- 1Brainlab–Cognitive Neuroscience Research Group. Department of Clinical Psychology and Psychobiology, University of Barcelona, Barcelona, Spain
- 2Institute of Neurosciences, University of Barcelona, Barcelona, Spain
- 3Institut de Recerca Sant Joan de Déu, Esplugues de Llobregat, Barcelona, Spain
- 4BCNatal–Barcelona Center for Maternal Fetal and Neonatal Medicine (Hospital Sant Joan de Déu and Hospital Clínic), University of Barcelona, Barcelona, Spain
Introduction: Infants born very early preterm are at high risk of language delays. However, less is known about the consequences of late prematurity. Hence, the aim of the present study is to characterize the neural encoding of speech sounds in late preterm neonates in comparison with those born at term.
Methods: The speech-evoked frequency-following response (FFR) was recorded to a consonant-vowel stimulus /da/ in 36 neonates in three different groups: 12 preterm neonates [mean gestational age (GA) 36.05 weeks], 12 “early term neonates” (mean GA 38.3 weeks), and “late term neonates” (mean GA 41.01 weeks).
Results: From the FFR recordings, a delayed neural response and a weaker stimulus F0 encoding in premature neonates compared to neonates born at term was observed. No differences in the response time onset nor in stimulus F0 encoding were observed between the two groups of neonates born at term. No differences between the three groups were observed in the neural encoding of the stimulus temporal fine structure.
Discussion: These results highlight alterations in the neural encoding of speech sounds related to prematurity, which were present for the stimulus F0 but not for its temporal fine structure.
Introduction
According to the World Health Organization (2023) preterm infants are those born at less than 37 weeks of gestation, with an incidence of around 1 in 10 babies of all births. Preterm birth is the leading cause of death in children under 5 years of age. However, the survival rates are increasing (Volpe, 2019) and with them the morbidities attributable to the immaturity of several organs, such as lungs, kidneys and brain (Swamy et al., 2008). Preterm infants are at increased risk for language, cognitive, sensory, and motor deficits (Hee Chung et al., 2020), and numerous studies have highlighted language and social interaction skills as the major areas affected compared to infants born at term (Barre et al., 2011; Nazzi et al., 2015; Carter and Msall, 2017).
Regarding language, impairments were observed in both expressive and receptive domains, as well as in word retrieval (Vohr, 2014; Palumbi et al., 2018). These language impairments have been associated with poor academic performance, and poor social, behavioral and emotional functioning across the life span (Cusson, 2003; Wolke et al., 2008; Howard et al., 2011; Dong et al., 2012; Stipdonk et al., 2018). Thus, a clear effort is necessary to identify neonatal biomarkers which make possible to understand the neurobiological basis of early language impairments associated with preterm births. Such biomarkers may promote early interventions, thereby reducing the negative neurodevelopmental consequences of prematurity.
Recent studies have used cranial ultrasonography (CUS) and magnetic resonance imaging (MRI) to characterize the anatomical underpinnings of language impairments in full-term and preterm neonates. Several MRI studies based on diffusion tensor imaging (DTI) observed that microstructural changes in cerebral white matter at the school-aged period were associated with language impairments in preterm and full term birth (Travis et al., 2016; Dodson et al., 2018). Greater increases in axonal diffusivity of the left posterior thalamic radiation from term-equivalent postmenstrual age to age 4 years was associated with poorer receptive and expressive language ability at age four (Young et al., 2017). Higher Mean diffusivity (MD) in the centrum semiovale and left superior temporal gyrus has also been linked to poorer language outcomes in preterm children (Aeby et al., 2013; Pogribna et al., 2014). Consistent with these early childhood findings, alterations in the uncinate fasciculus, splenium of the corpus callosum, and anterior commissure have been linked to language outcomes among preterm adolescents (Northam et al., 2012).
Since speech rate for clear speech is roughly 100 words per minute (wpm) and in conversational speech, around 200 wpm (Picheny et al., 1986), other techniques with higher temporal resolution have also been used to investigate the language abilities required to encode speech. One of such techniques is event-related brain potentials (ERPs)–retrieved from the electroencephalogram (EEG)–(Näätänen et al., 2007) which are defined as electrical brain responses time-locked to a specific sensory stimulus (Luck, 2005). Several auditory evoked potentials (AEPs) have been used to explore language impairments (Luck, 2005; Friederici, 2006; Barman et al., 2021). But, recently, an electrophysiological response termed frequency-following response (FFR) have gained interest.
The FFR represents a snapshot into the neural encoding of the temporal and spectral features of the eliciting speech stimulus, allowing to explore the accuracy of neural encoding along the entire auditory system (Coffey et al., 2019; Gorina-Careta et al., 2021). A precise neural tracking of these two frequency components is critical to ensure language acquisition and comprehension (He et al., 2007; Moon and Hong, 2014; Lau et al., 2017). F0 is determined by the rate of glottis pulse and its neural encoding provides syntactic, phonological, and semantic cues (Nakatani and Schaffer, 1978), crucial for speaker identification (Mary and Yegnanarayana, 2008) and for dividing the continuous speech into word-form units (François et al., 2017). On the other hand, the temporal fine structure of the speech input, particularly its formants, is relevant for phoneme discrimination (Diehl and Lindblom, 2004; Hornickel et al., 2009) and, indeed, for speech perception in compromised comprehension contexts (Hopkins et al., 2008; Moore, 2008).
The FFR has been recorded in normal and clinical populations at the adulthood (White-Schwoch et al., 2020; Bidelman and Momtaz, 2021), childhood (Thompson et al., 2019, 2021), and the neonatal periods (Gardi et al., 1979; Jeng et al., 2010, 2011, 2016a,b; Ribas-Prats et al., 2019; Richard et al., 2020; Lemos et al., 2021; Ribas-Prats et al., 2022, 2023; see reviews in Gorina-Careta et al., 2022). In premature populations, recent FFR studies disclosed altered neural encoding of several speech cues crucial to ensure proper language acquisition (Madrid et al., 2021; Novitskiy et al., 2023). Madrid et al. (2021) enrolled 28 preterm infants with ≤32 weeks of gestational age (GA) in a longitudinal study. The FFR was recorded at 33, 35, 48–52, and 62–66 weeks of GA, and a decreased onset with age was observed on several FFR components. In the spectral domain, spectral amplitude of the stimulus fundamental frequency (F0) and for the lower frequencies of the first formant (F1; i.e., <270 Hz) showed an increase with age. However, for higher frequencies of the first formant and higher harmonics (>270 Hz) such increase was not found. Novitskiy et al. (2023) recorded the FFR from 45 preterm infants with a GA ≤ 34 weeks and 45 term infants from 0 to 12 months of age during natural sleep. In this study, three stimuli that differed in pitch were employed although no main effect of stimulus was found. Nevertheless, a main effect of age and prematurity in different FFR parameters related to synchronization and power were reported.
As discussed above, existing FFR studies in premature populations conducted during the first years of life suggest that premature infants born before 34 weeks of GA have limited neural encoding abilities of complex sound features (Madrid et al., 2021; Novitskiy et al., 2023). However, no previous studies explored preterm babies born from 34 0/7 through 36 6/7 weeks of GA. These neonates are defined as late preterm neonates (Raju et al., 2006; Engle et al., 2007) and, although they have higher rates of mortality and morbidity than term neonates (Kramer et al., 2000; Shapiro-Mendoza et al., 2006; Tomashek et al., 2006), understanding of the mechanisms of disease experienced by late-preterm neonates is largely incomplete (Seubert et al., 1999; Escobar et al., 2006; Hunt, 2006; Kinney, 2006; Laptook and Jackson, 2006; Ward, 2006). Hence, our study was set to investigate by means of FFR recordings the neural encoding of two important stimulus characteristics: pitch, quantified as the spectral amplitude to the stimulus fundamental frequency (F0), and the stimulus temporal fine structure, which relates to vowel formant composition, measured as the spectral amplitude at the first formant (F1) of the stimulus. Also, the FFR’s neural lag was computed. To contribute to explore, differentially, of being born premature from age effects, two terms neonate groups were included: one aged from 37.57 weeks to 39.14 weeks and another aged from 40.14 weeks to 42.29 weeks.
Materials and methods
Participants
A total of 36 infants (9 females) were enrolled from the Sant Joan de Déu Barcelona Children’s Hospital (Catalonia, Spain) between April 2019 and January 2020. Their gestational age (GA) was from 35.14 to 42.29 weeks. Exclusion criteria were an arterial pH ≤ 7.15 at the time of birth, and an APGAR score < 7 after 5 min of birth, multiple gestations, chromosomal or major structural abnormalities, familiar history of hearing loss and other risk factors associated with hearing impairment (American Academy of Pediatrics, Joint Committee on Infant Hearing, 2019). All infants passed the newborn hearing screening using an automated ABR system (ALGO 3i, Natus Medical Incorporated, San Carlos, CA) as part of standard medical practice. The study was approved by the Bioethics Committee of Sant Joan de Déu Barcelona Children’s Hospital (Approval ID: PIC-53-17) and all parents gave their written informed consent in accordance with the Declaration of Helsinki.
Infants were divided into three groups according to their GA with the same ratio of females to males in each group (1,3). Terms neonates were divided according to Lavie et al. (2023): infants born preterm (PRE group, 36.05 ± 0.63 weeks), early term neonates (EARLY group, 38.30 ± 0.57 weeks) and late term neonates (LATE group, 41.01 ± 0.64 weeks). Significant birth weight differences were observed between the three groups (H(2) = 25.377, p < 0.0001), and all Post hoc pairwise comparisons yielded significant differences [PRE vs. EARLY: mean = 2633.75 g vs. 3021.67 g, p = 0.004, 95% CI (69.66, 706.17); PRE vs. LATE: mean = 2633.75 g vs. 3530.00 g, p < 0.0001, 95% CI (577.99, 1214.51); EARLY vs. LATE: mean = 3021.67 g vs. 3530.00 g, p < 0.001, 95% CI (190.08, 826.59)].
