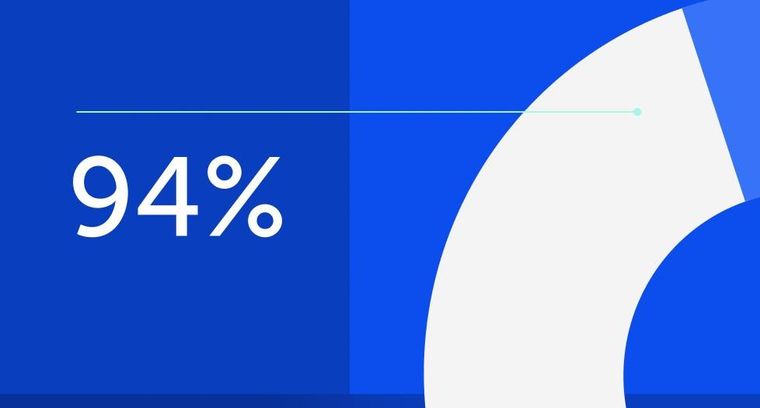
94% of researchers rate our articles as excellent or good
Learn more about the work of our research integrity team to safeguard the quality of each article we publish.
Find out more
ORIGINAL RESEARCH article
Front. Psychol., 13 July 2022
Sec. Psychology for Clinical Settings
Volume 13 - 2022 | https://doi.org/10.3389/fpsyg.2022.944838
This article is part of the Research TopicPresent and Future of EMDR in Clinical Psychology and Psychotherapy, volume IIView all 17 articles
Eye movement desensitization and reprocessing (EMDR) therapy is a well-established therapeutic method to treat post-traumatic stress disorder (PTSD). However, how EMDR exerts its therapeutic action has been studied in many types of research but still needs to be completely understood. This is in part due to limited knowledge of the neurobiological mechanisms underlying EMDR, and in part to our incomplete understanding of PTSD. In order to model PTSD, we used a biologically inspired computational model based on firing rate units, encompassing the cortex, hippocampus, and amygdala. Through the modulation of its parameters, we fitted real data from patients treated with EMDR or classical exposure therapy. This allowed us to gain insights into PTSD mechanisms and to investigate how EMDR achieves trauma remission.
Post-traumatic stress disorder (PTSD) is a maladaptive reaction to traumatic events characterized by American Psychiatric Association (2013): (a) intrusiveness of distressing memories of the traumatic event, that occur in response to reminder cues of the trauma; (b) avoidance of the trauma-associated cues; (c) negative alterations in cognitions and mood; (d) hyperarousal. Many studies found that the activation of the amygdala during the exposure to trauma reminders or fearful stimuli is significantly correlated with the severity of PTSD symptoms (Rauch et al., 2000; Pissiota et al., 2002; Fredrikson and Furmark, 2003; Shin et al., 2004; Protopopescu et al., 2005; Ganzel et al., 2007; Brohawn et al., 2010; Jacques et al., 2011; McLaughlin et al., 2014; Neumeister et al., 2017; Stevens et al., 2017). Also, the reduction of the amygdala activation after the treatment correlates with the success of psychotherapy in attenuating the symptoms (Peres et al., 2011; Thomaes et al., 2014; King et al., 2016). Moreover, patients suffering from PTSD show reduced recruitment of the brain areas involved in emotion regulation, such as the ventromedial prefrontal cortex (vmPFC) and the dorsolateral prefrontal cortex (dlPFC), when facing cues associated with trauma (Rauch et al., 2006; Liberzon and Sripada, 2007; Kasai et al., 2008; Milad et al., 2009; Shin and Liberzon, 2010).
Post-traumatic stress disorder does not occur in all individuals that experience trauma, suggesting that specific susceptibility factors determine if the disorder will develop or not (Alisic et al., 2014; Musazzi et al., 2018). For instance, during the extinction of Pavlovian fear conditioning, patients with PTSD reveal hypoactivation of the vmPFC, compared to healthy controls (Milad et al., 2009; Rougemont-Bücking et al., 2011), although it is not known whether this alteration has to be considered a cause or a consequence of the trauma (Yehuda, 1999; Kasai et al., 2008; Sun et al., 2013; Miller et al., 2017; Alexander et al., 2020).
Prolonged exposure (PE) to threatening stimuli and eye movement desensitization and reprocessing (EMDR) are first line therapeutic strategies for PTSD (World Health Organization, 2013; Schnyder et al., 2015; Cusack et al., 2016; Gainer et al., 2020). PE consists of several sessions of exposure to the trauma-related stimuli in a safe context. The exposure can also be imaginal. In the latter case, the therapist asks the patient to relive the traumatic experience as it was happening at that precise moment (Foa and Rothbaum, 2001). When the distress arising from physical or imaginal exposure is too high, PE can be paired with anxiety reduction techniques, such as slow breathing or mindfulness (Brewer, 2001; Frye and Spates, 2012).
It has been suggested that vmPFC activation during exposure and the resulting downregulation of the amygdala are key factors of PE therapy (Stojek et al., 2018). It is worth noting that patients with PTSD in whom the vmPFC is more active during emotional conflict tasks benefit from a greater symptoms reduction after PE (Fonzo et al., 2017). This is in agreement with the proposed role of the vmPFC in discriminating safety signals and inhibiting the amygdala during fear extinction (Phelps et al., 2004; Schiller et al., 2008; Feng et al., 2016; Fullana et al., 2016; Via et al., 2018; Tashjian et al., 2021). Murine studies and simulations confirm this picture. Indeed, the homologous brain region in mice—the infralimbic cortex—is progressively recruited during the exposure to no longer threatening conditioned stimuli and promotes synaptic plasticity and fear extinction in the amygdala through long range projections (Garcia et al., 1999; Milad and Quirk, 2002; Cho et al., 2013; Moustafa et al., 2013; Senn et al., 2014; Do-Monte et al., 2015a; Mattera et al., 2020). Even though the vmPFC is believed to fire in response to safe stimuli and context in an automatic way (Gyurak et al., 2011), it has been shown that it can also be recruited endogenously and actively, for example through exercises of mindfulness (Zeidan et al., 2014).
The mechanisms of action of EMDR have been widely debated (Lohr et al., 1998; Herbert et al., 2000; Rogers and Silver, 2002; Lee et al., 2006; Pagani et al., 2017; de Voogd et al., 2018; Landin-Romero et al., 2018; Baek et al., 2019; Holmes, 2019). During EMDR sessions, the patient is instructed to keep the most disturbing image, the negative feelings, beliefs, emotions, and the body sensations associated with the trauma in mind, while following an alternating bilateral stimulation (e.g., right-left hand movements, bilateral fingers tapping, or bilateral auditory stimuli) from the therapist (Gainer et al., 2020). An important characteristic of this therapy is that patients show a faster symptom improvement, usually in 6–8 sessions (Power et al., 2002; Shapiro, 2014; Proudlock and Peris, 2020), compared to the PE recovery that lasts on average 12 sessions (Foa and Rothbaum, 2001; Banducci, 2021).
The neurobiological correlates of EMDR have been investigated in real time, with millisecond resolution, through electroencephalography (EEG) recorded during the whole session. Notably, the bilateral stimulation induces an immediate synchronization of all cortical areas in the delta band (1–4 Hz; Harper et al., 2009; Pagani et al., 2011, 2012). On the basis of these results, it has been proposed that slow waves arising during EMDR enact a sleep-like mechanism of memory consolidation (Pagani et al., 2017). Indeed, during sleep, recent memory traces are reactivated simultaneously in the hippocampus and the slowly oscillating sensory and prefrontal cortices (Sirota et al., 2003; Ji and Wilson, 2007; Peyrache et al., 2009; Helfrich et al., 2019). It is thought that this process is the basis of a hippocampus-to-cortex transfer, where episodic memories can be integrated into the existing cognitive schemes (Sirota et al., 2003; Mölle and Born, 2009; Diekelmann and Born, 2010). Experimental disruption of memory reactivation or impairment of slow waves impinges memory consolidation (Miyamoto et al., 2016, 2017). On the other hand, a stimulation mimicking slow waves induces long term potentiation in neocortical neurons (Chauvette et al., 2012; Sandler et al., 2016) and enhances memory retention (Miyamoto et al., 2016). Theoretical investigations indicate that slow oscillations boost synaptic plasticity and associative learning between cortical areas (Wei et al., 2016; Capone et al., 2019; Golosio et al., 2021).
The adaptive information processing (AIP) model of Shapiro proposes that traumatic memories are not integrated into the existing memory networks and remain stored in a maladaptive form (Solomon and Shapiro, 2008). The slow waves-promoting effect of EMDR suggests that therapy would promote the transfer of memories from the hippocampus-amygdala complex to the cortex, where they can be integrated into the associative cortical networks; this would allow to process the traumatic memory in an adaptive form, leaving the cognitive aspects of the memory intact, while erasing the associated emotional trace (Stickgold, 2002; Pagani et al., 2017). As in Shapiro's AIP model, the cortical transfer would help to make sense of the trauma by connecting the memory with the previously acquired cognitive schemes (Solomon and Shapiro, 2008).
Besides slow oscillations, another important insight into the mechanisms of EMDR comes from the Working Memory Hypothesis (de Voogd et al., 2018; Landin-Romero et al., 2018). This hypothesis suggests that tasks engaging in working memory reduce traumatic memory intrusion and downregulate the amygdala (Holmes et al., 2009; Qin et al., 2009; Schweizer et al., 2013; James et al., 2015; Iyadurai et al., 2018). It has been observed that bilateral eye movements during the presentation of conditioned stimuli previously associated with an electric shock activate the dlPFC and inhibit the amygdala, similarly to a working memory task. Moreover, it has been shown that, contrary to PE therapy, the vmPFC is deactivated by bilateral eye movement during the processing of traumatic memories (de Voogd et al., 2018). This evidence suggests that PE and EMDR exert their therapeutic action through the recruitment of different subsets of the PFC areas possibly having different efficacy in fear inhibition.
As many brain areas are involved, the integration of the phenomena involved in PTSD and its therapies in a whole coherent framework posits a challenge. Moreover, we still lack models of PTSD able to account for the whole complexity of the disease. On one hand, imaging research in patients often has substantial problems with resolution and reliability (Nord et al., 2017; Kredlow et al., 2022), and does not allow insight into the actual computations exerted by the investigated brain area (Logothetis, 2008). On the other hand, murine models permit a finer resolution and manipulation of the circuits and neuronal populations, but often fail to recapitulate the actual characteristics of the disorder. In particular, while Pavlovian fear conditioning—the most used PTSD-mimicking protocol in mice—reproduces some aspects of PTSD (Mahan and Ressler, 2012; Verbitsky et al., 2020), the continuous exposure to the conditioned stimulus no longer associated with the noxious stimulus causes the extinction of conditioned fear after some trials (Mattera et al., 2020), while PTSD symptoms are particularly resistant to extinction and can last decades (Morgan et al., 2003; Chapman et al., 2012; Careaga et al., 2016). Moreover, although humans can extinguish Pavlovian fear with exposure (Kalisch et al., 2006), in the case of PTSD the exposure to trauma reminders outside a therapeutic context can worsen the symptoms (Eysenck, 1982; Hassija and Gray, 2007).
Computational models can be a tool to face the outlined complex problem through the integration of disparate experimental information in the same theoretical framework (Eliasmith and Trujillo, 2014; Nair et al., 2016). Models are constrained by experimental data to gain biological plausibility and, at the same time, can incorporate new hypotheses to be tested. The emergent properties of the model produce insights into the mechanisms possibly underlying the studied phenomena, and allow predictions that are testable in future experiments, grounded on the hypotheses incorporated in the model and the data used to constraint it (Shen and McNaughton, 1996; Nair et al., 2016). Here, we used a biologically inspired neural network to model PTSD, and the effects of PE and EMDR, to verify the computational plausibility and coherence of the proposed mechanisms of action. In particular, to reproduce the overall effect of EMDR, we adjusted two parameters representing the inhibitory activity of PFC on the amygdala and the enhanced cortical learning rate induced by the slow waves. Moreover, the simulations allowed us to reproduce experimental data and gain insights into their neurobiological implications.
The article is organized as follows. First, we describe the PTSD model and its biological underpinnings (Section 2). Then, we test its robustness through the reproduction of different PTSD related phenomena (Sections 3.1, 3.2). Next, we reproduce the proposed mechanisms underlying PE and EMDR to simulate data from patients (Nijdam et al., 2012) and derive information regarding the different time courses of the two therapies (Sections 3.3, 3.4). Finally, we discuss the results of the simulations and their contribution to the understanding of PTSD (Section 4.1) and EMDR (Section 4.2).
