- 1Environmental Ergonomics Laboratory, Department of Kinesiology, Brock University, St. Catharines, ON, Canada
- 2N2M Consulting Inc., St. Catharines, ON, Canada
- 3Department of Physical and Health Education, Nipissing University, North Bay, ON, Canada
Increases in body temperature from heat stress (i.e., hyperthermia) generally impairs cognitive function across a range of domains and complexities, but the relative contribution from skin versus core temperature changes remains unclear. Hyperthermia also elicits a hyperventilatory response that decreases the partial pressure of end-tidal carbon dioxide (PetCO2) and subsequently cerebral blood flow that may influence cognitive function. We studied the role of skin and core temperature along with PetCO2 on cognitive function across a range of domains. Eleven males completed a randomized, single-blinded protocol consisting of poikilocapnia (POIKI, no PetCO2 control) or isocapnia (ISO, PetCO2 maintained at baseline levels) during passive heating using a water-perfused suit (water temperature ~ 49°C) while middle cerebral artery velocity (MCAv) was measured continuously as an index of cerebral blood flow. Cognitive testing was completed at baseline, neutral core-hot skin (37.0 ± 0.2°C-37.4 ± 0.3°C), hot core-hot skin (38.6 ± 0.3°C-38.7 ± 0.2°C), and hot core-cooled skin (38.5 ± 0.3°C-34.7 ± 0.6°C). The cognitive test battery consisted of a detection task (psychomotor processing), 2-back task (working memory), set-shifting and Groton Maze Learning Task (executive function). At hot core-hot skin, poikilocapnia led to significant (both p < 0.05) decreases in PetCO2 (∆−21%) and MCAv (∆−26%) from baseline, while isocapnia clamped PetCO2 (∆ + 4% from baseline) leading to a significantly (p = 0.023) higher MCAv (∆−18% from baseline) compared to poikilocapnia. There were no significant differences in errors made on any task (all p > 0.05) irrespective of skin temperature or PetCO2 manipulation. We conclude that neither skin temperature nor PetCO2 maintenance significantly alter cognitive function during passive hyperthermia.
Introduction
Elevations in core temperature (i.e., hyperthermia) increases physiological (e.g., cardiovascular, metabolic), psychological (e.g., thermal discomfort), and neurological (e.g., central processing) strain relative to thermoneutral environments and can lead to impairments in cognitive function (Hancock et al., 2007; Taylor et al., 2016; Schmit et al., 2017). Thermal stress is proposed to induce an inverted-U response with cognitive function, where either heating or cooling beyond a narrow optimal range causes a progressive impairment (Hancock and Vasmatzidis, 1998; Liu et al., 2013). The magnitude of impairment from thermal stress may also be task-dependent, where higher-order cognitive tasks (e.g., executive function, vigilance, working memory) or those requiring motor coordination are more vulnerable to impairment compared to where simple task performance (e.g., psychomotor processing) (Gaoua et al., 2011; Piil et al., 2017).
Cognitive changes may happen well before major changes in whole-body temperature, as changes in skin temperature may alter arousal or distraction, or increase cognitive workload through dual-tasking between performing the cognitive task and increasing effort from monitoring of thermal state and discomfort (Hancock et al., 2007). With heat stress, higher skin temperature with no or just minor elevations in core temperature increased thermal perception and discomfort, along with eliciting more errors (Gaoua et al., 2012) and slower reaction times (Malcolm et al., 2018) on executive function tasks. Skin cooling has been shown to increase variability in detection time during prolonged vigilance tasks almost immediately upon cold water immersion, with no further decrement upon either −0.5°C or − 1.0°C rectal temperature decrease (Cheung et al., 2007). However, high levels of expertise may offset impairment from cold skin or core, as a military simulation of vigilance did not report any decrements over nearly 3 h of cold exposure (0°C air in addition to 5°C water circulating through a water-perfused suit) compared to thermoneutral (22°C air) in trained soldiers (Tikuisis and Keefe, 2007). Overall, the lack of consensus about temperature effects on cognitive function may arise from a lack of control and isolation of skin versus core temperature changes. Based on the potential influence of skin temperature as an independent factor, it is of interest to tease out the relative contribution of skin versus core temperature influences on cognitive function across a range of task domains and complexities.
Another potential mechanism influencing cognitive function during heat stress is changes in arterial CO2, as hyperthermic hypocapnia (reduced CO2) increases cerebrovascular resistance and decreases cerebral blood flow (CBF; Ainslie et al., 2005; Al-Khazraji et al., 2019). In thermoneutral environments, hyperventilation-induced hypocapnia impaired executive function (Stroop task) through slowed reaction time and increased error rate (Van Diest et al., 2000), while clamping of end-tidal carbon dioxide (PetCO2) to eucapnic levels during hypoxic stress (isocapnic hypoxia) countered impairments in psychomotor processing reaction time due to hypoxia-induced hypocapnia (Friend et al., 2019). However, countering hyperventilatory-induced hypocapnia during high-intensity exercise (~80% peak maximal oxygen consumption) through the maintenance of PetCO2 and middle cerebral artery velocity (MCAv) did not prevent declines in executive function performance (inhibitory control and spatial working memory; Komiyama et al., 2019). Gibbons et al. (2020) reported that maintaining PetCO2 at eucapnic levels during passive hyperthermia (∆ + 1.5°C in Tcore) did not affect changes in reaction time on a simple psychomotor and inhibition task (Gibbons et al., 2020). However, it remains unknown if manipulating PetCO2 influences higher-order cognitive tasks (executive function, working memory) during passive heat exposure.
The purpose of this study was to investigate the role of skin versus core temperature with passive hyperthermia on cognitive function. This was done by eliciting four distinct thermal states: baseline (BASE, neutral core and skin), Neutral Core–Hot Skin (NC-HS), Hot Core–Hot Skin (HC-HS), and Hot Core–Cooled Skin (HC-CS). To isolate the potential confounding role of end-tidal carbon dioxide due to hyperthermic hyperventilation, trials were performed under poikilocapnia (no PetCO2 manipulation) or with isocapnia (maintenance of PetCO2 at eucapnic levels). We hypothesized that (i) cognitive performance would be impaired with moderate hyperthermia and predominantly driven by skin rather than core temperature, (ii) cognitive decrements would be greater in poikilocapnia than isocapnia due to a hyperthermia-induced hypocapnia.
