- Department of Psychology, Wilfrid Laurier University, Waterloo, ON, Canada
The hippocampal formation (HF) is a structure critical to navigation and many forms of memory. In mammals, the firing of place cells is widely regarded as the fundamental unit of HF information processing. Supporting homology between the avian and mammalian HF, context-specific patterns of Egr1 have been reported in birds that are comparable to those produced by place cell firing in mammals. Recent electrophysiological data, however, suggest that many avian species lack place cells, potentially undermining the correspondence between Egr1 and place cell-related firing in the avian brain. To clarify this, the current study examines Egr1 expression in Japanese quail under conditions known to elicit only weakly spatially modulated firing patterns and report robust context-dependent Egr1 expression. These data confirm that context-dependent expression of Egr1 is not dependent on precise place fields and provide insight into how these birds are able to perform complex spatial tasks despite lacking mammalian-like place cells.
Introduction
The hippocampal formation (HF) is a structure critical to many forms of memory and spatial navigation in numerous species, including birds. For instance, the HF is required for learning maps of familiar landmarks (Gagliardo et al., 2001; White et al., 2002), locating baited food in a maze (e.g., Hough and Bingman, 2004; Ruploh et al., 2011; Damphousse et al., 2022), and the recovery of hidden food items (Sherry and Vaccarino, 1989).
One of the most striking features of mammalian HF is the ability for its cells to fire in a manner that reports an animal’s location in space (O’Keefe and Dostrovsky, 1971). Further observations have shown that these place cells respond to much more than merely spatial location—factors, such as task demands or internal state influence the firing of these cells (e.g., Markus et al., 1995; Skaggs and McNaughton, 1998; Wood et al., 2000; Kennedy and Shapiro, 2009), suggesting that place cell-like firing patterns form the fundamental units for the HF to record experience (reviewed by Eichenbaum, 2017; Epstein et al., 2017; Bellmund et al., 2018). Importantly, single-unit recordings have revealed some degree of spatially tuned firing patterns in a wide variety of animals, including several avian species (e.g., Hough and Bingman, 2004; Siegel et al., 2005; Agarwal et al., 2021; Ben-Yishay et al., 2021; Payne et al., 2021), suggesting that there may be widespread homology in this form of information processing across different classes of animal. Characterizing the homology of spatial information processing across the animal kingdom will require increasing the variety of animals in which place cell-like activity can be either confirmed or refuted.
Investigating the homology of spatial information processing may be aided by expanding the techniques available for assessing place cell-like activity. In mammals, the dynamics of place cell-related firing can also be examined through the expression of immediate-early genes, such as Egr1, which is tightly coupled to neuronal activity, a critical mediator of plasticity (Bozon et al., 2003), and reliably reports place cell activity (Marrone et al., 2011). Context-specific Egr1 expression has been reported in hippocampal area DM in the brown-headed cowbird (Grella et al., 2016), suggesting place cell-like activity in these birds. Unfortunately, the spatial-tuning of cells in this species remains uncharacterized. This is problematic in that recent data show that both the abundance and location of spatially tuned cells in birds can vary substantially across species (Payne et al., 2021) and tasks (Kahn et al., 2008). Generating comparable data using both a species and behavioral conditions where the firing patterns are known will permit stronger conclusions to be made regarding the coupling between firing patterns and gene expression in the avian HF.
To accomplish this, the current experiment examines Japanese quail as they freely explore an open field, mimicking the conditions under which quail HF cells are weakly spatially coupled and are responsive largely to head direction (Ben-Yishay et al., 2021) in order to determine the extent to which these firing patterns can drive context-specific Egr1 expression.
Materials and Methods
Subjects
Twenty-four Japanese quail (Spring Creek Quail Farms, St Ann’s, ON) were used in this experiment. All birds were group housed on a 12:12 h light cycle with ad lib access to food and water. Prior to behavioral testing, all birds were handled 15 min/day for at least 7 days. All procedures were approved by the animal care committee of Wilfrid Laurier University and were carried out in accordance with the guidelines of the Canadian Council on Animal Care.