The neural units forming the model are leaky units (Dayan and Abbott, 2001), each representing the activity of a population of neurons with the same electrophysiological properties (Moustafa et al., 2013; Carrere and Alexandre, 2015; Mannella et al., 2016; Mattera et al., 2020). These units are characterized by membrane potential and a firing rate. In leaky units the change of the unit potential in time, , is represented with the following differential equation, approximated with the Euler method:
where τ is the time constant, I is the external input to the unit (representing the activation of the sensory cortices, the recalling signal to the hippocampus, the safety signal to the vmPFC in PE or the eye movement in EMDR, the trauma to the amygdala; refer to Figure 1), wpost, pre is the weight of the connection between the pre- and post-synaptic unit, Fpre is the firing rate of the presynaptic units. The firing rate of the unit, Fpost, was calculated with the hyperbolic tangent function tanh(x):
where θ is the firing threshold and [x]+ the positive function ([x]+ = x if x≥0, and [x]+ = 0 if x <0). The connection weights at the beginning of the simulation, as well as the firing thresholds of the neurons, are listed in the Supplementary Table 1.
Figure 1. Model architecture: units and connections of the model. EMDR acts on the strength of the inhibition from the PFC (vmPFC in PE and dlPFC in EMDR) to the amygdala through the parameter ϕ, and on the learning rate of the connections between the sensory units and the PFC through the parameter ψ.
The model involves four areas (Figure 1), representing the sensory cortices, the hippocampus, the amygdala, and the PFC. The hippocampal-amygdala network is organized as a bidirectional associative network, inspired by Alvarez and Squire (1994). It has been theorized (Tryon, 1998), but never shown with simulations (Radell et al., 2017) that this kind of neural network would recapitulate the symptoms of PTSD. Associative networks are indeed capable of pattern completion, i.e., the ability to reconstruct a whole pattern from a single cue (Alvarez and Squire, 1994; Tryon, 1998), and this is a characteristic of the intrusive memory flashbacks that follow a trauma-reminder cue (Brewin, 2015; Ehlers, 2015).
The hippocampus receives input from unimodal and multimodal sensory cortices, that can converge in the same hippocampal population (Fried et al., 1997; Quiroga et al., 2009). Lateral inhibition and sparse connectivity at the dentate gyrus level ensure the separation of information, thus strongly segregated patterns of contextual input from sensory cortices activate non-overlapping hippocampal populations (Norman and O'Reilly, 2003; Rolls, 2013; Espinoza et al., 2018). In the model, these characteristics are captured by a winner-take-all architecture (Alvarez and Squire, 1994). The initial weights from the neuronal units in the sensory cortices to the hippocampal units and from the hippocampus to the sensory cortices are extracted randomly from a uniform distribution (Supplementary Table 1). This allows a different activation of the hippocampal units for each possible input pattern. A strong lateral inhibition between the hippocampal units ensures that only one hippocampal unit wins the competition and fires persistently in response to a sensory pattern. On the other hand, each sensory modality is represented by two mutually inhibiting units (units A1 and A2 represent the auditory cortex, S1 and S2 the somatosensory cortex, and V1 and V2 the visual cortex). Importantly, the sensory cortices in Figure 1 represent both primary cortices and also higher level unimodal cortices. Differently from Alvarez and Squire (1994), which used simplified firing rate neurons, we did not implement lateral excitatory connections between the sensory cortices. This has been done to avoid a perpetual activation induced by an excessive recurrent excitation due to the more realistic dynamic nature of the leaky neurons used here. This implies that, in our model implementation, the memory trace is not transferred form the hippocampus to the cortex, as in Alvarez and Squire (1994). Since obtaining this feature with the more realistic neurons used here would have been overly complicated, we decided to not consider this aspect as it goes beyond the scope of the research objective pursued here.
It has been shown that recalling a particular experience reactivates the same hippocampal population that fired at the moment of the actual experience (Rolls and Xiang, 2006; Gelbard-Sagiv et al., 2008). Moreover, this internally generated hippocampal reactivation is capable of reinstating the original cortical representations (Wheeler et al., 2000; Tanaka et al., 2014). Our model reproduces this property through an associative learning process taking place at the level of the reciprocals connections between the sensory cortices and the hippocampus (Alvarez and Squire, 1994; Rolls, 2007; Schwindel and McNaughton, 2011). This learning process is driven by the Bienenstock-Cooper-Munro (BCM) learning rule (refer to Section 2.3).
Hippocampus and amygdala are reciprocally connected (Figure 1), as shown by anatomical investigation (Pitkänen et al., 2000). In murine, hippocampal projections directly target amygdala fear neurons, i.e., the neuronal population that becomes active after the establishment of fear conditioning (Herry et al., 2008). In particular, the hippocampus conveys contextual information to the amygdala (Phillips and LeDoux, 1992; Kim and Cho, 2017) through synaptic projections that are potentiated by fear learning (Kim and Cho, 2020). In humans, after contextual fear conditioning, the context associated with an electric shock evokes a coupled activation in both hippocampus and amygdala, as revealed by imaging studies (Alvarez et al., 2008; Marschner et al., 2008; Lang et al., 2009; Baeuchl et al., 2015).
Finally, the amygdala is under the inhibitory control of the PFC. vmPFC and dlPFC are respectively engaged in PE (Phelps et al., 2004; Schiller et al., 2008; Feng et al., 2016; Fullana et al., 2016; Via et al., 2018; Tashjian et al., 2021) and in EMDR therapy (de Voogd et al., 2018). In the model (Figure 1), the PFC unit represents vmPFC or dlPFC, depending on the therapy that is being simulated and receives plastic inputs from the unimodal sensory cortices. These should not be intended to represent direct anatomical projection, but functional indirect connections that are modulated by learning (Bhanji et al., 2019; Ginty et al., 2019). In Figure 1, and throughout the article, the weight of the connection between the PFC and amygdala is indicated by ϕ. The initial value of ϕ (Sections 3.1, 3.2, 3.3) was set to −1 (Supplementary Table 1). Subsequently (Section 3.4), it was modified according to the results of the grid search algorithm that was used to tune the parameters: this was done to reflect a possible difference in the amygdala inhibition efficacy of vmPFC and dlPFC. Indeed, we reasoned that if EMDR recruits different PFC areas compared to PE, this would be reflected in a different total efficacy of amygdala inhibition (the parameter ϕ) by the whole vmPFC/dlPFC compound.
The weights of the plastic connections (refer to Figure 1) are updated according to a simplified BCM learning rule (Bienenstock et al., 1982):
where α is the learning rate, ρ is the plasticity threshold (Supplementary Table 1), Fpost and Fpre are the post- and pre-synaptic firing rates. Following this rule, a connection undergoes long-term potentiation (LTP) or long-term depression (LTD) if the firing of the post-synaptic unit is respectively above or below the threshold ρ. The weights are clipped within a (Wmax, Wmin) range (Supplementary Table 1).
An influential paradigm states that the cortex is a slow learner, while the subcortical structures are fast learners. This would allow the gradual integration of episodic memories with previous knowledge through slow consolidation, and favor the integration rather than interference between old and newly acquired memories (McClelland et al., 1995; Frankland and Bontempi, 2005). This theory is supported by experimental findings concerning the temporal dynamics of memory transfer from subcortical to neocortical zones (Zola-Morgan and Squire, 1990; Kim and Fanselow, 1992; Quinn et al., 2008; Do-Monte et al., 2015b), by the well-known difficulty of producing synaptic plasticity in neocortical slices compared to hippocampal ones (Bear et al., 1992; Bear and Kirkwood, 1993), and by computational models (Alvarez and Squire, 1994; Hattori, 2014). Even though some cases of remarkably fast cortical engram formation have been found (Kitamura et al., 2017; Brodt et al., 2018), it has been shown that rapid cortical learning requires that the new information is consistent with previously acquired cognitive schemes (Tse et al., 2007, 2011; Squire et al., 2015; Kumaran et al., 2016).
We incorporated slow cortical learning into the equation driving synaptic plasticity in our model. In particular, the learning rate of the connections between the sensory cortex and the dlPFC/vmPFC is 10 times slower. We choose this value accordingly to Alvarez and Squire (1994), where an order of magnitude of difference in cortico-cortical learning rate compared to the hippocampal learning rate allowed the reproduction of the gradual hippocampus-cortex information transfer found in experiments. The model also reproduces the plasticity-promoting effect of slow waves induced by bilateral stimulation in EMDR (Chauvette et al., 2012; Sandler et al., 2016; refer to Section 1). In particular, we simulated this effect by modifying the strength of the plasticity acting on the cortical connections through the parameter ψ (refer to Figure 1):
Input units to the model (Figure 1) are binary units that can be in two different states, “off” and “on”. Only in the “on” state do they send an input equal to 1 to the postsynaptic unit.
The sensory cortex units are organized in mutually inhibiting couples: A1- A2, S1-S2, V1-V2, representing neuronal populations in the auditory cortices, somatosensory cortices, and visual cortices. Among the 8 possible patterns of stimulation, we have chosen A1-S1-V1 to be associated with the trauma (Pattern 1) and A2-S2-V2 as a control (Pattern 2). The model receives other three inputs: the trauma unit, which is connected to the amygdala, the recalling unit, which is connected to the hippocampus, and the safety/eye movement unit, which represents the input to the PFC during the PE or EMDR therapy.
To model trauma establishment and the subsequent PTSD, we used a protocol consisting of 35 trials (Section 3.1). First, the baseline activation of the hippocampus and amygdala were measured with a single trial of stimulation (Trial 1; one trial lasted 104 time steps; an interval of 104 time steps separated the trials). In trial 1, we activated the input to V1 or the input to V2 without the other elements of the patterns. During the PTSD establishment (Trial 2, corresponding to 3 · 104 timesteps), we activated the whole Pattern 1 together with the trauma binary unit, or Pattern 2 without the trauma input. For the remaining trials (each lasting 104 timesteps) we stimulated V1 or V2 to observe if a single cue was able to activate (a) the amygdala (PTSD establishment), and (b) the hippocampus and the other units of the pattern (memory acquisition).
The simulation of the increased vmPFC excitability in Section 3.2 was obtained by halving the firing threshold θ of the unit. The experience of an event with mild emotional activation was simulated in Section 3.2 by reducing the activation of the unit Trauma (refer to Figure 1) from 1 to 0.3.
To model PE or EMDR therapy (Sections 3.3, 3.4), we first established PTSD as aforementioned. Then we activated the recalling unit projecting to the hippocampal unit associated with the memory trace from trial 11. At the same time, we activated the PFC unit to represent a safety signal delivered to the vmPFC in case of PE, or the eye movement-induced activation of dlPFC in case of EMDR. This protocol corresponds to the reactivation of the traumatic memories during the therapy (imaginal exposure or recalling), associated with the recruitment of the respective cortical areas recruited by the PE and the EMDR (refer to Section 1). After each therapy trial, we delivered a test trial, with only the V1 activation while stopping learning, to measure the PE or EMDR trial-by-trial effect on the cue-induced traumatic memory reactivation. Note that in the figures in Sections 3.3, 3.4, we only show the test trials (V1 activation) after each therapy trial (hippocampus plus PFC activation).
We fitted the data from Nijdam et al. (2012) through a grid search of the parameters ϕ and ψ (Section 2.4). The test reported in Nijdam et al. (2012) compared the timing of symptoms decline in PTSD patients treated with brief eclectic psychotherapy (consisting of session 1 of psychoeducation, sessions from 2 to 6 of imaginal exposure, and the following sessions of cognitive therapy) with that of patients treated with EMDR. Through the measurement of the “Impact of Events Scale - Revised” score (IES-R), they showed a session-by-session decline of symptoms in the two groups of patients. We took the average group data from sessions 2 to 6, where imaginal exposure was administrated to the patients under brief eclectic psychotherapy, and compared them to the corresponding sessions in the EMDR group. The IES-R was normalized to the maximum value and the values were re-scaled, subtracting the IES-R baseline reached after the whole therapy. In order to reproduce the patients' curves of symptom remission after PE and EMDR in Nijdam et al. (2012) we leveraged the fact that the activation of the amygdala is significantly correlated with PTSD symptoms severity (Rauch et al., 2000; Pissiota et al., 2002; Fredrikson and Furmark, 2003; Shin et al., 2004; Protopopescu et al., 2005; Ganzel et al., 2007; Brohawn et al., 2010; Jacques et al., 2011; Peres et al., 2011; McLaughlin et al., 2014; Thomaes et al., 2014; King et al., 2016; Neumeister et al., 2017; Stevens et al., 2017) to reproduce, with the activation of the amygdala unit in our model, the curves of symptom remission after the PE and EMDR in Nijdam et al. (2012) patients. The parameters ϕ and ψ were allowed to vary respectively in steps of 0.05 and 0.5 and ranges of 0.5–2.0 and 0.5–8.5. We measured the distance of the simulations from the real data with the root-mean-squared error (RMSE; Granato and Baldassarre, 2021).