Materials and Methods
The experimental protocol was approved by the Bioscience Research Ethics Board at Brock University (REB 17–385) and conformed to the latest revision of the Declaration of Helsinki. Eleven healthy male volunteers (for participant characteristics see Table 1), who were free from cardiovascular, respiratory, and neurological disorders were recruited from the university and community population; all participants were screened by a physician and then provided informed written consent.
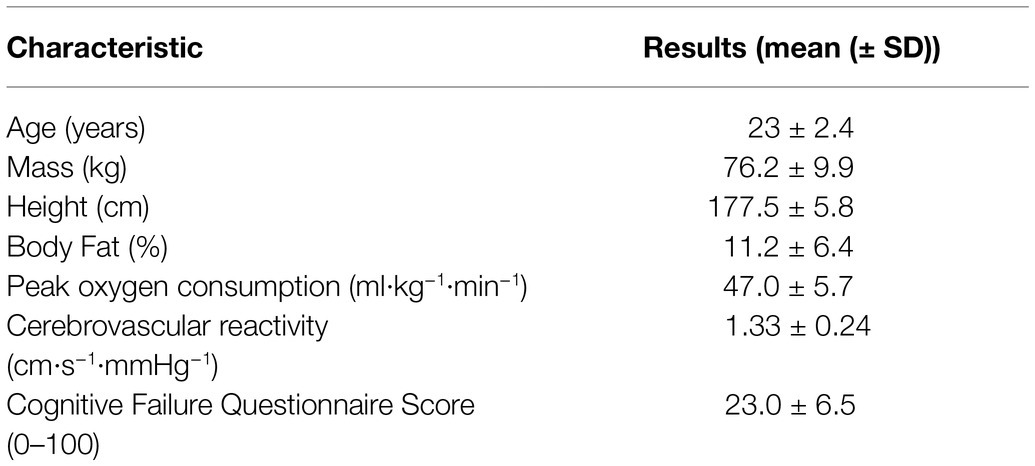
Table 1. The mean (± SD) participant (n = 11) characteristics collected during the preliminary assessment.
Experimental Design
The experiment implemented a randomized crossover design consisting of a familiarization trial and 2 experimental trials (Figure 1, see details below). Experimental trials were separated by at least one week to reduce the potential for heat acclimation and were performed at the same time of day for each participant to control for circadian fluctuations in core temperature. Participants were instructed to avoid vigorous exercise and alcohol consumption for 24 h, caffeine for 12 h prior to each experimental session, and to follow their typical meal and hydration practices 24 h prior to each session. Randomization was performed online (random.org) and participants were blinded to the trial type being performed.
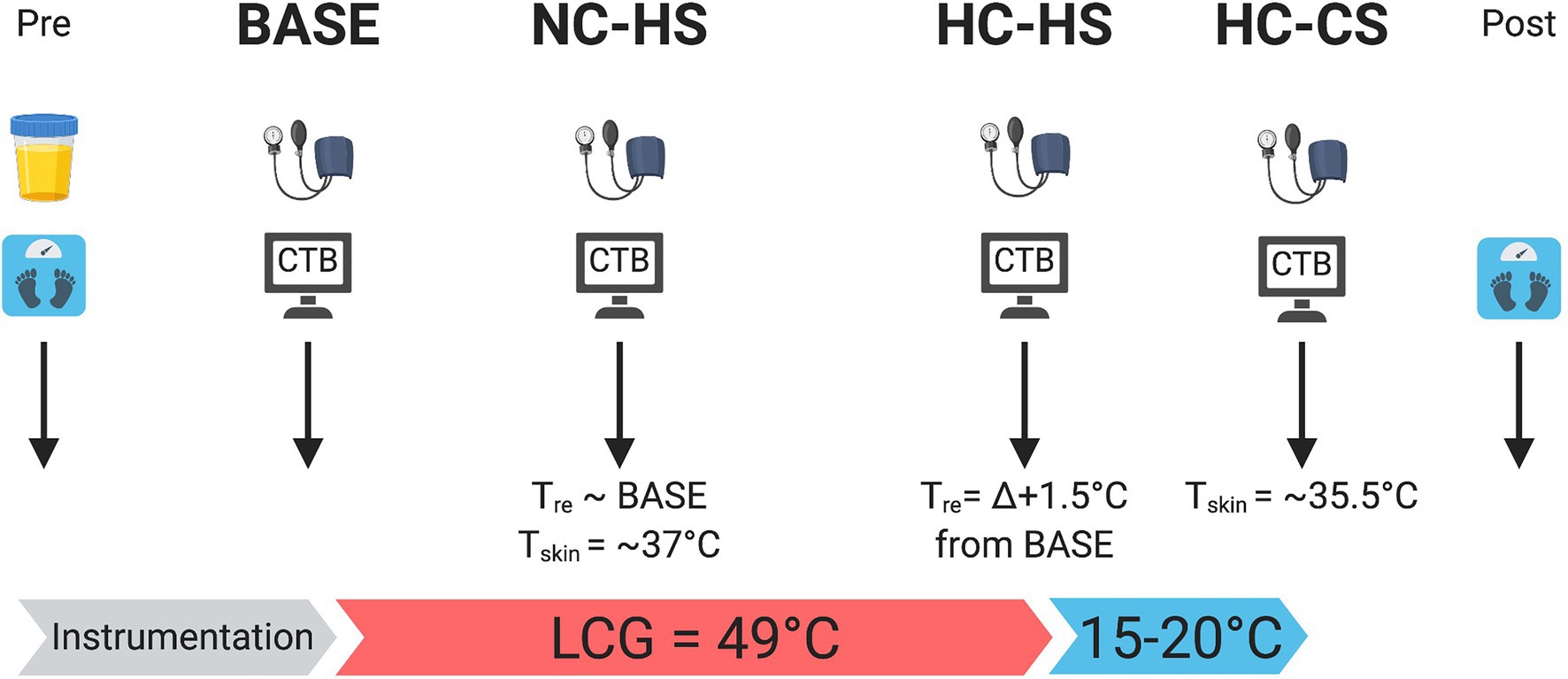
Figure 1. Schematic of experimental protocol. At each experimental time-point, participant’s blood pressure was measured followed by performing the CTB. Body mass was recorded pre and post trial. Urine specific gravity was measured pre trial. Created with BioRender.com.
