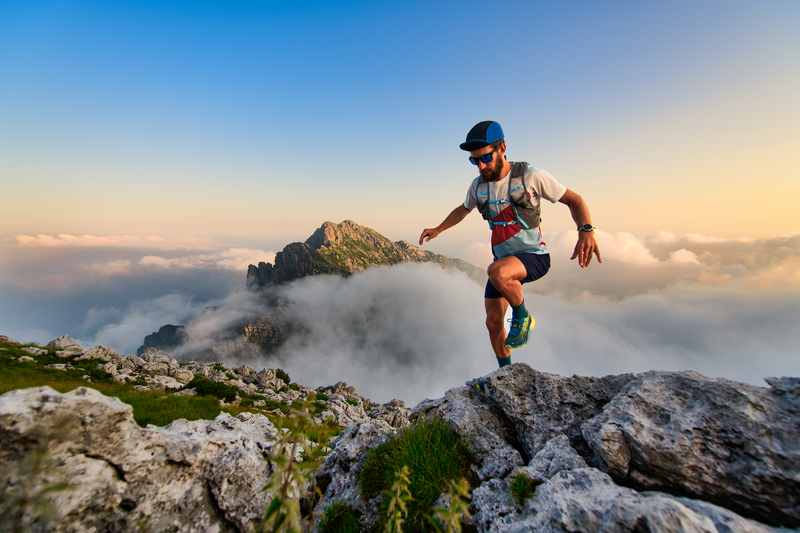
95% of researchers rate our articles as excellent or good
Learn more about the work of our research integrity team to safeguard the quality of each article we publish.
Find out more
REVIEW article
Front. Psychol. , 26 April 2022
Sec. Health Psychology
Volume 13 - 2022 | https://doi.org/10.3389/fpsyg.2022.831819
This article is part of the Research Topic Exercise Priming: The Use of Physical Exercise to Support Motor and Cognitive Function View all 5 articles
Neuroplasticity refers to the brain’s ability to undergo structural and functional adaptations in response to experience, and this process is associated with learning, memory and improvements in cognitive function. The brain’s propensity for neuroplasticity is influenced by lifestyle factors including exercise, diet and sleep. This review gathers evidence from molecular, systems and behavioral neuroscience to explain how these three key lifestyle factors influence neuroplasticity alone and in combination with one another. This review collected results from human studies as well as animal models. This information will have implications for research, educational, fitness and neurorehabilitation settings.
Contrary to prior assumptions regarding the rigidity of the mammalian nervous system during adulthood, mounting neuroscientific evidence has acknowledged that the adult brain changes in response to our experiences. Neuroplasticity refers to the brain’s ability to undergo structural and functional reorganization in response to learning or experience. The classic theory which posits that “neurons that fire together, wire together” (Hebb, 1949) accurately describes how plasticity of the brain is the result of strengthening the connections between neurons by repetitive firing patterns which activates distinct neurobiological mechanisms. Conversely, the “use it or lose it” principle also applies to neuroplasticity, where a lack of activity between neurons within a circuit leads to a decrease in the strength of connection between these neurons (Liepert et al., 1995). The strengthening of synaptic connections between neurons is known as long-term potentiation (LTP), and the activity-dependent reduction in strength is known as long-term depression (LTD) (Bear and Malenka, 1994). These processes are thought to be mediated by the activity of N-methyl-D-aspartate (NDMA) glutamate receptors (Peters et al., 2018). However, neuroplastic adaptations can be evaluated in humans using several neuroscientific techniques since changes occur at the molecular, cellular, systems, and behavioral levels (El-Sayes et al., 2019a). Each of these forms of measures are reflective of different aspects of neural activity, and neuroplasticity refers to any changes in the structure or function of our brain activity. Therefore, from this point forward in the review, neuroplasticity will be defined as changes in any of these measures discussed.
The timeline of neurophysiological processes underlying neuroplasticity varies from rapid adaptations of synaptic strength that are transient in nature, to more long-term structural modifications (Sagi et al., 2012). Molecular mechanisms including synaptogenesis (formation of new synaptic connections), neurogenesis (development of new neurons), angiogenesis (formation of new blood vessels) and gliogenesis (generation of non-neuronal glial cells in the brain) contribute to neuroplasticity (El-Sayes et al., 2019a). Cellular adaptations in response to experience include presynaptic changes such as greater neurotransmitter and neurotrophin release from the presynaptic neuron, decreased neurotransmitter reuptake and breakdown in the synaptic cleft, and postsynaptic adaptations resulting in deposition of additional neurotransmitter receptors on the cell membrane (Gulyaeva, 2017). From a systems neuroscience perspective, the size of the neural representation of muscles in the motor cortex increases in response to frequent and repetitive use of the muscle (Pascual-Leone et al., 1993). The increased cortical representation means that more neurons in the brain are dedicated to controlling that muscle. Increased cortical representation facilitates improved motor control of those muscles, revealing dynamic functional neuroplasticity as a result of experience (Martin, 2005). Conversely, neuroplasticity can also occur in the brain when intact adjacent brain regions overtake the function of nearby neurons disrupted by brain injury or disease (Leemhuis et al., 2019). Behavioral approaches to studying neuroplasticity also highlight how changes in the structure and function of neural circuits are associated with learning (Sagi et al., 2012), memory (Amtul and Atta-Ur-Rahman, 2015), and cognitive function (Vance et al., 2010). Changes in cognitive functioning serve as a measure of neuroplasticity because the improvements in performance on a cognitive task represent changes in the activity of the neural networks which execute these mental processes (Ji et al., 2017).
The magnitude of neuroplasticity that occurs in response to learning or experience is partially dependent on factors that influence brain activity and the predisposition for plasticity induction. Exercise, diet, and sleep are three behaviours that represent essential pillars of mental health because of their impact on the structure and function of the brain (Wickham et al., 2020). It is well understood that good nutrition, regular exercise and sufficient sleep are fundamental to maintaining a healthy lifestyle (Farooqui, 2014). A cross-sectional study revealed that sleep quality, adequate fruit and vegetable intake, and regular physical activity improve depressive symptoms and wellbeing in young adults (Wickham et al., 2020). However, the role of exercise, diet, sleep, and the combined influence of these factors on neuroplasticity is not yet fully understood. The purpose of this review is to discuss evidence from human and animal models that demonstrates the role of these three fundamental lifestyle factors in facilitating neuroplasticity from a behavioral, molecular, and systems neuroscience perspective. A stronger understanding of these influences will allow students and educators to better understand how exercising, eating healthy and getting proper sleep can benefit learning and memory for educational purposes. Findings from this review will also permit neurophysiology researchers to recognize how these factors influence neurophysiological biomarkers of neuroplasticity and provide them with suggestions for potential methods to account for these factors. Further, this review will help identify the importance of considering these factors when attempting to maximize exercise-induced neuroplasticity. As such, findings from this review have implications for educational, research, fitness and neurorehabilitation settings. A graphical summary of the main findings from each of the major sections in this review can be found in Figure 1.
Figure 1. Diagram representing the individual and combined influences of exercise, diet and sleep on neuroplasticity. This figure summarizes the key points from each of the major sections of this article and visually depicts how they are interrelated. Findings from this review are from studies in human and animal models. Neuroplasticity was measured using several techniques including neurophysiological measures, molecular biomarkers, and cognitive assessments (see Tables 1, 2 for further information). Keto: Ketogenic diet, MDiet: Mediterranean diet, EGCG: (–)epigallocatechin-3-gallate, REM sleep: rapid-eye movement sleep, PA levels = physical activity levels. ∗Healthy diet here refers to evidence from the Ketogenic diet, Mediterranean diet and nutritional supplements. ∗∗Adequate sleep quality is specific to individuals depending on age and other health factors. See Ohayon et al. (2017) for further information related to sleep recommendations.
Aerobic exercise refers to physical activities such as biking, running, or swimming that are repetitive in nature and promote the circulation of oxygen through the cardiovascular system. Aerobic exercise enhances the expression of neuroplasticity biomarkers including brain-derived neurotrophic factor (BDNF), insulin-like growth factor 1 (IGF-1), and vascular endothelial growth factor (VEGF) (Cotman et al., 2007; Knaepen et al., 2010; Mang et al., 2013). The cascade of molecular changes upregulates cellular processes of synaptogenesis, neurogenesis, angiogenesis and gliogenesis (El-Sayes et al., 2019a), elevates cerebral blood flow (Smith J. C. et al., 2010; Zimmerman et al., 2014), increases grey and white matter volume (Colcombe et al., 2006; Erickson et al., 2014; Fletcher et al., 2016; Sexton et al., 2016), and neuronal activity (Moraes et al., 2007; Bailey et al., 2008; Rajab et al., 2014; Gutmann et al., 2015). As such, aerobic exercise has been linked to improved cognitive and motor function. In a group of 10 young healthy individuals, 30 min of moderate-intensity cycling improved performance of several measures of memory, reasoning, concentration and planning compared to baseline (Nanda et al., 2013). In another study, 24 healthy young participants performed a pinch-grip motor task before and after 30 min of moderate intensity running. Results indicated that an acute bout of aerobic exercise led to improved motor skill acquisition mainly driven by improvements in accuracy (Statton et al., 2015). These findings demonstrate the usefulness of aerobic exercise as an adjunct tool for rehabilitation, as it appears to prime the nervous system for learning and skill acquisition. The effectiveness of exercise to promote cognitive functioning and neuroplasticity has been demonstrated in young and older healthy individuals (Sibley et al., 2006; Wang et al., 2016), as well as clinical populations such as stroke (Moriya et al., 2016), multiple sclerosis (Sandroff et al., 2015) and depression (Vasques et al., 2011). Aerobic exercise also aided in functional recovery and learning by mobilizing neural resources which prepare the brain for subsequent performance on cognitive tasks (Moriarty et al., 2019).
The capacity for aerobic exercise to induce neuroplasticity can be assessed using transcranial magnetic stimulation (TMS). TMS is a non-invasive form of brain stimulation that provides a measurement of cortical and corticospinal excitability. A change in corticospinal excitability following an acute bout of exercise is suggested to indicate that neuroplasticity has been induced. As reviewed previously (Turco and Nelson, 2021), studies have reported inconsistent changes in TMS measures following aerobic exercise. As an example, a single session of aerobic exercise has been reported to increase (Lulic et al., 2017; Neva et al., 2017; El-Sayes et al., 2019b; MacDonald et al., 2019; Nicolini et al., 2020) or not change (McDonnell et al., 2013; Singh et al., 2016; Brown et al., 2020; El-Sayes et al., 2020) corticospinal excitability as assessed with motor-evoked potentials (MEPs). Further, short-interval intracortical inhibition (SICI), an assessment of primary motor cortex (M1) microcircuitry, has been reported to decrease (Singh et al., 2014; Smith et al., 2014; Stavrinos and Coxon, 2017; Yamazaki et al., 2019) or not change (Mooney et al., 2016; Andrews et al., 2020; Morris et al., 2020; Hu et al., 2021) following a single session of aerobic exercise. Studies have also shown inconsistent effects of aerobic exercise on other common TMS measures including the contralateral silent period (CSP), intracortical facilitation (ICF), long-interval intracortical inhibition (LICI), and short-latency afferent inhibition (SAI) (see Turco and Nelson, 2021). Table 1 provides descriptions of the neurophysiological and molecular measures of neuroplasticity that are discussed in this review. Future research should continue to explore the effect of aerobic exercise on these TMS measures, since the conflicting results reported here could be due to differences in the type, intensity or volume of aerobic exercise prescribed, as well as the fitness levels of the individuals in the study.
Resistance exercise refers to repeated contraction of muscles against an external resistance. Some examples of resistance exercises include bodyweight exercises like pushups, and the use of dumbbells, resistance bands or exercise machines. This form of training leads to an increase in muscle strength driven by cellular responses in the short-term (days-weeks) that transition to structural changes through synaptogenesis following long-term training (months-years) (Tallent et al., 2021). A meta-analysis of 31 studies showed that resistance training increases MEPs and reduces SICI and CSP when the muscle is active (Siddique et al., 2020). This suggests that short-term resistance training overall leads to an increase in corticospinal output to the trained muscle. Evidence showing that resistance training leads to enhanced corticospinal output to the trained as well as the untrained limb demonstrates the global impact of training-induced neuroplasticity. For instance, MEP amplitude increased and SICI decreased in the untrained leg following 9 training sessions of single right leg squatting (Goodwill et al., 2012). No studies have been conducted to measure corticospinal adaptations to resistance training performed greater than 3 months in duration. Therefore, research on long-term adaptations is limited to findings from studies using a cross-sectional design. Studies on individuals with multiple years of resistance training experience found no differences in corticospinal excitability when compared to untrained controls, despite differences in strength (Del Olmo et al., 2006; Tallent et al., 2013). Another study reported a significant increase in spinal excitability with no differences in corticospinal excitability compared to controls in the biceps brachii of the non-dominant arm (Philpott et al., 2015). Future work should focus on implementing longitudinal designs to assess spinal and supraspinal adaptations following resistance training regimens lasting several months to several years in duration. Although these types of studies may pose some logistical challenges, they will provide fundamental new insights into the neural responses to chronic resistance training and allow us to begin to understand how they differ from acute and short-term responses.