During the first trial of the protocol, we activated the input unit to V1 or V2 only, representing two different visual cues to the visual cortex. In both cases, we observed no activity in the hippocampus or the amygdala (trial 1 in Figures 2A,B). In the second trial, the whole pattern 1 (traumatic pattern: A1-S1-V1 + trauma) or the whole pattern 2 (control pathway: A2-S2-V2) was activated. In this case, the hippocampus firing is induced for both patterns, whereas the amygdala firing is caused only by pattern 1, as a consequence of the activation of the trauma input unit (trial 2 in Figures 2A,B). Note that we observed hippocampal activity only in one unit per pattern, as we show below. During the following trials (trials 3 to 35, Figures 2A,B), we activated only the sensory units V1 or V2.
Figure 2. Effect of trauma establishment in hippocampus and amygdala, compared to the control. In the first trial, the baseline activity of the hippocampus (A) and amygdala (B) were measured during the stimulation of the V1 or the V2 sensory units. In trial 2, pattern 1 (A1-S1-V1) was coactivated with the trauma unit; as a control, pattern 2 (A2-S2-V2) was activated with the trauma unit turned off. During trial 3 and succeeding trials, V1 and V2 were repeatedly activated to investigate the dynamics of the hippocampus and amygdala response. After memory establishment in trial 2, V2 stimulation induces the activation of the hippocampus (A, white dots, trials 3–30), but not of the amygdala (B, white dots, trials 3-35). On the other hand, the trauma-associated stimulus V1 induces both hippocampus (A, black dots, trials 3–35) and amygdala (B, black dots, trials 3–35). Over time, the non-traumatic memory trace is lost (A, white dots, trial 35), while traumatic associated stimulus V1 is persistently capable to activate the hippocampus (A, black dots, trial 35) and amygdala (B, black dots, trial 35).
Because of the winner-take-all architecture described in Materials and methods, the patterns 1 and 2 activate different units in the hippocampus (Figures 3A,C). While V2 induces an hippocampal activation that slowly fades away during subsequent trials (Figure 2A, white dots: compare trial 3 with trial 35), V1 induces a robust and persistent firing in both hippocampus and amygdala (Figures 2A,B, black dots). This indicates that the initially neutral cue V1 became associated with a threat, causing a persistent amygdala activation.
Figure 3. Model memory formation in PTSD and control conditions. (A) The graph corresponds to trial 3 of Figure 2 in the condition with the trauma. V1 activates the hippocampal unit H3 and the cortical units S1 and A1 encoding the other elements of pattern 1. (B) The graph corresponds to trial 35 of Figure 2 in the trauma condition. The memory trace continues to be activated by V1 even after many trials. (C) The graph corresponds to trial 3 of Figure 2 in the control condition with no trauma. V2 activates H2, a different unit of the hippocampus with respect to V1, and also the other cortical elements of the pattern, S2 and A2, although to a lesser extent with respect to PTSD [note the different scales in (A,C)]. (D) The graph corresponds to trial 35 of Figure 2 in the control condition. After 35 trials, the memory trace is lost and V1 does not elicit activations (the memory has been transferred to the cortex).
One of the principal features of PTSD is the emotional flashbacks induced by a trauma reminder. We observed that the presentation of V1 after trauma establishment drives the activation of the hippocampus and also of the other sensory cortices, reinstating the whole pattern 1 (traumatic flashback; Figure 3A). This reinstatement effect is persistent even after several trials (Figure 3B). Instead, the presentation of V2 weakly activates another hippocampal unit (corresponding to a different hippocampal engram) and transiently reinstates the memory trace in the cortex (pattern completion, with the activation of S2 and A2; Figure 3C). However, the memory retrieval induced by V2 is not emotionally loaded (there is no amygdala activation, Figure 2A) and is lost after some trials (Figure 3D).
It has been hypothesized that reduced recruitment of vmPFC is a risk factor for developing PTSD while its higher activation might protect from it (refer to Section 1; Milad et al., 2009; Rougemont-Bücking et al., 2011). We, thus, investigated whether an increase in the excitability of vmPFC would protect the model from developing a persistent activation of the amygdala after the exposure to a traumatic event. We observed that, with a more excitable vmPFC induced by halving its activation threshold (refer to Sections 2, 2.4, for details), the V1 cue still induces amygdala unit activation (trial 3). However, similar to a fear extinction protocol (Mattera et al., 2020), after some trials of exposure to V1 the amygdala unit ceases to activate while the hippocampus remains active even at trial 35 (Figures 4A,B). Thus, the model where the vmPFC is less excitable is predisposed to develop PTSD after a traumatic experience while the model where the vmPFC is more easily recruited is resilient to PTSD and shows a spontaneous fear extinction with exposure to stimuli associated with the trauma.
Figure 4. Effect of the high excitability of vmPFC on the triggering of post-traumatic stress disorder (PTSD). Hippocampus (A) and amygdala (B) activity before (trial 1: V1 input), during (trial 2: V1-A1-S1-Trauma input) and after (trial 3–35: V1 input) trauma, in a model where the vmPFC firing threshold is halved (white dots) compared to the model shown in Figure 2 acquiring the PTSD (black dots).
As observed in Figures 2A, 3D (Section 3.1), the model does not establish a long term memory of the elements of the pattern if the emotional (Trauma) unit is not activated. In humans and mice, the longevity of memory traces depends on the emotional charge associated with experiences to be encoded (Hamann et al., 1999; Akirav and Richter-Levin, 2002; Kilpatrick and Cahill, 2003; Huff and Rudy, 2004; Phelps et al., 2004; Huff et al., 2006; Roozendaal et al., 2009; Murty et al., 2011; Hansen, 2017). We, thus, reasoned that in the model a weak amygdala activation during the experience of a sensory pattern (V1-A1-S1) would induce a subsequent enhancement of memory retention. This might correspond to a mild emotional engagement accompanying an experience. To test this, during trial 2 of the protocol we exposed the version of the model seen above, non-predisposed to develop PTSD (high vmPFC excitability), to the pattern V1-A1-S1, together with an activation of 0.1 (instead of 1) of the emotional (Trauma) unit. In this condition, we observed that the hippocampal unit activation persisted throughout all the subsequent trials of V1 presentation, while a pattern that was not associated with the mild amygdala unit activity progressively faded away (Figures 5A,B).
Figure 5. Effect a mild emotional engagement on memory retention. Hippocampus (A) and amygdala (B) activity with an activation protocol as in Figure 2, except that the vmPFC firing threshold is halved (model robust with respect to PTSD) and that during the second trial the emotional (Trauma) unit is weakly activated (1/10 of activation) to represent a mild (non-traumatic) emotional engagement of the amygdala.
Next, we investigated whether the PTSD model is capable of trauma symptoms remission through different trials of hippocampal reactivation (mimicking imaginal exposure) coupled with vmPFC activation (mimicking safety signals from the therapy setting). As shown in Figures 6A,B, after the first 10 trials (baseline: trial 1; trauma: trial 2; after-trauma: trials 3–10), we delivered PE therapy for 20 trials (trials 11–30). For each trial of therapy (not shown in figures), we did a subsequent test trial of V1 exposure, to observe the effect of PE on hippocampus and amygdala activation (dots in the figure). PE progressively reduces V1-induced recruitment of the hippocampus (Figure 6A) and amygdala (Figure 6B).
Figure 6. Effect of PE on hippocampus (A) and amygdala (B). The first 10 trials were as in Figure 1: trial 1 was the baseline V1 activation of hippocampus and amygdala; trial 2 marked the trauma experience (A1-S1-V1-Trauma); trials 3–10 involved the re-experience of V1 to observe the effect of the trauma. After trial 10, however, we administered PE for 20 sessions (20 trials in the model). PE consisted in the activation of the hippocampal memory trace (unit H3 from the Figures 3A and B), coupled with the activation of vmPFC. Each time point in trials 11–30 corresponds to a V1-activation test after a PE session (trial). After the 20 trials of therapy, hippocampal activation is reduced to a plateau (A) and amygdala activation becomes zero (B): the memory has been freed from the negative emotional load.
Finally, we investigated whether the fast symptom improvement that follows EMDR could be explained by an increase in the cortical learning rate, induced by slow waves, or by a more powerful amygdala inhibition from dlPFC, compared to vmPFC. For this purpose, we modulated the cortical learning rate ψ and the weight ϕ of the inhibitory connection from the PFC to the amygdala to find the best fit of the data from Nijdam et al. (2012). The results of this test show that the set of ϕ and ψ parameters that provide the most accurate fitting of the PE data and EMDR data are well-segregated from other values producing a worse fitting (Figures 7A,B, 8A,B).
Figure 7. Patients data fitting. (A) Root square MSE for the fitting of the PE data from Nijdam et al. (2012). (B) Root square MSE for the fitting of the EMDR data from Nijdam et al. (2012).
Figure 8. Eye movement desensitization and reprocessing therapy is compared to PE therapy. Each dot in (A,B) represents a simulation to fit the data of PE (A) and EMDR (B) in Nijdam et al. (2012). The size and the color (from blue to yellow) of the dots are proportional to the root square MSE: the best fitting model is marked by a red dot. (C) Comparison between the symptoms remission curves obtained with the model, using the parameter combinations indicated by the red dots in graphs (A) PE and (B) EMDR, and the actual experimental data from Nijdam et al. (2012). For the real data, the PTSD index is represented by the normalized IES-R score (Nijdam et al., 2012; refer to Section 3.4); in the model, the PTSD index represents the normalized amygdala unit activation following the presentation of the reminder cue. (D) PFC activation in correspondence to the simulation trials in (C).
In particular, the best combination for PE (ψ = 1.5, ϕ = 1; MSE = 0.039; Figure 8A) and the best combination for EMDR (ψ = 5, ϕ = 1.3; MSE = 0.075; Figure 8B) imply that the cortical learning rate is more than tripled during EMDR with respect to PE, and dlPFC has a 30% higher capacity to inhibit amygdala compared to vmPFC in PE. This results in a faster amygdala deactivation (Figure 8C) and a higher PFC recruitment (Figure 8D) over the course of the therapy sessions.
To investigate the mechanisms of trauma establishment and extinction in PE and EMDR, we analyzed the modifications of the connection weights after trauma and PE or EMDR therapy (Figures 8A,B). After trauma, the connection from the hippocampus to the amygdala is potentiated (Figure 8B), while the sensory connections to the vmPFC and dlPFC are almost unchanged (Figure 8A). Both therapies cause LTP onto the sensory-prefrontal connections (Figure 8A) and LTD onto the hippocampus-amygdala connections (Figure 8B). However, compared to PE, EMDR induces a stronger cortical connection strengthening, and this results in a higher PFC activation (Figure 9A) and a faster amygdala inhibition (Figure 9B).
Figure 9. Model weight modification due to trauma and therapy. (A) Connection weights linking the sensory cortices to the PFC. (B) Connection weights linking the hippocampus to the amygdala.
This study has presented a model capable of recapitulating a core symptom of PTSD, namely the emotional flashbacks enacted by a trauma-reminding cue and the consequent amygdala hyperactivation. An initial neutral cue can be associated with other neutral cues through the sensory-cortex-to-hippocampus and hippocampus-to-sensory-cortex plastic connections. Then, the representation of one of the cues can reactivate the other cortices through the hippocampal hub (Figures 2A, 3C), similarly to the associative cortex-hippocampus network in Alvarez and Squire (1994).
Following previous theoretical predictions (Tryon, 1998; Radell et al., 2017), we showed in Figures 3A,B how a bidirectional associative neural network can indeed be used to model the traumatic flashback in PTSD. Indeed, after a pattern of cues has been temporally coupled with the amygdala activation, a reminding cue reinstates not only different associated elements encoded in other cortices, but also activates the amygdala (Figure 2B). This is in line with data reporting amygdala activation in patients with PTSD exposed to trauma-related stimuli (Liberzon et al., 1999; Pissiota et al., 2002; Shin et al., 2004; Protopopescu et al., 2005).