Stimuli
In the present study, a click stimulus and the consonant-vowel /da/ were presented in alternating polarities at 60 dB SPL to elicit the auditory brainstem response (ABR) and the FFR, respectively. According to Jeng et al. (2011) and see Lemos et al. (2021), both stimuli were delivered to the right ear through ER3C shielded earphones of 300 Ω (Etymotic Research Inc., Elk Grove Village, IL, United States) connected to Flexicoupler® (Natus Medical Incorporated, San Carlos, CA) adaptor.
Click stimulus
Auditory brainstem responses (ABRs) to click stimuli were obtained to verify neural integrity in the right ear in all participants (Intelligent Hearing System SmartEP system, IHS, Miami, FL). A square wave click stimulus with a duration of 100 μs was used to elicit an ABR for each neonate analyzed. The stimuli were presented at a rate of 19.30 Hz and a silent inter-stimulus interval of 51.71 ms, based on the procedure followed in previous newborn FFR studies (Ribas-Prats et al., 2019; Arenillas-Alcón et al., 2021, 2023; Ribas-Prats et al., 2022, 2023).
/da/ stimulus
To obtain the FFR, the consonant-vowel syllable /da/ was chosen, as this is the most commonly stimulus used in neonatal FFR research (Richard et al., 2020; Lemos et al., 2021; Gorina-Careta et al., 2022). The stimulus fundamental frequency (F0) was 113 Hz, chosen to avoid electric power-line frequency interference in Europe at 50 Hz. The stimulus duration was 170 ms, with 10 ms for the onset period, 47 ms for the consonant transition, and 113 ms for the steady vowel section. The presentation rate was 3.7 Hz and the silent inter-stimulus interval was 100.27 ms. In contrast to the stimulus F0, which remained at the same frequency along the entire stimulus duration, the first (F1) and the second (F2) formants of the stimulus varied during the consonant transition, from 553 to 688 Hz and 1,438 to 1,214 Hz, respectively. Both formants at the vowel section remained constant at 688 Hz and 1,214 Hz, respectively.
Procedure
Stimuli presentation and EEG recordings were conducted with SmartEP platform including the cABR and Advanced Hearing Research modules (Intelligent Hearing Systems, Miami, FL, USA). Disposable Ag/AgCl electrodes were used for each infant and placed according to a vertical montage: active electrode at Fpz location, ground electrode upon the forehead, and references upon mastoids. EEG recording sessions took place in the hospital room while babies slept in their cradle, following the same procedure employed in previous studies with newborns (see Ribas-Prats et al., 2019; Arenillas-Alcón et al., 2021, 2023; Ribas-Prats et al., 2022, 2023).
A sampling rate of 13,333 Hz was used to acquire the continuous EEG. Previous to cut the EEG signal into epochs, recordings were filtered online from 30 to 1,500 Hz. The neural responses to click stimuli were epoched into sweeps of 51.81 ms, including 10.93 ms for the baseline and 40.88 ms for post-stimulus recording period. The epoch for the neural response to /da/ stimulus was 270.27 ms, including 40.95 ms for the baseline and 229.32 ms for post-stimulus recording period. The detection in any sweep of a voltage deflection above ±30 μV resulted in the online rejection as an artifact.
A total of 4000 artifact-free responses to click and to /da/ were analyzed. For the click stimulus, neural responses were acquired in two blocks of 2000 sweeps, and for the /da/ stimulus condition, it was recorded in four blocks of 1,000 sweeps. Data epoching and rejection were performed online. Less than 10% of sweeps were rejected in click or /da/ stimuli blocks in the all sample, respectively. Impedances remained under 10 kΩ during the whole duration of the recording session.
Data processing
Based on previous studies, only the recordings corresponding to the right ear (ipsilateral to the auditory stimulation) were employed in the statistical analysis (Hornickel et al., 2009; Ribas-Prats et al., 2019; Arenillas-Alcón et al., 2021; Ribas-Prats et al., 2022, 2023). To verify the auditory pathway integrity, the Wave V peak was identified in the ABR recording to click stimulus (Rushaidin et al., 2009) by an automated peak detection algorithm developed in Matlab R2019b (Mathworks). The script output was the highest value of the recorded response within a specified time window according to the age of the sample. Wave V peak latency and amplitudes were retrieved for statistical analysis.
For FFR analysis, the software from Intelligent Hearing Systems (Miami, FL, EEUU) and an off-line bandpass filter from 80 to 1,500 Hz with an infinite slope was applied to the neural responses to /da/ stimulus. The FFR parameters were calculated based on recent FFR tutorials (Krizman and Kraus, 2019) and according to our FFR analysis pipeline (Ribas-Prats et al., 2019; Arenillas-Alcón et al., 2021; Ribas-Prats et al., 2022, 2023). In sum, four FFR parameters were retrieved, three from neural responses to averaged stimulus polarities [(Rarefaction + Condensation)/2] and one from subtracted polarities [(Rarefaction – Condensation)/2].
The first parameter, called Pre-stimulus RMS, was computed to estimate background EEG activity through the pre-stimulus region. The second parameter was the neural lag, defined as the time elapsed between the stimulus onset and the neural phase-locking. Prior to the cross-correlation computation, the /da/ stimulus was resampled and bandpass filtered with a sampling rate of 13,333 Hz and a filter from 80 to 1,500 Hz, according to the neural response parameters. All subsequent parameters were calculated from the onset of the FFR which was defined as the individual neural lag value.
The third and fourth measurements were related to the neural encoding of F0 and temporal fine structure (i.e., the harmonics and formants of the stimulus). These parameters were calculated from spectral information in the FFR after the neural response was zero padded and the Fast Fourier Transform (FFT) was applied (Skoe and Kraus, 2010).
To explore the stimulus F0 encoding, neural responses to each stimulus polarity were averaged before applying the FFT. On the other hand, to investigate the stimulus temporal fine structure encoding, neural responses to each stimulus polarity were subtracted and the FFT was applied to the subtracted waveforms. Moreover, since the FFR phase-locking bounds (i.e., 1,500 Hz; Krizman and Kraus, 2019) limit the possibility to explore neural encoding of the stimulus F2, the temporal fine structure analysis was calculated on the harmonics located at the frequency region of the F1. Since F1 increases along the consonant transition (from 553 to 688 Hz) while remaining constant during the vowel region (688 Hz), the neural encoding of the stimulus F1 was analyzed for the consonant transition (10–57 ms) by averaging the spectral amplitude of the fifth and the sixth harmonics (i.e., HH5-6), whereas for the vowel region (57–170 ms, HH6) it was equivalent to the spectral amplitude of the sixth harmonic only. Since the spectral content of each stimulus region differs, the neural encoding of the stimulus F0 were calculated separately for the consonant transition and for the vowel region.
The spectral amplitude of the neural response for each stimulus region (i.e., the consonant transition and the vowel region) and each frequency peak of interest [i.e., F0 = 113 Hz at both the consonant transition and the vowel region; F1 = average HH5 (565 Hz) and HH6 (678 Hz) at the consonant transition region, and F1 = HH6 (678 Hz) at the vowel region] were calculated. All parameters described were computed with routines provided by Intelligent Hearing Systems (Miami, Fl, EEUU) and scripts developed in our laboratory under Matlab R2019b (Mathworks).
Statistical analysis
The statistical analysis was performed with IBM SPSS Statistics 23.0 (IBM Corporation, Armonk, NY). Normality was assessed with the Shapiro–Wilk’s test. Parameters normally distributed were analyzed by means of one factor ANOVA with group (PRE; EARLY; LATE) as factor. Those parameters which did not follow a normal distribution were analyzed by Kruskal-Wallis’ H. Accordingly, pairwise comparisons were conducted by Bonferroni-corrected t tests or by Mann–Whitney’s U test. A result was considered significant when p < 0.05.
Results
Auditory brainstem response
Wave V peak was identified in all preterm, early at term and late at term neonates. Grand-average ABR waveforms are shown in Figure 1A and violin plots of wave V amplitude and latency values are reported in Figures 1B,C, respectively. Wave V amplitude was not significantly different between groups [H(2) = 3.938, p = 0.140; Table 1]. Regarding wave V latency, a significant main group effect was found [F(2/33) = 4.506, p = 0.019, η2 = 0.215; Table 1]. Post hoc pairwise comparisons yielded significant differences between PRE and LATE neonates [PRE vs. LATE: mean = 9.07 ms vs. 8.57 ms, p = 0.029, 95% CI (0.04, 0.95)].
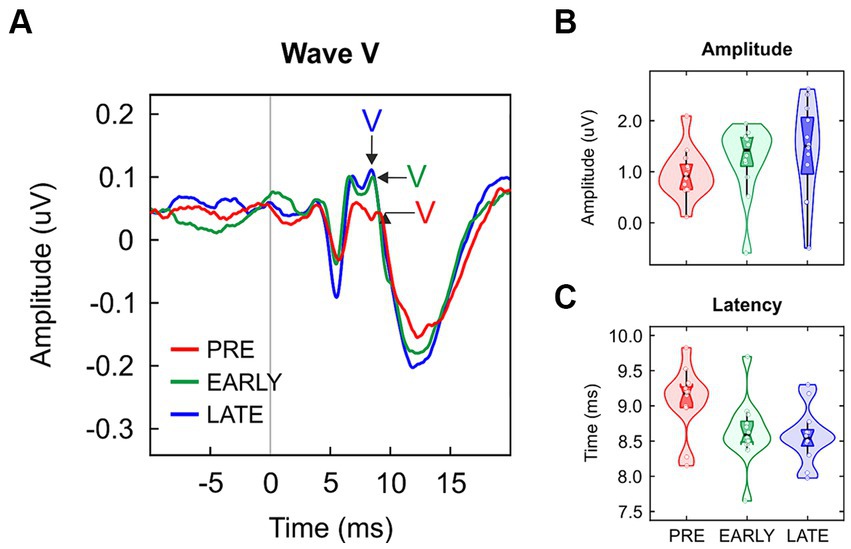
Figure 1. (A) Grand-averaged click ABRs for preterm neonates (PRE, red), early at term neonates (EARLY, green) and late at term neonates (LATE, blue), with the wave V peak pointed out. Data distribution (violin plots) for (B) wave V amplitude and (C) its latency. The black horizontal line illustrates the median and the black vertical line delimits the interquartile range (IQR).