Results from functional magnetic resonance imaging (fMRI) have showed greater hemodynamic activity in the anterior left middle temporal gyrus, left anterior insula and lateral orbital frontal cortex following 12 months of twice weekly resistance training in healthy senior women. These hemodynamic changes were also associated with improvements in cognition measured using the Flanker test (Liu-Ambrose et al., 2011). Performance improvements on the Flanker test induced by resistance training were partly mediated by more efficient engagement of response inhibition processes. It is also suggested that improvements in cognitive performance and neuroplasticity following training are influenced by mechanisms involving elevated IGF-1 (Cassilhas et al., 2007) and reduced homocysteine levels (Vincent et al., 2003). Another fMRI study investigated changes in functional connectivity and memory following resistance training in elderly women with Mild Cognitive Impairment. Results indicated that resistance training increased activity in three regions of the cortex during encoding and recall processes: right lingual gyrus, occipital fusiform gyrus, and the right frontal pole (Nagamatsu et al., 2012). There was also a positive correlation between the increased activity in right lingual gyrus and improvements in associative memory performance (Nagamatsu et al., 2012). These findings from fMRI studies further support the notion that exercising leads to increased activity in several brain regions implicated in important cognitive functions, and that these changes facilitate improved performance on assessments of these cognitive abilities.
Electroencephalography (EEG) is another neuroimaging method that has been used to investigate neural adaptations in response to resistance exercise. Event related potentials (ERP’s) are EEG measures that provide a non-invasive approach to assess brain functioning by measuring electrical activity over the scalp in response to repeated stimuli such as flashing lights or audible tones. The ERP is composed of several components identified by their polarity and order of occurrence. For example, the P1 component represents the first positive component of the response following stimulus onset, while N2 represents the second negative component (Beck and Dustman, 1975). Early ERP components reflect arrival and processing of sensory input, while later components are related to more complex cognitive functions such as attention and working memory (Kügler et al., 1993). In one study performed in older adults, participants were randomized into either a control, aerobic or resistance training group and underwent 9 weeks of training with three sessions per week. ERPs were collected in response to an auditory tone before and after training. Results from this study indicated that resistance training reduced latencies of the N1, N2, and P2 components as well as increased amplitudes of the N1P2, P2N2, and N2P3 components at the Cz and Fz locations (Özkaya et al., 2005). It was suggested that these changes in ERP’s highlight how resistance training can facilitate sensory processing and cognitive function in an older population (Özkaya et al., 2005).
Resistance exercise leads to neurophysiological adaptations through mechanisms unique from those induced by aerobic training (Cassilhas et al., 2012; Vilela et al., 2017). Evidence has suggested that major contributors to exercise induced neuroplasticity following both aerobic and resistance training are related to increased cerebral blood flow and increased IGF-1 levels (Loprinzi et al., 2020). However, aerobic exercise has a greater influence on BDNF levels, while resistance exercise is thought to have a greater influence on IGF-1 levels and reductions in Interleukin 6 (IL-6) levels (Loprinzi et al., 2020). Spatial memory is influenced by differential molecular pathways depending on the modality of exercise performed (Cassilhas et al., 2012). In a study using rats, it was found that aerobic training increased hippocampal levels of IGF-1, BDNF, tropomyosin receptor kinase B (TrkB), and calmodulin-dependent protein kinase II (β-CaMKII). Alternatively, resistance training increased the peripheral and hippocampal levels of IGF-1, along with activation of protein-kinase B (AKT) and the IGF-1 receptor (Cassilhas et al., 2012). In another study using rats, aerobic treadmill training was compared to ladder climbing with a weight attached to the rat’s tail (resistance training). Aerobic and resistance exercise both lead to improved spatial memory along with increased expression of proteins involved in synaptic plasticity (Vilela et al., 2017). However, each type of training resulted in unique molecular adaptations related to signaling proteins and neurotrophic factors. Specifically, aerobic exercise increased the expression of glutamatergic proteins and decreased DNA damage, while resistance training increased protein kinase C alpha (PKC α), tumor necrosis factor (TNF- α) and IL-1 β levels.
Due to these unique neurophysiological adaptations that occur in response to aerobic compared to resistance exercise discussed, studies have found that the combination of aerobic and resistance exercise can lead to greater levels of exercise-induced neuroplasticity than either type alone (Marinus et al., 2019). Meta-analyses have found that improvements in cognitive domains including attention, processing speed and working memory are greater following a combination of aerobic and resistance training compared to either form of training alone (Colcombe and Kramer, 2003; Smith P. J. et al., 2010). Many behavioral studies have also demonstrated improvements in cognition following aerobic and resistance training using several psychological tools which are described further in Table 2 (Cassilhas et al., 2007; Liu-Ambrose et al., 2010; Kluding et al., 2011; Nagamatsu et al., 2012; Shafer, 2020). Further, several studies have explored the influence of combined training on neurophysiological measures of neuroplasticity as well. For example, 6 months of performing a combined aerobic and resistance exercise training protocol significantly increased serum BDNF levels in healthy middle-aged women compared to a non-exercising control group (Cho et al., 2014). Combined training did not induce significantly larger changes in BDNF levels compared to aerobic training only in this study, but authors suggested that this may have been due to the low level of exercise intensity prescribed in this study (1 set of 30/40% 1RM) (Cho et al., 2014). However, another study that assessed changes in BDNF levels following either aerobic, resistance, or combined training in adolescents with obesity had different results. There were no significant increases in BDNF levels in the aerobic, resistance or combined groups compared to a diet-only control group following a 22-week training intervention (Goldfield et al., 2018). These conflicting findings regarding changes in BDNF may be limited to the specific age, sex or fitness levels of the individuals collected in these studies, as well as the differences in the specific frequency, intensity, time and type of aerobic and resistance exercise prescribed by the investigators. For this reason, further research in humans is needed to determine the relationship between combined exercise protocols and changes in BDNF and other biomarkers of neuroplasticity. This would improve our current understanding of the neurophysiological benefits of combining both types of training, as well as the optimal balance of the two types for individual fitness goals.
Improvements in cognition have also been reported following aerobic combined with resistance training in clinical populations. A randomized control trial in Chronic Obstructive Pulmonary Disease (COPD) reported additional improvements in multiple cognitive functions including long-term memory, apraxia, and reasoning skills compared to aerobic training only (Aquino et al., 2016). Similar findings were also reported in other reviews on the effects of fitness training on cognitive function in older adults (Colcombe and Kramer, 2003; Smith P. J. et al., 2010). The effects of combined exercise, aerobic exercise, or no exercise on cognitive function following 9 weeks of training was investigated in 109 patients with Dementia. The most pronounced improvements in cognitive (higher global cognition, visual memory, verbal memory and executive function) and motor (walking endurance, leg muscle strength and balance) performance occurred in the combined training group (Bossers et al., 2015). These findings demonstrate that combined training is more effective than aerobic training in isolation for slowing cognitive and motor decline in patients with dementia. However, improvements in motor and cognitive functioning returned toward baseline 9 weeks after training. This trend highlights the need for regular exercise to be able to acquire and maintain the neurological benefits of combined training. This is important information that can inform future studies trying to determine the minimum frequency and intensity of exercise required for patients with dementia, as well as other special populations.
Other studies in clinical populations have shown that exercise training led to improvements in other clinical cognitive assessments including the Fugl-Meyer Score and Stroke Impact Scale (SIS) (Kluding et al., 2011), Mini Mental Status Examination (MMSE) (Lee et al., 2016) and the Montreal Cognitive Assessment (MoCA) (Marzolini et al., 2013). These cognitive assessments of neuroplasticity are described further in Table 2. Overall, the evidence reviewed here suggests that aerobic and resistance exercise each profoundly influence neuroplasticity through distinct mechanisms. Further, it is likely that the combination of these types of training induces the greatest amount of exercise-induced neuroplasticity, both in healthy and clinical populations. This information may have considerable implications for neurorehabilitation programs that aim to recover motor and cognitive functioning in diseased states, or fitness programs aiming to enhance the mental and neurological benefits of exercise training. Nonetheless, further research in humans is still necessary to clarify the neurophysiological mechanisms underpinning these synergistic benefits in acute and prolonged exercise protocols.
Diet is a lifestyle factor known to influence brain structure and function that could alter the propensity for neuroplasticity (Gomez-Pinilla and Gomez, 2011; Murphy et al., 2014). Diet influences TMS measures of cortical and corticospinal activity. Acute intake of glucose increases MEPs and LICI (Specterman et al., 2005), with no change in SICI, ICF or resting motor threshold (RMT) (Badawy et al., 2013). However, glucose intake does not always influence corticospinal excitability (Toepp et al., 2019). The ketogenic diet and the Mediterranean diet (high in vegetables, fruits, legumes, nuts, grains, fish, and unsaturated fats but low in meat and dairy) both claim to support brain function among several other benefits (Petersson and Philippou, 2016). The Mediterranean diet appears to facilitate these benefits by preserving the integrity and structural connectivity of essential brain circuits associated with cognitive functioning (Pelletier et al., 2015). It has also been shown that adhering to a ketogenic diet for 2 weeks led to an increase in SICI, with no change in RMT, CSP or ICF (Cantello et al., 2007). Alternatively, a gluten-free diet decreases RMT without changes in CSP, MEP’s, SICI or ICF in patients with Celiac Disease (Bella et al., 2015). Together, these results identify the influence of several dietary factors on TMS measures of cortical and corticospinal activity.
Dietary interventions such as caloric restriction, intermittent fasting, and diet supplementation modulate markers of neuroplasticity and influence cognition and mood (Murphy et al., 2014). For example, caloric restriction involves a 20–40% reduction in daily caloric intake (Lee et al., 2002). Caloric restriction elevates BDNF levels (Lee et al., 2002), enhances performance on memory tests in humans (Witte et al., 2009) and sensorimotor function and learning in rats (Singh et al., 2012). Further, caloric restriction has also been shown to have positive implications for clinical populations including reduced risk of developing Alzheimer’s Disease (Luchsinger et al., 2002) and reduced severity of neurochemical imbalances and motor dysfunction in a primate model of Parkinson’s Disease (Maswood et al., 2004). Intermittent fasting is a form of restrictive eating where individuals cycle through stages of voluntary fasting and non-fasting throughout a day. An intermittent fasting cycle of 16 h fasting and 8 h fed has also been shown to increase hippocampal neurogenesis in mice, and this relationship is thought to be mediated by mechanisms involving Notch 1 signaling (Baik et al., 2020).
There is increasing interest in the use of dietary interventions to improve symptoms in Autism (Cieślińska et al., 2017), Alzheimer’s Disease (Rusek et al., 2019) and Parkinson’s Disease (Shah and Duda, 2015). There are the positive effects of the ketogenetic diet on the brain and various clinical conditions. The ketogenic diet is a high-fat, adequate-protein, low-carbohydrate diet which shifts energy metabolism to rely on fats instead of carbohydrates and has been used as an intervention for individuals with epilepsy (D’Andrea Meira et al., 2019). The ketogenic diet has proposed neuroprotective effects including a reduction in free radicals (Gasior et al., 2006; Masino and Rho, 2012), upregulation of the glutathione antioxidant enzyme in the hippocampus (Ziegler et al., 2003), reduction in inflammation, and normalization of synapses between neurons (Stafstrom and Rho, 2012). In individuals with Alzheimer’s Disease, the ketogenic diet improved cognitive function (Henderson et al., 2009; Kashiwaya et al., 2013; Ota et al., 2018), motor function (Beckett et al., 2013; Brownlow et al., 2013) and reduced beta amyloids and tau proteins in the brain (Van DerAuwera et al., 2005; Studzinski et al., 2008). In Parkinson’s Disease, the ketogenic diet relieved motor and non-motor symptoms (Phillips et al., 2018), reduced cell death (Cheng et al., 2007) and improved motor performance (Tieu et al., 2003; Shaafi et al., 2016). However, neurological conditions like these have a complex pathophysiology that can negatively influence processes of neuroplasticity in the brain. To discuss these effects in detail is beyond the scope of this review. To learn more about this, see the following reviews on neuroplasticity in special populations including Alzheimer’s Disease (Koch and Spampinato, 2022), Parkinson’s Disease (Hirsch and Farley, 2009), Stroke (Hara, 2015) and other neurological conditions (Thickbroom and Mastaglia, 2009).