Behavioral Procedures
A modified version of the procedures outlined by Marrone et al. (2011) was used here (Figure 1). Briefly, two groups of quail were taken to a room containing several local and distal landmarks and passively moved through a 1 m2 open field. That is, the environment was divided into nine equally sized grids and the quail were moved to a new grid every 30 s for 5 min. Quail were then returned to their housing room. After a 25 min delay, quail were passively moved through either the same environment again (same, n = 8) or another open field in a second room containing distinct local and distal cues (different, n = 8). A third control group remained in the colony room undisturbed (caged, n = 8).
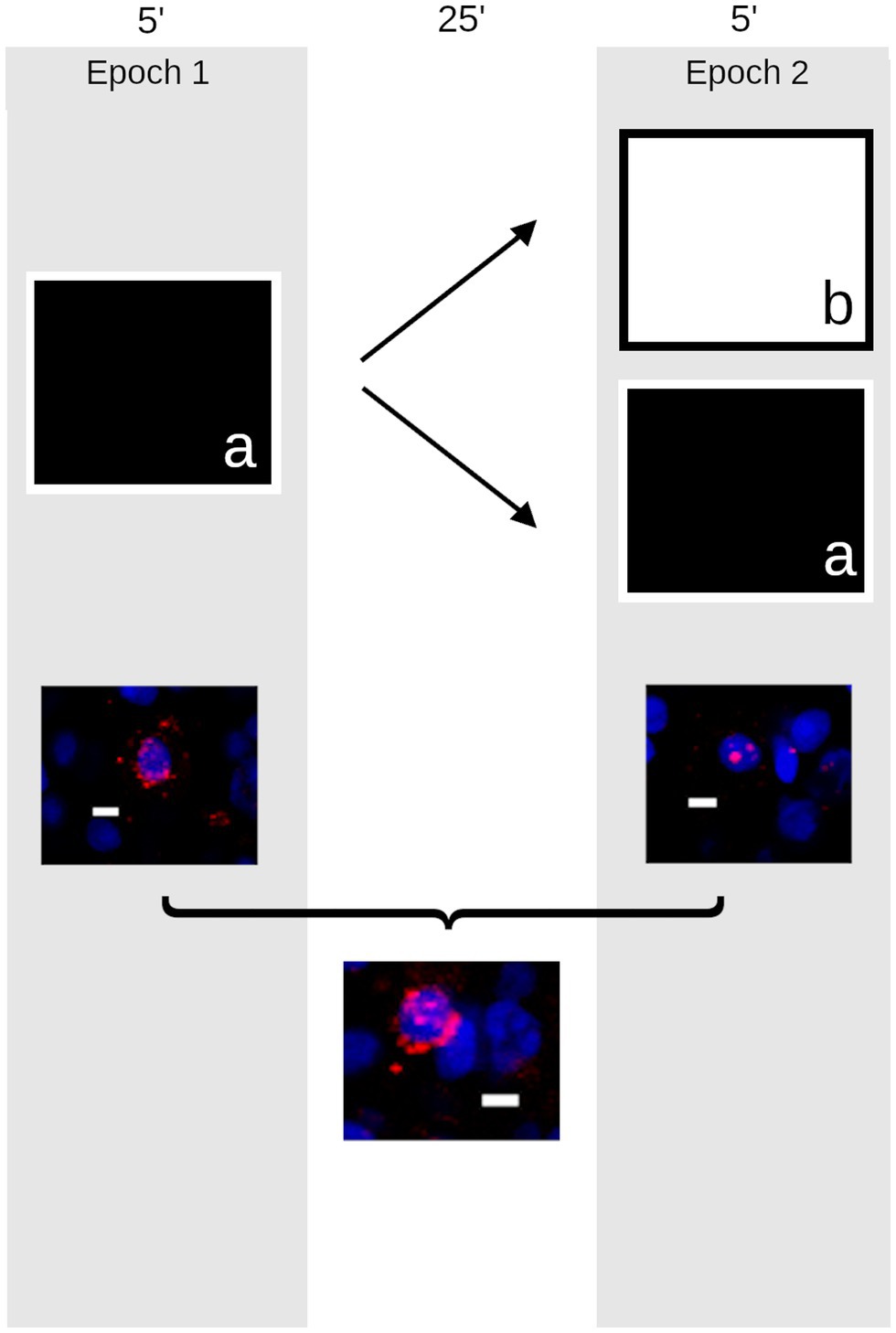
Figure 1. Schematic outline of the experiment. The timeline presented above shows that quail were taken to an open field (a) approximately 1 m2 containing distinct local and distal cues and provided passive exploration for 5 min (left). They returned to their housing room for 25 min before either being returned to the same environment (a) or placed in a new environment (b) in a different room containing a different complement of local and distal cues (right). Following these trials (below), the animals’ brains were harvested and the compartmental expression of Egr1 (red) was measured to provide a histological record of activity in neurons counterstained with DAPI (blue) in the quail hippocampus (Scale bar = 10 μm). Cells active during the first epoch on the maze expressed Egr1 within the cytoplasm (left); those active during the second epoch express Egr1 within the nucleus (right). Cells active during both epochs express Egr1 within both cellular compartments (bottom).