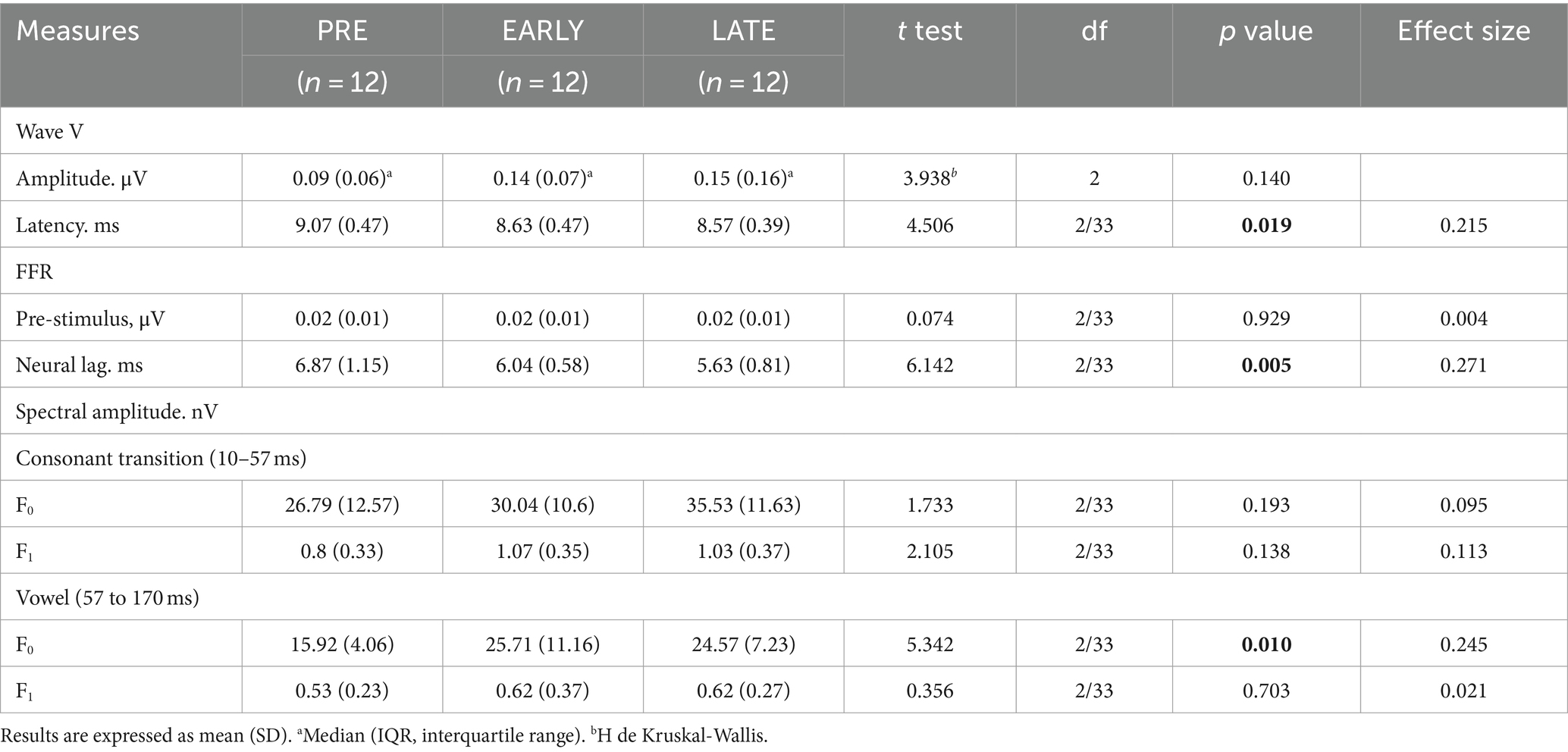
Table 1. Descriptive statistics and comparisons between preterm neonates (PRE), early at term neonates (EARLY) and late at term neonates (LATE) for each wave V and FFR parameter assessed.
Frequency-following response
Clear FFR waveforms were obtained in the three groups (Figure 2). Figure 2A shows the grand-average waveforms for each group for averaged polarities; Figure 2B shows the grand-average waveforms for each group for subtracted polarities. Based on pre-stimulus RMS parameter, similar background EEG activity was observed in the three groups of the study [F(2/33) = 0.074, p = 0.929, ηp2 = 0.004; Table 1; Figure 3A]. However, significant differences were found in the response onset as quantified by the neural lag [F(2/33) = 6.142, p = 0.005, ηp2 = 0.271; Table 1; Figure 3B]. Post hoc pairwise comparisons revealed significant delayed response in the PRE group compared to the LATE group [PRE vs. LATE: mean = 6.87 ms vs. 5.63 ms, p = 0.005, 95% CI (0.33, 2.15)].
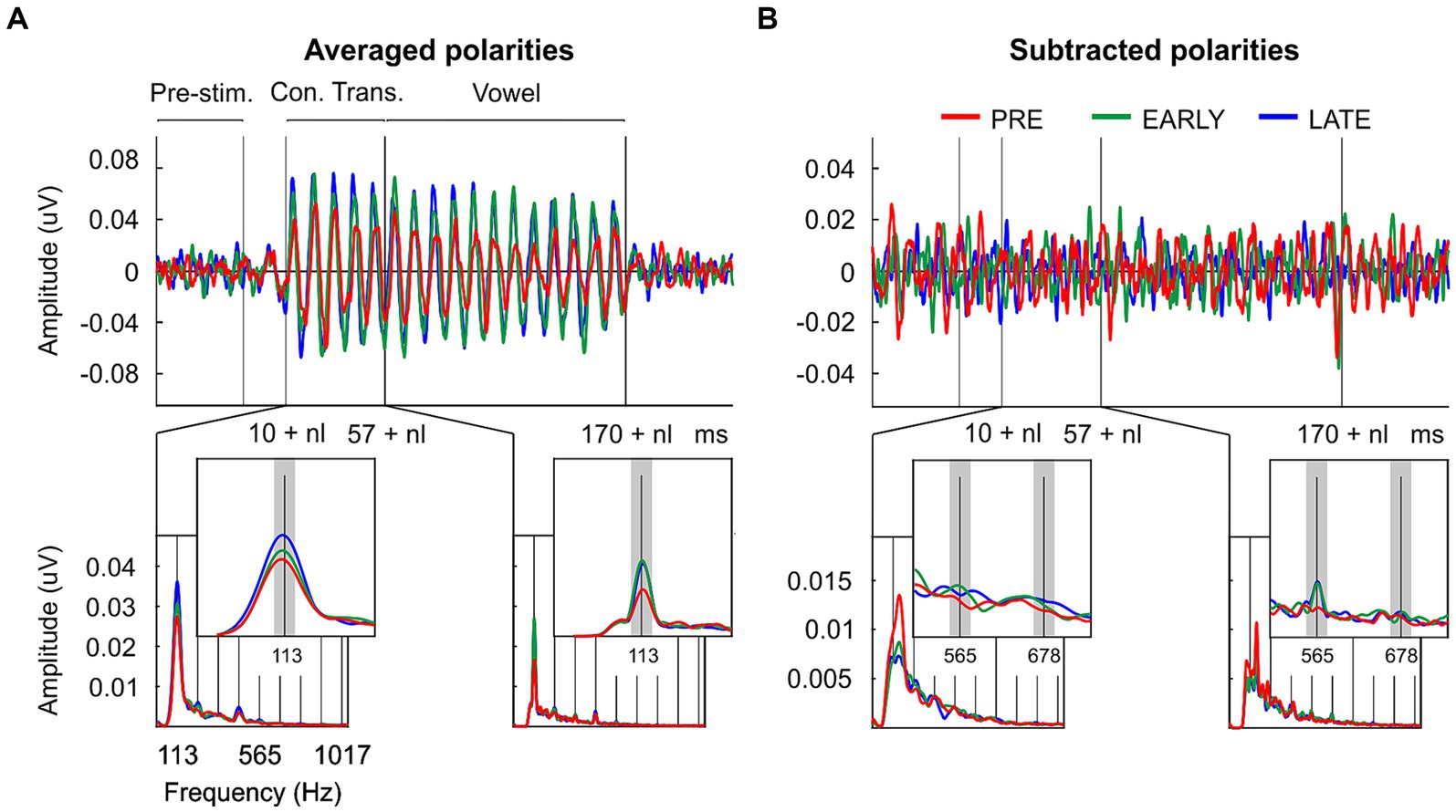
Figure 2. Temporal and spectral neural representation of the consonant-vowel /da/ in the neonate’s auditory brain. (A) Grand-averaged FFR waveforms and ampltude FFR spectra extracted from the consonant transition and from the vowel regions after averaged neural response polarities; and (B) after subtracted neural response polarities from preterm neonates (PRE, red), early at term neonates (EARLY, green) and late at term neonates (LATE, blue). The signal (s) and noise (n) spectral windows used for FFR quantification are marked with dark and light gray rectangles, respectively.