Supplementation of omega-3 fatty acids, catechin polyphenols and curcumin have been shown to support cognitive processes (McCann and Ames, 2005) and exert neuroprotective effects (Murphy et al., 2014). Potential mechanisms of the effect of omega-3 fatty acids on neuroplasticity include its influence on depolarization-induced glutamate transmitter release, increased hippocampal neurotrophin levels (Wu et al., 2004) and increased expression of genes essential for synaptic plasticity (Wu et al., 2007). Docosahexaenoic acid (DHA) is an omega-3 fatty acid that allows for phospholipid signal transduction to occur at the synapse. This is an essential process for brain glucose uptake and preventing oxidative stress (Pifferi et al., 2005). Mechanisms involved in learning, memory, and cognitive decline are modulated by catechin polyphenols (Mandel and Youdim, 2004; Vauzour, 2012). Their antioxidant properties allow them to release hydrogens to reduce the reactive oxygen species and free radicals in the body. (–)epigallocatechin-3-gallate (EGCG), a catechin polyphenol has been shown to be implicated in synaptic plasticity as well as learning and memory (Wroblewski and Danysz, 1989; Bliss and Collingridge, 1993; Haque et al., 2006). EGCG modulates glutamate release by upregulating calcium entry which in turn activates PKC, a pathway correlated to learning and memory (Levites et al., 2003; Chou et al., 2007). Studies have shown that the structure of curcumin may underly its positive effects on brain function, as it can move across the blood brain barrier (Garcia-Alloza et al., 2007) and reduces oxidative stress by transferring electrons to readily collect free radicals (Phillips et al., 2018). Curcumin supplementation for 3 weeks before and after induced traumatic brain injuries (Wu et al., 2006; Sharma et al., 2010) led to increased BDNF and cAMP response element binding proteins. These are both markers of synaptic plasticity and facilitation of neurogenesis of the hippocampus in rats (Wu et al., 2006). This supplement has also been found to modulate chronic inflammation via lowering TNF- alpha concentration levels which aid neurotransmission and neurogenesis (Sahebkar et al., 2016). Alternatively, there is evidence suggesting that unhealthy dietary habits such as caloric heavy diets and consumption of saturated fats and sugars can have negative consequences on brain health and functioning (Molteni et al., 2002; Gomez-Pinilla, 2008). Spatial learning and BDNF levels in the hippocampus were reduced in rats following 2 months on a high saturated fat and refined sugar diet. These findings were interpreted as impairments in synaptic plasticity and motor learning. In support of this, the chronic consumption of a diet high in saturated fat is associated with an increased risk of cognitive decline and eventually dementia through mechanisms mediated by the development of insulin resistance and type II diabetes (Greenwood and Winocur, 2005). Although the evidence of dietary interventions is promising, it is important to note that these studies have been done in animal models and future steps require more widespread evaluations in human populations.
In summary, an individual’s dietary choices clearly effect neuroplastic processes in the brain. Adherence to certain dietary interventions such as the Mediterranean diet, ketogenic diet, caloric restriction, intermittent fasting and diet supplementation appear to increase measures of neuroplasticity in the brain. Therefore, routine consumption of unhealthy diets such as those high in calories, saturated fats and sugars conversely leads to negative consequences to brain functioning. Together, these results suggest that dietary factors should be a major consideration when attempting to maximize neuroplasticity induced by training or learning. An individual’s dietary habits will also be valuable information for researchers trying to understand individual differences in responses to the same intervention attempting to induce neuroplasticity, such as exercise or non-invasive brain stimulation.
Evidence suggests that the combination of diet and exercise provides synergistic benefits (Kishi and Sunagawa, 2012; Murphy et al., 2014; Hutton et al., 2015). Exercise combined with caloric restriction enhanced BDNF levels in mice more than fasting or exercise alone (Stranahan et al., 2009). Supplementation of omega-3 fatty acids such as DHA increased rat hippocampal BDNF levels, and this effect is increased when applied concurrently with exercise (Wu et al., 2008). Further, axonal growth and synaptic function were enhanced by a 1.25% DHA diet and exercise more than DHA or exercise alone (Chytrova et al., 2010). A study comparing the effects of a 6-week polyphenol-rich diet alone to a regimen combining this diet with aerobic exercise (treadmill running) on spatial memory in mice found that the combination of diet and exercise evoked greater enhancements in spatial memory, and these changes were associated with increased angiogenesis and neuronal spine density in the hippocampus (van Praag et al., 2007). Further, there was an upregulation of genes associated with learning, alongside decreases in genes associated with neurodegeneration in the neurons of the hippocampus (van Praag et al., 2007).
Long-term adherence to both an aerobic exercise and healthy diet regimen such as the Mediterranean Diet (Hardman et al., 2020) or minimum consumption of > 400 g/day of fruits/vegetables and > 2 servings of fish per week led to significant improvements in cognition within middle-aged and older adults (Komulainen et al., 2021). This is particularly important, because these diets alone were not reported to induce long-term cognitive benefits (Hardman et al., 2020; Komulainen et al., 2021). Further, another study recruited 148 active Air Force Airmen and placed them on a 12-week randomized controlled trial. The intervention was characterized by combining aerobic and strength training with a novel nutritional supplement [comprised of β-hydroxy β-methylbutyrate (HMB), lutein, phospholipids, DHA and selected micronutrients including B12 and folic acid] which led to greater improvements in working memory and reaction time compared to aerobic and strength training alone (Zwilling et al., 2020). This evidence suggests that exercise-induced neuroplasticity may be facilitated by modifications in diet. However, these results may have limited generalizability since this study was done in healthy, young, highly trained military personnel only. Therefore, even though the study has uncovered a plausible link, further research to quantify the brain changes via neuroimaging must be done to uncover the effect of these multimodal interventions. Furthermore, future directions would require an individualized dietary-exercise regime as individual physiological variability is rather robust.
Sleep is an important factor implicated in brain plasticity (Frank, 2015), recovery from brain injury (Jasey and Ward, 2019), as well as cognitive functioning, learning and memory (Ellenbogen, 2005). Neuroplasticity is facilitated by the adequate delivery of cerebral blood flow to supply active neurons with oxygen while simultaneously removing waste products. There are dynamic changes in cerebral blood flow during the sleep-wake cycle, and these involuntary patterns may have an influence on the passive processes of neuroplasticity that occur during sleep (Elvsåshagen et al., 2019). Sleep quantity also influences active processes of neuroplasticity, as evidenced by the influence of sleep deprivation on task-related cerebral blood flow and cognitive functioning. Twenty-four hours of sleep deprivation resulted in impaired attention and reaction time, as well as decreased cerebral blood flow from the right middle cerebral artery during a finger tapping task in young healthy males (Csipo et al., 2021). Similarly, cerebral blood flow has been shown to be reduced in the cerebellum, cuneus and fusiform gyrus of the right hemisphere, along with increased cerebral blood flow in the inferior occipital gyrus in the opposite hemisphere in night shift workers compared to daytime workers (Park et al., 2019). This study also noted that these decreases were significantly correlated with self-reported severity of depression and insomnia. Together, these findings suggest that decreased cerebral blood flow induced by poor sleep can have a negative influence on cognitive functioning and processes of neuroplasticity. Sleep also influences the regulation of several important neurotrophic factors that could mediate the influence of sleep on neuroplasticity. For example, sleep regulates the growth hormone (GH)/IGF-1 axis such that IGF-1 levels are decreased in the context of “sleep loss” and increased in situations of “sleep extension” (Chennaoui et al., 2020). Similarly, sleep disturbances over a long-term period leads to decreased BDNF levels, whereas acute sleep deprivation causes an increase in BDNF levels as part of the physiological stress response in humans (Schmitt et al., 2016).
Sleep deprivation is known to alter brain excitability and intracortical inhibition, which may consequently diminish the potential for neuroplasticity (Kreuzer et al., 2011). Specifically, studies investigating the influence of sleep deprivation on TMS measures have consistently reported a decrease in SICI and CSP in comparison to a night of normal sleep (Scalise et al., 2006; Kreuzer et al., 2011; Placidi et al., 2013). Likewise, conditions including insomnia, restless leg syndrome or sleep apnea (which are characterized by a reduced quality and/or quantity of sleep) have abnormal single- and paired-pulse TMS measures of cortical excitability and inhibition compared to control groups as reviewed previously (Lanza et al., 2015). M1 excitability is decreased in sleep apnea and increased in RLS. Other conditions such as insomnia and sleep deprivation present imbalanced inhibitory/excitatory intracortical circuitry resulting in heightened intracortical inhibition (Lanza et al., 2015). Further, individuals with RLS and sleep apnea have demonstrated irregular responses to TMS-induced plasticity. Repetitive TMS (rTMS) is a plasticity-inducing TMS paradigm consisting of a rapid (5–10 Hz) succession of pulses delivered to the cortex which induces lasting changes in brain excitability (Fitzgerald et al., 2006). A 10 Hz rTMS over M1 increased MEP amplitude in healthy controls, but not in untreated patients with severe obstructive sleep apnea (Das et al., 2013). Continuous theta-burst stimulation (cTBS) is form of rTMS involving clusters of three pulses delivered in rapid succession, which is believed to induce LTD and suppress cortical excitability (Huang et al., 2005). When performed in patients with sleep apnea, cTBS at 50 Hz over the left M1 did not decrease M1 excitability unlike the decrease seen in healthy controls (Opie et al., 2013). Finally, paired-associative stimulation (PAS) is a paradigm involving the repeated pairing TMS and peripheral electrical nerve stimulation which can strengthen corticospinal excitability (Alder et al., 2019). Delivery of a 90 pulse PAS protocol increased corticospinal excitability in healthy controls but not patients with RLS. However, the propensity for associative plasticity was restored in patients following 4 weeks of dopaminergic treatment (Rizzo et al., 2009). These disrupted responses to plasticity-inducing paradigms in individual’s with sleep disorders suggest that normal processes of neuroplasticity are dysregulated by a reduction in sleep. These results highlight how the proper quantity and quality of sleep is essential to promote neuroplasticity in the brain. The amount of sleep that an individual should get every night depends on their age and other health factors. For further information on sleep recommendations, see Ohayon et al. (2017).
Sleep can largely be divided into non-rapid eye movement (NREM) and rapid eye movement (REM) stages, with the NREM stages further divided into NREM 1, 2, 3, and 4. During normal human sleep, individuals cycle through these stages every 90 min, with the ratio of REM/NREM shifting from largely NREM 3 and 4 in the first half, to primarily NREM 1, 2 and REM sleep later in the night (Walker, 2009). Each of these stages is characterized by distinct brain activity and serves a unique role in the restoration and maintenance of the body during sleep (Moser et al., 2009). Due to these differences, certain stages of sleep may have a more pronounced effect on neuroplasticity than others. For example, REM sleep is known to actively involve processes of neuroplasticity in the hippocampus during the night (Frank, 2015). These changes are thought to have implications with consolidation of learning and memory (Maquet, 2001). When comparing NREM to REM sleep deprivation, REM sleep deprivation resulted in a reduction of CSP and SICI, while NREM sleep deprivation did not change these measures (Placidi et al., 2013). These findings suggest that REM sleep may have significant influences on processes of neuroplasticity during waking hours. In contrast, another study done in rats found that sleep continuity, but not the amount of REM sleep, was critical for learning and memory on a novel object recognition task (Rolls et al., 2011). However, this discrepancy may be due to the difference in species being investigated in these two studies. To test this theory, future research should develop more investigation into the role of REM sleep on neuroplasticity in the human brain using different neuroimaging and neurophysiological techniques.
There is evidence for the effect of sleep on memory processing at the molecular, neuronal, systems and behavioral level (Maquet, 2001). However, the neurophysiological mechanisms underlying these effects are not yet fully understood (Walker, 2009). The inconsistent results mentioned in this review demonstrate the need for more studies to directly compare the effect of REM to NREM sleep deprivation on memory and other neurophysiological measures of plasticity. It has been shown that memory consolidation and synaptic remodeling processes occur actively while learning (Mansvelder et al., 2019). Interestingly though, these processes have also been shown to continue during sleep due to mechanisms related to post-learning plasticity (Stee and Peigneux, 2021). These findings highlight the importance of sleep quality before and after learning or training when attempting to promote neuroplasticity.
In conclusion, sleep is a complex biological process which has a multitude of effects on neuroplastic processes in the brain, both during sleep and while awake. Our findings here would suggest that getting the adequate amount of sleep based on recommended guidelines (Ohayon et al., 2017) may increase the propensity for neuroplasticity in the brain. Alternatively, sleep deprivation, reduced REM sleep duration, or the presence of a sleep disorder may result in disrupted neuroplastic processes which can have a negative influence on memory consolidation during sleep, as well as motor control and cognition during waking hours. These results highlight the importance of controlling for an individual’s sleep status in studies measuring neuroplasticity, and of promoting good sleeping habits to allow for optimal performance in cognitive or physical tasks.
Few studies have investigated the combined influences of exercise and sleep on neuroplasticity. One recent study found that higher levels of daily physical activity at the expense of sedentary behaviors and sleep are associated with greater cortical neuroplasticity (Smith et al., 2021). Interestingly, it was shown that the increases in neuroplasticity were equal regardless of whether physical activity replaces time spent sedentary or sleeping (Smith et al., 2021). However, evidence showing disrupted neuroplasticity due to poor sleep discussed in the previous section of this review would suggest that the most beneficial decision for neuroplasticity would be to replace sedentary activity with physical activity rather than sacrifice your sleep. Sleep quality has been identified as a mediator of the relationship between physical activity and neurocognitive function along with stress, mood and pain (Stillman et al., 2016). Sleep efficiency (total amount of time spent sleeping in bed) also mediated the relationship between physical activity and multiple measures of cognitive performance including working memory, recall and verbal skills in younger and older adults (Wilckens et al., 2018). Exercise, sleep and diet are all important factors contributing to the neural pathways involved in major depression which is characterized by disrupted neural processes related to plasticity inducing neurotransmitter imbalances, HPA-axis disturbances, oxidative stress, mitochondrial disturbances and inflammation (Lopresti et al., 2013). Exercise and sleep are also primary factors that influence the maximization of recovery from brain injury and neuroplasticity (Jasey and Ward, 2019).