On the other hand, a pattern of stimuli non-associated with the emotional activation of the amygdala is not retained for long in the hippocampus (Figures 2A, 3C,D). This is coherent with the well-known role of the amygdala in the modulation of synaptic plasticity and memory formation in the hippocampus, both in rodents and in humans (Hamann et al., 1999; Akirav and Richter-Levin, 2002; Kilpatrick and Cahill, 2003; Huff and Rudy, 2004; Phelps et al., 2004; Huff et al., 2006; Roozendaal et al., 2009; Murty et al., 2011). Interestingly, it has been shown that, besides fear neurons, the murine amygdala contains reward neurons activated by positive experiences (Kim et al., 2016; Zhang and Li, 2018; Lutas et al., 2019). These neurons send projections to the hippocampus and potentiate spatial memory consolidation (Yang et al., 2016; Yang and Wang, 2017). Together, these observations contribute to the idea that the amygdala, as well as other brain areas associated with a positive or negative emotional content (e.g., locus coeruleus; Hansen, 2017) signal salient memories to be retained in the hippocampus, as also shown in our simulations (Figures 5A,B). The mechanism of amygdala-induced memory consolidation described above, however, can become maladaptive and lead to PTSD. In our model, the ability of PFC to recruit the amygdala inhibiting areas determines if a traumatic memory will be spontaneously extinguished by the exposure to a trauma-reminding cue or not (Figures 4A,B). In particular, when PFC is not sufficiently intrinsically excitable for a spontaneous trauma remission, the exposure must be paired with an external activation of the PFC, represented in our model by a “safety” input (Figure 1) and in real patients by the therapeutic setting. Indeed, feeling safe during the imaginal reliving of traumatic memories is an important component of the therapy, and it has been suggested that patients gradually learn to incorporate safety information into the traumatic memories (Rothbaum and Schwartz, 2002). Coherently with this view, over the course of the PE sessions, our model learns to autonomously recruit the PFC when the trauma-reminding cue is presented (refer to PE simulation in Figure 8D). This relies on an increase in the strength of the connections between the sensory areas and the PFC (Figure 9A).
While a high percentage of the population is exposed to traumatic events, PTSD only occurs in a subgroup (Alisic et al., 2014; Musazzi et al., 2018) of people for which the symptoms can last even 40 years (Orr et al., 1993). Three main hypotheses, each supported by some experimental findings, have been proposed to explain the susceptibility to the trauma and its long term persistence (Careaga et al., 2016). One hypothesis suggests that some individuals are predisposed to PTSD because of a higher conditionability, meaning that the association between the conditioned stimulus and the unconditioned stimulus is acquired more strongly than in the healthy population (Orr et al., 2000; Blechert et al., 2007; but refer to Milad et al., 2008). A second hypothesis proposes that, in patients with PTSD, the distressing conditioned responses acquire themselves the role of unconditioned stimuli, resulting in a self-strengthening cycle. This predicts a fear incubation effect (Eysenck, 1982), where the conditioned fear responses would become greater over time with the repeated presentation of the trauma-reminding cues, instead of being extinguished (Sandin and Chorot, 2002; but see Nicholaichuk et al., 1982). A third hypothesis postulates a defective extinction (Davis et al., 2000), supported by the fact that patients with PTSD, compared to healthy controls, show deficits in extinction learning and recall in Pavlovian fear paradigms (Blechert et al., 2007; Milad et al., 2008).
In our model, the PFC excitability determines the susceptibility to the trauma. We did not find any difference in the conditionability when the models with hypo- and hyper-excitable PFC were confronted (Figures 4A,B), thus supporting the experimental findings of Milad et al. (2008) rather than those of Orr et al. (2000). Instead, the model shows that reduced PFC recruitment results in defective extinction (Figures 4A,B). Coherently with these results, it has been observed that extinction retention in a protocol of Pavlovian fear and the vmPFC activation are correlated and that patients with PTSD are defective in both (Milad et al., 2009).
Finally, we found a fear incubation effect in the model (refer to trials 3–8 in Figure 2B), where the presentation of the trauma-related cue V1, without the trauma unit activation, induces further conditioning. This occurs because, in the model, the association between the memory trace and the trauma resides in the strength of the connection between the hippocampus and the amygdala (Figure 9B), as suggested by research in murine (contextual fear conditioning; Kim and Cho, 2020) and humans (correlation between hippocampus-amygdala functional connectivity and IES-R scores; Li et al., 2017). In the absence of sufficient inhibition from the PFC, as in the simulation of Figure 2, the loop between the hippocampus and amygdala (Figure 1) enacts the self-strengthening cycle between the conditioned stimulus and the conditioned response hypothesized by the fear incubation paradigm (Eysenck, 1982).
Despite initial skepticism, due to the discussion about underlying neurobiological mechanisms (Lohr et al., 1998; Herbert et al., 2000), several meta-analyses and international guidelines show that EMDR is an effective treatment for PTSD (INSERM Collective Expertise Centre, 2004; Ursano et al., 2004; Bisson et al., 2007, 2019; World Health Organization, 2013; de Roos et al., 2017; Khan et al., 2018; Lewey et al., 2018; Navarro et al., 2018; Wilson et al., 2018; Karatzias et al., 2019; Bastien et al., 2020; Mavranezouli et al., 2020). Our model allowed us to analyze patients' data in search of the most suitable parameter set that explains the experimental findings. Coherently with the positive correlation found between IES-R measurement of PTSD symptoms and amygdala activation (McLaughlin et al., 2014), we could reproduce the IES-R curves from Nijdam et al. (2012) using the activity of the amygdala as a proxy. The parameters found by the grid search analysis suggest that PE and EMDR make use of different mechanisms to exert their therapeutic effect (Figures 7, 8). While cognitive and exposure therapies are centered on the activities focusing on the traumatic memories, during an EMDR session, the patient is invited to notice the trauma with a distant attitude (“Imagine you are on the train and the scenery is passing by. Just notice the scenery without trying to grab hold of it or make it significant.”; from Shapiro, 1995). It is known that distancing and distraction activate the prefrontal, cingulate, and parietal cortices (among which are the dlPFC) and are very effective in emotion regulation, in particular in amygdala downregulation (McRae et al., 2010; Kanske et al., 2011; Dörfel et al., 2014). Moreover, de Voogd et al. (2018) observed that the dlPFC is activated following bilateral eye stimulation. The parameter ϕ of the model, which in EMDR resulted to be 30% higher than in PE (Figures 8A,B), indicates that: (1) the regions recruited by EMDR are different from the regions recruited by PE; (2) EMDR-recruited regions have a higher capacity to inhibit amygdala compared to the regions activated during PE. The parameter ψ, which is 3.3 times higher in EMDR than PE (Figures 8A,B), indicates an enhanced cortical learning rate during the bilateral stimulation, as suggested by the slow waves recorded during the therapy in patients (Harper et al., 2009; Pagani et al., 2011, 2012). The physiological alternation during sleep between slow waves and rapid eye movement periods promotes memorization and facilitates the elaboration and contextualization of traumatic memories (Carletto et al., 2017). The evidence that EMDR therapy induces the appearance of slow waves concurrently with bilateral stimulation speaks in favor of faster synaptic and neuronal plasticity and hence faster processing of traumatic memories as compared to other psychotherapies (Pagani et al., 2012, 2017).
The model reproduces simplified connectomics between sensory cortical areas and the PFC, where direct connections reach the vmPFC and dlPFC from unimodal sensory areas. Indeed, secondary sensory cortices have a role in storing and retrieval of fear memory content (Sacco and Sacchetti, 2010) and PFC receives direct inputs from the whole cortex in murine, primates, and humans (Öngür and Price, 2000; Ährlund-Richter et al., 2019). However, relevant evidence suggests the presence, for fear-related input, of at least a relay station (the anterior cingulate cortex) between secondary cortices and PFC (van Heukelum et al., 2020; Kredlow et al., 2022). Another area not considered in the model, that has been suggested to be involved in PTSD (Yoshii, 2021) and, at least in murine, is the effect of alternating bilateral sensory stimulation (Baek et al., 2019), is the thalamus. The level of detail and the number of brain regions included in the model are a trade-off between biological plausibility and computational complexity, in order to test a large scale hypothesis of the PTSD network and the PE and EMDR mechanisms while maintaining the number of parameters reasonably low (Eliasmith and Trujillo, 2014). This allowed us to avoid a large number of model degrees of freedom as well as to perform a grid search with hundreds of simulations. Future study could include more brain areas in this model in order to investigate their potential role in trauma and therapy.
Two limitations concern the data fitting. First, we did not fit data from the single patients, but only from the average curve reported in the literature (Nijdam et al., 2012). This provided a proof of concept of the putative PE and EMDR mechanisms, but a more robust analysis will be performed in the future on the single patients if the data will be obtainable. This would test the robustness of the conclusions drawn from the present study on a dataset containing individual participant data. Moreover, it would allow the use of the model as a tool to investigate the individual differences in trauma remission, response to the therapy, and PTSD susceptibility factors. Second, we used the amygdala activation as a proxy of patient symptoms, measured in the original work with the IES-R. Although several experiments have found a linear correlation between the symptoms severity and the amygdala recruitment (refer to Section 1; in particular, for the IES-R refer to McLaughlin et al., 2014), the best solution would be to use the amygdala activation in the model to fit fMRI data from patients. This would require fMRI measurements of the amygdala activation during the exposition to trauma reminders, taken between different therapeutic sessions of EMDR or PE. To our knowledge, these datasets are currently not available in the literature. Despite the technical limitations of the procedure, this would furnish a novel and powerful link between the time course of amygdala deactivation, the time course of symptoms remission during therapy, and the computational modeling of the underlying mechanisms.
The original contributions presented in the study are included in the article/Supplementary Material, further inquiries can be directed to the corresponding author/s.
AM, MP, and GB conceived the model and wrote this article. AM and AC conducted the simulation. AM, AC, GG, MP, and GB analyzed the results. All authors reviewed the article and approved the submitted version.
This project has received funding from the European Union's Horizon 2020 Research and Innovation Programme under Grant Agreement No. 713010 (GOAL-Robots—Goal-based Open-ended Autonomous Learning Robots) and a dedicated Grant from EMDR Italy Association.
The authors declare that the research was conducted in the absence of any commercial or financial relationships that could be construed as a potential conflict of interest.
All claims expressed in this article are solely those of the authors and do not necessarily represent those of their affiliated organizations, or those of the publisher, the editors and the reviewers. Any product that may be evaluated in this article, or claim that may be made by its manufacturer, is not guaranteed or endorsed by the publisher.