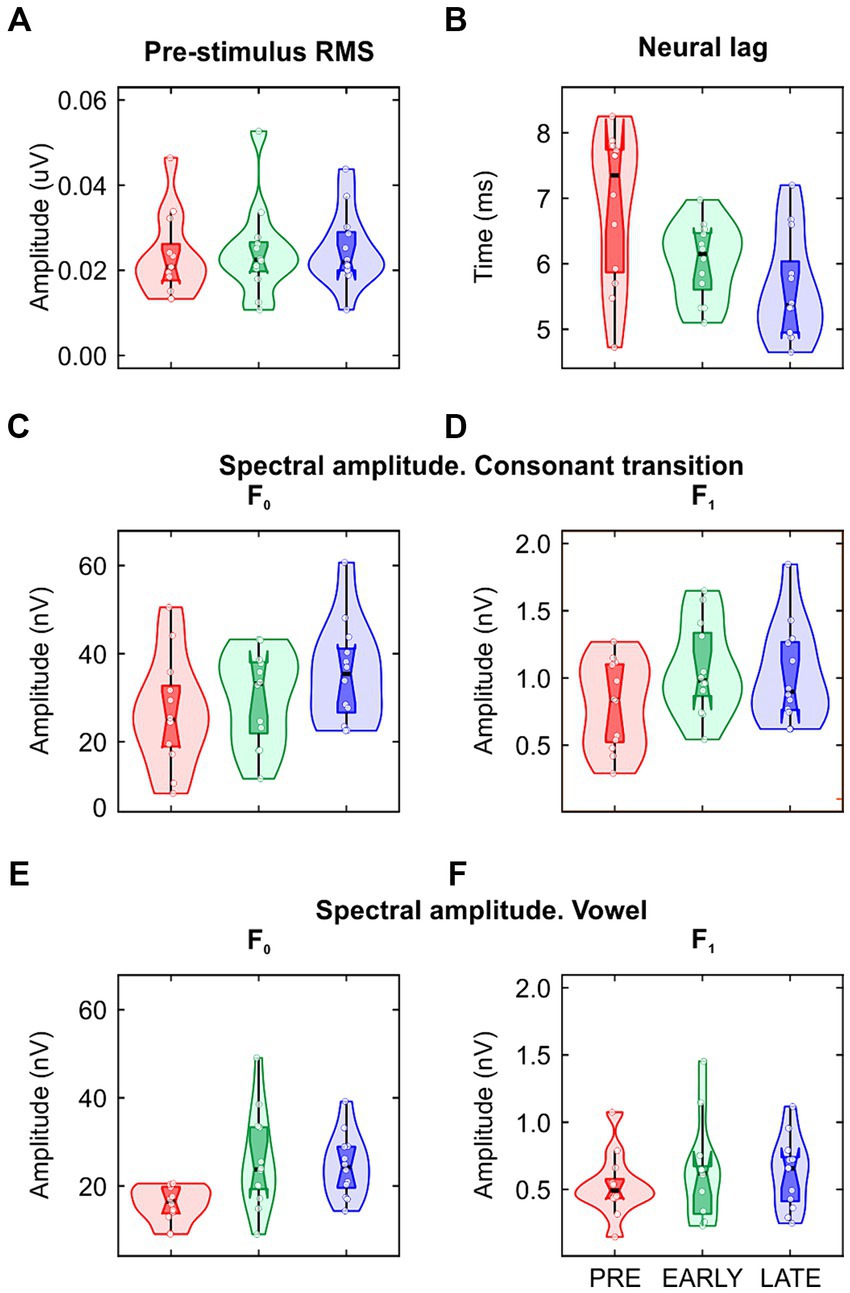
Figure 3. Data distribution (violin plots) of the (A) pre-stimulus RMS; (B) neural lag; (C,D) spectral amplitude at fundamental frequency (F0) and first formant (F1) extracted from the consonant transition and (E,F) from the vowel. The layout is the same to that used in Figures 1B,C.
As described in the data processing section, the FFR was analyzed in each group by calculating the spectral amplitude at the stimulus F0 for averaged stimulus polarities (Figure 2A; Figures 3C,E), and at the stimulus F1 for subtracted stimulus polarities (Figure 2B; Figures 3D,F; Aiken and Picton, 2008), for each stimulus region of interest: the stimulus consonant transition and the vowel region. Regarding the neural encoding of the stimulus F0, no significant differences were found in the spectral amplitudes extracted from the consonant transition [F(2/33) = 1.733, p = 0.193, ηp2 = 0.095; Table 1]. However, significant differences emerged at the vowel region [F(2/33) = 5.342, p = 0.010, ηp2 = 0.245; Table 1]. A main effect was observed between PRE and EARLY groups [PRE vs. EARLY: mean = 15.92 nV vs. 25.71 nV, p = 0.016, 95% CI (−18.05, −1.53)] and between PRE and LATE groups [PRE vs. LATE: mean = 15.92 nV vs. 24.57 nV, p = 0.038, 95% CI (−16.92, −0.39)]. The F1 neural encoding analysis yielded no significant differences for either the consonant transition [F(2/33) = 2.105, p = 0.138, ηp2 = 0.113; Table 1] or the vowel [F(2/33) = 0.356, p = 0.703, ηp2 = 0.021; Table 1].
Discussion
This study reveals functional impairments in the neural encoding of speech sound features in late preterm neonates, by analyzing electrophysiological brain responses elicited to the /da/ syllable. Our results align with previous studies in which an impaired neurodevelopment was associated to being born prematurely (Barre et al., 2011; Nazzi et al., 2015; Carter and Msall, 2017).
Since the earlier a neonate is born, the less likely the neonate is to survive and, in the case of survival to face long-term, health problems and disrupted neurodevelopment (ACOG Practice Bulletin, 2021), most of the clinical studies centered on improving the management of preterm populations are focused on the extreme preterm (<28 0/7 weeks), very preterm (28 0/7–31 6/7 weeks) or moderate (32 0/7–33 6/7 weeks) preterm population. The few clinical reports that compared late preterm infants (34 0/7–36 6/7 weeks) with term infants already suggested the presence of a higher risk for neurodevelopmental handicaps, such as speech disorders (Engle et al., 2007; Karnati et al., 2020). Stene-Larsen et al. (2014) found that late preterm infants were at increased risk for speech and language delays at 18 and 36 months, and Nepomnyaschy et al. (2012) described lower scores in language scales at 2 and 4 years in late preterm infants compared to term infants. In a longitudinal study, Rabie et al. (2015) examined the incidence differences of developmental speech or language disorders in late preterm infants and in term infants, and found an increased proportion of developmental speech and/or language delays in the clinical group.
Given that at 34 weeks of gestation (late-preterm) the fetal brain has reached only about 65% of its development (Kinney, 2006), white matter quintuples its weight from 35 to 41 weeks of gestation (Hüppi et al., 1998), and at this time several cerebral changes crucial for language development take place, such as an exponential neural connectivity growth between the cochlea and the auditory brainstem and its expansion to the auditory cortex (Pujol and Lavigne-Rebillard, 1992; Hepper and Shahidullah, 1994; Hall, 2000), more studies characterizing the abnormal functionality of neural underpinnings of language processing in these cohort of preterm neonates are needed.
Thus, in the present study we used the frequency-following response (FFR) to contribute to this aim. This electrophysiological response has a high temporal resolution allowing to explore one of the crucial processing abilities to ensure the first steps for appropriate language acquisition, namely, the capacity to catch the temporal and spectral characteristics of the speech input (Coffey et al., 2019; Gorina-Careta et al., 2021). Several studies conducted in different age cohorts (Gardi et al., 1979; Jeng et al., 2010, 2011, 2016a,b; Ribas-Prats et al., 2019; Thompson et al., 2019, 2021; White-Schwoch et al., 2020; Bidelman and Momtaz, 2021) and different language impairments (Russo et al., 2008; White-Schwoch et al., 2015, 2020; Otto-Meyer et al., 2018; Font-Alaminos et al., 2021) suggest the potential clinical use of the FFR.
Since language development can be affected by the presence of auditory impairment (Yoshinaga-Itano et al., 1998; American Academy of Pediatrics, Joint Committee on Infant Hearing, 2019), prior to the FFR recording to the /da/ syllable we tested the auditory pathway integrity by identifying the Wave V in ABR, elicited to a click stimulus (Hall, 2006; Sharma et al., 2016). Once the presence of the Wave V was confirmed in every individual participant, we analyzed group differences in the main Wave V parameters. Results revealed a significant latency delay in late preterm neonates compared to term neonates. These results aligned with those from previous studies in which, although different click rate and filter settings were used, a clear delayed Wave V was observed (Despland and Galambos, 1980; Sleifer et al., 2007; for a revision of 14 studies, see Stipdonk et al., 2016). This delay was associated with disruptions in the myelination and synaptogenesis process taking place on the fetal brain of the clinical group with direct affections on the central auditory system (Javel, 1980; Sleifer et al., 2007; Volpe, 2019).
Although ABR and FFR are both electrophysiological responses originating during the first milliseconds after the stimulus onset, their characteristics are considerably different. While the ABR morphology is characterized by a series of peaks related to each station of the auditory pathway from the eighth cranial nerve up to the inferior colliculus (Felix et al., 2018), the FFR morphology depends on the stimulus used to elicit it, becoming a mirror of it. Thus, this electrophysiological response allows us to investigate more precisely how the auditory brain captures the stimulus spectro-temporal components (Krizman and Kraus, 2019).