Higher physical activity levels are associated with better sleep, so the interaction between these two factors may be particularly important. For example, in a 16-week randomized control trial to determine the effects of exercise training on sleep in healthy older adults, individuals in the exercise group displayed significant improvements in the global sleep score on the Pittsburgh Sleep Quality Index (PSQI) compared to a non-exercising control group (King et al., 1997). In another longitudinal study of older adults, better sleep quality at baseline predicted higher levels of physical activity at the follow up measurement, regardless of prior physical activity (Holfeld and Ruthig, 2014). Further, baseline physical activity levels did not predict sleep quality at the follow up (Holfeld and Ruthig, 2014). Together, these findings suggest a bidirectional relationship between physical activity and sleep quality, though further investigation is necessary to determine the underlying mechanisms mediating this relationship. Since these studies were both done in healthy older adults, future work should investigate this relationship in different age groups and demographic populations to ensure that these findings are not limited to this group only.
Several animal model studies have shown that engaging in exercise may be able to prevent sleep-deprivation related reductions in neuroplasticity in mice and rats. Seventy-two hours of sleep deprivation leads to aberrant signaling in the hippocampus and impaired short-term memory performance in mice when compared to a sedentary control group, but these changes were prevented by aerobic exercise prior to the sleep deprivation (Tuan et al., 2021). Authors suggested that aerobic exercise had a neuroprotective effect on microglia-mediated synaptic pruning that occurs in sleep-deprived brains and can be used as a method to cope with sleep insufficiency (Tuan et al., 2021). Two other studies exploring this relationship in rats have found that regular treadmill running prevented sleep-deprivation related disruptions in synaptic plasticity and neuronal signaling (Zagaar et al., 2013) as well as late-phase LTP in the dentate gyrus (Zagaar et al., 2016). Finally, 4 weeks of voluntary aerobic exercise in female rats prevented sleep deprivation related impairments in early-phase LTP in the hippocampus along with reductions in behavioral assessments of learning and memory (Rajizadeh et al., 2020). Collectively, these findings support the existence of a complex interaction between exercise and sleep that is mediated by several neurophysiological mechanisms not yet fully understood. Further investigation is required to comprehensively understand the protective effects of exercise on the negative health consequences of sleep-deprivation, as well as the ideal combination of sleep and exercise to prime neuroplasticity.
Previous research studies have suggested a bidirectional relationship between diet and sleep quality that is mediated through mechanisms related to neuroinflammation, neurogenesis, and neuroplasticity (Godos et al., 2020a,b, 2021). Several studies have investigated the influence of diet on sleep in healthy and clinical populations. Adherence to a Mediterranean diet over 2 years was associated with lower risk of poor sleep quality and duration compared to those with lower adherence (Campanini et al., 2017). Similarly, another study revealed that adherence to a Mediterranean diet was associated with better sleep quality (Godos et al., 2019). The association between adherence to this diet and sleep quality may be indirectly mediated by reduced weight and improved body composition (Godos et al., 2019). Related findings were reported in a study that examined the effects of a ketogenic diet in adolescents with morbid obesity. The dietary intervention induced weight loss that consequently led to increases in REM sleep and decreases in NREM sleep toward normal levels. This was suggested to be due to less obstructive blockage of the airways during sleep (Willi et al., 1998).
Another study evaluated the antiepileptic properties of the ketogenic diet by exploring it’s effect on sleep in children with epilepsy. Adherence to the ketogenic diet increased REM sleep duration and improved sleep quality, which contributed to an increased in quality of life (Hallböök et al., 2007). Findings from this study are limited by it’s small sample of 18 participants all younger than 18 years of age, so more work must be done to confirm these findings and extend them into other age groups. In another study, improvements in several health measures including sleep quality and daytime sleepiness resulted in improved quality of life following ketogenic dietary intervention in patients with obesity (Castro et al., 2018). Although limited by a small sample size, these promising results may also help contribute to an increasing use of the ketogenic diet as a form of therapy in the upcoming years.
Results from studies exploring the combination of sleep and diet demonstrate a clear interplay between these lifestyle factors. In summary, maintaining or introducing a healthy diet will result in positive outcomes in the brain which have beneficial effects on sleep quality. This interplay between factors suggests that the proper combination of diet and sleep may result in the optimization of the brains ability to undergo neuroplasticity. Despite the abundance of evidence highlighting the relationship between sleep, diet, and brain function, no study has been done to directly understand how different combinations of sleep quality or quantity and dietary interventions effect the propensity for neuroplasticity in response to exercise or non-invasive plasticity-inducing protocols like rTMS or PAS.
This manuscript does not provide an exhaustive review on the literature related to exercise, diet, sleep and neuroplasticity. Instead, the intention is to provide a comprehensive understanding of each of these topics and their combinations using publications that have directed these fields of research. Importantly, this review serves as an opportunity to identify gaps in knowledge for which future research directions will pursue.
Future research studies are encouraged to compare the neurophysiological responses to aerobic, resistance and combined training in the context of acute and long-term exercise programs in both healthy and clinical populations in order to translate findings to neurorehabilitation. The intensity of the exercise protocol and fitness level of individuals involved are particularly important factors that must be considered when designing these studies. Further, researchers might consider assessing responses in the resting and active muscle states to fully elucidate the neuroplastic adaptations induced by exercise training paradigms. These studies will provide invaluable information regarding the differences in neurophysiological adaptations to these two types of exercise and how a combination of the two may provide additional neural benefits.
However, there are also alternative forms of exercise that exist such as balance training, flexibility training, and other complex or coordinative exercises. These types of exercise were not discussed in this review because there is a limited amount of research on these topics, and the purpose of this review was to discuss the most common types of exercise used most frequently. Yet, evidence suggests that these types of exercise also possess the potential to influence the structure and function of the brain in healthy individuals (Rogge et al., 2018) as well as in special populations such as individuals with Parkinson’s Disease (Sehm et al., 2014). Therefore, future work should also investigate how these alternative forms of exercise influence measures of neuroplasticity in isolation, and in combination with aerobic and resistance exercise.
In relation to diet, it is suggested that future research explores popular and emerging diets from a neurophysiological perspective to improve our understanding of the potential benefits they offer in conjunction with exercise training and sleep quality. Also, dietary supplements with reported benefits to learning, memory or brain function can be assessed using neurophysiological measurements such as TMS or changes in biomarkers like BDNF or IGF-1. Doing so will allow for researchers to determine if there is any evidence of neuroplasticity following consumption, or if regular/prolonged consumption leads to lasting changes in brain excitability.
There is a need for further investigation into the influence of the stages of sleep on the neuroplastic responses to interventions such as exercise or rTMS by assessing the effect of total or partial sleep deprivation on neurophysiological measures of neuroplasticity, similar to the work done by Kreuzer et al. (2011). This work will be important to understand the complex relationship between REM sleep and neuroplasticity, as well as to elucidate the role of NREM sleep in these processes.
Researchers measuring neuroplasticity in future studies are strongly encouraged to incorporate assessments of physical activity, sleep quality and eating/dietary habits at baseline and throughout the duration of longitudinal studies. This practice can be used to evaluate the influence of these factors as mediators of the relationship between the intervention and neuroplasticity in a study. Examples of commonly used tools that can evaluate these factors include the PSQI for sleep, International Physical Activity Questionnaire (IPAQ) for exercise, and food logs for diet. Finally, researchers should continue to identify other lifestyle factors that also may influence neuroplasticity. Some examples of other factors to explore include mood, motivation, mindfulness, stress, socioeconomic status, intelligence, education level, and substance use. In addition, it is recommended that experimenters systematically investigate the interplay of exercise, diet and sleep in relation to neuroplasticity in a single study to converge on the optimal balance of these factors necessary to promote neuroplasticity.
Neuroplasticity underlies the basis of learning as well as recovery from brain injury and neurological impairment, making it an essential component of the research and neurorehabilitation efforts. We reviewed evidence from molecular, systems and behavioral neuroscience from human and animal models to summarize the influence of exercise, diet, and sleep patterns on neuroplasticity. Studies investigating how the combination of these factors affects neuroplasticity were also discussed, highlighting the importance of a holistic approach when aiming to induce neuroplasticity. Nevertheless, it is important to note that this paper does not provide an exhaustive list, and researchers should continue to identify the role of other potential lifestyle factors to expand our current understanding of how they influence the neurophysiological mechanisms underpinning neuroplasticity. Findings from such studies will continue provide valuable insight into how future research should control for these fundamental lifestyle factors in their studies. Examples of methods to control for these factors includes the screening process, inclusion/exclusion criteria, baseline and outcome measures recorded, and forms of analysis adopted. We also offered several suggestions for future studies to address some important gaps in the literature that will be vital in progressing toward a more efficient induction of neuroplasticity for research, learning, fitness, and rehabilitative purposes.
JP, CT, and KR: writing – original draft and writing – review and editing. RR and SF: writing – review and editing. AN: funding acquisition and writing – review and editing. All authors contributed to manuscript revision, read, and approved the submitted version.
This work was supported by a grant from the Natural Sciences and Engineering Research Council of Canada (NSERC) to AN (RGPIN-2015-06309).
The authors declare that the research was conducted in the absence of any commercial or financial relationships that could be construed as a potential conflict of interest.
All claims expressed in this article are solely those of the authors and do not necessarily represent those of their affiliated organizations, or those of the publisher, the editors and the reviewers. Any product that may be evaluated in this article, or claim that may be made by its manufacturer, is not guaranteed or endorsed by the publisher.
We would like to thank the members of AN’s Neurophysiology and Imaging Lab at McMaster University for the continued assistance with the writing and editing process.
BDNF, brain-derived neurotrophic factor; CSP, contralateral silent period; cTBS, continuous theta-burst stimulation; DHA, Docosahexaenoic acid; EEG, electroencephalography; ERP, event-related potential; fMRI, functional magnetic resonance imaging; ICF, intracortical facilitation; IGF-1, insulin-like growth factor 1; IPAQ, international physical activity questionnaire; LICI, long-interval intracortical inhibition; LTD, long-term depression; LTP, long-term potentiation; M1, primary motor cortex; MEP, motor-evoked potential; MMSE, mini mental status examination; MoCA, Montreal Cognitive Assessment; NREM, non-rapid eye movement; PAS, paired-associative stimulation; PKC, protein kinase C; PSQI, Pittsburgh Sleep Quality Index; REM, rapid eye movement; RMT, resting motor threshold; rTMS, repetitive TMS; SAI, short-latency afferent inhibition; SICI, short-interval intracortical inhibition; SIS, Stroke Impact Scale; VEGF, vascular endothelial growth factor.
Alder, G., Signal, N., Olsen, S., and Taylor, D. (2019). A systematic review of paired associative stimulation (PAS) to modulate lower limb corticomotor excitability: implications for stimulation parameter selection and experimental design. Front. Neurosci. 13:895. doi: 10.3389/fnins.2019.00895
Amtul, Z., and Atta-Ur-Rahman. (2015). Neural plasticity and memory: molecular mechanism. Rev. Neurosci. 26, 253–268. doi: 10.1515/revneuro-2014-0075
Andrews, S. C., Curtin, D., Hawi, Z., Wongtrakun, J., Stout, J. C., and Coxon, J. P. (2020). Intensity matters: high-intensity interval exercise enhances motor cortex plasticity more than moderate exercise. Cereb. Cortex 30, 101–112. doi: 10.1093/cercor/bhz075
Aquino, G., Iuliano, E., di Cagno, A., Vardaro, A., Fiorilli, G., Moffa, S., et al. (2016). Effects of combined training vs aerobic training on cognitive functions in COPD: a randomized controlled trial. Int. J. Chron. Obstruct. Pulmon. Dis. 11, 711–718. doi: 10.2147/COPD.S96663
Badawy, R. A. B., Vogrin, S. J., Lai, A., and Cook, M. J. (2013). Cortical excitability changes correlate with fluctuations in glucose levels in patients with epilepsy. Epilepsy Behav. 27, 455–460. doi: 10.1016/j.yebeh.2013.03.015
Baik, S. H., Rajeev, V., Fann, D. Y. W., Jo, D. G., and Arumugam, T. V. (2020). Intermittent fasting increases adult hippocampal neurogenesis. Brain Behav. 10:e01444. doi: 10.1002/brb3.1444
Bailey, S. P., Hall, E. E., Folger, S. E., and Miller, P. C. (2008). Changes in EEG during graded exercise on a recumbent cycle ergometer. J. Sport. Sci. Med. 7, 505–511.