Familiarization Trial
Upon arrival to the laboratory, anthropometric measurements (height, mass) and body fat percentage (calculated using the 7-site skinfold technique) were recorded. Participants then completed the Cognitive Failure Questionnaire, which is a 25-item questionnaire that is a self-evaluative measure of general fluid intelligence and related to four factors of absentmindness (memory, distractibility, blunders, and names; Broadbent et al., 1982) where total scores can range between 0 to 100 and the average score is between 19 and 35. Participants were excluded from the study if their score was ≥45, as this score indicates considerable difficulties in completing tasks that require vigilance and may be easily distracted, which could potentially influence the cognitive function data. Of 12 participants initially recruited, one was excluded based on Cognitive Failure Questionnaire score and did not perform the experimental assessments. Next, cerebrovascular reactivity to CO2 was assessed through using a custom-built end-tidal forcing system. The cerebrovascular reactivity assessment protocol comprised a 5-min baseline (eucapnia), a subsequent 5-min at hypercapnia (+10 mmHg from eucapnia) followed by 5-min at hypocapnia (−10 mmHg from eucapnia), where reactivity was determined by the change in CBF per change in PetCO2. Next, participants performed a cognitive test battery (CTB; see details below) in a thermoneutral environment (~22°C, 30% RH) for a total of 3 times to reduce the possibility of a learning effect from repeated exposures (Wallace et al., 2017, 2021). Lastly, an incremental test to exhaustion was performed on a cycle ergometer (Velotron, RacerMate Inc., United States) to determine peak oxygen consumption. The test began with a standardized 5-min warm-up at 100 W, followed by workload increase of 25 W each minute until exhaustion. Peak oxygen consumption was defined as the highest 30-s value measured breath by breath from expired gases collected through a soft silicone facemask connected to an online gas collection system.
Thermal Manipulations
Upon arrival, participants voided their bladder and nude body mass (kg) was recorded. A sample of the urine was tested for urine specific gravity (PAL-10S, Atago, Japan) to determine hydration status. Participants were considered euhydrated if USG was ≤1.020, or else the test was rescheduled (ultimately, no trials were rescheduled from hypohydration). Participants were then instrumented and fitted with a two-piece liquid conditioning garment (BCS 4 Cooling System, Med Eng, Canada) consisting of 1/8″ diameter Tygon tubing sewn into a stretchable jacket and pant; the head, hands and feet uncovered. Participants then rested in a semi-recumbent seated position (~5-min) and a baseline measure of PetCO2 was recorded. Next participants performed the BASE measure of the CTB (see details below) in a thermoneutral room (~22°C, 30% RH) with no temperature manipulation. Following BASE, participants were fitted with a polyvinyl rain suit and thermal blanket over the liquid conditioning garment with the hands and head uncovered to minimize evaporative heat loss for the rest of the heating protocol.
Similar to a previous study (Wallace et al., 2021), four time-points were tested manipulating both physiological and perceptual thermal strain (Figure 2). The first time-point was BASE where no temperature manipulation occurred. Next, to delineate the sensory displeasure of hot skin separate from increases in core temperature, ~49.0°C water was circulated at 2.5 l·min−1 through the liquid cooling garment and the next CTB was performed once a mean skin temperature ( ) of ~37.0°C was achieved, creating the NC-HS time-point. To test the effects of hyperthermia on cognitive performance, passive heat stress was continued until there was a rise in rectal temperature by ~∆ + 1.5°C creating a HC-HS time-point; a ∆ + 1.5°C change in Tre was used to ensure a sufficient thermal strain to induce a hyperventilatory hypocapnia response. Additionally, based on pilot work and unpublished reports, our passive heating protocol has interindividual variability in thermal tolerance >38.5°C and led to alterations in ventilatory breathing patterns causing difficulties to clamp PetCO2. Therefore, a ∆ + 1.5°C ensured thermal tolerance between all participants and allowed for better control of PetCO2 using the end-tidal forcing system. Lastly, upon completion of the CTB, to test the effects of hyperthermia without sensory displeasure of hot skin, ~15–20°C water was circulated through the liquid conditioning garment until of 35.5°C while minimizing changes in core temperature, creating the HC-CS time-point.