The FFR analysis of the present study revealed that late preterm neonates, compared to term neonates, exhibit a delayed neural response of complex sounds, as revealed by the neural lag parameter, and an impoverished neural encoding of a specific component of the speech input–its fundamental frequency (F0)–, as revealed by means of the spectral amplitude parameter. Regarding the neural lag, Madrid et al. (2021) described a latency decrement with age in preterm neonates. However, the GA of their preterm neonates (i.e., ≤ 32 weeks of GA), as well as the lack of a control group, limits the possibility to compare their results directly with the present findings. Novitskiy et al. (2023) explored whether the neural encoding of three different speech stimuli differed between a group of preterm infants delivered from 22 to 34 weeks of gestation and a group of term infants delivered from 38 to 40 weeks of gestation. To that end, different parameters related to response synchronization and response power were retrieved from the FFR. A main group effect was found for some synchronization parameters, with higher values in the term than in the preterm infants. On the other hand, no group differences were observed in measures related to response power. However, the length of gestation of the preterm sample, the age of the sample and other methodological differences hinders the comparison our results with those of Novitskiy et al. (2023).
The present study contributes to better understanding the variability and significance of FFR responses in different neonatal conditions (Musacchia et al., 2020; Ribas-Prats et al., 2022, 2023). In neonates affected by progressive moderate hyperbilirubinemia, the FFR parallels bilirubin levels, allowing to monitor neurotoxicity decrements resulting from phototherapy (Musacchia et al., 2020). In term small-for-gestational-age, a poor neural encoding of the stimulus F0 was observed by means of the normalized spectral amplitude, quantified as SNR at the vowel region, and these neural encoding disruptions were associated with specific white matter affections (Ribas-Prats et al., 2022). In those neonates located at the opposite extreme of the birth weight continuum -a condition known as large for gestational age (LGA)-, smaller spectral amplitudes for the consonant transition and for the vowel were found, and these observations in the LGA group were linked to a disrupted fine-grained central auditory system microstructure due to adipose tissue accumulation. Taking into account the potential clinical implications of the neonatal FFR, we suggest that the implementation of the FFR recordings in the clinical routines as a part of the auditory screening could have a promising impact to minimize cognitive consequences arising from any neonatal medical condition.
We speculate that the present FFR results could be related to disruptions occurring in the fetal brain growth of late preterm neonates, with special affection to synaptogenesis processes and white matter, in particular disruptions in the myelination progression (Javel, 1980; Sleifer et al., 2007; Volpe, 2019). By the 26th week of gestation linear arrays of oligodendrocytes outline the axon of the cochlear nerve and brainstem pathways (Moore et al., 1995). By week 29 this myelination process extended to the entire auditory pathway, including the commissure and the brachium of the inferior culliculus (IC; Moore et al., 1995) which is a primary generator of the FFR (Coffey et al., 2019; Gorina-Careta et al., 2021). From week 29 of gestation onwards, myelination density rises in all pathways and does not reach its maximum until at least 1 year of postnatal age (Moore et al., 1995). The increased density of myelination has been linked to a major synchronized conduction of auditory impulses from the cochlear nerve to the end of the auditory system, and several studies focused on the ABR disclosed a decreased wave V latency associated with these myelination trajectories (Johnson et al., 2008; Sharma et al., 2016). Regarding the FFR, differences in myelinization density between preterm and term neonates could lead to a decreased synchronization in the auditory impulses’ transmission in the clinical group and, thus, to a poorest robustness with which language stimuli are encoded, as observed here. Also, the functionality of the auditory nervous system in the clinical group may also be impaired due to hypoxic episodes occurring with a significant major incidence in late term neonates compared to term neonates during the first days of life (before 43 weeks corrected postconceptional age; Ramanathan et al., 2001; Williams et al., 2019).
On the other hand, the lack of significant results at the consonant transition region could be explained because of the rapid frequency changes occurring during a brief period (i.e., consonant transition length of 47 ms compared to the 113 ms for the vowel region). These fast fluctuations would demand a precise phase-locking at the stimulus F0 that is not detected in the neonates’ auditory system, which is still in structural and functional development (Moore and Guan, 2001; Moore and Linthicum, 2007), as it was observed in a previous FFR clinical study in which a group of term neonates affected by fetal growth restriction was compared with a healthy term neonate group (Ribas-Prats et al., 2022).
Regarding the neural encoding of the stimulus F1, the lack of group differences supports the existence of a different maturational pattern for each of the speech cues under analysis (i.e., stimulus F0 and F1; Anderson et al., 2015; Van Dyke et al., 2017; Arenillas-Alcón et al., 2021; Ribas-Prats et al., 2023). In addition, the lack of effects reported in the present study compared to the significant higher improvement in the neural encoding of F1 from 1 to 0 months of age recently described by Ribas-Prats et al. (2023), suggests that is the environmental exposure during the perinatal period which may explain the auditory system refinement needed to catch the neural encoding of the stimulus F1.
The interest to explore the neural encoding of both the stimulus F0 and its F1 is because they provide two different angles on speech processing abilities. Indeed, the neural encoding of F0 is critical for early language acquisition, as it facilitates the cutting of the continuous speech into the linguistic elements of the discourse (François et al., 2017) and contributes to talker identification, promoting first social interactions (Mary and Yegnanarayana, 2008). On the other hand, the neural encoding of the stimulus F1 supports phoneme discrimination (Diehl and Lindblom, 2004; Hornickel et al., 2009) and speech comprehension (Hopkins et al., 2008; Moore, 2008).
Finally, it should be noted that the inclusion of two term neonate groups allowed us to explore age effects, which has been largely described in longitudinal and cross sectional FFR studies (Anderson et al., 2015; Van Dyke et al., 2017; Arenillas-Alcón et al., 2021; Ribas-Prats et al., 2023). Exploring the magnitude of the FFR group differences in each of the parameters analyzed, it is evident that the differences are more abrupt between those neonates born preterm and those neonates born early term, than between neonates born early and those born late term. Thus, this finding suggests that the last weeks of gestation are crucial for brain development and for the white matter formation (Javel, 1980; Sleifer et al., 2007; Volpe, 2019). On the other hand, the lack of differences between early and late term neonates leads to better understanding of how FFR responses stabilize over time in early childhood as it was suggested by Llanos et al. (2017).
While the present study contributes to better understanding the neurodevelopmental limitations associated to late prematurity, further longitudinal studies are crucial, in which the neural encoding of stimulus F1 could be explored as the effects of specific social environment variables, since the experience-dependent auditory plasticity by means of the FFR have been widely described (Lau et al., 2017, 2019; Llanos et al., 2017; Reetzke et al., 2018; Ribas-Prats et al., 2023). Also, the presentation of more complex sounds such as the use of background noise (White-Schwoch et al., 2015; Musacchia et al., 2018; Thompson et al., 2019) or even the use of continuous speech in FFR studies could be promising to increase the potential implications for clinical assessments and interventions, the external validity and to give rise to stronger conclusions (Kulasingham et al., 2020; Arenillas-Alcón et al., 2021). As stated in the title of the present research, this study is just a pilot proof of concept on the investigation of FFR in late preterm neonates with the inclusion of two (early and late) term neonates for comparison. Thus, one of the major limitations of the study was the sample size. Another important limitation is the lack of sociodemographic data. To discriminate the effects of prematurity from other relevant variables such as social environment or economic status on different aspects of language encoding, those studies with a larger sample size should include these variables in the statistical analysis as covariates. Hence, future studies with larger samples, including cohorts for each term and preterm group based on the GA classification proposed by the WHO and the American College of Obstetricians and Gynecologists (ACOG Practice Bulletin, 2021; World Health Organization, 2023: for prematurity: extremely preterm, very preterm, moderate, and late preterm; for term deliveries: early term, full term, late term, and post term), are needed to better understand and address the specific needs of each of these preterm populations, thus fostering critical improvements in the current clinical guidelines for preterm infants.
Conclusion
The present study indicates that late preterm neonates exhibit a delayed neural response to complex sounds and an impoverished neural encoding of the stimulus fundamental frequency compared to term neonates born around the 38 and the 41 weeks of gestational age. Our results disclosed specific effects in the vowel region only and are compatible with the myelination and synaptogenesis affections observed in late preterm neonates. Yet, further studies are to be conducted to test whether the neural encoding affections disclosed here by means of the FFR could be causing a ‘knock-on effect’: a dysfunction at birth that may point to the beginning of a developmental problem that increases with age.
Data availability statement
The raw data supporting the conclusions of this article will be made available by the authors, without undue reservation.
Ethics statement
The studies involving humans were approved by Bioethics Committee of Sant Joan de Déu Barcelona Children’s Hospital. The studies were conducted in accordance with the local legislation and institutional requirements. Written informed consent for participation in this study was provided by the participants’ legal guardians/next of kin.
Author contributions
TR-P: Conceptualization, Data curation, Formal analysis, Investigation, Methodology, Software, Visualization, Writing – original draft, Writing – review & editing. SA-A: Data curation, Investigation, Supervision, Visualization, Writing – review & editing. SM: Conceptualization, Supervision, Writing – review & editing. MG-R: Conceptualization, Supervision, Validation, Writing – review & editing. CE: Conceptualization, Funding acquisition, Investigation, Project administration, Resources, Supervision, Validation, Writing – review & editing.
Funding
This work was supported by the Spanish Ministry of Science and Innovation PGC2018-094765-B-I00 project (MCIN/AEI/10.13039/501100011033/ FEDER “Una manera de hacer Europa”); the project PID2021-122255NB-100 supported by MCIN/AEI/10.13039/501100011033/FEDER, UE; the María de Maeztu Center of Excellence CEX2021-001159-M (supported by MCIN/AEI/10.13039/501100011033); the 2021SGR-00356 Consolidated Research Group of the Catalan Government, and the ICREA Acadèmia Distinguished Professorship awarded to CE.
Conflict of interest
The authors declare that the research was conducted in the absence of any commercial or financial relationships that could be construed as a potential conflict of interest.
The author(s) declared that they were an editorial board member of Frontiers, at the time of submission. This had no impact on the peer review process and the final decision.
Publisher’s note
All claims expressed in this article are solely those of the authors and do not necessarily represent those of their affiliated organizations, or those of the publisher, the editors and the reviewers. Any product that may be evaluated in this article, or claim that may be made by its manufacturer, is not guaranteed or endorsed by the publisher.