Bear, M. F., and Malenka, R. C. (1994). Synaptic plasticity: LTP and LTD. Curr. Opin. Neurobiol. 4, 389–399. doi: 10.1016/0959-4388(94)90101-5
Beck, E. C., and Dustman, R. E. (1975). “Changes in evoked responses during maturation and aging in man and macaque,” in Behavior and Brain Electrical Activity, eds N. Burch and H. L. Altshuler (Boston, MA: Springer), 431–472. doi: 10.1007/978-1-4613-4434-6_19
Beckett, T. L., Studzinski, C. M., Keller, J. N., Murphy, P., and Niedowicz, D. M. (2013). A ketogenic diet improves motor performance but does not affect β-amyloid levels in a mouse model of Alzheimer’s disease. Brain Res. 1505, 61–67. doi: 10.1016/j.brainres.2013.01.046
Bella, R., Lanza, G., Cantone, M., Giuffrida, S., Puglisi, V., Vinciguerra, L., et al. (2015). Effect of a gluten-free diet on cortical excitability in adults with celiac disease. PLoS One 10:e0129218. doi: 10.1371/journal.pone.0129218
Bliss, T. V., and Collingridge, G. L. (1993). A synaptic model of memory: long-term potentiation in the hippocampus. Nature 361, 31–39. doi: 10.1038/361031a0
Bossers, W. J. R., Van Der Woude, L. H. V., Boersma, F., Hortobágyi, T., Scherder, E. J. A., and Van Heuvelen, M. J. G. (2015). A 9-week aerobic and strength training program improves cognitive and motor function in patients with dementia: a randomized, controlled Trial. Am. J. Geriatr. Psychiatry 23, 1106–1116. doi: 10.1016/j.jagp.2014.12.191
Brown, K. E., Neva, J. L., Mang, C. S., Chau, B., Chiu, L. K., Francisco, B. A., et al. (2020). The influence of an acute bout of moderate-intensity cycling exercise on sensorimotor integration. Eur. J. Neurosci. 52, 4779–4790. doi: 10.1111/ejn.14909
Brownlow, M. L., Benner, L., D’Agostino, D., Gordon, M. N., and Morgan, D. (2013). Ketogenic diet improves motor performance but not cognition in two mouse models of Alzheimer’s pathology. PLoS One 8:e75713. doi: 10.1371/journal.pone.0075713
Campanini, M. Z., Guallar-Castillón, P., Rodríguez-Artalejo, F., and Lopez-Garcia, E. (2017). Mediterranean diet and changes in sleep duration and indicators of sleep quality in older adults. Sleep 40:zsw083. doi: 10.1093/sleep/zsw083
Cantello, R., Varrasi, C., Tarletti, R., Cecchin, M., D’Andrea, F., Veggiotti, P., et al. (2007). Ketogenic diet: electrophysiological effects on the normal human cortex. Epilepsia 48, 1756–1763. doi: 10.1111/j.1528-1167.2007.01156.x
Cassilhas, R. C., Lee, K. S., Fernandes, J., Oliveira, M. G. M., Tufik, S., Meeusen, R., et al. (2012). Spatial memory is improved by aerobic and resistance exercise through divergent molecular mechanisms. Neuroscience 202, 309–317. doi: 10.1016/j.neuroscience.2011.11.029
Cassilhas, R. C., Viana, V. A. R., Grassmann, V., Santos, R. T., Santos, R. F., Tufik, S., et al. (2007). The impact of resistance exercise on the cognitive function of the elderly. Med. Sci. Sports Exerc. 39:1401. doi: 10.1249/mss.0b013e318060111f
Castro, A. I., Gomez-Arbelaez, D., Crujeiras, A. B., Granero, R., Aguera, Z., Jimenez-Murcia, S., et al. (2018). Effect of a very low-calorie ketogenic diet on food and alcohol cravings, physical and sexual activity, sleep disturbances, and quality of life in obese patients. Nutrients 10:1348. doi: 10.3390/nu10101348
Cheng, B., Yang, X., Hou, Z., Lin, X., Meng, H., Li, Z., et al. (2007). D-beta-hydroxybutyrate inhibits the apoptosis of PC12 cells induced by 6-OHDA in relation to up-regulating the ratio of Bcl-2/Bax mRNA. Auton. Neurosci. 134, 38–44. doi: 10.1016/j.autneu.2007.02.002
Chennaoui, M., Léger, D., and Gomez-Merino, D. (2020). Sleep and the GH/IGF-1 axis: Consequences and countermeasures of sleep loss/disorders. Sleep Med. Rev. 49:101223. doi: 10.1016/j.smrv.2019.101223
Cho, H. C., Kim, J. K., Lee, N. J., Kim, S. Y., and Yoon, N. K. (2014). Effects of combined exercise on cardiovascular risk factors and serum BDNF level in mid-aged women. J. Exerc.Nutr. Biochem. 18:61. doi: 10.5717/jenb.2014.18.1.61
Chou, C. W., Huang, W. J., Tien, L. T., and Wang, S. J. (2007). (–)-Epigallocatechin gallate, the most active polyphenolic catechin in green tea, presynaptically facilitates Ca2+-dependent glutamate release via activation of protein kinase C in rat cerebral cortex. Synapse 61, 889–902. doi: 10.1002/syn.20444
Chytrova, G., Ying, Z., and Gomez-Pinilla, F. (2010). Exercise contributes to the effects of DHA dietary supplementation by acting on membrane-related synaptic systems. Brain Res. 1341, 32–40. doi: 10.1016/j.brainres.2009.05.018
Cieślińska, A., Kostrya, E., and Savelkoul, H. (2017). Treating autism spectrum disorder with gluten-free and casein-free diet: the underlying microbiota-gut-brain axis mechanisms. Clin. Immunol. Immunother. 3, 1–11. doi: 10.24966/ciit-8844/100009
Colcombe, S. J., Erickson, K. I., Scalf, P. E., Kim, J. S., Prakash, R., McAuley, E., et al. (2006). Aerobic exercise training increases brain volume in aging humans. J. Gerontol. Ser. A Biol. Sci. Med. Sci. 61, 1166–1170. doi: 10.1093/gerona/61.11.1166
Colcombe, S., and Kramer, A. F. (2003). Fitness effects on the cognitive function of older adults: a meta-analytic study. Psychol. Sci. 14, 125–130. doi: 10.1111/1467-9280.t01-1-01430
Cotman, C. W., Berchtold, N. C., and Christie, L. A. (2007). Exercise builds brain health: key roles of growth factor cascades and inflammation. Trends Neurosci. 30, 464–472. doi: 10.1016/j.tins.2007.06.011
Csipo, T., Lipecz, A., Owens, C., Mukli, P., Perry, J. W., Tarantini, S., et al. (2021). Sleep deprivation impairs cognitive performance, alters task-associated cerebral blood flow and decreases cortical neurovascular coupling-related hemodynamic responses. Sci. Rep. 11:20994. doi: 10.1038/s41598-021-00188-8
D’Andrea Meira, I., Romão, T. T., Pires do Prado, H. J., Krüger, L. T., Pires, M., and da Conceição, P. O. (2019). Ketogenic diet and epilepsy: what we know so far. Front. Neurosci. 13:5. doi: 10.3389/fnins.2019.00005
Das, A., Anupa, A. V., and Radhakrishnan, A. (2013). Reduced plastic brain responses to repetitive transcranial magnetic stimulation in severe obstructive sleep apnea syndrome. Sleep Med. 14, 636–640. doi: 10.1016/j.sleep.2013.04.008
Del Olmo, M. F., Reimunde, P., Viana, O., Acero, R. M., and Cudeiro, J. (2006). Chronic neural adaptation induced by long-term resistance training in humans. Eur. J Appl. Physiol. 96, 722–728. doi: 10.1007/s00421-006-0153-5
Ellenbogen, J. M. (2005). Cognitive benefits of sleep and their loss due to sleep deprivation. Neurology 64, E25–E27. doi: 10.1212/01.wnl.0000164850.68115.81
El-Sayes, J., Harasym, D., Turco, C. V., Locke, M. B., and Nelson, A. J. (2019a). Exercise-induced neuroplasticity: a mechanistic model and prospects for promoting plasticity. Neuroscientist 25, 65–85. doi: 10.1177/1073858418771538
El-Sayes, J., Turco, C. V., Skelly, L. E., Nicolini, C., Fahnestock, M., Gibala, M. J., et al. (2019b). The effects of biological sex and ovarian hormones on exercise-induced neuroplasticity. Neuroscience 410, 29–40. doi: 10.1016/j.neuroscience.2019.04.054
El-Sayes, J., Turco, C. V., Skelly, L. E., Locke, M. B., Gibala, M. J., and Nelson, A. J. (2020). Acute high-intensity and moderate-intensity interval exercise do not change corticospinal excitability in low fit, young adults. PLoS One 15:e0227581. doi: 10.1371/journal.pone.0227581
Elvsåshagen, T., Mutsaerts, H. J., Zak, N., Norbom, L. B., Quraishi, S. H., Pedersen, P. Ø, et al. (2019). Cerebral blood flow changes after a day of wake, sleep, and sleep deprivation. NeuroImage 186, 497–509. doi: 10.1016/j.neuroimage.2018.11.032
Erickson, K. I., Leckie, R. L., and Weinstein, A. M. (2014). Physical activity, fitness, and gray matter volume. Neurobiol. Aging 35, S20–S28. doi: 10.1016/j.neurobiolaging.2014.03.034
Farooqui, A. A. (2014). “The effects of diet, exercise, and sleep on brain metabolism and function,” in Inflammation and Oxidative Stress in Neurological Disorders, ed. A. A. Farooqui (Cham: Springer), 1–42. doi: 10.1007/978-3-319-04111-7_1
Fitzgerald, P. B., Fountain, S., and Daskalakis, Z. J. (2006). A comprehensive review of the effects of rTMS on motor cortical excitability and inhibition. Clin. Neurophysiol. 117, 2584–2596. doi: 10.1016/j.clinph.2006.06.712
Fletcher, M. A., Low, K. A., Boyd, R., Zimmerman, B., Gordon, B. A., Tan, C. H., et al. (2016). Comparing aging and fitness effects on brain anatomy. Front. Hum. Neurosci. 10:286. doi: 10.3389/fnhum.2016.00286
Frank, M. G. (2015). Sleep and synaptic plasticity in the developing and adult brain. Curr. Top. Behav. Neurosci. 25, 123–149. doi: 10.1007/7854_2014_305
Garcia-Alloza, M., Borrelli, L. A., Rozkalne, A., Hyman, B. T., and Bacskai, B. J. (2007). Curcumin labels amyloid pathology in vivo, disrupts existing plaques, and partially restores distorted neurites in an Alzheimer mouse model. J. Neurochem. 102, 1095–1104. doi: 10.1111/j.1471-4159.2007.04613.x
Gasior, M., Rogawski, M. A., and Hartman, A. L. (2006). Neuroprotective and disease-modifying effects of the ketogenic diet. Behav. Pharmacol. 17, 431–439. doi: 10.1097/00008877-200609000-00009
Godos, J., Currenti, W., Angelino, D., Mena, P., Castellano, S., Caraci, F., et al. (2020a). Diet and mental health: review of the recent updates on molecular mechanisms. Antioxidants 9:346. doi: 10.3390/antiox9040346
Godos, J., Ferri, R., Castellano, S., Angelino, D., Mena, P., Del Rio, D., et al. (2020b). Specific dietary (Poly)phenols are associated with sleep quality in a cohort of Italian adults. Nutrients 12:1226. doi: 10.3390/nu12051226
Godos, J., Ferri, R., Caraci, F., Cosentino, F. I. I., Castellano, S., Galvano, F., et al. (2019). Adherence to the mediterranean diet is associated with better sleep quality in Italian adults. Nutrients 11, 1–15. doi: 10.3390/nu11050976
Godos, J., Grosso, G., Castellano, S., Galvano, F., Caraci, F., and Ferri, R. (2021). Association between diet and sleep quality: a systematic review. Sleep Med. Rev. 57:101430. doi: 10.1016/j.smrv.2021.101430
Goldfield, G. S., Kenny, G. P., Prud’homme, D., Holcik, M., Alberga, A. S., Fahnestock, M., et al. (2018). Effects of aerobic training, resistance training, or both on brain-derived neurotrophic factor in adolescents with obesity: the hearty randomized controlled trial. Physiol. Behav. 191, 138–145. doi: 10.1016/j.physbeh.2018.04.026
Gomez-Pinilla, F. (2008). Brain foods: the effects of nutrients on brain function. Nat. Rev. Neurosci. 9, 568–578. doi: 10.1038/nrn2421
Gomez-Pinilla, F., and Gomez, A. G. (2011). The influence of dietary factors in central nervous system plasticity and injury recovery. PM R 3(6 Suppl. 1) S111–S116. doi: 10.1016/j.pmrj.2011.03.001
Goodwill, A. M., Pearce, A. J., and Kidgell, D. J. (2012). Corticomotor plasticity following unilateral strength training. Musc. Nerve 46, 384–393. doi: 10.1002/mus.23316
Greenwood, C. E., and Winocur, G. (2005). High-fat diets, insulin resistance and declining cognitive function. Neurobiol. Aging 26, 42–45. doi: 10.1016/j.neurobiolaging.2005.08.017
Gulyaeva, N. V. (2017). Molecular mechanisms of neuroplasticity: an expanding universe. Biochemistry 82, 237–242. doi: 10.1134/S0006297917030014
Gutmann, B., Mierau, A., Hülsdünker, T., Hildebrand, C., Przyklenk, A., Hollmann, W., et al. (2015). Effects of physical exercise on individual resting state EEG alpha peak frequency. Neural Plast. 2015:717312. doi: 10.1155/2015/717312
Hallböök, T., Lundgren, J., and Rosén, I. (2007). Ketogenic diet improves sleep quality in children with therapy-resistant epilepsy. Epilepsia 48, 59–65.