Tissue Preparation
At the end of behavioral testing, quail were anesthetized by isoflurane and decapitated. Brains were removed and flash frozen in a container of 2-methyl butane submerged in a secondary container containing an ethanol and dry ice slurry. Brains were then mounted in optimal cutting temperature (OCT) medium (Fischer Scientific, Whitby, ON), sectioned (20 μm thick) on a cryostat, and thaw-mounted on Superfrost Plus slides (VWR Scientific).
Fluorescent in situ hybridization was then performed as previously described (Grella et al., 2016). Briefly, digoxigenin-labeled Egr1 antisense riboprobes were synthesized using a transcription kit and digoxigenin-labeled UTP, denatured, and hybridized with the tissue overnight at 56°C. Following post-hybridization washes of gradually increasing stringency and 2% H2O2 to quench endogenous peroxidase, the riboprobe was detected with anti-digoxigenin-HRP and a CY3 tyramide signal amplification kit (Superglow red, Fluorescent Solutions, Augusta, GA). Slides were counterstained with DAPI, cover-slipped with anti-fade media, and sealed. All reagents are from Millipore Sigma (Oakville, ON) unless otherwise specified.
Imaging
As described previously (Grella et al., 2016), z-stacks (optical thickness: 1.1 μm, interval: 0.7 μm) were obtained throughout the entire thickness (~20 μm) from area DM of the rostral hippocampus, approximately ~5.0–6.0 mm anterior to the interaural plane (Baylé et al., 1974) from 5 to 6 slides per animal. The lateral boundary of DM was defined by a shallow sulcus running rostrocaudally on the dorsal ventricular surface, and images were taken at the midway point from that boundary and the midline. Images were collected using an Olympus FV1000 laser scanning confocal microscope at 40× with consistent photomultiplier tube assignments, confocal aperture size, and contrast values for each slide. Image analysis was conducted using FIJI (Schindelin et al., 2012). Neurons were differentiated from glia on the basis of their size and uneven chromatin staining and were counted by the optical dissector within the median 20% of planes in each confocal stack and were classified as: (a) Egr1-negative, (b) Egr1-positive in the nucleus, (c) Egr1-positive in the surrounding cytoplasm, or (d) expressing Egr1 in both the nucleus and cytoplasm (Figure 1). Because the localization of Egr1 provides a marker of the time at which each neuron was engaged in transcription, gene expression profiles were used to calculate the number of cells active during spatial exploration, rest, or both time periods. Cell counts were conducted blind to the experimental group. As previously described (Grella et al., 2016), an overlap measurement was calculated as the percentage of cells containing Egr1 in both cellular compartments divided by the lesser of the number of cells active during epoch 1 and epoch 2.
Group differences in Egr1 transcription were analyzed with a one-way ANOVA, with condition (i.e., same, different, and caged control) as the factor, followed by Tukey’s HSD post-hoc tests. In addition, the observed probability that cells express Egr1 in response to both environmental exposures was compared to the probability expected by chance using a paired t-test. All statistical comparisons were completed using JASP (JASP Team, 2021).