References
ACOG Practice Bulletin (2021). Prediction and prevention of spontaneous preterm birth. Number 234. Obstet. Gynecol. 138, e65–e90. doi: 10.1097/AOG.0000000000004479
Aeby, A., De Tiège, X., Creuzil, M., David, P., Balériaux, D., Van Overmeire, B., et al. (2013). Language development at 2 years is correlated to brain microstructure in the left superior temporal gyrus at term equivalent age: a diffusion tensor imaging study. NeuroImage. 78, 145–151. doi: 10.1016/j.neuroimage.2013.03.076
Aiken, S. J., and Picton, T. W. (2008). Envelope and spectral frequency-following responses to vowel sounds. Hear. Res. 245, 35–47. doi: 10.1016/j.heares.2008.08.004
American Academy of Pediatrics, Joint Committee on Infant Hearing (2019). Year 2019 position statement: principles and guidelines for early hearing detection and intervention programs. J. Early Hear. Detect. Intervent. 4, 1–44. doi: 10.15142/fptk-b74
Anderson, S., Parbery-Clark, A., White-Schwoch, T., and Kraus, N. (2015). Development of subcortical speech representation in human infants. J. Acoust. Soc. Am. 137, 3346–3355. doi: 10.1121/1.4921032
Arenillas-Alcón, S., Costa-Faidella, J., Ribas-Prats, T., Gómez-Roig, M. D., and Escera, C. (2021). Neural encoding of voice pitch and formant structure at birth as revealed by frequency-following responses. Sci. Rep. 11:6660. doi: 10.1038/s41598-021-85799-x
Arenillas-Alcón, S., Ribas-Prats, T., Puertollano, M., Mondéjar-Segovia, A., Gómez-Roig, M. D., Costa-Faidella, J., et al. (2023). Prenatal daily musical exposure is associated with enhanced neural representation of speech fundamental frequency: evidence from neonatal frequency-following responses. Dev. Sci. 26:e13362. doi: 10.1111/desc.13362
Barman, A., Prabhu, P., Mekhala, V. G., Vijayan, K., and Narayanan, S. (2021). Electrophysiological findings in specific language impairment: a scoping review. Hear. Balance Commun 19, 26–30. doi: 10.1080/21695717.2020.1807277
Barre, N., Morgan, A., Doyle, L. W., and Anderson, P. J. (2011). Language abilities in children who were very preterm and/or very low birth weight: a meta-analysis. J. Pediatr. 158, 766–774.e1. doi: 10.1016/j.jpeds.2010.10.032
Bidelman, G. M., and Momtaz, S. (2021). Subcortical rather than cortical sources of the frequency-following response (FFR) relate to speech-in-noise perception in normal-hearing listeners. Neurosci. Lett. 746:135664. doi: 10.1016/j.neulet.2021.135664
Carter, F. A., and Msall, M. E. (2017). Language abilities as a framework for understanding emerging cognition and social competencies after late, moderate, and very preterm birth. J. Pediatr. 181, 8–9. doi: 10.1016/j.jpeds.2016.10.077
Coffey, E., Nicol, T., White-Schwoch, T., Chandrasekaran, B., Krizman, J., Skoe, E., et al. (2019). Evolving perspectives on the sources of the frequency-following response. Nat. Commun. 10:5036. doi: 10.1038/s41467-019-13003-w
Cusson, R. M. (2003). Factors influencing language development in preterm infants. J. Obstet. Gynecol. Neonatal Nurs. 32, 402–409. doi: 10.1177/0884217503253530
Despland, P. A., and Galambos, R. (1980). Use of the auditory brainstem responses by prematures and newborns infants. Neuropadiatrie 11, 99–107. doi: 10.1055/s-2008-1071381
Diehl, R. L., and Lindblom, B. (2004). “Explaining the structure of feature and phoneme inventories: the role of auditory distinctiveness” in Speech processing in the auditory system. Springer handbook of auditory research. eds. A. B. Coffin and J. Sisneros, vol. 18 (New York: Springer).
Dodson, C. K., Travis, K. E., Borchers, L. R., Marchman, V. A., Ben-Shachar, M., and Feldman, H. M. (2018). White matter properties associated with pre-reading skills in 6-year-old children born preterm and at term. Dev. Med. Child Neurol. 60, 695–702. doi: 10.1111/dmcn.13783
Dong, Y., Chen, S. J., and Yu, J. L. (2012). A systematic review and meta-analysis of long-term development of early term infants. Neonatology 102, 212–221. doi: 10.1159/000338099
Engle, W. A., Tomashek, K. M., and Wallman, C.Committee on Fetus and Newborn, American Academy of Pediatrics (2007). “Late-preterm” infants: a population at risk. Pediatrics 120, 1390–1401. doi: 10.1542/peds.2007-2952
Escobar, G. J., Clark, R. H., and Greene, J. D. (2006). Short-term outcomes of infants born at 35 and 36 weeks gestation: we need to ask more questions. Semin. Perinatol. 30, 28–33. doi: 10.1053/j.semperi.2006.01.005
Felix, R. A., Gourévitch, B., and Portfors, C. V. (2018). Subcortical pathways: towards a better understanding of auditory disorders. Hear. Res. 362, 48–60. doi: 10.1016/j.heares.2018.01.008
Font-Alaminos, M., Ribas-Prats, T., Gorina-Careta, N., and Escera, C. (2021). Emergence of prediction error along the human auditory hierarchy. Hear. Res. 399:107954. doi: 10.1016/j.heares.2020.107954
François, C., Teixidó, M., Takerkart, S., Agut, T., Bosch, L., and Rodriguez-Fornells, A. (2017). Enhanced neonatal brain responses to sung streams predict vocabulary outcomes by age 18 months. Sci. Rep. 7:12451. doi: 10.1038/s41598-017-12798-2
Friederici, A. D. (2006). The neural basis of language development and its impairment. Neuron 52, 941–952. doi: 10.1016/j.neuron.2006.12.002
Gardi, J., Salamy, A., and Mendelson, T. (1979). Scalp-recorded frequency-following responses in neonates. Int. J. Audiol. 18, 494–506. doi: 10.3109/00206097909072640
Gorina-Careta, N., Kurkela, J., Hämäläinen, J., Astikainen, P., and Escera, C. (2021). Neural generators of the frequency-following response elicited to stimuli of low and high frequency: a magnetoencephalographic (MEG) study. NeuroImage 231:117866. doi: 10.1016/j.neuroimage.2021.117866
Gorina-Careta, N., Ribas-Prats, T., Arenillas-Alcón, S., Puertollano, M., Gómez-Roig, M. D., and Escera, C. (2022). Neonatal frequency-following responses: a methodological framework for clinical applications. Semin. Hear. 43, 162–176. doi: 10.1055/s-0042-1756162
Hall, J. W. (2000). Development of the ear and hearing. J Perinatol: official journal of the California Perinatal Association 20, S12–S20. doi: 10.1038/sj.jp.7200439
He, C., Hotson, L., and Trainor, L. J. (2007). Mismatch responses to pitch changes in early infancy. J. Cogn. Neurosci. 19, 878–892. doi: 10.1162/jocn.2007.19.5.878
Hee Chung, E., Chou, J., and Brown, K. A. (2020). Neurodevelopmental outcomes of preterm infants: a recent literature review. Transl. Pediatr. 9, S3–S8. doi: 10.21037/tp.2019.09.10
Hepper, P. G., and Shahidullah, B. S. (1994). Development of fetal hearing. Arch. Dis. Child. Fetal Neonatal Ed. 71, F81–F87. doi: 10.1136/fn.71.2.f81
Hopkins, K., Moore, B. C., and Stone, M. A. (2008). Effects of moderate cochlear hearing loss on the ability to benefit from temporal fine structure information in speech. J. Acoust. Soc. Am. 123, 1140–1153. doi: 10.1121/1.2824018
Hornickel, J., Skoe, E., Nicol, T., Zecker, S., and Kraus, N. (2009). Subcortical differentiation of stop consonants relates to reading and speech-in-noise perception. Proc. Natl. Acad. Sci. USA 106, 13022–13027. doi: 10.1073/pnas.0901123106
Howard, K., Roberts, G., Lim, J., Lee, K. J., Barre, N., Treyvaud, K., et al. (2011). Biological and environmental factors as predictors of language skills in very preterm children at 5 years of age. J. Dev. Behav. Pediatr. 32, 239–249. doi: 10.1097/DBP.0b013e31820b7882
Hunt, C. E. (2006). Ontogeny of autonomic regulation in late preterm infants born at 34-37 weeks postmenstrual age. Semin. Perinatol. 30, 73–76. doi: 10.1053/j.semperi.2006.02.005
Hüppi, P. S., Warfield, S., Kikinis, R., Barnes, P. D., Zientara, G. P., Jolesz, F. A., et al. (1998). Quantitative magnetic resonance imaging of brain development in premature and mature newborns. Ann. Neurol. 43, 224–235. doi: 10.1002/ana.410430213
Javel, E. (1980). Neurophysiological correlates of auditory maturation. The annals of otology, rhinology & laryngology. Supplement 89, 103–113. doi: 10.1177/00034894800890s526
Jeng, F. C., Hu, J., Dickman, B., Montgomery-Reagan, K., Tong, M., Wu, G., et al. (2011). Cross-linguistic comparison of frequency-following responses to voice pitch in American and Chinese neonates and adults. Ear Hear. 32, 699–707. doi: 10.1097/AUD.0b013e31821cc0df
Jeng, F. C., Lin, C. D., Chou, M. S., Hollister, G. R., Sabol, J. T., Mayhugh, G. N., et al. (2016b). Development of subcortical pitch representation in three-month-old Chinese infants. Percept. Mot. Skills 122, 123–135. doi: 10.1177/0031512516631054