Haque, A. M., Hashimoto, M., Katakura, M., Tanabe, Y., Hara, Y., and Shido, O. (2006). Long-term administration of green tea catechins improves spatial cognition learning ability in rats. J. Nutr. 136, 1043–1047. doi: 10.1093/jn/136.4.1043
Hara, Y. (2015). Brain plasticity and rehabilitation in stroke patients. J. Nippon Med. Sch. 82, 4–13. doi: 10.1272/jnms.82.4
Hardman, R. J., Meyer, D., Kennedy, G., MacPherson, H., Scholey, A. B., and Pipingas, A. (2020). Findings of a pilot study investigating the effects of mediterranean diet and aerobic exercise on cognition in cognitively healthy older people living independently within aged-care facilities: the lifestyle intervention in independent living aged care (LIILAC) study. Curr. Dev. Nutr. 4:nzaa077. doi: 10.1093/cdn/nzaa077
Hebb, D. O. (1949). The Organization of Behavior; A Neuropsycholocigal Theory. New York, NY: Wiley, 62–78.
Henderson, S. T., Vogel, J. L., Barr, L. J., Garvin, F., Jones, J. J., and Costantini, L. C. (2009). Study of the ketogenic agent AC-1202 in mild to moderate Alzheimer’s disease: a randomized, double-blind, placebo-controlled, multicenter trial. Nutr. Metab. (Lond.) 6:31. doi: 10.1186/1743-7075-6-31
Hirsch, M. A., and Farley, B. G. (2009). Exercise and neuroplasticity in persons living with Parkinson’s disease. Eur. J. Phys. Rehabil. Med. 45, 215–229.
Holfeld, B., and Ruthig, J. C. (2014). A longitudinal examination of sleep quality and physical activity in older adults. J. Appl. Gerontol. 33, 791–807. doi: 10.1177/0733464812455097
Hu, M., Zeng, N., Gu, Z., Zheng, Y., Xu, K., Xue, L., et al. (2021). Short-term high-intensity interval exercise promotes motor cortex plasticity and executive function in sedentary females. Front. Hum. Neurosci. 15:620958. doi: 10.3389/fnhum.2021.620958
Huang, Y. Z., Edwards, M. J., Rounis, E., Bhatia, K. P., and Rothwell, J. C. (2005). Theta burst stimulation of the human motor cortex. Neuron 45, 201–206.
Hutton, C. P., Déry, N., Rosa, E., Lemon, J. A., Rollo, C. D., Boreham, D. R., et al. (2015). Synergistic effects of diet and exercise on hippocampal function in chronically stressed mice. Neuroscience 308, 180–193. doi: 10.1016/j.neuroscience.2015.09.005
Jasey, N., and Ward, I. (2019). Neuroplasticity in brain injury: maximizing recovery. Curr. Phys. Med. Rehabil. Rep. 7, 333–340. doi: 10.1007/s40141-019-00242-7
Ji, L., Zhang, H., Potter, G. G., Zang, Y. F., Steffens, D. C., Guo, H., et al. (2017). Multiple neuroimaging measures for examining exercise-induced neuroplasticity in older adults: a quasi-experimental study. Front. Aging Neurosci. 9:102. doi: 10.3389/fnagi.2017.00102
Kashiwaya, Y., Bergman, C., Lee, J. H., Wan, R., King, M. T., Mughal, M. R., et al. (2013). A ketone ester diet exhibits anxiolytic and cognition-sparing properties, and lessens amyloid and tau pathologies in a mouse model of Alzheimer’s disease. Neurobiol. Aging 34, 1530–1539. doi: 10.1016/j.neurobiolaging.2012.11.023
King, A. C., Oman, R. F., Brassington, G. S., Bliwise, D. L., and Haskell, W. L. (1997). Moderate-intensity exercise and self-rated quality of sleep in older adults: a randomized controlled trial. JAMA 277, 32–37. doi: 10.1001/jama.1997.03540250040029
Kishi, T., and Sunagawa, K. (2012). Exercise training plus calorie restriction causes synergistic protection against cognitive decline via up-regulation of BDNF in hippocampus of stroke-prone hypertensive rats. Proc. Annu. Int. Conf. IEEE Eng. Med. Biol. Soc. EMBS 2012, 6764–6767. doi: 10.1109/EMBC.2012.6347547
Kluding, P. M., Tseng, B. Y., and Billinger, S. A. (2011). Exercise and executive function in individuals with chronic stroke: a pilot study. J. Neurol. Phys. Ther. 35, 11–17. doi: 10.1097/NPT.0b013e318208ee6c
Knaepen, K., Goekint, M., Heyman, E. M., and Meeusen, R. (2010). Neuroplasticity exercise-induced response of peripheral brain-derived neurotrophic factor: a systematic review of experimental studies in human subjects. Sport. Med. 40, 765–801. doi: 10.2165/11534530-000000000-00000
Koch, G., and Spampinato, D. (2022). Alzheimer disease and neuroplasticity. Handb. Clin. Neurol. 184, 473–479. doi: 10.1016/B978-0-12-819410-2.00027-8
Komulainen, P., Tuomilehto, J., Savonen, K., Männikkö, R., Hassinen, M., Lakka, T. A., et al. (2021). Exercise, diet, and cognition in a 4-year randomized controlled trial: dose-responses to exercise training (DR’s EXTRA). Am. J. Clin. Nutr. 113, 1428–1439. doi: 10.1093/ajcn/nqab018
Kreuzer, P., Langguth, B., Popp, R., Raster, R., Busch, V., Frank, E., et al. (2011). Reduced intra-cortical inhibition after sleep deprivation: A transcranial magnetic stimulation study. Neurosci. Lett. 493, 63–66. doi: 10.1016/j.neulet.2011.02.044
Kügler, C. F. A., Taghavy, A., and Platt, D. (1993). The event-related P300 potential analysis of cognitive human brain aging: a review. Gerontology 39, 280–303. doi: 10.1159/000213544
Lanza, G., Cantone, M., Lanuzza, B., Pennisi, M., Bella, R., Pennisi, G., et al. (2015). Distinctive patterns of cortical excitability to transcranial magnetic stimulation in obstructive sleep apnea syndrome, restless legs syndrome, insomnia, and sleep deprivation. Sleep Med. Rev. 19, 39–50. doi: 10.1016/j.smrv.2014.04.001
Lee, J., Seroogy, K. B., and Mattson, M. P. (2002). Dietary restriction enhances neurotrophin expression and neurogenesis in the hippocampus of adult mice. J. Neurochem. 80, 539–547. doi: 10.1046/j.0022-3042.2001.00747.x
Lee, N. H., Lee, C. M., and Lee, S. (2016). Effects of combined exercise on blood lipids, estradiol, and cognitive function in the elderly women with mild cognitive loss. Int. J. Phys. Educ. Sports Health 3, 249–254.
Leemhuis, E., De Gennaro, L., and Pazzaglia, M. (2019). Disconnected body representation: Neuroplasticity following spinal cord injury. J. Clin. Med. 8:2144. doi: 10.3390/jcm8122144
Levites, Y., Amit, T., Mandel, S., and Youdim, M. B. (2003). Neuroprotection and neurorescue against Abeta toxicity and PKC-dependent release of nonamyloidogenic soluble precursor protein by green tea polyphenol (–)-epigallocatechin-3-gallate. FASEB J. 17, 952–954. doi: 10.1096/fj.02-0881fje
Liepert, J., Tegenthoff, M., and Malin, J. P. (1995). Changes of cortical motor area size during immobilization. Electroencephalogr. Clin. Neurophysiol. Electromyogr. 97, 382–386. doi: 10.1016/0924-980X(95)00194-P
Liu-Ambrose, T., Nagamatsu, L. S., Graf, P., Beattie, B. L., Ashe, M. C., and Handy, T. C. (2010). Resistance training and executive functions: a 12-month randomized controlled trial. Arch. Intern. Med. 170, 170–178. doi: 10.1001/archinternmed.2009.494
Liu-Ambrose, T., Nagamatsu, L. S., Voss, M. W., Khan, K. M., and Handy, T. C. (2011). Resistance training and functional plasticity of the aging brain: a 12-month randomized controlled trial. Neurobiol. Aging 33, 1690–1698. doi: 10.1016/j.neurobiolaging.2011.05.010
Lopresti, A. L., Hood, S. D., and Drummond, P. D. (2013). A review of lifestyle factors that contribute to important pathways associated with major depression: diet, sleep and exercise. J. Affect. Disord. 148, 12–27. doi: 10.1016/j.jad.2013.01.014
Loprinzi, P. D., Moore, D., and Loenneke, J. P. (2020). Does aerobic and resistance exercise influence episodic memory through unique mechanisms? Brain Sci. 10:913. doi: 10.3390/brainsci10120913
Luchsinger, J. A., Tang, M. X., Shea, S., and Mayeux, R. (2002). Caloric intake and the risk of Alzheimer disease. Arch. Neurol. 59, 1258–1263. doi: 10.1001/archneur.59.8.1258
Lulic, T., El-Sayes, J., Fassett, H. J., and Nelson, A. J. (2017). Physical activity levels determine exercise-induced changes in brain excitability. PLoS One 12:e0173672. doi: 10.1371/journal.pone.0173672
MacDonald, M. M., Khan, H., Kraeutner, S. N., Usai, F., Rogers, E. A., Kimmerly, D. S., et al. (2019). Intensity of acute aerobic exercise but not aerobic fitness impacts on corticospinal excitability. Appl. Physiol. Nutr. Metab. 44, 869–878. doi: 10.1139/apnm-2018-0643
Mandel, S., and Youdim, M. B. (2004). Catechin polyphenols: neurodegeneration and neuroprotection in neurodegenerative diseases. Free Radic. Biol. Med. 37, 304–317. doi: 10.1016/j.freeradbiomed.2004.04.012
Mang, C. S., Campbell, K. L., Ross, C. J. D., and Boyd, L. A. (2013). Promoting neuroplasticity for motor rehabilitation after stroke: considering the effects of aerobic exercise and genetic variation on brain-derived neurotrophic factor. Phys. Ther. 93, 1707–1716. doi: 10.2522/ptj.20130053
Mansvelder, H. D., Verhoog, M. B., and Goriounova, N. A. (2019). Synaptic plasticity in human cortical circuits: cellular mechanisms of learning and memory in the human brain? Curr. Opin. Neurobiol. 54, 186–193. doi: 10.1016/j.conb.2018.06.013
Maquet, P. (2001). The role of sleep in learning and memory. Science 294, 1048–1052. doi: 10.1126/science.1062856
Marinus, N., Hansen, D., Feys, P., Meesen, R., Timmermans, A., and Spildooren, J. (2019). The impact of different types of exercise training on peripheral blood brain-derived neurotrophic factor concentrations in older adults: a meta-analysis. Sport. Med. 49, 1529–1546. doi: 10.1007/s40279-019-01148-z
Martin, J. H. (2005). The corticospinal system: from development to motor control. Neuroscientist 11, 161–173. doi: 10.1177/1073858404270843
Marzolini, S., Oh, P., McIlroy, W., and Brooks, D. (2013). The effects of an aerobic and resistance exercise training program on cognition following stroke. Neurorehabil. Neural Repair 27, 392–402. doi: 10.1177/1545968312465192
Masino, S. A., and Rho, J. M. (2012). “Mechanisms of ketogenic diet action,” in Jasper’s Basic Mechanisms of the Epilepsies [Internet], 4th Edn, eds J. L. Noebels, M. Avoli, M. A. Rogawski, R. W. Olsen, and A. V. Delgado-Escueta (Bethesda, MD: National Center for Biotechnology Information (US)).