Results
Equivalent Behaviorally Induced Egr1 in the Left and Right Quail Hippocampus
No significant hemispheric differences were observed for Egr1 expression in either epoch 1 (F1,42 = 1.24; p = 0.27) or 2 (F1,42 = 2.80; p = 0.10), or in the overlap between the 2 (F1,42 = 2.01; p = 0.16). Moreover, no hemisphere by condition interactions were observed (p > 0.53 in all cases). These data indicate that this behavioral paradigm does not elicit a distinct response from the left and right hippocampus of quail.
The Contexts Visited Alter the Pattern, but Not Number, of Cells Expressing Egr1
Exploration robustly increased Egr1 in the quail HF both in epoch 1 (main effect: F2,42 = 241.94; p < 0.001) and 2 (main effect: F2,42 = 245.43; p < 0.001). In the HF of exploring quail, Egr1 was consistently expressed in ~20% of cells (Figure 2A), somewhat higher than reported for cowbirds (Grella et al., 2016) but considerably lower than comparable estimates of cellular recruitment in rodents (e.g., Gheidi et al., 2012).
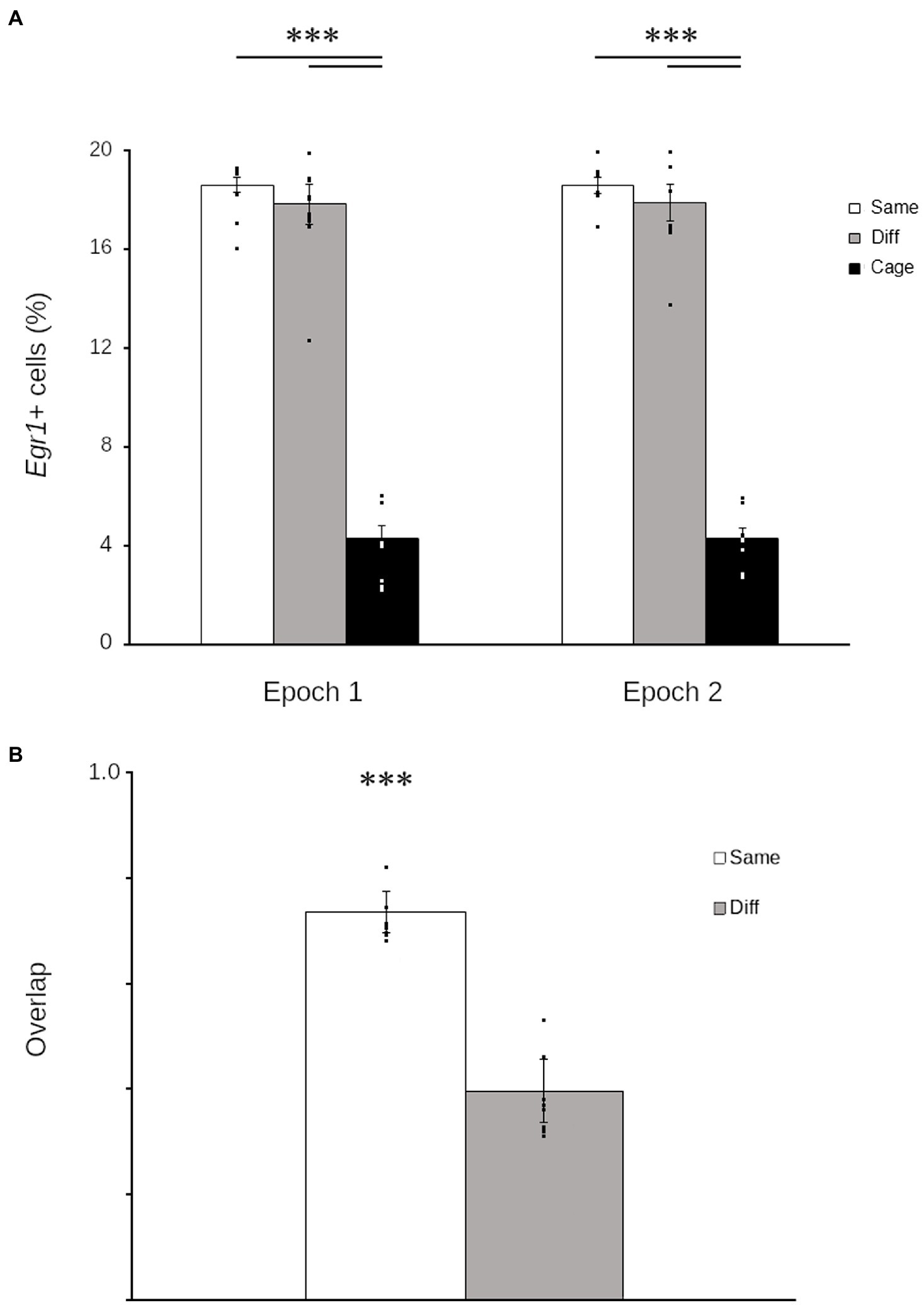
Figure 2. Hippocampal Egr1 expression is context-specific in Japanese quail. Graphs of Egr1 expression during each epoch (A) show that quail that explored either the same environment repeatedly (white) or two different environments (grey) both expressed Egr1 in significantly more cells than quail that remained in the housing room (black). Similarity scores (B) show that the proportion of cells that repeatedly expressed Egr1 across both explorations was significantly higher in birds that explored the same room twice (Same: white) relative to birds that explored different rooms (Different: grey). Data are means ± SEM (***p < 0.001). Individual data points are for each quail are superimposed on each bar.
Despite the consistency in the number of cells transcribing Egr1, the number of environments explored significantly altered the pattern of Egr1 expression (t30 = 14.07; p < 0.001). Quail that explored the same context twice demonstrated a significantly higher overlap relative to birds that explored two different contexts (Figure 2B). These data demonstrate that, as observed in mammals (e.g., Guzowski et al., 1999; Marrone et al., 2011) and other birds (Grella et al., 2016), the pattern of Egr1 expression in the HF of quail is contextually mediated.
Discussion