The Supplementary Material for this article can be found online at: https://www.frontiersin.org/articles/10.3389/fpsyg.2022.944838/full#supplementary-material
Ährlund-Richter, S., Xuan, Y., van Lunteren, J. A., Kim, H., Ortiz, C., Dorocic, I. P., et al. (2019). A whole-brain atlas of monosynaptic input targeting four different cell types in the medial prefrontal cortex of the mouse. Nat. Neurosci. 22, 657–668. doi: 10.1038/s41593-019-0354-y
Akirav, I., and Richter-Levin, G. (2002). Mechanisms of amygdala modulation of hippocampal plasticity. J. Neurosci. 22, 9912–9921. doi: 10.1523/JNEUROSCI.22-22-09912.2002
Alexander, K. S., Nalloor, R., Bunting, K. M., and Vazdarjanova, A. (2020). Investigating individual pre-trauma susceptibility to a PTSD-like phenotype in animals. Front. Syst. Neurosci. 13, 85. doi: 10.3389/fnsys.2019.00085
Alisic, E., Zalta, A. K., Van Wesel, F., Larsen, S. E., Hafstad, G. S., Hassanpour, K., et al. (2014). Rates of post-traumatic stress disorder in trauma-exposed children and adolescents: meta-analysis. Brit. J. Psychiatry 204, 335–340. doi: 10.1192/bjp.bp.113.131227
Alvarez, P., and Squire, L. R. (1994). Memory consolidation and the medial temporal lobe: a simple network model. Proc. Natl. Acad. Sci. U.S.A. 91, 7041–7045. doi: 10.1073/pnas.91.15.7041
Alvarez, R. P., Biggs, A., Chen, G., Pine, D. S., and Grillon, C. (2008). Contextual fear conditioning in humans: cortical-hippocampal and amygdala contributions. J. Neurosci. 28, 6211–6219. doi: 10.1523/JNEUROSCI.1246-08.2008
American Psychiatric Association (2013). Diagnostic and Statistical Manual of Mental Disorders: DSM-5, 5th Edn. Washington, DC: Autor. doi: 10.1176/appi.books.9780890425596
Baek, J., Lee, S., Cho, T., Kim, S.-W., Kim, M., Yoon, Y., et al. (2019). Neural circuits underlying a psychotherapeutic regimen for fear disorders. Nature 566, 339–343. doi: 10.1038/s41586-019-0931-y
Baeuchl, C., Meyer, P., Hoppstädter, M., Diener, C., and Flor, H. (2015). Contextual fear conditioning in humans using feature-identical contexts. Neurobiol. Learn. Mem. 121, 1–11. doi: 10.1016/j.nlm.2015.03.001
Banducci, A. N. (2021). Prolonged exposure therapy in the time of covid-19: modifying PTSD treatment for a military sexual trauma survivor who contracted covid-19 mid-treatment. Clin. Case Stud. 20, 331–348. doi: 10.1177/1534650121993547
Bastien, R. J.-B., Jongsma, H. E., Kabadayi, M., and Billings, J. (2020). The effectiveness of psychological interventions for post-traumatic stress disorder in children, adolescents and young adults: a systematic review and meta-analysis. Psychol. Med. 50, 1598–1612. doi: 10.1017/S0033291720002007
Bear, M. F., and Kirkwood, A. (1993). Neocortical long-term potentiation. Curr. Opin. Neurobiol. 3, 197–202. doi: 10.1016/0959-4388(93)90210-P
Bear, M. F., Press, W. A., and Connors, B. W. (1992). Long-term potentiation in slices of kitten visual cortex and the effects of nmda receptor blockade. J. Neurophysiol. 67, 841–851. doi: 10.1152/jn.1992.67.4.841
Bhanji, J., Smith, D. V., and Delgado, M. (2019). A brief anatomical sketch of human ventromedial prefrontal cortex. Nat. Neurosci. 19, 1545–1552. doi: 10.31234/osf.io/zdt7f
Bienenstock, E. L., Cooper, L. N., and Munro, P. W. (1982). Theory for the development of neuron selectivity: orientation specificity and binocular interaction in visual cortex. J. Neurosci. 2, 32–48. doi: 10.1523/JNEUROSCI.02-01-00032.1982
Bisson, J. I., Berliner, L., Cloitre, M., Forbes, D., Jensen, T. K., Lewis, C., et al. (2019). The international society for traumatic stress studies new guidelines for the prevention and treatment of posttraumatic stress disorder: methodology and development process. J. Traumat. Stress 32, 475–483. doi: 10.1002/jts.22421
Bisson, J. I., Ehlers, A., Matthews, R., Pilling, S., Richards, D., and Turner, S. (2007). Psychological treatments for chronic post-traumatic stress disorder: systematic review and meta-analysis. Brit. J. Psychiatry 190, 97–104. doi: 10.1192/bjp.bp.106.021402
Blechert, J., Michael, T., Vriends, N., Margraf, J., and Wilhelm, F. H. (2007). Fear conditioning in posttraumatic stress disorder: evidence for delayed extinction of autonomic, experiential, and behavioural responses. Behav. Res. Therapy 45, 2019–2033. doi: 10.1016/j.brat.2007.02.012
Brewin, C. R. (2015). Re-experiencing traumatic events in PTSD: New avenues in research on intrusive memories and flashbacks. Eur. J. Psychotraumatol. 6, 27180. doi: 10.3402/ejpt.v6.27180
Brodt, S., Gais, S., Beck, J., Erb, M., Scheffler, K., and Schönauer, M. (2018). Fast track to the neocortex: a memory engram in the posterior parietal cortex. Science 362, 1045–1048. doi: 10.1126/science.aau2528
Brohawn, K. H., Offringa, R., Pfaff, D. L., Hughes, K. C., and Shin, L. M. (2010). The neural correlates of emotional memory in posttraumatic stress disorder. Biol. Psychiatry 68, 1023–1030. doi: 10.1016/j.biopsych.2010.07.018
Capone, C., Pastorelli, E., Golosio, B., and Paolucci, P. S. (2019). Sleep-like slow oscillations improve visual classification through synaptic homeostasis and memory association in a thalamo-cortical model. Sci. Rep. 9, 1–11. doi: 10.1038/s41598-019-45525-0
Careaga, M. B. L., Girardi, C. E. N., and Suchecki, D. (2016). Understanding posttraumatic stress disorder through fear conditioning, extinction and reconsolidation. Neurosci. Biobehav. Rev. 71, 48–57. doi: 10.1016/j.neubiorev.2016.08.023
Carletto, S., Borsato, T., and Pagani, M. (2017). The role of slow wave sleep in memory pathophysiology: focus on post-traumatic stress disorder and eye movement desensitization and reprocessing. Front. Psychol. 8, 2050. doi: 10.3389/fpsyg.2017.02050
Carrere, M., and Alexandre, F. (2015). A pavlovian model of the amygdala and its influence within the medial temporal lobe. Front. Syst. Neurosci. 9, 41. doi: 10.3389/fnsys.2015.00041
Chapman, C., Mills, K., Slade, T., McFarlane, A. C., Bryant, R., Creamer, M., et al. (2012). Remission from post-traumatic stress disorder in the general population. Psychol. Med. 42, 1695–1703. doi: 10.1017/S0033291711002856
Chauvette, S., Seigneur, J., and Timofeev, I. (2012). Sleep oscillations in the thalamocortical system induce long-term neuronal plasticity. Neuron 75, 1105–1113. doi: 10.1016/j.neuron.2012.08.034
Cho, J.-H., Deisseroth, K., and Bolshakov, V. Y. (2013). Synaptic encoding of fear extinction in MPFC-amygdala circuits. Neuron 80, 1491–1507. doi: 10.1016/j.neuron.2013.09.025
Cusack, K., Jonas, D. E., Forneris, C. A., Wines, C., Sonis, J., Middleton, J. C., et al. (2016). Psychological treatments for adults with posttraumatic stress disorder: a systematic review and meta-analysis. Clin. Psychol. Rev. 43, 128–141. doi: 10.1016/j.cpr.2015.10.003
Davis, M., Falls, W. A., and Gewirtz, J. (2000). “Neural systems involved in fear inhibition: extinction and conditioned inhibition,” in Contemporary Issues in Modeling Psychopathology (Boston, MA: Springer), 113–141. doi: 10.1007/978-1-4757-4860-4_8
Dayan, P., and Abbott, L. F. (2001). Theoretical Neuroscience: Computational and Mathematical Modeling of Neural Systems. Cambridge: MIT Press.
de Roos, C., van der Oord, S., Zijlstra, B., Lucassen, S., Perrin, S., Emmelkamp, P., et al. (2017). Comparison of eye movement desensitization and reprocessing therapy, cognitive behavioral writing therapy, and wait-list in pediatric posttraumatic stress disorder following single-incident trauma: a multicenter randomized clinical trial. J. Child Psychol. Psychiatry 58, 1219–1228. doi: 10.1111/jcpp.12768
de Voogd, L. D., Kanen, J. W., Neville, D. A., Roelofs, K., Fernández, G., and Hermans, E. J. (2018). Eye-movement intervention enhances extinction via amygdala deactivation. J. Neurosci. 38, 8694–8706. doi: 10.1523/JNEUROSCI.0703-18.2018
Diekelmann, S., and Born, J. (2010). The memory function of sleep. Nat. Rev. Neurosci. 11, 114–126. doi: 10.1038/nrn2762
Do-Monte, F. H., Manzano-Nieves, G., Qui nones-Laracuente, K., Ramos-Medina, L., and Quirk, G. J. (2015a). Revisiting the role of infralimbic cortex in fear extinction with optogenetics. J. Neurosci. 35, 3607–3615. doi: 10.1523/JNEUROSCI.3137-14.2015
Do-Monte, F. H., Quinones-Laracuente, K., and Quirk, G. J. (2015b). A temporal shift in the circuits mediating retrieval of fear memory. Nature 519, 460–463. doi: 10.1038/nature14030
Dörfel, D., Lamke, J.-P., Hummel, F., Wagner, U., Erk, S., and Walter, H. (2014). Common and differential neural networks of emotion regulation by detachment, reinterpretation, distraction, and expressive suppression: a comparative fMRI investigation. Neuroimage 101, 298–309. doi: 10.1016/j.neuroimage.2014.06.051
Ehlers, A. (2015). Understanding and treating unwanted trauma memories in posttraumatic stress disorder. Z. Psychol. 218, 141–145. doi: 10.1027/0044-3409/a000021
Eliasmith, C., and Trujillo, O. (2014). The use and abuse of large-scale brain models. Curr. Opin. Neurobiol. 25, 1–6. doi: 10.1016/j.conb.2013.09.009
Espinoza, C., Guzman, S. J., Zhang, X., and Jonas, P. (2018). Parvalbumin+ interneurons obey unique connectivity rules and establish a powerful lateral-inhibition microcircuit in dentate gyrus. Nat. Commun. 9, 1–10. doi: 10.1038/s41467-018-06899-3
Eysenck, H. J. (1982). Why do conditioned responses show incrementation, while unconditioned responses show habituation? Behav. Cogn. Psychotherapy 10, 217–220. doi: 10.1017/S0141347300007771
Feng, P., Zheng, Y., and Feng, T. (2016). Resting-state functional connectivity between amygdala and the ventromedial prefrontal cortex following fear reminder predicts fear extinction. Soc. Cogn. Affect. Neurosci. 11, 991–1001. doi: 10.1093/scan/nsw031
Foa, E. B., and Rothbaum, B. O. (2001). Treating the Trauma of Rape: Cognitive-Behavioral Therapy for PTSD. New York, NY: Guilford Press.
Fonzo, G. A., Goodkind, M. S., Oathes, D. J., Zaiko, Y. V., Harvey, M., Peng, K. K., et al. (2017). Ptsd psychotherapy outcome predicted by brain activation during emotional reactivity and regulation. Am. J. Psychiatry 174, 1163–1174. doi: 10.1176/appi.ajp.2017.16091072
Frankland, P. W., and Bontempi, B. (2005). The organization of recent and remote memories. Nat. Rev. Neurosci. 6, 119–130. doi: 10.1038/nrn1607
Fredrikson, M., and Furmark, T. (2003). Amygdaloid regional cerebral blood flow and subjective fear during symptom provocation in anxiety disorders. Ann. N. Y. Acad. Sci. 985, 341–347. doi: 10.1111/j.1749-6632.2003.tb07092.x
Fried, I., MacDonald, K. A., and Wilson, C. L. (1997). Single neuron activity in human hippocampus and amygdala during recognition of faces and objects. Neuron 18, 753–765. doi: 10.1016/S0896-6273(00)80315-3
Frye, L. A., and Spates, C. R. (2012). Prolonged exposure, mindfulness, and emotion regulation for the treatment of PTSD. Clin. Case Stud. 11, 184–200. doi: 10.1177/1534650112446850
Fullana, M., Harrison, B., Soriano-Mas, C., Vervliet, B., Cardoner, N., Ávila-Parcet, A., et al. (2016). Neural signatures of human fear conditioning: an updated and extended meta-analysis of fMRI studies. Mol. Psychiatry 21, 500–508. doi: 10.1038/mp.2015.88
Gainer, D., Alam, S., Alam, H., and Redding, H. (2020). A flash of hope: eye movement desensitization and reprocessing (EMDR) therapy. Innov. Clin. Neurosci. 17, 12–20.