Maswood, N., Young, J., Tilmont, E., Zhang, Z., Gash, D. M., Gerhardt, G. A., et al. (2004). Caloric restriction increases neurotrophic factor levels and attenuates neurochemical and behavioral deficits in a primate model of Parkinson’s disease. Proc. Natl. Acad. Sci. U.S.A. 101, 18171–18176. doi: 10.1073/pnas.0405831102
McCann, J. C., and Ames, B. N. (2005). Is docosahexaenoic acid, an n-3 long-chain polyunsaturated fatty acid, required for development of normal brain function? An overview of evidence from cognitive and behavioral tests in humans and animals. Am. J. Clin. Nutr. 82, 281–295. doi: 10.1093/ajcn.82.2.281
McDonnell, M. N., Buckley, J. D., Opie, G. M., Ridding, M. C., and Semmler, J. G. (2013). A single bout of aerobic exercise promotes motor cortical neuroplasticity. J. Appl. Physiol. 114, 1174–1182. doi: 10.1152/japplphysiol.01378.2012
Molteni, R., Barnard, R. J., Ying, Z., Roberts, C. K., and Gómez-Pinilla, F. (2002). A high-fat, refined sugar diet reduces hippocampal brain-derived neurotrophic factor, neuronal plasticity, and learning. Neuroscience 112, 803–814. doi: 10.1016/S0306-4522(02)00123-9
Mooney, R. A., Coxon, J. P., Cirillo, J., Glenny, H., Gant, N., and Byblow, W. D. (2016). Acute aerobic exercise modulates primary motor cortex inhibition. Exp. Brain Res. 234, 3669–3676. doi: 10.1007/s00221-016-4767-5
Moraes, H., Ferreira, C., Deslandes, A., Cagy, M., Pompeu, F., Ribeiro, P., et al. (2007). Beta and alpha electroencephalographic activity changes after acute exercise. Arq. Neuropsiquiatr. 65, 637–641. doi: 10.1590/S0004-282X2007000400018
Moriarty, T., Bourbeau, K., Bellovary, B., and Zuhl, M. N. (2019). Exercise intensity influences prefrontal cortex oxygenation during cognitive testing. Behav. Sci. (Basel) 9:83. doi: 10.3390/bs9080083
Moriya, M., Aoki, C., and Sakatani, K. (2016). Effects of physical exercise on working memory and prefrontal cortex function in post-stroke patients. Adv. Exp. Med. Biol. 923, 203–208. doi: 10.1007/978-3-319-38810-6_27
Morris, T. P., Fried, P. J., Macone, J., Stillman, A., Gomes-Osman, J., Costa-Miserachs, D., et al. (2020). Light aerobic exercise modulates executive function and cortical excitability. Eur. J. Neurosci. 51, 1723–1734. doi: 10.1111/ejn.14593
Moser, D., Anderer, P., Gruber, G., Parapatics, S., Loretz, E., Boeck, M., et al. (2009). Sleep classification according to AASM and Rechtschaffen and Kales: effects on sleep scoring parameters. Sleep 32, 139–149. doi: 10.1093/sleep/32.2.139
Murphy, T., Dias, G. P., and Thuret, S. (2014). Effects of diet on brain plasticity in animal and human studies: Mind the gap. Neural Plast. 2014:563160. doi: 10.1155/2014/563160
Nagamatsu, L. S., Handy, T. C., Hsu, C. L., Voss, M., and Liu-Ambrose, T. (2012). Resistance training promotes cognitive and functional brain plasticity in seniors with probable mild cognitive impairment. Arch. Intern. Med. 172, 666–668. doi: 10.1001/archinternmed.2012.379
Nanda, B., Balde, J., and Manjunatha, S. (2013). The acute effects of a single bout of moderate-intensity aerobic exercise on cognitive functions in healthy adult males. J. Clin. Diagn. Res. 7, 1883–1885. doi: 10.7860/JCDR/2013/5855.3341
Neva, J. L., Brown, K. E., Mang, C. S., Francisco, B. A., and Boyd, L. A. (2017). An acute bout of exercise modulates both intracortical and interhemispheric excitability. Eur. J. Neurosci. 45, 1343–1355. doi: 10.1111/ejn.13569
Nicolini, C., Michalski, B., Toepp, S. L., Turco, C. V., D’Hoine, T., Harasym, D., et al. (2020). A Single bout of high-intensity interval exercise increases corticospinal excitability, brain-derived neurotrophic factor, and uncarboxylated osteolcalcin in sedentary, healthy males. Neuroscience 437, 242–255. doi: 10.1016/j.neuroscience.2020.03.042
Ohayon, M., Wickwire, E. M., Hirshkowitz, M., Albert, S. M., Avidan, A., Daly, F. J., et al. (2017). National Sleep Foundation’s sleep quality recommendations: first report. Sleep Health 3, 6–19. doi: 10.1016/j.sleh.2016.11.006
Opie, G. M., Catcheside, P. G., Usmani, Z. A., Ridding, M. C., and Semmler, J. G. (2013). Motor cortex plasticity induced by theta burst stimulation is impaired in patients with obstructive sleep apnoea. Eur. J. Neurosci. 37, 1844–1852. doi: 10.1111/ejn.12203
Ota, M., Matsuo, J., Ishida, I., Takano, H., Yokoi, Y., Hori, H., et al. (2018). Effects of a medium-chain triglyceride-based ketogenic formula on cognitive function in patients with mild-to-moderate Alzheimer’s disease. Neurosci. Lett. 690, 232–236. doi: 10.1016/j.neulet.2018.10.048
Özkaya, G. Y., Aydin, H., Toraman, F. N., Kizilay, F., Özdemir, Ö, and Cetinkaya, V. (2005). Effect of strength and endurance training on cognition in older people. J. Sport. Sci. Med. 4:300.
Park, Y. K., Kim, J. H., Choi, S. J., Kim, S. T., and Joo, E. Y. (2019). Altered regional cerebral blood flow associated with mood and sleep in shift workers: cerebral perfusion magnetic resonance imaging study. J. Clin. Neurol. 15, 438–447. doi: 10.3988/jcn.2019.15.4.438
Pascual-Leone, A., Cammarota, A., Wassermann, E. M., Brasil, N. J., Cohen, L. G., Hallett, M., et al. (1993). Modulation of motor cortical outputs to the reading hand of braille readers. Ann. Neurol. 34, 33–37. doi: 10.1002/ana.410340108
Pelletier, A., Barul, C., Féart, C., Helmer, C., Bernard, C., Periot, O., et al. (2015). Mediterranean diet and preserved brain structural connectivity in older subjects. Alzheimer’s Dement. 11, 1023–1031. doi: 10.1016/j.jalz.2015.06.1888
Peters, A., Reisch, C., and Langemann, D. (2018). LTP or LTD? Modeling the influence of stress on synaptic plasticity. Eneuro 5, 1–14.
Petersson, S. D., and Philippou, E. (2016). Mediterranean diet, cognitive function, and dementia: a systematic review of the evidence. Adv. Nutr. 7, 889–904. doi: 10.3945/an.116.012138
Phillips, M. C. L., Murtagh, D. K. J., Gilbertson, L. J., Asztely, F. J. S., and Lynch, C. D. P. (2018). Low-fat versus ketogenic diet in Parkinson’s disease: a pilot randomized controlled trial. Mov. Disord. 33, 1306–1314. doi: 10.1002/mds.27390
Philpott, D. T., Pearcey, G. E., Forman, D., Power, K. E., and Button, D. C. (2015). Chronic resistance training enhances the spinal excitability of the biceps brachii in the non-dominant arm at moderate contraction intensities. Neurosci. Lett. 585, 12–16. doi: 10.1016/j.neulet.2014.11.009
Pifferi, F., Roux, F., Langelier, B., Alessandri, J. M., Vancassel, S., Jouin, M., et al. (2005). (n-3) polyunsaturated fatty acid deficiency reduces the expression of both isoforms of the brain glucose transporter GLUT1 in rats. J. Nutr. 135, 2241–2246. doi: 10.1093/jn/135.9.2241
Placidi, F., Zannino, S., Albanese, M., Romigi, A., Izzi, F., Marciani, M. G., et al. (2013). Increased cortical excitability after selective REM sleep deprivation in healthy humans: a transcranial magnetic stimulation study. Sleep Med. 14, 288–292. doi: 10.1016/j.sleep.2012.11.020
Rajab, A. S., Crane, D. E., Middleton, L. E., Robertson, A. D., Hampson, M., and MacIntosh, B. J. (2014). A single session of exercise increases connectivity in sensorimotor-related brain networks: a resting-state fMRI study in young healthy adults. Front. Hum. Neurosci. 8:625. doi: 10.3389/fnhum.2014.00625
Rajizadeh, M. A., Esmaeilpour, K., Haghparast, E., Ebrahimi, M. N., and Sheibani, V. (2020). Voluntary exercise modulates learning & memory and synaptic plasticity impairments in sleep deprived female rats. Brain Res. 1729:146598. doi: 10.1016/j.brainres.2019.146598
Rizzo, V., Aricò, I., Mastroeni, C., Morgante, F., Liotta, G., Girlanda, P., et al. (2009). Dopamine agonists restore cortical plasticity in patients with idiopathic restless legs syndrome. Mov. Disord. 24, 710–715. doi: 10.1002/mds.22436
Rogge, A. K., Röder, B., Zech, A., and Hötting, K. (2018). Exercise-induced neuroplasticity: Balance training increases cortical thickness in visual and vestibular cortical regions. Neuroimage 179, 471–479. doi: 10.1016/j.neuroimage.2018.06.065
Rolls, A., Colas, D., Adamantidis, A., Carter, M., Lanre-Amos, T., Heller, H. C., et al. (2011). Optogenetic disruption of sleep continuity impairs memory consolidation. Proc. Natl. Acad. Sci. U.S.A. 108, 13305–13310. doi: 10.1073/pnas.1015633108
Rusek, M., Pluta, R., Ułamek-Kozioł, M., and Czuczwar, S. J. (2019). Ketogenic diet in alzheimer’s disease. Int. J. Mol. Sci. 20:3892. doi: 10.3390/ijms20163892
Sagi, Y., Tavor, I., Hofstetter, S., Tzur-Moryosef, S., Blumenfeld-Katzir, T., and Assaf, Y. (2012). Learning in the fast lane: new insights into neuroplasticity. Neuron 73, 1195–1203. doi: 10.1016/j.neuron.2012.01.025
Sahebkar, A., Cicero, A. F., Simental-Mendia, L. E., Aggarwal, B. B., and Gupta, S. C. (2016). Curcumin downregulates human tumor necrosis factor-alpha levels: a systematic review and meta-analysis of randomized controlled trials. Pharmacol. Res. 107, 234–242. doi: 10.1016/j.phrs.2016.03.026
Sandroff, B. M., Hillman, C. H., Benedict, R. H. B., and Motl, R. W. (2015). Acute effects of walking, cycling, and yoga exercise on cognition in persons with relapsing-remitting multiple sclerosis without impaired cognitive processing speed. J. Clin. Exp. Neuropsychol. 37, 209–219. doi: 10.1080/13803395.2014.1001723
Scalise, A., Desiato, M. T., Gigli, G. L., Romigi, A., Tombini, M., Marciani, M. G., et al. (2006). Increasing cortical excitability: a possible explanation for the proconvulsant role of sleep deprivation. Sleep 29, 1595–1598. doi: 10.1093/sleep/29.12.1595
Schmitt, K., Holsboer-Trachsler, E., and Eckert, A. (2016). BDNF in sleep, insomnia, and sleep deprivation. Ann. Med. 48, 42–51. doi: 10.3109/07853890.2015.1131327
Sehm, B., Taubert, M., Conde, V., Weise, D., Classen, J., Dukart, J., et al. (2014). Structural brain plasticity in Parkinson’s disease induced by balance training. Neurobiol. Aging 35, 232–239. doi: 10.1016/j.neurobiolaging.2013.06.021
Sexton, C. E., Betts, J. F., Demnitz, N., Dawes, H., Ebmeier, K. P., and Johansen-Berg, H. (2016). A systematic review of MRI studies examining the relationship between physical fitness and activity and the white matter of the ageing brain. Neuroimage 131, 81–90. doi: 10.1016/j.neuroimage.2015.09.071
Shaafi, S., Najmi, S., Aliasgharpour, H., Mahmoudi, J., Sadigh-Etemad, S., Farhoudi, M., et al. (2016). The efficacy of the ketogenic diet on motor functions in Parkinson’s disease: a rat model. Iran. J. Neurol. 15, 63–69. doi: 10.1093/med/9780197501207.003.0008
Shafer, M. (2020). The Effects of Acute Bouts of Aerobic and Resistance Exercise on Neuroplasticity. Master’s theses. West Chester, PA: West Chester University.
