 (5), e0284736. doi:10.1371/journal.pone.0284736
Schulten, K., and Hayashi, S. (2014). Quantum biology of retinal. Cambridge: Cambridge University Press, 237–263. chapter 11.
Schulten, K., Swenberg, C. E., and Weller, A. (1978). A biomagnetic sensory mechanism based on magnetic field modulated coherent electron spin motion. Z. für Phys. Chem. 111 (1), 1–5. doi:10.1524/zpch.1978.111.1.001
Sen, S., Kar, R. K., Borin, V. A., and Schapiro, I. (2022). Insight into the isomerization mechanism of retinal proteins from hybrid quantum mechanics/molecular mechanics simulations. Wiley Interdiscip. Rev. Comput. Mol. Sci. 12 (1), e1562. doi:10.1002/wcms.1562
Shen, H. Z., Xu, S., Li, H., Wu, S. L., and Yi, X. X. (2018). Linear response theory for periodically driven systems with non-markovian effects. Opt. Lett. 43 (12), 2852–2855. doi:10.1364/ol.43.002852
Singer, W. (1999). Neuronal synchrony: a versatile code for the definition of relations? Neuron 24 (1), 49–65. doi:10.1016/s0896-6273(00)80821-1
Smith, J., Zadeh Haghighi, H., Salahub, D., and Simon, C. (2021). Radical pairs may play a role in xenon-induced general anesthesia. Sci. Rep. 11 (1), 6287. doi:10.1038/s41598-021-85673-w
Smith, L. D., Chowdhury, F. T., Peasgood, I., Dawkins, N., and Kattnig, D. R. (2022). Driven radical motion enhances cryptochrome magnetoreception: toward live quantum sensing. J. Phys. Chem. Lett. 13 (45), 10500–10506. doi:10.1021/acs.jpclett.2c02840
Solov’yov, A. V., Verkhovtsev, A. V., Mason, N. J., Amos, R. A., Bald, I., Baldacchino, G., et al. (2024). Condensed matter systems exposed to radiation: multiscale theory, simulations, and experiment. Chem. Rev. 124, 8014–8129. doi:10.1021/acs.chemrev.3c00902
Solov’yov, I. A., Chandler, D. E., and Schulten, K. (2008). Exploring the possibilities for radical pair effects in cryptochrome. Plant Signal. and Behav. 3 (9), 676–677. doi:10.4161/psb.3.9.5809
Solov’yov, I. A., and Greiner, W. (2008). Iron-mineral-based magnetoreceptor in birds: polarity or inclination compass? Eur. Phys. J. D 51 (1), 161–172. doi:10.1140/epjd/e2008-00118-y
Solov’yov, I. A., and Greiner, W. (2009). Micromagnetic insight into a magnetoreceptor in birds: existence of magnetic field amplifiers in the beak. Phys. Rev. E 80, 041919. doi:10.1103/physreve.80.041919
Solov’yov, I. A., Ritz, T., Schulten, K., and Hore, P. J. (2014). A chemical compass for bird navigation. Cambridge: Cambridge University Press, 218–236. chapter 10.
Spudich, J. L., and Jung, K.-H. (2005). Microbial rhodopsins: phylogenetic and functional diversity. New Jersey: John Wiley and Sons, Ltd, 1–23. chapter 1.
Starr, C., Evers, C., and Starr, L. (2006). Biology: concepts and applications. Brooks/cole biology series. Pacific Grove: Thomson, Brooks/Cole.
Steinmann, C., Reinholdt, P., Nørby, M. S., Kongsted, J., and Olsen, J. M. H. (2019). Response properties of embedded molecules through the polarizable embedding model. Int. J. Quantum Chem. 119 (1), e25717. doi:10.1002/qua.25717
Tang, H., Shang, X.-W., Shi, Z.-Y., He, T.-S., Feng, Z., Wang, T.-Y., et al. (2024). Simulating photosynthetic energy transport on a photonic network. npj Quantum Inf. 10 (1), 29. doi:10.1038/s41534-024-00824-x
Tegmark, M. (2000). Importance of quantum decoherence in brain processes. Phys. Rev. E 61 (4), 4194–4206. doi:10.1103/physreve.61.4194
Timmer, D., Frederiksen, A., Lünemann, D. C., Thomas, A. R., Xu, J., Bartölke, R., et al. (2023). Tracking the electron transfer cascade in european robin cryptochrome 4 mutants. J. Am. Chem. Soc. 145 (21), 11566–11578. doi:10.1021/jacs.3c00442
Tronrud, D. E., Wen, J., Gay, L., and Blankenship, R. E. (2009). The structural basis for the difference in absorbance spectra for the fmo antenna protein from various green sulfur bacteria. Photosynth. Res. 100 (2), 79–87. doi:10.1007/s11120-009-9430-6
Truhlar, D. G. (2003). “Potential energy surfaces,” in Encyclopedia of physical science and technology Editor R. A. Meyers Third Edition (New York: Academic Press), 9–17.