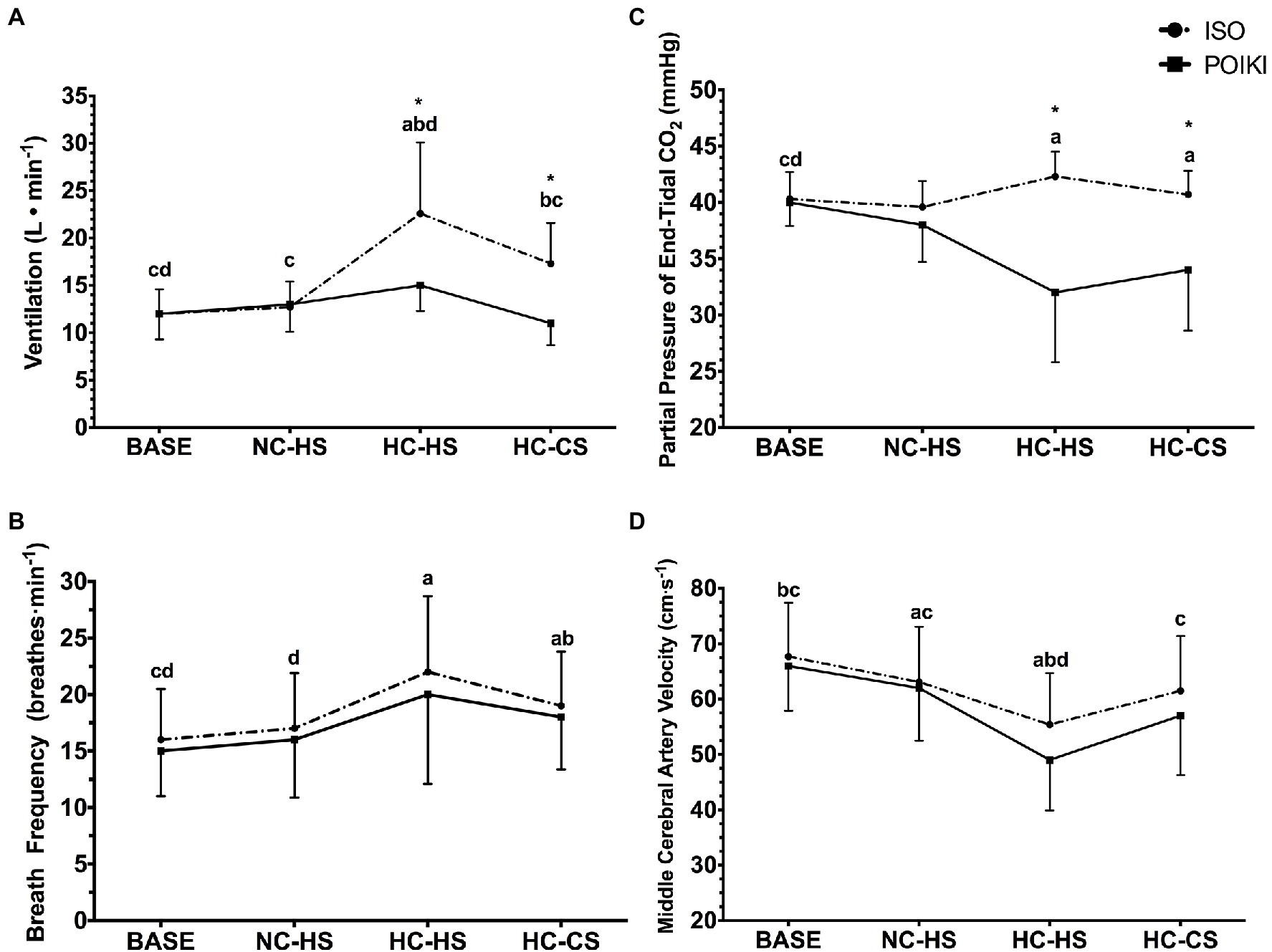
Figure 2. Ventilation [ Panel (A)], Breath Frequency [ Panel (B)], Partial Pressure of End Tidal Carbon Dioxide [PetCO2; Panel (C)], and Middle Cerebral Artery Velocity [MCAv; Panel (D)] responses (presented as mean ± SD) for the four experimental time-points. For significant time-point effects (p ≤ 0.05), significant (p ≤ 0.05) Bonferroni pairwise comparisons can be interpreted as: a significantly different from BASE, b significantly different from NC-HS, c significantly different from HC-HS, d significantly different from HC-CS. For condition effects, * indicates significant (p < 0.05) differences between ISO and POIKI at that specific time-point.
PetCO2 Manipulations
To determine the role of PetCO2 on cognitive function during passive heat stress, participants performed identical experimental trials that differed only based on inspired gas manipulation: i) a poikilocapnic (POIKI) condition where PetCO2 was recorded but not manipulated; and ii) an isocapnic (ISO) condition where PetCO2 was clamped to eucapnic levels determined at BASE. Participants were fitted with a soft silicone facemask (Hans Rudolph, United States) attached to a T-shaped two-way non-rebreathing valve connected to an online gas collection system (ML206 Gas Analyzer, AD Instruments; United States). Measures of inspired ventilation ( L·min−1) and breathing frequency ( breaths·min−1), were measured using a pneumotach. Inspired and expired fractions of CO2 were sampled at 1 kHz to determine breath-by-breath PetCO2 and were sampled through nafion tubing in a desiccant-packed drying chamber at a flow rate of 200 ml·min−1. A custom built end-tidal forcing system (for details see: Hartley et al., 2016) was used to manipulate PetCO2 throughout the experimental trials by manipulating inspired CO2 concentration to ‘force’ PetCO2 towards the desired value. Gas concentrations were controlled through solenoid valves which independently controlled gas flow from cylinders of compressed medical grade breathing air (20.93% oxygen, 0.03% CO2, balance nitrogen), and 100% CO2. Inspired air volumes were delivered to an air reservoir (~5 l) via a humidification chamber (~500 ml). Both temperature and humidity of the gas was regulated to replicate ambient room conditions through the end-tidal forcing system.
As a separate quality control for determining the accuracy and signal drift of our gas analyzer over the course of the experimental duration, CO2% and ventilation volume were tested every 60-min over a 180-min period on one participant under thermoneutral conditions using ventilation rates of 10–20 l·min−1. The average CO2 signal drift was ∆ + 0.01 ± 0.01% CO2 compared to room air, ∆–0.01 ± 0.01% CO2 compared to the calibration gas, and volume was ∆ + 0.01 ± 0.025 l over the 180-min period. Overall, minimal CO2 signal drift over the course of the experimental trials (< 3 h in duration) ensuring accuracy of the end-tidal forcing system in clamping PetCO2 throughout the experimental protocol.
Instrumentation
Before the commencement of BASE, participants were instrumented with a flexible thermistor (Mon-A-Therm Core, Mallinkrodt Medical, United States), self-inserted 15 cm beyond the anal sphincter to measure rectal temperature (Tre) sampled at 4 Hz. Four thermocouples (VC-T-24-190 Omega Environmental Inc., Canada) were used to determine weighted at four sites defined as on the right side of the body sampled at 4 Hz. Heart rate was calculated using R-R intervals using a standard three-lead electrocardiogram (MLA2340, AD Instruments; United States). Bi-lateral middle cerebral artery velocity (MCAv) was assessed using a 2 MHz pulsed transcranial Doppler ultrasound system (Doppler-Box, Compumedics GmbH, Germany). The probes were positioned over the temporal window and were held in place using a secure and comfortable head frame (M600 Headframe, Spencer Technologies, United States). To identify and optimize signals, positioning of the probes were determined by using techniques described by Willie et al. (2011). Data was visually inspected and removed, post-hoc, if significant artifacts were detected (e.g., movement during CTB) or poor signal waveform quality. Systolic blood pressure (SBP) and diastolic blood pressure (DBP) were taken from a manual sphygmomanometer (Aneroid Sphygmomanometer, Welch Allyn Hillrom, United States) on their left arm by the same experienced researcher before the CTB at each time-point. Mean arterial pressure (MAP) was calculated as: . All physiological data (except MAP) were continuously sampled throughout the experiment and are the average over the course of the entire CTB. Subjective assessments of the environmental conditions were assessed using a 1–4 scale to measure thermal comfort and a 1–7 scale for thermal sensation (Gagge et al., 1967) and were collected upon the completion of the CTB at BASE, NC-HS, HC-HS, and HC-CS.