The current findings show that Japanese quail tested under conditions known to generate little place cell-like firing still recruit unique populations of cells in a context-specific manner. That is, when quail traverse through an environment, nearly 20% of cells transcribe Egr1, a proportion comparable, although marginally higher, than electrophysiological estimates under the same circumstances (Ben-Yishay et al., 2021). Moreover, the population of responsive neurons is fairly stable over time in the same location, as the vast majority of the cells are recruited again when quail return to the same environment. Importantly, a visually distinct environment results in the recruitment of a distinct population of cells, and the similarity of the recruited population of cells drops significantly when quail visit two distinct environments.
These results are remarkable in part because they expand our knowledge of the link between neural activity and immediate-early gene expression. Observing a context-specific pattern of Egr1 under conditions that do not produce place cell-like firing demonstrates that place cell-like activity is not required to trigger context-specific transcription. Rather, the high frequency firing of either a head direction cell responding to its preferred heading or a place cell responding to its preferred location are equally capable of triggering plasticity-related gene expression cascade. Unfortunately, this observation limits the utility of activity-dependent gene expression as an a priori tool in the search for place cell-like patterns of activity in the avian hippocampus. The current data add to these observations by showing that the head direction system in quail is capable of recruiting a context-specific cohort of cells. Many questions remain to be answered about how this is accomplished, but even a rudimentary binding of head directions to the features of the stimuli present could accomplish this. The fact that the preferred direction of many head direction cells of quail rotate when cues are rotated (Ben-Yishay et al., 2021) supports this kind of binding. It is intriguing to note that while simple binding of visual information with head direction likely provides an effective basis for discrimination under many circumstances, it would be vulnerable to interference in the face of similar stimuli. This is consistent with the available behavioral evidence showing that Japanese quail perform poorly when discriminating multiple environments with shared features (Damphousse et al., 2021).