Jeng, F. C., Lin, C. D., and Wang, T. C. (2016a). Subcortical neural representation to mandarin pitch contours in American and Chinese newborns. J. Acoust. Soc. Am. 139, EL190–EL195. doi: 10.1121/1.4953998
Jeng, F.-C., Schnabel, E. A., Dickman, B. M., Hu, J., Li, X., Lin, C.-D., et al. (2010). Early maturation of frequency-following responses to voice pitch in infants with Normal hearing. Percept. Mot. Skills 111, 765–784. doi: 10.2466/10.22.24.PMS.111.6.765-784
Johnson, K. L., Nicol, T., Zecker, S. G., and Kraus, N. (2008). Developmental plasticity in the human auditory brainstem. J. Neurosci. 28, 4000–4007. doi: 10.1523/JNEUROSCI.0012-08.2008
Karnati, S., Kollikonda, S., and Abu-Shaweesh, J. (2020). Late preterm infants - changing trends and continuing challenges. Int. J. Pediatr. Adolesc. Med. 7, 36–44. doi: 10.1016/j.ijpam.2020.02.006
Kinney, H. C. (2006). The near-term (late preterm) human brain and risk for periventricular leukomalacia: a review. Semin. Perinatol. 30, 81–88. doi: 10.1053/j.semperi.2006.02.006
Kramer, M. S., Demissie, K., Yang, H., Platt, R. W., Sauvé, R., and Liston, R. (2000). The contribution of mild and moderate preterm birth to infant mortality. Fetal and infant health study Group of the Canadian Perinatal Surveillance System. JAMA 284, 843–849. doi: 10.1001/jama.284.7.843
Krizman, J., and Kraus, N. (2019). Analyzing the FFR: a tutorial for decoding the richness of auditory function. Hear. Res. 382:107779. doi: 10.1016/j.heares.2019.107779
Kulasingham, J. P., Brodbeck, C., Presacco, A., Kuchinsky, S. E., Anderson, S., and Simon, J. Z. (2020). High gamma cortical processing of continuous speech in younger and older listeners. NeuroImage 222:117291. doi: 10.1016/j.neuroimage.2020.117291
Laptook, A., and Jackson, G. L. (2006). Cold stress and hypoglycemia in the late preterm ("near-term") infant: impact on nursery of admission. Semin. Perinatol. 30, 24–27. doi: 10.1053/j.semperi.2006.01.014
Lau, J. C., Wong, P. C., and Chandrasekaran, B. (2017). Context-dependent plasticity in the subcortical encoding of linguistic pitch patterns. J. Neurophysiol. 117, 594–603. doi: 10.1152/jn.00656.2016
Lau, J. C. Y., Wong, P. C. M., and Chandrasekaran, B. (2019). Interactive effects of linguistic abstraction and stimulus statistics in the online modulation of neural speech encoding. Atten. Percept. Psychophys. 81, 1020–1033. doi: 10.3758/s13414-018-1621-9
Lavie, A., Fisch, S., Reicher, L., Zohav, E., and Maslovitz, S. (2023). Correlation of meconium-stained amniotic fluid and adverse pregnancy outcomes between 37 to 39 and 40 to 42 weeks of gestational age. Am. J. Perinatol. 2023:8018. doi: 10.1055/a-2053-8018
Lemos, F. A., da Silva Nunes, A. D., de Souza Evangelista, C. K., Escera, C., Taveira, K. V. M., and Balen, S. A. (2021). Frequency-following response in newborns and infants: a systematic review of acquisition parameters. J. Speech Lang. Hear. Res. 64, 2085–2102. doi: 10.1044/2021_JSLHR-20-00639
Llanos, F., Xie, Z., and Chandrasekaran, B. (2017). Hidden Markov modeling of frequency-following responses to mandarin lexical tones. J. Neurosci. Methods 291, 101–112. doi: 10.1016/j.jneumeth.2017.08.010
Luck, S. J. (2005). An introduction to the event-related potential technique. Cambridge, Mass: MIT Press.
Madrid, A. M., Walker, K. A., Smith, S. B., Hood, L. J., and Prieve, B. A. (2021). Relationships between click auditory brainstem response and speech frequency following response with development in infants born preterm. Hear. Res. 407:108277. doi: 10.1016/j.heares.2021.108277
Mary, L., and Yegnanarayana, B. (2008). Extraction and representation of prosodic features for language and speaker recognition. Speech Comm. 50, 782–796. doi: 10.1016/j.specom.2008.04.010
Moon, I. J., and Hong, S. H. (2014). What is temporal fine structure and why is it important? Korean J. Audiol. 18, 1–7. doi: 10.7874/kja.2014.18.1.1
Moore, B. C. (2008). The role of temporal fine structure processing in pitch perception, masking, and speech perception for normal-hearing and hearing-impaired people. J. Assoc. Res. Otolaryngol. 9, 399–406. doi: 10.1007/s10162-008-0143-x
Moore, J. K., and Guan, Y. L. (2001). Cytoarchitectural and axonal maturation in human auditory cortex. J. Assoc. Res. Otolaryngol. 2, 297–311. doi: 10.1007/s101620010052
Moore, J. K., and Linthicum, F. H. Jr. (2007). The human auditory system: a timeline of development. Int. J. Audiol. 46, 460–478. doi: 10.1080/14992020701383019
Moore, J. K., Perazzo, L. M., and Braun, A. (1995). Time course of axonal myelination in the human brainstem auditory pathway. Hear. Res. 87, 21–31. doi: 10.1016/0378-5955(95)00073-d
Musacchia, G., Ortiz-Mantilla, S., Roesler, C. P., Rajendran, S., Morgan-Byrne, J., and Benasich, A. A. (2018). Effects of noise and age on the infant brainstem response to speech. Clin. Neurophysiol. 129, 2623–2634. doi: 10.1016/j.clinph.2018.08.005
Musacchia, G., Hu, J., Bhutani, V. K., Wong, R. J., Tong, M. L., Han, S., et al. (2020). Frequency-following response among neonates with progressive moderate hyperbilirubinemia. Journal of perinatology: official journal of the California Perinatal Association. 40, 203–211. doi: 10.1038/s41372-019-0421-y
Näätänen, R., Paavilainen, P., Rinne, T., and Alho, K. (2007). The mismatch negativity (MMN) in basic research of central auditory processing: a review. Clin. Neurophysiol. 118, 2544–2590. doi: 10.1016/j.clinph.2007.04.026
Nakatani, L. H., and Schaffer, J. A. (1978). Hearing "words" without words: prosodic cues for word perception. J. Acoust. Soc. Am. 63, 234–245. doi: 10.1121/1.381719
Nazzi, T., Nishibayashi, L. L., Berdasco-Muñoz, E., Baud, O., Biran, V., and Gonzalez-Gomez, N. (2015). Acquisition du langage chez l'enfant prématuré durant la première année de vie [Language acquisition in preterm infants during the first year of life]. Arch. Pediatr. 22, 1072–1077. doi: 10.1016/j.arcped.2015.07.002
Nepomnyaschy, L., Hegyi, T., Ostfeld, B. M., and Reichman, N. E. (2012). Developmental outcomes of late-preterm infants at 2 and 4 years. Matern. Child Health J. 16, 1612–1624. doi: 10.1007/s10995-011-0853-2
Northam, G. B., Liégeois, F., Tournier, J. D., Croft, L. J., Johns, P. N., Chong, W. K., et al. (2012). Interhemispheric temporal lobe connectivity predicts language impairment in adolescents born preterm. Brain: a journal of neurology. 135, 3781–3798. doi: 10.1093/brain/aws276
Novitskiy, N., Chan, P. H. Y., Chan, M., Lai, C. M., Leung, T. Y., Leung, T. F., et al. (2023). Deficits in neural encoding of speech in preterm infants. Dev. Cogn. Neurosci. 61:101259. doi: 10.1016/j.dcn.2023.101259
Otto-Meyer, S., Krizman, J., White-Schwoch, T., and Kraus, N. (2018). Children with autism spectrum disorder have unstable neural responses to sound. Exp. Brain Res. 236, 733–743. doi: 10.1007/s00221-017-5164-4
Palumbi, R., Peschechera, A., Margari, M., Craig, F., Cristella, A., Petruzzelli, M. G., et al. (2018). Neurodevelopmental and emotional-behavioral outcomes in late-preterm infants: an observational descriptive case study. BMC Pediatr. 18:318. doi: 10.1186/s12887-018-1293-6
Picheny, M. A., Durlach, N. I., and Braida, L. D. (1986). Speaking clearly for the hard of hearing. II: acoustic characteristics of clear and conversational speech. J. Speech Hear. Res. 29, 434–446. doi: 10.1044/jshr.2904.434
Pogribna, U., Burson, K., Lasky, R. E., Narayana, P. A., Evans, P. W., and Parikh, N. A. (2014). Role of diffusion tensor imaging as an independent predictor of cognitive and language development in extremely low-birth-weight infants. AJNR Am. J. Neuroradiol. 35, 790–796. doi: 10.3174/ajnr.A3725
Pujol, R., and Lavigne-Rebillard, M. (1992). Development of neurosensory structures in the human cochlea. Acta Otolaryngol. 112, 259–264. doi: 10.1080/00016489.1992.11665415
Rabie, N. Z., Bird, T. M., Magann, E. F., Hall, R. W., and McKelvey, S. S. (2015). ADHD and developmental speech/language disorders in late preterm, early term and term infants. J Perinatol: official journal of the California Perinatal Association 35, 660–664. doi: 10.1038/jp.2015.28
Raju, T. N., Higgins, R. D., Stark, A. R., and Leveno, K. J. (2006). Optimizing care and outcome for late-preterm (near-term) infants: a summary of the workshop sponsored by the National Institute of Child Health and Human Development. Pediatrics 118, 1207–1214. doi: 10.1542/peds.2006-0018