Cognitive Assessment
To measure progressive changes in cognitive performance, a ~ 12-15 min customized CTB (CogState, United States) was performed at BASE, NC-HS, HC-HS, and HC-CS. This customized CTB focused on the assessment of executive function and working memory. Executive function is an umbrella term for a collection of higher-order cognitive processes and is the ability to plan and execute behavior while being able to dynamically update goals, store and acquire information in working memory, switch among tasks, inhibit behaviors, process errors and being able to perform these behaviors in changing environments (McCabe et al., 2010). Multiple tested were chosen to encapsulate different components of executive function in order to determine potential task-dependent changes in cognitive function. The CTB consisted of a Groton Maze Learning Task (GMLT), a detection task, a two-back task, a set-shifting task, and a GMLT recall task. The order of the tasks was identical CTB was performed. However, the stimuli in each task was randomized to ensure similar task difficulty each time the CTB was performed. For each task, participants were instructed to respond as quickly and accurately as they could.
The GMLT is a touch screen-based test that measures executive function through error detection and spatial memory. The test consists of a hidden 28-step pathway on a 10 × 10 grid of squares. A blue tile on the top left corner of the screen indicates the starting location while a red circle on the bottom right indicates the finish location. The GMLT is performed five times per test and is randomized and matched for difficulty on every trial assessment to minimize learning effects. Performance changes were measured for the total duration (s) and total number of errors (#) measured during the five-block period and for the last maze (GMLT 5). A GMLT recall test (GLMT–Recall) was performed at the end of each CTB, which required the participant to recall the same hidden pathway from the initial five-block period. The total testing period took ~5–7-min.
A detection task was used to test psychomotor reaction time. A face down card was presented on the screen and the participant was required to press a key as soon as the card was turned over. This process continued until the task is completed with 35 cards being presented with a 2 s interval in between. Performance was measured for speed (mean of the log10 transformed reaction times for correct responses) and for total number of errors (#). The task required ~2-min to complete.
A 2-back test was used to measure of attention and visual working memory. This test required the participant to determine if the card being presented is identical to the card presented two cards ago. There was a total of 48 cards presented and the participant could select either “yes” or “no” for each card. Performance for this task was measured for speed of processing (mean of the log10 transformed reaction times for correct responses) and total number of errors (#). The task required ~2-min to complete.
A set-shifting task was used measure of cognitive flexibility. In this test, the participant was asked to answer the question “is this the target card?.” The participant was presented with a playing card in the center of the screen with either the word “number” or “colour” above it and had to select “yes” or “no.” The only feedback presented to the participant was that the next card would not be displayed until the correct response is made. The target card changes throughout the test which could be either from one colour to the other (i.e., from a red target card to a black target card or intra-dimensional shift) or from “colour” to “number” (i.e., from a red target card to a number two target card or extra-dimensional shift). The participant was not told when these changes occurred and needed to re-learn the new target card to continue with the test. Performance was measured based on speed of processing (mean of the log10 transformed reaction times for correct responses) and total number of errors (#). The task required ~3-min to complete.
Statistical Analyses
All continuous variable data are presented as the mean ± SD averaged over each time-point while completing the CTB. All physiological and cognitive variables were analyzed using separate condition (ISO vs. POIKI) x time-point (BASE, NC-HS, HC-HS, HC-CS) repeated measures ANOVAs. If data violated sphericity (Mauchley’s test, p < 0.05) the Greenhouse–Geisser was used. A Bonferroni post-hoc analysis was used to test significant timepoint main effects. Pre and Post body mass was assessed using a condition (ISO x POIKI) by time (PRE x POST) repeated measures ANOVA. If a significant condition x timepoint interaction was calculation, paired sample t tests were used to test the main effects at specific time-points (e.g., BASE vs. BASE) to determine if there was a condition effect. To reduce the likelihood of Type I error due to multiple comparison, the α value was revised based on number comparisons (2, i.e., HC-HS POIKI verus HC-HS ISO), therefore p = 0.025 for paired samples t test comparisons.
All ordinal data (Thermal Comfort, Thermal Sensation) is presented as the median (quartiles 1 and 3) and were analyzed using separate condition (ISO vs. POIKI) x time-point (BASE, NC-HS, HC-HS, HC-CS) repeated measures ANOVAs, with a Wilcoxon signed-rank test used to compare at specific time points if a condition effect was present. All analyses were performed using IBM SPSS Statistics for Windows (version 26.0; IBM Corp., Armonk, N.Y., United States).
Results
Experimental Manipulation
The experimental design was successful in creating four distinct temperature and perceptual time-points (Table 2). There was a time-point effect for Tre (Table 2; p < 0.001, ηp2 = 1.00), where pairwise comparisons determined Tre was significantly higher in the HC-HS and HC-CS compared to BASE and NC-HS (all p ≤ 0.001). Pairwise comparisons demonstrated no differences in Tre between BASE and NC-HS (p = 1.00), but a significant difference compared to either HC-HS and HC-CS (both p < 0.05). There was a significant conditon effect (p = 0.014, ηp2 = 0.766) and time-point effect for T̅skin (Table 2; p < 0.001, ηp2 = 1.00), where was significantly different at all time-points (all p ≤ 0.001). There was a significant timepoint effect for Thermal Comfort (p ≤ 0.001, ηp2 = 1.00); where NC-HS (p = 0.001) HC-HS (p ≤ 0.001), but not HC-CS (p = 0.057) were different from BASE; there was no Thermal Comfort difference between NC-HS and HC-CS (p = 0.296). Additionally, there was a significant time-point effect for Thermal Sensation (p ≤ 0.001, ηp2 = 1.00), where pairwise comparisons demonstrate differences between each time-point (all p < 0.05).