Ganzel, B., Casey, B., Glover, G., Voss, H. U., and Temple, E. (2007). The aftermath of 9/11: effect of intensity and recency of trauma on outcome. Emotion 7, 227. doi: 10.1037/1528-3542.7.2.227
Garcia, R., Vouimba, R.-M., Baudry, M., and Thompson, R. F. (1999). The amygdala modulates prefrontal cortex activity relative to conditioned fear. Nature 402, 294–296. doi: 10.1038/46286
Gelbard-Sagiv, H., Mukamel, R., Harel, M., Malach, R., and Fried, I. (2008). Internally generated reactivation of single neurons in human hippocampus during free recall. Science 322, 96–101. doi: 10.1126/science.1164685
Ginty, A. T., Kraynak, T. E., Kuan, D. C., and Gianaros, P. J. (2019). Ventromedial prefrontal cortex connectivity during and after psychological stress in women. Psychophysiology 56, e13445. doi: 10.1111/psyp.13445
Golosio, B., De Luca, C., Capone, C., Pastorelli, E., Stegel, G., Tiddia, G., et al. (2021). Thalamo-cortical spiking model of incremental learning combining perception, context and nrem-sleep. PLoS Comput. Biol. 17, e1009045. doi: 10.1371/journal.pcbi.1009045
Granato, G., and Baldassarre, G. (2021). Internal manipulation of perceptual representations in human flexible cognition: a computational model. Neural Netw. 143, 572–594. doi: 10.1016/j.neunet.2021.07.013
Gyurak, A., Gross, J. J., and Etkin, A. (2011). Explicit and implicit emotion regulation: a dual-process framework. Cogn. Emot. 25, 400–412. doi: 10.1080/02699931.2010.544160
Hamann, S. B., Ely, T. D., Grafton, S. T., and Kilts, C. D. (1999). Amygdala activity related to enhanced memory for pleasant and aversive stimuli. Nat. Neurosci. 2, 289–293. doi: 10.1038/6404
Hansen, N. (2017). The longevity of hippocampus-dependent memory is orchestrated by the locus coeruleus-noradrenergic system. Neural Plasticity 2017, 2727602. doi: 10.1155/2017/2727602
Harper, M. L., Rasolkhani-Kalhorn, T., and Drozd, J. F. (2009). On the neural basis of EMDR therapy: Insights from qeeg studies. Traumatology 15, 81–95. doi: 10.1177/1534765609338498
Hassija, C. M., and Gray, M. J. (2007). Behavioral interventions for trauma and posttraumatic stress disorder. Int. J. Behav. Consult. Therapy 3, 166. doi: 10.1037/h0100797
Hattori, M. (2014). A biologically inspired dual-network memory model for reduction of catastrophic forgetting. Neurocomputing 134, 262–268. doi: 10.1016/j.neucom.2013.08.044
Helfrich, R. F., Lendner, J. D., Mander, B. A., Guillen, H., Paff, M., Mnatsakanyan, L., et al. (2019). Bidirectional prefrontal-hippocampal dynamics organize information transfer during sleep in humans. Nat. Commun. 10, 1–16. doi: 10.1038/s41467-019-11444-x
Herbert, J. D., Lilienfeld, S. O., Lohr, J. M., Montgomery, R. W. T, O'Donohue, W., et al. (2000). Science and pseudoscience in the development of eye movement desensitization and reprocessing: implications for clinical psychology. Clin. Psychol. Rev. 20, 945–971. doi: 10.1016/S0272-7358(99)00017-3
Herry, C., Ciocchi, S., Senn, V., Demmou, L., Müller, C., and Lüthi, A. (2008). Switching on and off fear by distinct neuronal circuits. Nature 454, 600–606. doi: 10.1038/nature07166
Holmes, A. (2019). Biological clues to an enigmatic treatment for traumatic stress. Nature 566, 335–346. doi: 10.1038/d41586-019-00294-8
Holmes, E. A., James, E. L., Coode-Bate, T., and Deeprose, C. (2009). Can playing the computer game “tetris” reduce the build-up of flashbacks for trauma? A proposal from cognitive science. PLoS ONE 4, e4153. doi: 10.1371/journal.pone.0004153
Huff, N. C., Frank, M., Wright-Hardesty, K., Sprunger, D., Matus-Amat, P., Higgins, E., et al. (2006). Amygdala regulation of immediate-early gene expression in the hippocampus induced by contextual fear conditioning. J. Neurosci. 26, 1616–1623. doi: 10.1523/JNEUROSCI.4964-05.2006
Huff, N. C., and Rudy, J. W. (2004). The amygdala modulates hippocampus-dependent context memory formation and stores cue-shock associations. Behav. Neurosci. 118, 53. doi: 10.1037/0735-7044.118.1.53
INSERM Collective Expertise Centre (2004). Psychotherapy: Three Approaches Evaluated. INSERM Collective Expert Reports. Institut national de la santé et de la recherche médicale.
Iyadurai, L., Blackwell, S. E., Meiser-Stedman, R., Watson, P. C., Bonsall, M. B., Geddes, J. R., et al. (2018). Preventing intrusive memories after trauma via a brief intervention involving tetris computer game play in the emergency department: a proof-of-concept randomized controlled trial. Mol. Psychiatry 23, 674–682. doi: 10.1038/mp.2017.23
Jacques, P. L. S., Botzung, A., Miles, A., and Rubin, D. C. (2011). Functional neuroimaging of emotionally intense autobiographical memories in post-traumatic stress disorder. J. Psychiatr. Res. 45, 630–637. doi: 10.1016/j.jpsychires.2010.10.011
James, E. L., Bonsall, M. B., Hoppitt, L., Tunbridge, E. M., Geddes, J. R., Milton, A. L., et al. (2015). Computer game play reduces intrusive memories of experimental trauma via reconsolidation-update mechanisms. Psychol. Sci. 26, 1201–1215. doi: 10.1177/0956797615583071
Ji, D., and Wilson, M. A. (2007). Coordinated memory replay in the visual cortex and hippocampus during sleep. Nat. Neurosci. 10, 100–107. doi: 10.1038/nn1825
Kalisch, R., Korenfeld, E., Stephan, K. E., Weiskopf, N., Seymour, B., and Dolan, R. J. (2006). Context-dependent human extinction memory is mediated by a ventromedial prefrontal and hippocampal network. J. Neurosci. 26, 9503–9511. doi: 10.1523/JNEUROSCI.2021-06.2006
Kanske, P., Heissler, J., Schönfelder, S., Bongers, A., and Wessa, M. (2011). How to regulate emotion? Neural networks for reappraisal and distraction. Cereb. Cortex 21, 1379–1388. doi: 10.1093/cercor/bhq216
Karatzias, T., Murphy, P., Cloitre, M., Bisson, J., Roberts, N., Shevlin, M., et al. (2019). Psychological interventions for ICD-11 complex PTSD symptoms: systematic review and meta-analysis. Psychol. Med. 49, 1761–1775. doi: 10.1017/S0033291719000436
Kasai, K., Yamasue, H., Gilbertson, M. W., Shenton, M. E., Rauch, S. L., and Pitman, R. K. (2008). Evidence for acquired pregenual anterior cingulate gray matter loss from a twin study of combat-related posttraumatic stress disorder. Biol. Psychiatry 63, 550–556. doi: 10.1016/j.biopsych.2007.06.022
Khan, A. M., Dar, S., Ahmed, R., Bachu, R., Adnan, M., and Kotapati, V. P. (2018). Cognitive behavioral therapy versus eye movement desensitization and reprocessing in patients with post-traumatic stress disorder: systematic review and meta-analysis of randomized clinical trials. Cureus 10, e3250. doi: 10.7759/cureus.3250
Kilpatrick, L., and Cahill, L. (2003). Amygdala modulation of parahippocampal and frontal regions during emotionally influenced memory storage. Neuroimage 20, 2091–2099. doi: 10.1016/j.neuroimage.2003.08.006
Kim, J., Pignatelli, M., Xu, S., Itohara, S., and Tonegawa, S. (2016). Antagonistic negative and positive neurons of the basolateral amygdala. Nat. Neurosci. 19, 1636–1646. doi: 10.1038/nn.4414
Kim, J. J., and Fanselow, M. S. (1992). Modality-specific retrograde amnesia of fear. Science 256, 675–677. doi: 10.1126/science.1585183
Kim, W. B., and Cho, J.-H. (2017). Synaptic targeting of double-projecting ventral ca1 hippocampal neurons to the medial prefrontal cortex and basal amygdala. J. Neurosci. 37, 4868–4882. doi: 10.1523/JNEUROSCI.3579-16.2017
Kim, W. B., and Cho, J.-H. (2020). Encoding of contextual fear memory in hippocampal-amygdala circuit. Nat. Commun. 11, 1–22. doi: 10.1038/s41467-020-15121-2
King, A. P., Block, S. R., Sripada, R. K., Rauch, S. A., Porter, K. E., Favorite, T. K., et al. (2016). A pilot study of mindfulness-based exposure therapy in OEF/OIF combat veterans with PTSD: altered medial frontal cortex and amygdala responses in social-emotional processing. Front. Psychiatry 7:154. doi: 10.3389/fpsyt.2016.00154
Kitamura, T., Ogawa, S. K., Roy, D. S., Okuyama, T., Morrissey, M. D., Smith, L. M., et al. (2017). Engrams and circuits crucial for systems consolidation of a memory. Science 356, 73–78. doi: 10.1126/science.aam6808
Kredlow, M., Fenster, R. J., Laurent, E. S., Ressler, K. J., and Phelps, E. A. (2022). Prefrontal cortex, amygdala, and threat processing: implications for PTSD. Neuropsychopharmacology 47, 247–259. doi: 10.1038/s41386-021-01155-7
Kumaran, D., Hassabis, D., and McClelland, J. L. (2016). What learning systems do intelligent agents need? Complementary learning systems theory updated. Trends Cogn. Sci. 20, 512–534. doi: 10.1016/j.tics.2016.05.004
Landin-Romero, R., Moreno-Alcazar, A., Pagani, M., and Amann, B. L. (2018). How does eye movement desensitization and reprocessing therapy work? A systematic review on suggested mechanisms of action. Front. Psychol. 9, 1395. doi: 10.3389/fpsyg.2018.01395
Lang, S., Kroll, A., Lipinski, S. J., Wessa, M., Ridder, S., Christmann, C., et al. (2009). Context conditioning and extinction in humans: differential contribution of the hippocampus, amygdala and prefrontal cortex. Eur. J. Neurosci. 29, 823–832. doi: 10.1111/j.1460-9568.2009.06624.x
Lee, C. W., Taylor, G., and Drummond, P. D. (2006). The active ingredient in EMDR: is it traditional exposure or dual focus of attention? Clin. Psychol. Psychother. 13, 97–107. doi: 10.1002/cpp.479
Lewey, J. H., Smith, C. L., Burcham, B., Saunders, N. L., Elfallal, D., and O'Toole, S. K. (2018). Comparing the effectiveness of EMDR and tf-cbt for children and adolescents: a meta-analysis. J. Child Adolesc. Trauma 11, 457–472. doi: 10.1007/s40653-018-0212-1
Li, Y., Hou, X., Wei, D., Du, X., Zhang, Q., Liu, G., et al. (2017). Long-term effects of acute stress on the prefrontal-limbic system in the healthy adult. PLoS ONE 12, e0168315. doi: 10.1371/journal.pone.0168315
Liberzon, I., and Sripada, C. S. (2007). The functional neuroanatomy of PTSD: a critical review. Prog. Brain Res. 167, 151–169. doi: 10.1016/S0079-6123(07)67011-3
Liberzon, I., Taylor, S. F., Amdur, R., Jung, T. D., Chamberlain, K. R., Minoshima, S., et al. (1999). Brain activation in PTSD in response to trauma-related stimuli. Biol. Psychiatry 45, 817–826. doi: 10.1016/S0006-3223(98)00246-7
Logothetis, N. K. (2008). What we can do and what we cannot do with fMRI. Nature 453, 869–878. doi: 10.1038/nature06976
Lohr, J. M., Tolin, D. F., and Lilienfeld, S. O. (1998). Efficacy of eye movement desensitization and reprocessing: implications for behavior therapy. Behav. Therapy 29, 123–156. doi: 10.1016/S0005-7894(98)80035-X
Lutas, A., Kucukdereli, H., Alturkistani, O., Carty, C., Sugden, A. U., Fernando, K., et al. (2019). State-specific gating of salient cues by midbrain dopaminergic input to basal amygdala. Nat. Neurosci. 22, 1820–1833. doi: 10.1038/s41593-019-0506-0
Mahan, A. L., and Ressler, K. J. (2012). Fear conditioning, synaptic plasticity and the amygdala: implications for posttraumatic stress disorder. Trends Neurosci. 35, 24–35. doi: 10.1016/j.tins.2011.06.007
Mannella, F., Mirolli, M., and Baldassarre, G. (2016). Goal-directed behavior and instrumental devaluation: a neural system-level computational model. Front. Behav. Neurosci. 10, 181. doi: 10.3389/fnbeh.2016.00181
Marschner, A., Kalisch, R., Vervliet, B., Vansteenwegen, D., and Büchel, C. (2008). Dissociable roles for the hippocampus and the amygdala in human cued versus context fear conditioning. J. Neurosci. 28, 9030–9036. doi: 10.1523/JNEUROSCI.1651-08.2008
Mattera, A., Pagani, M., and Baldassarre, G. (2020). A computational model integrating multiple phenomena on cued fear conditioning, extinction, and reinstatement. Front. Syst. Neurosci. 14, 65. doi: 10.3389/fnsys.2020.569108
Mavranezouli, I., Megnin-Viggars, O., Daly, C., Dias, S., Welton, N. J., Stockton, S., et al. (2020). Psychological treatments for post-traumatic stress disorder in adults: a network meta-analysis. Psychol. Med. 50, 542–555. doi: 10.1017/S0033291720000070
McClelland, J. L., McNaughton, B. L., and O'Reilly, R. C. (1995). Why there are complementary learning systems in the hippocampus and neocortex: insights from the successes and failures of connectionist models of learning and memory. Psychol. Rev. 102, 419. doi: 10.1037/0033-295X.102.3.419
McLaughlin, K. A., Busso, D. S., Duys, A., Green, J. G., Alves, S., Way, M., et al. (2014). Amygdala response to negative stimuli predicts PTSD symptom onset following a terrorist attack. Depression Anxiety 31, 834–842. doi: 10.1002/da.22284
McRae, K., Hughes, B., Chopra, S., Gabrieli, J. D., Gross, J. J., and Ochsner, K. N. (2010). The neural bases of distraction and reappraisal. J. Cogn. Neurosci. 22, 248–262. doi: 10.1162/jocn.2009.21243