The observation that quail can generate context-specific patterns of activity despite having a hippocampal network composed largely of head direction cells may not be surprising, as head direction cells are thought to provide a potential neural basis of vector learning (Pearce et al., 1998; Kosaki et al., 2015). Moreover, the attractor dynamics in the head direction system are thought to confer mnemonic ability (Sharp et al., 2001). Thus, head direction cells in quail (and likely many other birds) may provide a navigational system that can support a wide variety of spatial computations. In fact, the Japanese quail’s ability to perform a number of complex spatial learning tasks is consistent with this idea. Quail show preference for previously blocked arms in a Y-maze (Damphousse et al., 2021), show conditioned place preference (Mace et al., 1997; Eaton et al., 2022), and complete foraging arrays comparable to a Barnes maze or Morris water maze (Ruploh et al., 2011; Damphousse et al., 2022).
It is important to note, however, that the observation that quail do not express large numbers of place fields under these conditions does not necessarily mean that they cannot express place fields. Previous observations in the pigeon establish that the spatial information in avian hippocampus is dramatically reduced in the absence of a spatial task with fixed reward locations (Kahn et al., 2008). In fact, a similar, although much less pronounced, phenomenon has been demonstrated in rodents, as the presence of a spatial task increases both the information content and stability of hippocampal firing patterns (Kentros et al., 2004).
If further observations support a lack of large numbers of place cells in Japanese quail, these animals will help us to define the role of place cells in spatial learning networks in which they are present in large numbers. In this way, Japanese quail may provide a strong model animal to test models of navigation without cognitive maps (e.g., Kubie and Fenton, 2009; Cruse and Wehner, 2011; Le Moel et al., 2019; Bouchekioua et al., 2021). These data promise to greatly clarify the unique contributions of the head direction system and place cells to spatial cognition.
Data Availability Statement
The raw data supporting the conclusions of this article will be made available by the authors, without undue reservation.
Ethics Statement
The animal study was reviewed and approved by Wilfrid Laurier University Animal Care Committee.
Author Contributions
DM, NM, and CD designed the experiment and wrote the manuscript. CD and DM conducted the research and analyzed the data. All authors contributed to the article and approved the submitted version.
Funding
This research was supported by the Natural Sciences and Engineering Research Council of Canada.
Conflict of Interest
The authors declare that the research was conducted in the absence of any commercial or financial relationships that could be construed as a potential conflict of interest.
Publisher’s Note
All claims expressed in this article are solely those of the authors and do not necessarily represent those of their affiliated organizations, or those of the publisher, the editors and the reviewers. Any product that may be evaluated in this article, or claim that may be made by its manufacturer, is not guaranteed or endorsed by the publisher.
References
Agarwal, A., Sarel, A., Derdikman, D., Ulanovsky, N., and Gutfreund, Y. (2021). Spatial coding in the hippocampus of flying owls. bioRxiv [Preprint]. doi: 10.1101/2021.10.24.465553
Bellmund, J., Gärdenfors, P., Moser, E. I., and Doeller, C. F. (2018). Navigating cognition: spatial codes for human thinking. Science 362:eaat6766. doi: 10.1126/science.aat6766
Ben-Yishay, E., Krivoruchko, K., Ron, S., Ulanovsky, N., Derdikman, D., and Gutfreund, Y. (2021). Directional tuning in the hippocampal formation of birds. Curr. Biol. 31, 2592.e4–2602.e4. doi: 10.1016/j.cub.2021.04.029
Bouchekioua, Y., Blaisdell, A. P., Kosaki, Y., Tsutsui-Kimura, I., Craddock, P., Mimura, M., et al. (2021). Spatial inference without a cognitive map: the role of higher-order path integration. Biol. Rev. 96, 52–65. doi: 10.1111/brv.12645
Bozon, B., Davis, S., and Laroche, S. (2003). A requirement for the immediate early gene zif268 in reconsolidation of recognition memory after retrieval. Neuron 40, 695–701.