Ramanathan, R., Corwin, M. J., Hunt, C. E., Lister, G., Tinsley, L. R., Baird, T., et al. (2001). Cardiorespiratory events recorded on home monitors: comparison of healthy infants with those at increased risk for SIDS. JAMA 285, 2199–2207. doi: 10.1001/jama.285.17.2199
Reetzke, R., Xie, Z., Llanos, F., and Chandrasekaran, B. (2018). Tracing the trajectory of sensory plasticity across different stages of speech learning in adulthood. Curr. Biol. 28, 1419–1427.e4. doi: 10.1016/j.cub.2018.03.026
Ribas-Prats, T., Almeida, L., Costa-Faidella, J., Plana, M., Corral, M. J., Gómez-Roig, M. D., et al. (2019). The frequency-following response (FFR) to speech stimuli: a normative dataset in healthy newborns. Hear. Res. 371, 28–39. doi: 10.1016/j.heares.2018.11.001
Ribas-Prats, T., Arenillas-Alcón, S., Lip-Sosa, D. L., Costa-Faidella, J., Mazarico, E., Gómez-Roig, M. D., et al. (2022). Deficient neural encoding of speech sounds in term neonates born after fetal growth restriction. Dev. Sci. 25:e13189. doi: 10.1111/desc.13189
Ribas-Prats, T., Arenillas-Alcón, S., Pérez-Cruz, M., Costa-Faidella, J., Gómez-Roig, M. D., and Escera, C. (2023). Speech-encoding deficits in neonates born large-for-gestational age as revealed with the envelope frequency-following response. Ear Hear. 44, 829–841. doi: 10.1097/AUD.0000000000001330
Richard, C., Neel, M. L., Jeanvoine, A., Connell, S. M., Gehred, A., and Maitre, N. L. (2020). Characteristics of the frequency-following response to speech in neonates and potential applicability in clinical Practice: a systematic review. J. Speech Lang. Hear. Res. 63, 1618–1635. doi: 10.1044/2020_JSLHR-19-00322
Rushaidin, M. M., Salleh, S. H., Swee, T. T., Najeb, J. M., and Arooj, A. (2009). Wave V detection using instantaneous energy of auditory brainstem response signal. Am. J. Appl. Sci. 6, 1669–1674. doi: 10.3844/ajassp.2009.1669.1674
Russo, N. M., Skoe, E., Trommer, B., Nicol, T., Zecker, S., Bradlow, A., et al. (2008). Deficient brainstem encoding of pitch in children with autism Spectrum disorders. Clin. Neurophysiol. 119, 1720–1731. doi: 10.1016/j.clinph.2008.01.108
Seubert, D. E., Stetzer, B. P., Wolfe, H. M., and Treadwell, M. C. (1999). Delivery of the marginally preterm infant: what are the minor morbidities? Am. J. Obstet. Gynecol. 181, 1087–1091. doi: 10.1016/s0002-9378(99)70086-4
Shapiro-Mendoza, C. K., Tomashek, K. M., Kotelchuck, M., Barfield, W., Weiss, J., and Evans, S. (2006). Risk factors for neonatal morbidity and mortality among "healthy," late preterm newborns. Semin. Perinatol. 30, 54–60. doi: 10.1053/j.semperi.2006.02.002
Sharma, M., Bist, S. S., and Kumar, S. (2016). Age-related maturation of wave V latency of auditory brainstem response in children. J. Audiol. Otol. 20, 97–101. doi: 10.7874/jao.2016.20.2.97
Skoe, E., and Kraus, N. (2010). Auditory brain stem response to complex sounds: a tutorial. Ear Hear. 31, 302–324. doi: 10.1097/AUD.0b013e3181cdb272
Sleifer, P., da Costa, S. S., Cóser, P. L., Goldani, M. Z., Dornelles, C., and Weiss, K. (2007). Auditory brainstem response in premature and full-term children. Int. J. Pediatr. Otorhinolaryngol. 71, 1449–1456. doi: 10.1016/j.ijporl.2007.05.029
Stene-Larsen, K., Brandlistuen, R. E., Lang, A. M., Landolt, M. A., Latal, B., and Vollrath, M. E. (2014). Communication impairments in early term and late preterm children: a prospective cohort study following children to age 36 months. J. Pediatr. 165, 1123–1128. doi: 10.1016/j.jpeds.2014.08.027
Stipdonk, L. W., Franken, M. J. P., and Dudink, J. (2018). Language outcome related to brain structures in school-aged preterm children: a systematic review. PLoS One 13:e0196607. doi: 10.1371/journal.pone.0196607
Stipdonk, L. W., Weisglas-Kuperus, N., Franken, M. C., Nasserinejad, K., Dudink, J., and Goedegebure, A. (2016). Auditory brainstem maturation in normal-hearing infants born preterm: a meta-analysis. Dev. Med. Child Neurol. 58, 1009–1015. doi: 10.1111/dmcn.13151
Swamy, G. K., Ostbye, T., and Skjaerven, R. (2008). Association of preterm birth with long-term survival, reproduction, and next-generation preterm birth. JAMA 299, 1429–1436. doi: 10.1001/jama.299.12.1429
Thompson, E. C., Estabrook, R., Krizman, J., Smith, S., Huang, S., White-Schwoch, T., et al. (2021). Auditory neurophysiological development in early childhood: a growth curve modeling approach. Clin. Neurophysiol. 132, 2110–2122. doi: 10.1016/j.clinph.2021.05.025
Thompson, E. C., Krizman, J., White-Schwoch, T., Nicol, T., Estabrook, R., and Kraus, N. (2019). Neurophysiological, linguistic, and cognitive predictors of children's ability to perceive speech in noise. Dev. Cogn. Neurosci. 39:100672. doi: 10.1016/j.dcn.2019.100672
Tomashek, K. M., Shapiro-Mendoza, C. K., Weiss, J., Kotelchuck, M., Barfield, W., Evans, S., et al. (2006). Early discharge among late preterm and term newborns and risk of neonatal morbidity. Semin. Perinatol. 30, 61–68. doi: 10.1053/j.semperi.2006.02.003
Travis, K. E., Ben-Shachar, M., Myall, N. J., and Feldman, H. M. (2016). Variations in the neurobiology of reading in children and adolescents born full term and preterm. NeuroImage Clin. 11, 555–565. doi: 10.1016/j.nicl.2016.04.003
Van Dyke, K. B., Lieberman, R., Presacco, A., and Anderson, S. (2017). Development of phase locking and frequency representation in the infant frequency-following response. J. Speech Lang. Hear. Res. 60, 2740–2751. Advance online publication. doi: 10.1044/2017_JSLHR-H-16-0263
Vohr, B. (2014). Speech and language outcomes of very preterm infants. Semin. Fetal Neonatal Med. 19, 78–83. doi: 10.1016/j.siny.2013.10.007
Volpe, J. J. (2019). Dysmaturation of premature brain: importance, cellular mechanisms, and potential interventions. Pediatr. Neurol. 95, 42–66. doi: 10.1016/j.pediatrneurol.2019.02.016
Ward, R. M. (2006). Drug disposition in the late preterm ("near-term") newborn. Semin. Perinatol. 30, 48–51. doi: 10.1053/j.semperi.2006.01.013
White-Schwoch, T., Magohe, A. K., Fellows, A. M., Rieke, C. C., Vilarello, B., Nicol, T., et al. (2020). Auditory neurophysiology reveals central nervous system dysfunction in HIV-infected individuals. Clin. Neurophysiol. 131, 1827–1832. doi: 10.1016/j.clinph.2020.04.165
White-Schwoch, T., Woodruff Carr, K., Thompson, E. C., Anderson, S., Nicol, T., Bradlow, A. R., et al. (2015). Auditory processing in noise: a preschool biomarker for literacy. PLoS Biol. 13:e1002196. doi: 10.1371/journal.pbio.1002196
World Health Organization . (2023) Sexual and reproductive health. Available at: www.who.int/reproductive-health. (Accessed July 21, 2023).
Williams, L. Z. J., McNamara, D., and Alsweiler, J. M. (2019). Intermittent hypoxemia in infants born late preterm: a prospective cohort observational study. J. Pediatr. 204, 89–95.e1. doi: 10.1016/j.jpeds.2018.08.048
Wolke, D., Samara, M., Bracewell, M., and Marlow, N. (2008). Specific language difficulties and school achievement in children born at 25 weeks of gestation or less. J. Pediatr. 152, 256–262.e1. doi: 10.1016/j.jpeds.2007.06.043
Yoshinaga-Itano, C., Sedey, A. L., Coulter, D. K., and Mehl, A. L. (1998). Language of early- and later-identified children with hearing loss. Pediatrics 102, 1161–1171. doi: 10.1542/peds.102.5.1161
Keywords: speech-ABR, FFR, infants, preterm, language
Citation: Ribas-Prats T, Arenillas-Alcón S, Martínez SIF, Gómez-Roig MD and Escera C (2024) The frequency-following response in late preterm neonates: a pilot study. Front. Psychol. 15:1341171. doi: 10.3389/fpsyg.2024.1341171
Edited by:
Antonio Pereira, Federal University of Pará, BrazilReviewed by:
Gabriella Musacchia, University of the Pacific, United StatesMaria Elizabeth Barnes-Davis, Cincinnati Children’s Hospital Medical Center, United States
Copyright © 2024 Ribas-Prats, Arenillas-Alcón, Martínez, Gómez-Roig and Escera. This is an open-access article distributed under the terms of the Creative Commons Attribution License (CC BY). The use, distribution or reproduction in other forums is permitted, provided the original author(s) and the copyright owner(s) are credited and that the original publication in this journal is cited, in accordance with accepted academic practice. No use, distribution or reproduction is permitted which does not comply with these terms.
*Correspondence: Carles Escera, Y2VzY2VyYUB1Yi5lZHU=