Milad, M. R., Orr, S. P., Lasko, N. B., Chang, Y., Rauch, S. L., and Pitman, R. K. (2008). Presence and acquired origin of reduced recall for fear extinction in PTSD: results of a twin study. J. Psychiatr. Res. 42, 515–520. doi: 10.1016/j.jpsychires.2008.01.017
Milad, M. R., Pitman, R. K., Ellis, C. B., Gold, A. L., Shin, L. M., Lasko, N. B., et al. (2009). Neurobiological basis of failure to recall extinction memory in posttraumatic stress disorder. Biol. Psychiatry 66, 1075–1082. doi: 10.1016/j.biopsych.2009.06.026
Milad, M. R., and Quirk, G. J. (2002). Neurons in medial prefrontal cortex signal memory for fear extinction. Nature 420, 70–74. doi: 10.1038/nature01138
Miller, D. R., Hayes, S. M., Hayes, J. P., Spielberg, J. M., Lafleche, G., and Verfaellie, M. (2017). Default mode network subsystems are differentially disrupted in posttraumatic stress disorder. Biol. Psychiatry Cogn. Neurosci. Neuroimaging 2, 363–371. doi: 10.1016/j.bpsc.2016.12.006
Miyamoto, D., Hirai, D., Fung, C., Inutsuka, A., Odagawa, M., Suzuki, T., et al. (2016). Top-down cortical input during nrem sleep consolidates perceptual memory. Science 352, 1315–1318. doi: 10.1126/science.aaf0902
Miyamoto, D., Hirai, D., and Murayama, M. (2017). The roles of cortical slow waves in synaptic plasticity and memory consolidation. Front. Neural Circ. 11, 92. doi: 10.3389/fncir.2017.00092
Mölle, M., and Born, J. (2009). Hippocampus whispering in deep sleep to prefrontal cortex–for good memories? Neuron 61, 496–498. doi: 10.1016/j.neuron.2009.02.002
Morgan, L., Scourfield, J., Williams, D., Jasper, A., and Lewis, G. (2003). The aberfan disaster: 33-year follow-up of survivors. Brit. J. Psychiatry 182, 532–536. doi: 10.1192/bjp.182.6.532
Moustafa, A. A., Gilbertson, M. W., Orr, S. P., Herzallah, M. M., Servatius, R. J., and Myers, C. E. (2013). A model of amygdala-hippocampal-prefrontal interaction in fear conditioning and extinction in animals. Brain Cogn. 81, 29–43. doi: 10.1016/j.bandc.2012.10.005
Murty, V. P., Ritchey, M., Adcock, R. A., and LaBar, K. S. (2011). Reprint of: fMRI studies of successful emotional memory encoding: a quantitative meta-analysis. Neuropsychologia 49, 695–705. doi: 10.1016/j.neuropsychologia.2011.02.031
Musazzi, L., Tornese, P., Sala, N., and Popoli, M. (2018). What acute stress protocols can tell us about PTSD and stress-related neuropsychiatric disorders. Front. Pharmacol. 9, 758. doi: 10.3389/fphar.2018.00758
Nair, S. S., Paré, D., and Vicentic, A. (2016). Biologically based neural circuit modelling for the study of fear learning and extinction. NPJ Sci. Learn. 1, 1–7. doi: 10.1038/npjscilearn.2016.15
Navarro, P. N., Landin-Romero, R., Guardiola-Wanden-Berghe, R., Moreno-Alcázar, A., Valiente-Gómez, A., Lupo, W., et al. (2018). 25 years of eye movement desensitization and reprocessing (EMDR): the EMDR therapy protocol, hypotheses of its mechanism of action and a systematic review of its efficacy in the treatment of post-traumatic stress disorder. Revista de Psiquiatria y Salud Mental 11, 101–114. doi: 10.1016/j.rpsmen.2015.12.002
Neumeister, P., Feldker, K., Heitmann, C. Y., Helmich, R., Gathmann, B., Becker, M. P., et al. (2017). Interpersonal violence in posttraumatic women: brain networks triggered by trauma-related pictures. Soc. Cogn. Affect. Neurosci. 12, 555–568. doi: 10.1093/scan/nsw165
Nicholaichuk, T. P., Quesnel, L. J., and Tait, R. W. (1982). Eysenck's theory of incubation: an empirical test. Behav. Res. Therapy 20, 329–338. doi: 10.1016/0005-7967(82)90092-4
Nijdam, M. J., Gersons, B. P., Reitsma, J. B., de Jongh, A., and Olff, M. (2012). Brief eclectic psychotherapy v. eye movement desensitisation and reprocessing therapy for post-traumatic stress disorder: randomised controlled trial. Brit. J. Psychiatry 200, 224–231. doi: 10.1192/bjp.bp.111.099234
Nord, C. L., Gray, A., Charpentier, C. J., Robinson, O. J., and Roiser, J. P. (2017). Unreliability of putative fMRI biomarkers during emotional face processing. Neuroimage 156, 119–127. doi: 10.1016/j.neuroimage.2017.05.024
Norman, K. A., and O'Reilly, R. C. (2003). Modeling hippocampal and neocortical contributions to recognition memory: a complementary-learning-systems approach. Psychol. Rev. 110, 611. doi: 10.1037/0033-295X.110.4.611
Öngür, D., and Price, J. L. (2000). The organization of networks within the orbital and medial prefrontal cortex of rats, monkeys and humans. Cereb. Cortex 10, 206–219. doi: 10.1093/cercor/10.3.206
Orr, S. P., Metzger, L. J., Lasko, N. B., Macklin, M. L., Peri, T., and Pitman, R. K. (2000). De novo conditioning in trauma-exposed individuals with and without posttraumatic stress disorder. J. Abnormal Psychol. 109, 290. doi: 10.1037/0021-843X.109.2.290
Orr, S. P., Pitman, R. K., Lasko, N. B., and Herz, L. R. (1993). Psychophysiological assessment of posttraumatic stress disorder imagery in World War II and Korean combat veterans. J. Abnormal Psychol. 102, 152. doi: 10.1037/0021-843X.102.1.152
Pagani, M., Amann, B. L., Landin-Romero, R., and Carletto, S. (2017). Eye movement desensitization and reprocessing and slow wave sleep: a putative mechanism of action. Front. Psychol. 8, 1935. doi: 10.3389/fpsyg.2017.01935
Pagani, M., Di Lorenzo, G., Monaco, L., Niolu, C., Siracusano, A., Verardo, A. R., et al. (2011). Pretreatment, intratreatment, and posttreatment eeg imaging of EMDR: methodology and preliminary results from a single case. J. EMDR Pract. Res. 5, 42–56. doi: 10.1891/1933-3196.5.2.42
Pagani, M., Di Lorenzo, G., Verardo, A. R., Nicolais, G., Monaco, L., Lauretti, G., et al. (2012). Neurobiological correlates of EMDR monitoring-an EEG study. PLoS ONE 7, e45753. doi: 10.1371/journal.pone.0045753
Peres, J. F., Foerster, B., Santana, L. G., Fereira, M. D., Nasello, A. G., Savoia, M., et al. (2011). Police officers under attack: resilience implications of an fMRI study. J. Psychiatr. Res. 45, 727–734. doi: 10.1016/j.jpsychires.2010.11.004
Peyrache, A., Khamassi, M., Benchenane, K., Wiener, S. I., and Battaglia, F. P. (2009). Replay of rule-learning related neural patterns in the prefrontal cortex during sleep. Nat. Neurosci. 12, 919–926. doi: 10.1038/nn.2337
Phelps, E. A., Delgado, M. R., Nearing, K. I., and LeDoux, J. E. (2004). Extinction learning in humans: role of the amygdala and VMPFC. Neuron 43, 897–905. doi: 10.1016/j.neuron.2004.08.042
Phillips, R., and LeDoux, J. (1992). Differential contribution of amygdala and hippocampus to cued and contextual fear conditioning. Behav. Neurosci. 106, 274. doi: 10.1037/0735-7044.106.2.274
Pissiota, A., Frans, Ö., Fernandez, M., von Knorring, L., Fischer, H., and Fredrikson, M. (2002). Neurofunctional correlates of posttraumatic stress disorder: a pet symptom provocation study. Eur. Arch. Psychiatry Clin. Neurosci. 252, 68–75. doi: 10.1007/s004060200014
Pitkänen, A., Pikkarainen, M., Nurminen, N., and Ylinen, A. (2000). Reciprocal connections between the amygdala and the hippocampal formation, perirhinal cortex, and postrhinal cortex in rat: a review. Ann. N. Y. Acad. Sci. 911, 369–391. doi: 10.1111/j.1749-6632.2000.tb06738.x
Power, K., McGoldrick, T., Brown, K., Buchanan, R., Sharp, D., Swanson, V., et al. (2002). A controlled comparison of eye movement desensitization and reprocessing versus exposure plus cognitive restructuring versus waiting list in the treatment of post-traumatic stress disorder. Clinical Psychol. Psychother. 9, 299–318. doi: 10.1002/cpp.341
Protopopescu, X., Pan, H., Tuescher, O., Cloitre, M., Goldstein, M., Engelien, W., et al. (2005). Differential time courses and specificity of amygdala activity in posttraumatic stress disorder subjects and normal control subjects. Biol. Psychiatry 57, 464–473. doi: 10.1016/j.biopsych.2004.12.026
Proudlock, S., and Peris, J. (2020). Using EMDR therapy with patients in an acute mental health crisis. BMC Psychiatry 20, 14. doi: 10.1186/s12888-019-2426-7
Qin, S., Hermans, E. J., Van Marle, H. J., Luo, J., and Fernández, G. (2009). Acute psychological stress reduces working memory-related activity in the dorsolateral prefrontal cortex. Biol. Psychiatry 66, 25–32. doi: 10.1016/j.biopsych.2009.03.006
Quinn, J. J., Ma, Q. D., Tinsley, M. R., Koch, C., and Fanselow, M. S. (2008). Inverse temporal contributions of the dorsal hippocampus and medial prefrontal cortex to the expression of long-term fear memories. Learn. Mem. 15, 368–372. doi: 10.1101/lm.813608
Quiroga, R. Q., Kraskov, A., Koch, C., and Fried, I. (2009). Explicit encoding of multimodal percepts by single neurons in the human brain. Curr. Biol. 19, 1308–1313. doi: 10.1016/j.cub.2009.06.060
Radell, M. L., Myers, C. E., Sheynin, J., and Moustafa, A. A. (2017). Computational models of posttraumatic stress disorder (PTSD). Comput. Models Brain Behav. 43, 43–55. doi: 10.1002/9781119159193.ch4
Rauch, S. L., Shin, L. M., and Phelps, E. A. (2006). Neurocircuitry models of posttraumatic stress disorder and extinction: human neuroimaging research—past, present, and future. Biol. Psychiatry 60, 376–382. doi: 10.1016/j.biopsych.2006.06.004
Rauch, S. L., Whalen, P. J., Shin, L. M., McInerney, S. C., Macklin, M. L., Lasko, N. B., et al. (2000). Exaggerated amygdala response to masked facial stimuli in posttraumatic stress disorder: a functional mri study. Biol. Psychiatry 47, 769–776. doi: 10.1016/S0006-3223(00)00828-3
Rogers, S., and Silver, S. M. (2002). Is EMDR an exposure therapy? A review of trauma protocols. J. Clin. Psychol. 58, 43–59. doi: 10.1002/jclp.1128
Rolls, E. (2013). The mechanisms for pattern completion and pattern separation in the hippocampus. Front. Syst. Neurosci. 7, 74. doi: 10.3389/fnsys.2013.00074
Rolls, E. T. (2007). An attractor network in the hippocampus: theory and neurophysiology. Learn. Mem. 14, 714–731. doi: 10.1101/lm.631207
Rolls, E. T., and Xiang, J.-Z. (2006). Spatial view cells in the primate hippocampus and memory recall. Rev. Neurosci. 17, 175–200. doi: 10.1515/REVNEURO.2006.17.1-2.175
Roozendaal, B., McEwen, B. S., and Chattarji, S. (2009). Stress, memory and the amygdala. Nat. Rev. Neurosci. 10, 423–433. doi: 10.1038/nrn2651
Rothbaum, B. O., and Schwartz, A. C. (2002). Exposure therapy for posttraumatic stress disorder. Am. J. Psychother. 56, 59–75. doi: 10.1176/appi.psychotherapy.2002.56.1.59
Rougemont-Bücking, A., Linnman, C., Zeffiro, T. A., Zeidan, M. A., Lebron-Milad, K., Rodriguez-Romaguera, J., et al. (2011). Altered processing of contextual information during fear extinction in PTSD: an fMRI study. CNS Neurosci. Therapeut. 17, 227–236. doi: 10.1111/j.1755-5949.2010.00152.x
Sacco, T., and Sacchetti, B. (2010). Role of secondary sensory cortices in emotional memory storage and retrieval in rats. Science 329, 649–656. doi: 10.1126/science.1183165
Sandin, B., and Chorot, P. (2002). Resistance to extinction of conditioned electrodermal responses: a study of the incubation fear hypothesis. Psychol. Rep. 91, 37–46. doi: 10.2466/pr0.2002.91.1.37
Sandler, M., Shulman, Y., and Schiller, J. (2016). A novel form of local plasticity in tuft dendrites of neocortical somatosensory layer 5 pyramidal neurons. Neuron 90, 1028–1042. doi: 10.1016/j.neuron.2016.04.032
Schiller, D., Levy, I., Niv, Y., LeDoux, J. E., and Phelps, E. A. (2008). From fear to safety and back: reversal of fear in the human brain. J. Neurosci. 28, 11517–11525. doi: 10.1523/JNEUROSCI.2265-08.2008
Schnyder, U., Ehlers, A., Elbert, T., Foa, E. B., Gersons, B. P., Resick, P. A., et al. (2015). Psychotherapies for PTSD: what do they have in common? Eur. J. Psychotraumatol. 6, 28186. doi: 10.3402/ejpt.v6.28186
Schweizer, S., Grahn, J., Hampshire, A., Mobbs, D., and Dalgleish, T. (2013). Training the emotional brain: improving affective control through emotional working memory training. J. Neurosci. 33, 5301–5311. doi: 10.1523/JNEUROSCI.2593-12.2013
Schwindel, C. D., and McNaughton, B. L. (2011). Hippocampal-cortical interactions and the dynamics of memory trace reactivation. Prog. Brain Res. 193, 163–177. doi: 10.1016/B978-0-444-53839-0.00011-9
Senn, V., Wolff, S. B., Herry, C., Grenier, F., Ehrlich, I., Gründemann, J., et al. (2014). Long-range connectivity defines behavioral specificity of amygdala neurons. Neuron 81, 428–437. doi: 10.1016/j.neuron.2013.11.006
Shapiro, F. (1995). Eye Movement Desensitization and Reprocessing (EMDR) Therapy: Basic Principles, Protocols, and Procedures. New York, NY: Guilford Publications.