Cruse, H., and Wehner, R. (2011). No need for a cognitive map: decentralized memory for insect navigation. PLoS Comput. Biol. 7:e1002009. doi: 10.1371/journal.pcbi.1002009
Damphousse, C. C., Miller, N., and Marrone, D. F. (2021). Reaction to novelty as a behavioral assay of recognition memory in homing pigeons and Japanese quail. Learn. Behav. 50, 167–177. doi: 10.3758/s13420-021-00499-w
Damphousse, C. C., Miller, N., and Marrone, D. F. (2022). Dissociation of spatial and object memory in the hippocampal formation of Japanese quail. iScience 25:103805. doi: 10.1016/j.isci.2022.103805
Eaton, S. E., Dzhala, S., Robinson, L. E., Radevski, M. E., and Akins, C. K. (2022). Ethanol induces a dose-dependent conditioned place preference and conditioned place aversion in Japanese quail. Exp. Clin. Psychopharmacol. doi: 10.1037/pha0000548
Eichenbaum, H. (2017). The role of the hippocampus in navigation is memory. J. Neurophysiol. 117, 1785–1796. doi: 10.1152/jn.00005.2017
Epstein, R. A., Patai, E. Z., Julian, J. B., and Spiers, H. J. (2017). The cognitive map in humans: spatial navigation and beyond. Nat. Neurosci. 20, 1504–1513. doi: 10.1038/nn.4656
Gagliardo, A., Odetti, F., and Ioalé, P. (2001). Relevance of visual cues for orientation at familiar sites by homing pigeons: an experiment in a circular arena. Proc. R. Soc. Lond. B Biol. Sci. 268, 2065–2070. doi: 10.1098/rspb.2001.1746
Gheidi, A., Satvat, E., and Marrone, D. F. (2012). Experience-dependent recruitment of arc expression in multiple systems during rest. J. Neurosci. Res. 90, 1820–1829. doi: 10.1002/jnr.23057
Grella, S. L., Guigueno, M. F., White, D. J., Sherry, D. F., and Marrone, D. F. (2016). Context-dependent Egr1 expression in the avian hippocampus. PLoS One 11:e0164333. doi: 10.1371/journal.pone.0164333
Guzowski, J. F., McNaughton, B. L., Barnes, C. A., and Worley, P. F. (1999). Environment-specific expression of the immediate-early gene arc in hippocampal neuronal ensembles. Nat. Neurosci. 2, 1120–1124. doi: 10.1038/16046
Hough, G. E., and Bingman, V. P. (2004). Spatial response properties of homing pigeon hippocampal neurons: correlations with goal locations, movement between goals, and environmental context in a radial-arm arena. J. Comp. Physiol. A. 190, 1047–1062. doi: 10.1007/s00359-004-0562-z
Kahn, M. C., Siegel, J. J., Jechura, T. J., and Bingman, V. P. (2008). Response properties of avian hippocampal formation cells in an environment with unstable goal locations. Behav. Brain Res. 191, 153–163. doi: 10.1016/j.bbr.2008.03.023
Kennedy, P. J., and Shapiro, M. L. (2009). Motivational states activate distinct hippocampal representations to guide goal-directed behaviors. Proc. Natl. Acad. Sci. U. S. A. 106, 10805–10810. doi: 10.1073/pnas.0903259106
Kentros, C. G., Agnihotri, N. T., Streater, S., Hawkins, R. D., and Kandel, E. R. (2004). Increased attention to spatial context increases both place field stability and spatial memory. Neuron 42, 283–295. doi: 10.1016/S0896-6273(04)00192-8
Kosaki, Y., Poulter, S. L., Austen, J. M., and McGregor, A. (2015). Dorsolateral striatal lesions impair navigation based on landmark-goal vectors but facilitate spatial learning based on a “cognitive map”. Learn. Mem. 22, 179–191. doi: 10.1101/lm.037077.114
Kubie, J. L., and Fenton, A. A. (2009). Heading-vector navigation based on head-direction cells and path integration. Hippocampus 19, 456–479. doi: 10.1002/hipo.20532
Le Moel, F., Stone, T., Lihoreau, M., Wystrach, A., and Webb, B. (2019). The central complex as a potential substrate for vector based navigation. Front. Psychol. 10:690. doi: 10.3389/fpsyg.2019.00690