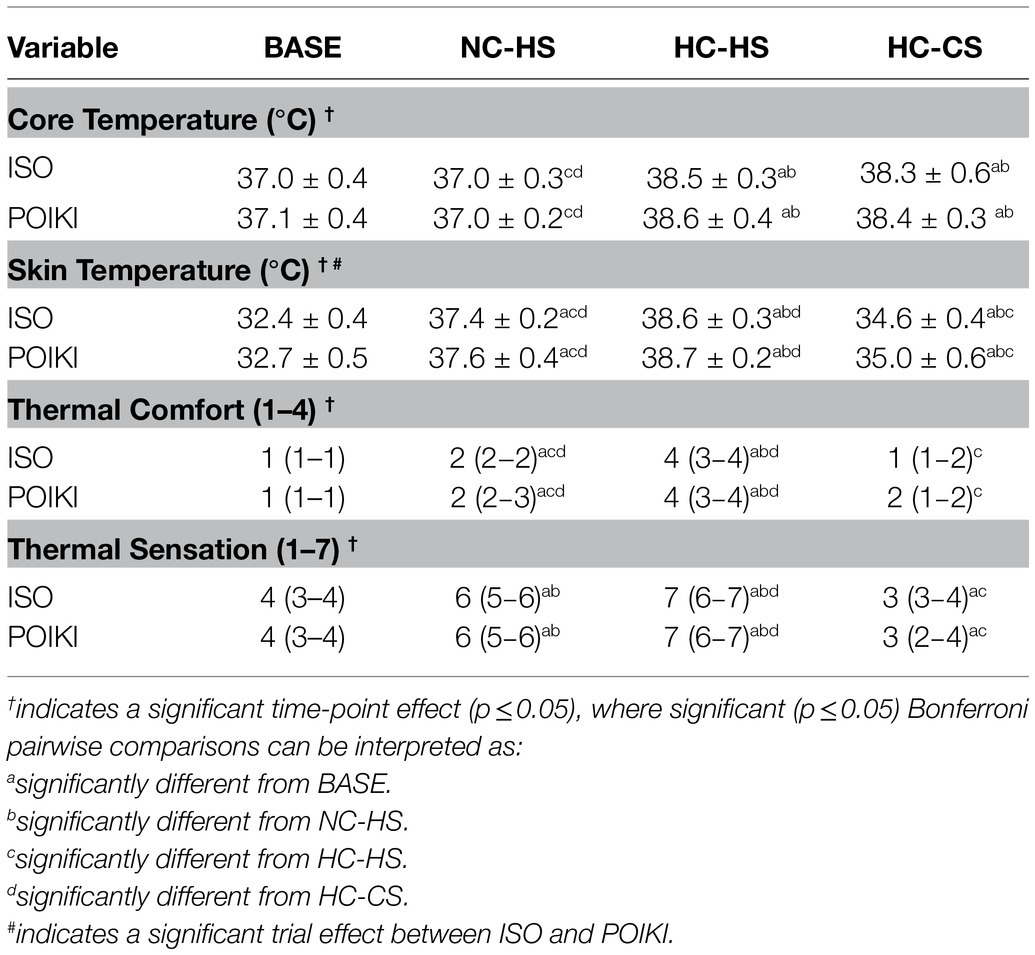
Table 2. Physiological (presented as mean ± SD) and perceptual (presented as quartiles 1 and 3) for the four experimental time-points.
Hydration and Body Mass
Urine specific gravity prior to the experimental trials was <1.020 and was not different between conditions (p = 0.138, ISO: 1.008 ± 0.006, POIKI: 1.011 ± 0.006). There was a significant time-point effect (p ≤ 0.001) for body mass from PRE (ISO: 76.2 ± 10.1 kg, POIKI: 76.2 ± 9.9 kg) to POST (ISO: 74.5 ± 10.2 kg ~ −2.0% loss, POIKI: 74.7 ± 10.1 kg, ~ −2.0% loss) with no differences between conditions (p = 0.640).
Respiratory Responses
There was a significant time-point effect for (p ≤ 0.001, ηp2 = 0.999), condition (p = 0.005, ηp2 = 0.894), and condition x time-point interaction (p = 0.001, ηp2 = 0.964; Figure 2A). Pairwise comparisons revealed a hyperthermia-induced hyperventilatory response where significantly increased from BASE in both HC-HS (p = 0.002) and HC-CS (p = 0.034). HC-HS was significantly higher than all other time-points (all p < 0.05), while there were no differences between NC-HS and BASE (p = 0.961) or NC-HS and HC-CS (p = 0.105). Paired samples t-tests indicated a significant difference in between ISO and POIKI at HC-HS (ISO: 22.6 ± 7.5 l·min−1, POIKI: 15.3 ± 2.7 l·min−1, p = 0.005) and HC-CS (ISO: 17.3 ± 4.3 l·min−1, POIKI: 11.2 ± 2.3 l·min−1, p = 0.002). Similarly, demonstrated a significant time-point (p = 0.007, ηp2 = 0.853) and condition (p = 0.03, ηp2 = 0.628) effect, with a non-significant condition × time-point effect (p = 0.619, ηp2 = 0.089; Figure 2B). Pairwise comparisons revealed that compared to BASE, was significantly higher at HC-HS (p = 0.021) and HC-CS (p ≤ 0.001). Furthermore, HC-CS was significantly higher than NC-HS (p = 0.006), with no other significant differences between conditions (all p > 0.05).
There was a significant condition (p = 0.002, ηp2 = 0.952), time-point (p = 0.002, ηp2 = 0.985), and condition x time-point interaction (p ≤ 0.001, ηp2 = 0.999) for PetCO2 (Figure 2C). Pairwise comparisons demonstrated that PetCO2 was significantly different from BASE at HC-HS (p = 0.01) and HC-CS (p = 0.022), but not NC-CS (p = 0.09). Paired samples t-tests demonstrated there was a significant difference between ISO and POIKI at HC-HS (ISO: 42.3 ± 2.2 mmHg, POIKI: 31.7 ± 6.2 mmHg, p = 0.001) and HC-CS (ISO: 40.7 ± 2.1 mmHg, POIKI: 34.2 ± 5.4 mmHg, p = 0.004), but not BASE (ISO: 40.3 ± 2.4 mmHg, POIKI: 39.9 ± 2.1 mmHg, p = 0.515), or NC-CS (ISO: 39.6 ± 2.3 mmHg, POIKI: 37.9 ± 3.3 mmHg, p = 0.203). At HC-HS, the relative change from BASE was ~ + 4.0% in ISO and ~ −26.0% in POIKI.