Vacchini, B., and Breuer, H.-P. (2010). Exact master equations for the non-markovian decay of a qubit. Phys. Rev. A 81, 042103. doi:10.1103/physreva.81.042103
Valeur, B., and Berberan-Santos, M. N. (2011). A brief history of fluorescence and phosphorescence before the emergence of quantum theory. J. Chem. Educ. 88 (6), 731–738. doi:10.1021/ed100182h
Varela, F. J., Lachaux, J.-P., Rodriguez, E., and Martinerie, J. (2001). The brainweb: phase synchronization and large-scale integration. Nat. Rev. Neurosci. 2, 229–239. doi:10.1038/35067550
Wald, G. (1968). The molecular basis of visual excitation. Nature 219 (5156), 800–807. doi:10.1038/219800a0
Whaley, K. B., Sarovar, M., and Ishizaki, A. (2011). Quantum entanglement phenomena in photosynthetic light harvesting complexes. Procedia Chem. 3 (1), 152–164. 22nd Solvay Conference on Chemistry. doi:10.1016/j.proche.2011.08.021
Wiltschko, R. (2012). Magnetic orientation in animals, Vol. 33. Germany: Springer Science and Business Media.
Wiltschko, R., Schiffner, I., Fuhrmann, P., and Wiltschko, W. (2010). The role of the magnetite-based receptors in the beak in pigeon homing. Curr. Biol. 20 (17), 1534–1538. doi:10.1016/j.cub.2010.06.073
Wiltschko, R., and Wiltschko, W. (2012). The magnetite-based receptors in the beak of birds and their role in avian navigation. J. Comp. Physiology A 199 (2), 89–98. doi:10.1007/s00359-012-0769-3
Wiltschko, W., and Wiltschko, R. (1972). Magnetic compass of european robins. Science 176 (4030), 62–64. doi:10.1126/science.176.4030.62
Wong, S. Y., Wei, Y., Mouritsen, H., Solov’yov, I. A., and Hore, P. J. (2021). Cryptochrome magnetoreception: four tryptophans could be better than three. J. R. Soc. Interface 18 (184), 20210601. doi:10.1098/rsif.2021.0601
Worster, S., Kattnig, D. R., and Hore, P. (2016). Spin relaxation of radicals in cryptochrome and its role in avian magnetoreception. J. Chem. Phys. 145 (3), 035104. doi:10.1063/1.4958624
Xin, L., Xu, S., Yi, X. X., and Shen, H. Z. (2022). Tunable non-markovian dynamics with a three-level atom mediated by the classical laser in a semi-infinite photonic waveguide. Phys. Rev. A 105, 053706. doi:10.1103/physreva.105.053706
Xu, J., Jarocha, L. E., Zollitsch, T., Konowalczyk, M., Henbest, K. B., Richert, S., et al. (2021). Magnetic sensitivity of cryptochrome 4 from a migratory songbird. Nature 594 (7864), 535–540. doi:10.1038/s41586-021-03618-9
Zhu, X.-G., Long, S. P., and Ort, D. R. (2010). Improving photosynthetic efficiency for greater yield. Annu. Rev. plant Biol. 61 (1), 235–261. doi:10.1146/annurev-arplant-042809-112206
Keywords: quantum biology, spin dynamics, molecular chemistry, magnetoreception, quantum mechanics
Citation: Alvarez PH, Gerhards L, Solov’yov IA and de Oliveira MC (2024) Quantum phenomena in biological systems. Front. Quantum Sci. Technol. 3:1466906. doi: 10.3389/frqst.2024.1466906
Received: 18 July 2024; Accepted: 30 September 2024;
Published: 30 October 2024.
Edited by:
Margit Christine Egg, University of Innsbruck, AustriaReviewed by:
Taras Plakhotnik, The University of Queensland, AustraliaH. Z. Shen, Northeast Normal University, China
Copyright © 2024 Alvarez, Gerhards, Solov’yov and de Oliveira. This is an open-access article distributed under the terms of the Creative Commons Attribution License (CC BY). The use, distribution or reproduction in other forums is permitted, provided the original author(s) and the copyright owner(s) are credited and that the original publication in this journal is cited, in accordance with accepted academic practice. No use, distribution or reproduction is permitted which does not comply with these terms.
*Correspondence: Pedro H. Alvarez, cGVkcm8uYWx2YXJlekB1bmktb2xkZW5idXJnLmRl