Shah, S. P., and Duda, J. E. (2015). Dietary modifications in Parkinson’s disease: a neuroprotective intervention? Med. Hypotheses 85, 1002–1005. doi: 10.1016/j.mehy.2015.08.018
Sharma, S., Ying, Z., and Gomez-Pinilla, F. (2010). A pyrazole curcumin derivative restores membrane homeostasis disrupted after brain trauma. Exp. Neurol. 226, 191–199. doi: 10.1016/j.expneurol.2010.08.027
Sibley, B. A., Etnier, J. L., and Le Masurier, G. C. (2006). Effects of an acute bout of exercise on cognitive aspects of stroop performance. J. Sport Exerc. Psychol. 28, 285–299. doi: 10.1123/jsep.28.3.285
Siddique, U., Rahman, S., Frazer, A. K., Pearce, A. J., Howatson, G., and Kidgell, D. J. (2020). Determining the sites of neural adaptations to resistance training: a systematic review and meta-analysis. Sport. Med. 50, 1107–1128. doi: 10.1007/s40279-020-01258-z
Singh, A. M., Duncan, R. E., Neva, J. L., and Staines, W. R. (2014). Aerobic exercise modulates intracortical inhibition and facilitation in a nonexercised upper limb muscle. BMC Sports Sci. Med. Rehabil. 6:23. doi: 10.1186/2052-1847-6-23
Singh, A. M., Neva, J. L., and Staines, W. R. (2016). Aerobic exercise enhances neural correlates of motor skill learning. Behav. Brain Res. 301, 19–26. doi: 10.1016/j.bbr.2015.12.020
Singh, R., Lakhanpal, D., Kumar, S., Sharma, S., Kataria, H., Kaur, M., et al. (2012). Late-onset intermittent fasting dietary restriction as a potential intervention to retard age-associated brain function impairments in male rats. Age (Omaha) 34, 917–933. doi: 10.1007/s11357-011-9289-2
Smith, A. E., Dumuid, D., Goldsworthy, M. R., Graetz, L., Hodyl, N., Thornton, N. L., et al. (2021). Daily activities are associated with non-invasive measures of neuroplasticity in older adults. Clin. Neurophysiol. 132, 984–992. doi: 10.1016/j.clinph.2021.01.016
Smith, A. E., Goldsworthy, M. R., Garside, T., Wood, F. M., and Ridding, M. C. (2014). The influence of a single bout of aerobic exercise on short-interval intracortical excitability. Exp. Brain Res. 232, 1875–1882. doi: 10.1007/s00221-014-3879-z
Smith, J. C., Paulson, E. S., Cook, D. B., Verber, M. D., and Tian, Q. (2010). Detecting changes in human cerebral blood flow after acute exercise using arterial spin labeling: Implications for fMRI. J. Neurosci. Methods 191, 258–262. doi: 10.1016/j.jneumeth.2010.06.028
Smith, P. J., Blumenthal, J. A., Hoffman, B. M., Cooper, H., Strauman, T. A., Welsh-Bohmer, K., et al. (2010). Aerobic exercise and neurocognitive performance: a meta-analytic review of randomized controlled trials. Psychosom. Med. 72, 239–252. doi: 10.1097/PSY.0b013e3181d14633
Specterman, M., Bhuiya, A., Kuppuswamy, A., Strutton, P. H., Catley, M., and Davey, N. J. (2005). The effect of an energy drink containing glucose and caffeine on human corticospinal excitability. Physiol. Behav. 83, 723–728. doi: 10.1016/j.physbeh.2004.09.008
Stafstrom, C. E., and Rho, J. M. (2012). The ketogenic diet as a treatment paradigm for diverse neurological disorders. Front. Pharmacol. 3:59. doi: 10.3389/fphar.2012.00059
Statton, M. A., Encarnacion, M., Celnik, P., and Bastian, A. J. (2015). A single bout of moderate aerobic exercise improves motor skill acquisition. PLoS One 10:e0141393. doi: 10.1371/journal.pone.0141393
Stavrinos, E. L., and Coxon, J. P. (2017). High-intensity interval exercise promotes motor cortex disinhibition and early motor skill consolidation. J. Cogn. Neurosci. 29, 593–604. doi: 10.1162/jocn_a_01078
Stee, W., and Peigneux, P. (2021). Post-learning micro- and macro-structural neuroplasticity changes with time and sleep. Biochem. Pharmacol. 191:114369. doi: 10.1016/j.bcp.2020.114369
Stillman, C. M., Cohen, J., Lehman, M. E., and Erickson, K. I. (2016). Mediators of physical activity on neurocognitive function: a review at multiple levels of analysis. Front. Hum. Neurosci. 10:626. doi: 10.3389/fnhum.2016.00626
Stranahan, A. M., Lee, K., Martin, B., Maudsley, S., Golden, E., Cutler, R. G., et al. (2009). Voluntary exercise and caloric restriction enhance hippocampal dendritic spine density and BDNF levels in diabetic mice. Hippocampus 19, 951–961. doi: 10.1002/hipo.20577
Studzinski, C. M., MacKay, W. A., Beckett, T. L., Henderson, S. T., Murphy, M. P., Sullivan, P. G., et al. (2008). Induction of ketosis may improve mitochondrial function and decrease steady-state amyloid-beta precursor protein (APP) levels in the aged dog. Brain Res. 1226, 209–217. doi: 10.1016/j.brainres.2008.06.005
Tallent, J., Goodall, S., Hortobágyi, T., Gibson, A. S. C., and Howatson, G. (2013). Corticospinal responses of resistance-trained and un-trained males during dynamic muscle contractions. J. Electromyogr. Kinesiol. 23, 1075–1081. doi: 10.1016/j.jelekin.2013.04.014
Tallent, J., Woodhead, A., Frazer, A. K., Hill, J., Kidgell, D. J., and Howatson, G. (2021). Corticospinal and spinal adaptations to motor skill and resistance training: potential mechanisms and implications for motor rehabilitation and athletic development. Eur. J. Appl. Physiol. 121, 707–719. doi: 10.1007/s00421-020-04584-2
Thickbroom, G. W., and Mastaglia, F. L. (2009). Plasticity in neurological disorders and challenges for noninvasive brain stimulation (NBS). J. NeuroEng. Rehabil. 6:4. doi: 10.1186/1743-0003-6-4
Tieu, K., Perier, C., Caspersen, C., Teismann, P., Wu, D. C., Yan, S. D., et al. (2003). D-beta-hydroxybutyrate rescues mitochondrial respiration and mitigates features of Parkinson disease. J. Clin. Investig. 112, 892–901. doi: 10.1172/JCI18797
Toepp, S. L., Turco, C. V., Locke, M. B., Nicolini, C., Ravi, R., and Nelson, A. J. (2019). The Impact of glucose on corticospinal and intracortical excitability. Brain Sci. 9:339. doi: 10.3390/brainsci9120339
Tuan, L. H., Tsao, C. Y., Lee, L. J. H., and Lee, L. J. (2021). Voluntary exercise ameliorates synaptic pruning deficits in sleep-deprived adolescent mice. Brain Behav. Immun. 93, 96–110. doi: 10.1016/j.bbi.2020.12.017
Turco, C. V., and Nelson, A. J. (2021). Transcranial magnetic stimulation to assess exercise-induced neuroplasticity. Front. Neuroergonom. 2:17. doi: 10.3389/fnrgo.2021.679033
Van DerAuwera, I., Wera, S., Van Leuven, F., and Henderson, S. T. (2005). A ketogenic diet reduces amyloid beta 40 and 42 in a mouse model of Alzheimer’s disease. Nutr. Metab. 2:28. doi: 10.1186/1743-7075-2-28
van Praag, H., Lucero, M. J., Yeo, G. W., Stecker, K., Heivand, N., Zhao, C., et al. (2007). Plant-derived flavanol (–)epicatechin enhances angiogenesis and retention of spatial memory in mice. J. Neurosci. 27, 5869–5878. doi: 10.1523/JNEUROSCI.0914-07.2007
Vance, D. E., Roberson, A. J., Mcguinness, T. M., and Fazeli, P. L. (2010). How neuroplasticity and cognitive reserve protect cognitive functioning. J. Psychosoc. Nurs. Ment. Health Serv. 48, 23–30. doi: 10.3928/02793695-20100302-01
Vasques, P. E., Moraes, H., Silveira, H., Deslandes, A. C., and Laks, J. (2011). Acute exercise improves cognition in the depressed elderly: The effect of dual-tasks. Clinics 66, 1553–1557. doi: 10.1590/S1807-59322011000900008
Vauzour, D. (2012). Dietary polyphenols as modulators of brain functions: biological actions and molecular mechanisms underpinning their beneficial effects. Oxid. Med. Cell. Longev. 2012:914273. doi: 10.1155/2012/914273
Vilela, T. C., Muller, A. P., Damiani, A. P., Macan, T. P., da Silva, S., Canteiro, P. B., et al. (2017). Strength and aerobic exercises improve spatial memory in aging rats through stimulating distinct neuroplasticity mechanisms. Mol. Neurobiol. 54, 7928–7937. doi: 10.1007/s12035-016-0272-x
Vincent, K. R., Braith, R. W., Bottiglieri, T., Vincent, H. K., and Lowenthal, D. T. (2003). Homocysteine, and lipoprotein levels following resistance training in older adults. Prev. Cardiol. 6, 197–203. doi: 10.1111/j.1520-037X.2003.01723.x
Walker, M. P. (2009). The role of sleep in cognition and emotion. Ann. N. Y. Acad. Sci. 1156, 168–197. doi: 10.1111/j.1749-6632.2009.04416.x
Wang, J., D’Amato, A., Bambrough, J., Swartz, A. M., and Miller, N. E. (2016). A positive association between active lifestyle and hemispheric lateralization for motor control and learning in older adults. Behav. Brain Res. 314, 38–44. doi: 10.1016/j.bbr.2016.07.048
Wickham, S. R., Amarasekara, N. A., Bartonicek, A., and Conner, T. S. (2020). The big three health behaviors and mental health and well-being among young adults: a cross-sectional investigation of sleep, exercise, and diet. Front. Psychol. 11:579205. doi: 10.3389/fpsyg.2020.579205
Wilckens, K. A., Erickson, K. I., and Wheeler, M. E. (2018). Physical activity and cognition: a mediating role of efficient sleep. Behav. Sleep Med. 16, 569–586. doi: 10.1080/15402002.2016.1253013
Willi, S. M., Oexmann, M. J., Wright, N. M., Collop, N. A., and Key, L. L. (1998). The effects of a high-protein, low-fat, ketogenic diet on adolescents with morbid obesity: body composition, blood chemistries, and sleep abnormalities. Pediatrics 101, 61–67. doi: 10.1542/peds.101.1.61
Witte, A. V., Fobker, M., Gellner, R., Knecht, S., and Flöel, A. (2009). Caloric restriction improves memory in elderly humans. Proc. Natl. Acad. Sci. U.S.A. 106, 1255–1260. doi: 10.1073/pnas.0808587106
Wroblewski, J. T., and Danysz, W. (1989). Modulation of glutamate receptors: molecular mechanisms and functional implications. Annu. Rev. Pharmacol. Toxicol. 29, 441–474. doi: 10.1146/annurev.pa.29.040189.002301
Wu, A., Ying, Z., and Gomez-Pinilla, F. (2004). Dietary omega-3 fatty acids normalize BDNF levels, reduce oxidative damage, and counteract learning disability after traumatic brain injury in rats. J. Neurotrauma 21, 1457–1467. doi: 10.1089/neu.2004.21.1457
Wu, A., Ying, Z., and Gomez-Pinilla, F. (2006). Dietary curcumin counteracts the outcome of traumatic brain injury on oxidative stress, synaptic plasticity, and cognition. Exp. Neurol. 197, 309–317. doi: 10.1016/j.expneurol.2005.09.004
Wu, A., Ying, Z., and Gomez-Pinilla, F. (2007). Omega-3 fatty acids supplementation restores mechanisms that maintain brain homeostasis in traumatic brain injury. J. Neurotrauma 24, 1587–1595. doi: 10.1089/neu.2007.0313
Wu, A., Ying, Z., and Gomez-Pinilla, F. (2008). Docosahexaenoic acid dietary supplementation enhances the effects of exercise on synaptic plasticity and cognition. Neuroscience 155, 751–759. doi: 10.1016/j.neuroscience.2008.05.061
Yamazaki, Y., Sato, D., Yamashiro, K., Nakano, S., Onishi, H., and Maruyama, A. (2019). Acute low-intensity aerobic exercise modulates intracortical inhibitory and excitatory circuits in an exercised and a non-exercised muscle in the primary motor cortex. Front. Physiol. 10:1361. doi: 10.3389/fphys.2019.01361
Zagaar, M. A., Dao, A. T., Alhaider, I. A., and Alkadhi, K. A. (2016). Prevention by regular exercise of acute sleep deprivation-induced impairment of late phase LTP and related signaling molecules in the dentate gyrus. Mol. Neurobiol. 53, 2900–2910. doi: 10.1007/s12035-015-9176-4
Zagaar, M., Dao, A., Alhaider, I., and Alkadhi, K. (2013). Regular treadmill exercise prevents sleep deprivation-induced disruption of synaptic plasticity and associated signaling cascade in the dentate gyrus. Mol. Cell. Neurosci. 56, 375–383. doi: 10.1016/j.mcn.2013.07.011
Ziegler, D. R., Ribeiro, L. C., Hagenn, M., Araújo, E., Torres, I. L., Gottfried, C., et al. (2003). Ketogenic diet increases glutathione peroxidase activity in rat hippocampus. Neurochem. Res. 28, 1793–1797. doi: 10.1023/a:1026107405399
Zimmerman, B., Sutton, B. P., Low, K. A., Fletcher, M. A., Tan, C. H., Schneider-Garces, N., et al. (2014). Cardiorespiratory fitness mediates the effects of aging on cerebral blood flow. Front. Aging Neurosci. 6:59. doi: 10.3389/fnagi.2014.00059
Keywords: exercise, diet, sleep, neuroplasticity, aerobic, resistance, lifestyle
Citation: Pickersgill JW, Turco CV, Ramdeo K, Rehsi RS, Foglia SD and Nelson AJ (2022) The Combined Influences of Exercise, Diet and Sleep on Neuroplasticity. Front. Psychol. 13:831819. doi: 10.3389/fpsyg.2022.831819
Received: 08 December 2021; Accepted: 25 March 2022;
Published: 26 April 2022.
Edited by:
Anjali Sivaramakrishnan, The University of Texas Health Science Center at San Antonio, United StatesReviewed by:
Carla Silva-Batista, University of São Paulo, BrazilCopyright © 2022 Pickersgill, Turco, Ramdeo, Rehsi, Foglia and Nelson. This is an open-access article distributed under the terms of the Creative Commons Attribution License (CC BY). The use, distribution or reproduction in other forums is permitted, provided the original author(s) and the copyright owner(s) are credited and that the original publication in this journal is cited, in accordance with accepted academic practice. No use, distribution or reproduction is permitted which does not comply with these terms.
*Correspondence: Aimee J. Nelson, bmVsc29uYWpAbWNtYXN0ZXIuY2E=
†These authors have contributed equally to this work
Disclaimer: All claims expressed in this article are solely those of the authors and do not necessarily represent those of their affiliated organizations, or those of the publisher, the editors and the reviewers. Any product that may be evaluated in this article or claim that may be made by its manufacturer is not guaranteed or endorsed by the publisher.
Research integrity at Frontiers
Learn more about the work of our research integrity team to safeguard the quality of each article we publish.