Cerebral Hemodynamic Responses
Due to technical issues, data for MCAv was limited to n = 8. Within this reduced dataset, there was a significant time-point effect (p = 0.006, ηp2 = 0.907), condition (p = 0.013, ηp2 = 0.790), but not condition x time-point interaction (p = 0.493, ηp2 = 0.198) for MCAv (Figure 2D). Pairwise comparisons demonstrated that, compared to BASE, MCAv was significantly different at NC-HS (p = 0.014) and HC-HS (p = 0.003), but not HC-CS (p = 0.216). HC-HS was significantly lower than all other time-points (all p < 0.05). NC-HS was not significantly different than HC-CS (p = 1.000). Values for MCAv were BASE (ISO: 67.7 ± 9.7 cm·s−1, POIKI: 66.3 ± 8.1 cm·s−1), NC-HS (ISO: 63.1 ± 10.0 cm·s−1, POIKI: 61.5 ± 9.5 cm·s−1), HC-HS (ISO: 55.4 ± 9.3 cm·s−1, POIKI: 48.6 ± 9.1 cm·s−1), and HC-CS (ISO: 61.0 ± 10.4 cm·s−1, POIKI: 57.0 ± 10.7 cm·s−1).
Cardiovascular Responses
Due to poor signal quality of electrocardiogram, one participant’s data was removed from the heart rate analyses (n = 10). A significant time-point effect was seen for heart rate (Figure 3A; p < 0.001, ηp2 = 1.00) with no condition (p = 0.512, ηp2 = 0.094) or interaction (p = 0.062, ηp2 = 0.600). Pairwise comparisons demonstrated that heart rate was significantly different from each other at all time-points (all p < 0.05). There was no condition (p = 0.759, ηp2 = 0.059), time-point (p = 0.473, ηp2 = 0.214), or condition x time-point interaction (p = 0.264, ηp2 = 0.331) for MAP (Figure 3B). There was a significant time-point effect for SBP (Figure 3C, p ≤ 0.001, ηp2 = 1.00), with no condition (p = 0.322, ηp2 = 0.157) or condition x time-point interaction (p = 0.06, ηp2 = 0.589). Pairwise comparisons revealed SBP was significantly higher at HC-HS compared to all other time-point (p ≤ 0.001), while there were no differences between the other time-point (all p = 1.000). For DBP (Figure 3D), the time-point effect approached significance (p = 0.052, ηp2 = 0.769), however pairwise comparisons demonstrated no differences between any time-point (all p > 0.05). There was no condition (p = 0.738, ηp2 = 0.061) or condition × time-point interaction (p = 0.268, ηp2 = 0.318) for DBP.
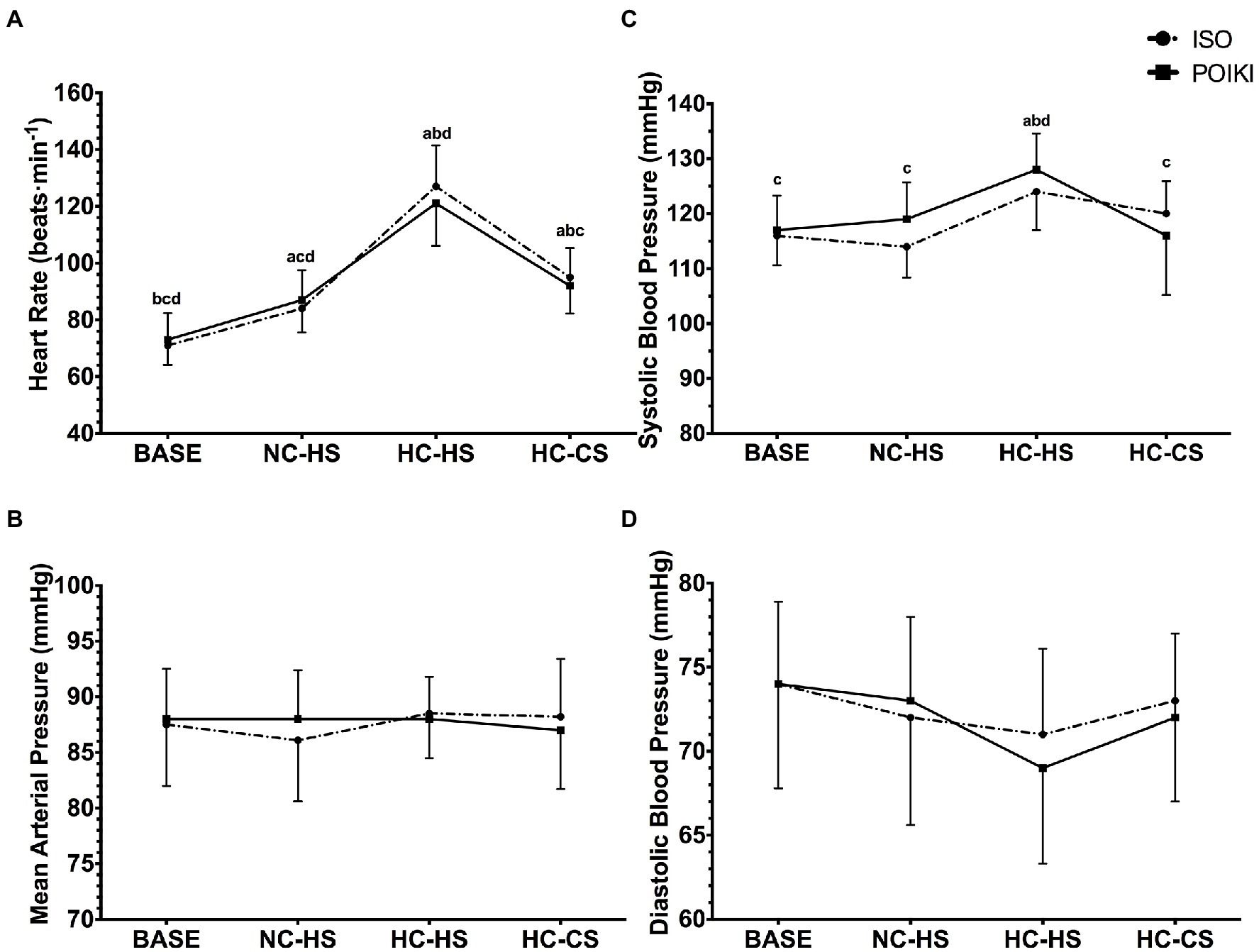
Figure 3. Heart rate Panel (A) and Blood Pressure (Mean Arterial Pressure Panel (B), Systolic Blood Pressure Panel (C), and Diastolic Blood Pressure Panel (D)) responses (presented as mean ± SD) for the four experimental time-points. For significant time-point effects (p ≤ 0.05), significant (p ≤ 0.05) Bonferroni pairwise comparisons can be interpreted as: a significantly different from BASE, b significantly different from NC-HS, c significantly different from HC-HS, d significantly different from HC-CS.