Mace, D. D., Kraemer, P. J., and Akins, C. K. (1997). Conditioned place preference in 12-day-old Japanese quail. Dev. Psychobiol. 31, 245–254. doi: 10.1002/(SICI)1098-2302(199712)31:4<245::AID-DEV2>3.0.CO;2-R
Markus, E. J., Qin, Y. L., Leonard, B., Skaggs, W. E., McNaughton, B. L., and Barnes, C. A. (1995). Interactions between location and task affect the spatial and directional firing of hippocampal neurons. J. Neurosci. 15, 7079–7094. doi: 10.1523/JNEUROSCI.15-11-07079.1995
Marrone, D. F., Adams, A. A., and Satvat, E. (2011). Increased pattern separation in the aged fascia dentata. Neurobiol. Aging 32, 2317.e23–2317.e32. doi: 10.1016/j.neurobiolaging.2010.03.021
O’Keefe, J., and Dostrovsky, J. (1971). The hippocampus as a spatial map. Preliminary evidence from unit activity in the freely-moving rat. Brain Res. 34, 171–175. doi: 10.1016/0006-8993(71)90358-1
Payne, H. L., Lynch, G. F., and Aronov, D. (2021). Neural representations of space in the hippocampus of a food-caching bird. Science 373, 343–348. doi: 10.1126/science.abg2009
Pearce, J. M., Roberts, A. D., and Good, M. (1998). Hippocampal lesions disrupt navigation based on cognitive maps but not heading vectors. Nature 396, 75–77. doi: 10.1038/23941
Ruploh, T., Kazek, A., and Bischof, H. J. (2011). Spatial orientation in Japanese quails (Coturnix coturnix japonica). PLoS One 6:e28202. doi: 10.1371/journal.pone.0028202
Schindelin, J., Arganda-Carreras, I., Frise, E., Kaynig, V., Longair, M., Pietzsch, T., et al. (2012). FIJI: an open-source platform for biological-image analysis. Nat. Methods 9, 676–682. doi: 10.1038/nmeth.2019
Sharp, P. E., Blair, H. T., and Cho, J. (2001). The anatomical and computational basis of the rat head-direction cell signal. Trends Neurosci. 24, 289–294. doi: 10.1016/S0166-2236(00)01797-5
Sherry, D. F., and Vaccarino, A. L. (1989). Hippocampus and memory for food cashes in black-capped chickadees. Behav. Neurosci. 103, 308–318. doi: 10.1037/0735-7044.103.2.308
Siegel, J. J., Nitz, D., and Bingman, V. P. (2005). Spatial-specificity of single-units in the hippocampal formation of freely moving homing pigeons. Hippocampus 15, 26–40. doi: 10.1002/hipo.20025
Skaggs, W. E., and McNaughton, B. L. (1998). Spatial firing properties of hippocampal CA1 populations in an environment containing two visually identical regions. J. Neurosci. 18, 8455–8466. doi: 10.1523/JNEUROSCI.18-20-08455.1998
White, A. R., Strasser, R., and Bingman, V. P. (2002). Hippocampus lesions impair landmark array spatial learning in homing pigeons: a laboratory study. Neurobiol. Learn. Mem. 78, 65–78. doi: 10.1006/nlme.2001.4053
Keywords: ZENK, avian, bird, place cell, navigation, cognitive maps
Citation: Damphousse CC, Miller N and Marrone DF (2022) Experience-Dependent Egr1 Expression in the Hippocampus of Japanese Quail. Front. Psychol. 13:887790. doi: 10.3389/fpsyg.2022.887790
Edited by:
Onur Güntürkün, Ruhr University Bochum, GermanyReviewed by:
Vern P. Bingman, Bowling Green State University, United StatesYoram Gutfreund, Technion Israel Institute of Technology, Israel
Copyright © 2022 Damphousse, Miller and Marrone. This is an open-access article distributed under the terms of the Creative Commons Attribution License (CC BY). The use, distribution or reproduction in other forums is permitted, provided the original author(s) and the copyright owner(s) are credited and that the original publication in this journal is cited, in accordance with accepted academic practice. No use, distribution or reproduction is permitted which does not comply with these terms.
*Correspondence: Diano F. Marrone, ZG1hcnJvbmVAd2x1LmNh