Shapiro, F. (2014). The role of eye movement desensitization and reprocessing (EMDR) therapy in medicine: addressing the psychological and physical symptoms stemming from adverse life experiences. Permanente J. 18, 71. doi: 10.7812/TPP/13-098
Shen, B., and McNaughton, B. L. (1996). Modeling the spontaneous reactivation of experience-specific hippocampal cell assembles during sleep. Hippocampus 6, 685–692. doi: 10.1002/(SICI)1098-1063(1996)6:6<685::AID-HIPO11>3.0.CO;2-X
Shin, L. M., and Liberzon, I. (2010). The neurocircuitry of fear, stress, and anxiety disorders. Neuropsychopharmacology 35, 169–191. doi: 10.1038/npp.2009.83
Shin, L. M., Orr, S. P., Carson, M. A., Rauch, S. L., Macklin, M. L., Lasko, N. B., et al. (2004). Regional cerebral blood flow in the amygdala and medial prefrontalcortex during traumatic imagery in male and female vietnam veterans with PTSD. Arch. Gen. Psychiatry 61, 168–176. doi: 10.1001/archpsyc.61.2.168
Sirota, A., Csicsvari, J., Buhl, D., and Buzsáki, G. (2003). Communication between neocortex and hippocampus during sleep in rodents. Proc. Natl. Acad. Sci. U.S.A. 100, 2065–2069. doi: 10.1073/pnas.0437938100
Solomon, R. M., and Shapiro, F. (2008). EMDR and the adaptive information processing modelpotential mechanisms of change. J. EMDR Pract. Res. 2, 315–325. doi: 10.1891/1933-3196.2.4.315
Squire, L. R., Genzel, L., Wixted, J. T., and Morris, R. G. (2015). Memory consolidation. Cold Spring Harbor Perspect. Biol. 7, a021766. doi: 10.1101/cshperspect.a021766
Stevens, J. S., Kim, Y. J., Galatzer-Levy, I. R., Reddy, R., Ely, T. D., Nemeroff, C. B., et al. (2017). Amygdala reactivity and anterior cingulate habituation predict PTSD symptom maintenance after acute civilian trauma. Biol. Psychiatry 81, 1023. doi: 10.1016/j.biopsych.2016.11.015
Stickgold, R. (2002). EMDR: a putative neurobiological mechanism of action. J. Clin. Psychol. 58, 61–75. doi: 10.1002/jclp.1129
Stojek, M. M., McSweeney, L. B., and Rauch, S. A. (2018). Neuroscience informed prolonged exposure practice: increasing efficiency and efficacy through mechanisms. Front. Behav. Neurosci. 12, 281. doi: 10.3389/fnbeh.2018.00281
Sun, Y., Wang, Z., Ding, W., Wan, J., Zhuang, Z., Zhang, Y., et al. (2013). Alterations in white matter microstructure as vulnerability factors and acquired signs of traffic accident-induced PTSD. PLoS ONE 8, e83473. doi: 10.1371/journal.pone.0083473
Tanaka, K. Z., Pevzner, A., Hamidi, A. B., Nakazawa, Y., Graham, J., and Wiltgen, B. J. (2014). Cortical representations are reinstated by the hippocampus during memory retrieval. Neuron 84, 347–354. doi: 10.1016/j.neuron.2014.09.037
Tashjian, S. M., Zbozinek, T. D., and Mobbs, D. (2021). A decision architecture for safety computations. Trends Cogn. Sci. 25, 342–354. doi: 10.1016/j.tics.2021.01.013
Thomaes, K., Dorrepaal, E., Draijer, N., Jansma, E. P., Veltman, D. J., and van Balkom, A. J. (2014). Can pharmacological and psychological treatment change brain structure and function in PTSD? A systematic review. J. Psychiatr. Res. 50, 1–15. doi: 10.1016/j.jpsychires.2013.11.002
Tryon, W. W. (1998). A neural network explanation of posttraumatic stress disorder. J. Anxiety Disord. 12, 373–385. doi: 10.1016/S0887-6185(98)00021-8
Tse, D., Langston, R. F., Kakeyama, M., Bethus, I., Spooner, P. A., Wood, E. R., et al. (2007). Schemas and memory consolidation. Science 316, 76–82. doi: 10.1126/science.1135935
Tse, D., Takeuchi, T., Kakeyama, M., Kajii, Y., Okuno, H., Tohyama, C., et al. (2011). Schema-dependent gene activation and memory encoding in neocortex. Science 333, 891–895. doi: 10.1126/science.1205274
Ursano, R. J., Bell, C., Eth, S., Friedman, M., Norwood, A., Pfefferbaum, B., et al. (2004). Practice guideline for the treatment of patients with acute stress disorder and posttraumatic stress disorder. Am. J. Psychiatry 161(11 Suppl):3–31.
van Heukelum, S., Mars, R. B., Guthrie, M., Buitelaar, J. K., Beckmann, C. F., Tiesinga, P. H., et al. (2020). Where is cingulate cortex? A cross-species view. Trends Neurosci. 43, 285–299. doi: 10.1016/j.tins.2020.03.007
Verbitsky, A., Dopfel, D., and Zhang, N. (2020). Rodent models of post-traumatic stress disorder: behavioral assessment. Transl. Psychiatry 10, 1–28. doi: 10.1038/s41398-020-0806-x
Via, E., Fullana, M., Goldberg, X., Tinoco-González, D., Martinez-Zalacain, I., Soriano-Mas, C., et al. (2018). Ventromedial prefrontal cortex activity and pathological worry in generalised anxiety disorder. Brit. J. Psychiatry 213, 437–443. doi: 10.1192/bjp.2018.65
Wei, Y., Krishnan, G. P., and Bazhenov, M. (2016). Synaptic mechanisms of memory consolidation during sleep slow oscillations. J. Neurosci. 36, 4231–4247. doi: 10.1523/JNEUROSCI.3648-15.2016
Wheeler, M. E., Petersen, S. E., and Buckner, R. L. (2000). Memory's echo: vivid remembering reactivates sensory-specific cortex. Proc. Natl. Acad. Sci. U.S.A. 97, 11125–11129. doi: 10.1073/pnas.97.20.11125
Wilson, G., Farrell, D., Barron, I., Hutchins, J., Whybrow, D., and Kiernan, M. D. (2018). The use of eye-movement desensitization reprocessing (EMDR) therapy in treating post-traumatic stress disorder–a systematic narrative review. Front. Psychol. 9, 923. doi: 10.3389/fpsyg.2018.00923
World Health Organization (2013). Guidelines for the Management of Conditions That Are Specifically Related to Stress. Geneva: World Health Organization.
Yang, Y., and Wang, J.-Z. (2017). From structure to behavior in basolateral amygdala-hippocampus circuits. Front. Neural Circ. 11, 86. doi: 10.3389/fncir.2017.00086
Yang, Y., Wang, Z.-H., Jin, S., Gao, D., Liu, N., Chen, S.-P., et al. (2016). Opposite monosynaptic scaling of BLP-VCA1 inputs governs hopefulness-and helplessness-modulated spatial learning and memory. Nat. Commun. 7, 1–14. doi: 10.1038/ncomms11935
Yehuda, R. (1999). Biological factors associated with susceptibility to posttraumatic stress disorder. Can. J. Psychiatry 44, 34–39. doi: 10.1177/070674379904400104
Yoshii, T. (2021). The role of the thalamus in post-traumatic stress disorder. Int. J. Mol. Sci. 22, 1730. doi: 10.3390/ijms22041730
Zeidan, F., Martucci, K. T., Kraft, R. A., McHaffie, J. G., and Coghill, R. C. (2014). Neural correlates of mindfulness meditation-related anxiety relief. Soc. Cogn. Affect. Neurosci. 9, 751–759. doi: 10.1093/scan/nst041
Zhang, X., and Li, B. (2018). Population coding of valence in the basolateral amygdala. Nat. Commun. 9, 1–14. doi: 10.1038/s41467-018-07679-9
Keywords: post-traumatic stress disorder, eye movement desensitization and reprocessing therapy, prolonged exposure, computational modeling, amygdala
Citation: Mattera A, Cavallo A, Granato G, Baldassarre G and Pagani M (2022) A Biologically Inspired Neural Network Model to Gain Insight Into the Mechanisms of Post-Traumatic Stress Disorder and Eye Movement Desensitization and Reprocessing Therapy. Front. Psychol. 13:944838. doi: 10.3389/fpsyg.2022.944838
Received: 15 May 2022; Accepted: 06 June 2022;
Published: 13 July 2022.
Edited by:
Antonio Onofri, Azienda Sanitaria Locale Roma 1, ItalyReviewed by:
Sophie Iuel-Brockdorff, EMDR Klinikken Denmark, DenmarkCopyright © 2022 Mattera, Cavallo, Granato, Baldassarre and Pagani. This is an open-access article distributed under the terms of the Creative Commons Attribution License (CC BY). The use, distribution or reproduction in other forums is permitted, provided the original author(s) and the copyright owner(s) are credited and that the original publication in this journal is cited, in accordance with accepted academic practice. No use, distribution or reproduction is permitted which does not comply with these terms.
*Correspondence: Andrea Mattera, YW5kcmVhLm1hdHRlcmFAaXN0Yy5jbnIuaXQ=
†These authors have contributed equally to this work and share last authorship
Disclaimer: All claims expressed in this article are solely those of the authors and do not necessarily represent those of their affiliated organizations, or those of the publisher, the editors and the reviewers. Any product that may be evaluated in this article or claim that may be made by its manufacturer is not guaranteed or endorsed by the publisher.
Research integrity at Frontiers
Learn more about the work of our research integrity team to safeguard the quality of each article we publish.