Cognitive Performance
GMLT
For the learning portion of the GMLT (i.e., GMLT-1 through 5), there was a significant time-point effect for duration (p = 0.017, ηp2 = 0.780), however pairwise comparisons revealed no difference between any time-point (all p > 0.05, Table 3A). There was no time-point (p = 0.854, ηp2 = 0.094) effect for number of errors made on the learning portion of the GMLT (Table 3A). For GMLT-5 duration (Table 3B), there was a time-point effect (p = 0.046, ηp2 = 0.650), however pairwise comparisons revealed no differences between specific time-points (all p > 0.05). There was no condition or condition x time-point interaction (both p > 0.05). For GMLT-5 errors (Table 3B), there was no time-point (p = 0.861, ηp2 = 0.092) or condition x time-point interaction (p = 0.981, ηp2 = 0.059) but there was a condition effect (p = 0.037, ηp2 = 0.585). For the GMLT-Recall (Table 3C), there were no condition, time-point, or condition x time-point interaction for both duration (all p > 0.05) and # of errors made (all p > 0.05).
Set-Shifting Task
There was a significant time-point effect for speed (p ≤ 0.001, ηp2 = 0.999), where participants were significantly faster at HC-HS compared to BASE (p = 0.005), and NC-HS (p = 0.008) but not HC-CS (p = 0.125; Table 3D). There were no differences between BASE and HC-CS (p = 0.055) for set-shifting speed. There was no condition (p = 0.901, ηp2 = 0.052) or condition x time-point interaction (p = 0.675, ηp2 = 0.142) for set-shifting speed. There was no condition, time-point, or condition x time-point interaction (all p > 0.05) for # of errors made on the set-shifting task (Table 3D).
2-Back Task
There was no condition (p = 0.896, ηp2 = 0.052), time-point (p = 0.061, ηp2 = 0.604), or condition x time-point interaction (p = 0.257, ηp2 = 0.337) for speed (Table 3E). There were no time-point (p = 0.155, ηp2 = 0.437), or condition x time-point interaction (p = 0.538, ηp2 = 0.188), with a condition (p = 0.025, ηp2 = 0.660) effect for # of errors on the 2-Back Task (Table 3E).
Detection Task
There was a significant time-point effect for Detection Task speed (p ≤ 0.001, ηp2 = 1.00), where participants were significantly faster at HC-HS and HC-CS compared to BASE (p = 0.008 and 0.039 respectively) and NC-HS (p = 0.005 and 0.021 respectively; Table 3F). There was no condition (p = 0.190, ηp2 = 0.247) or condition x time-point interaction (p = 0.712, ηp2 = 0.131) for Detection Task speed.
Discussion
This study tested the effect of changes in skin temperature, core temperature, and end-tidal CO2 (PetCO2) on executive function, working memory, and psychomotor processing. We hypothesized that cognitive performance would decrease with an increase in skin temperature and thermal discomfort, before significant core temperature elevation with continued decrements with increase in core temperature. Additionally, we hypothesized that maintaining PetCO2 and cerebral blood flow would restore some of the heat stress-induced cognitive impairment. Using our test protocol, we demonstrated that neither changes in skin nor core temperature impaired accuracy on any measured cognitive variables. However, hyperthermia (independent of skin temperature) led to faster psychomotor processing, with decreased reaction time on both the detection and set-shifting task. Furthermore, although PetCO2 was successfully clamped in ISO to slightly above BASE eucapnic levels (∆ + 2 mmHg), we found no benefit from isocapnia and partial restoration of MCAv on cognitive performance throughout our heating protocol.
While many studies report an impairment in cognitive function with whole-body hyperthermia, this finding is not universal. Recent studies with passive or active hyperthermia to ∆ + 1.3–2.0°C in core temperature have not led to decrements in errors for executive function, working memory, or visual perception performance (Schlader et al., 2013; Wallace et al., 2017, 2021; van den Heuvel et al., 2017). Similarly, we found no significant increases in errors made on any of the CTB tasks, but instead significantly faster reaction times on the detection task (psychomotor processing) and set-shifting task (executive function, inhibitory control). Our target hyperthermia of +1.5°C Tre was chosen as a compromise between being high enough to elicit hyperthermic hyperventilation versus sufficient capacity to control PetCO2 with our end-tidal forcing system. It is possible that a core temperature threshold of ≥39°C is needed before cognitive impairments occur (Schmit et al., 2017), such that the absolute thermal strain (Tre ~ 38.5–38.6°C) in our, and other null-finding studies, may have been insufficient. It is also possible that individual variability in thermal perception and tolerance may exist due to aerobic fitness and heat acclimation status, body composition, and neurotransmitter concentrations (e.g., dopamine; Cheung and Sleivert, 2004a), which may have influenced cognitive performance during heat stress. Future work is needed to determine the core temperature threshold for hyperthermic impairment and individual factors influencing cognitive function under thermal strain.
Thermal displeasure from sudden alterations in can impair cognitive function independent of core temperature changes, with skin cooling the primary driver for vigilance impairments compared to core cooling (Cheung et al., 2007). With heat stress, Gaoua et al. (2012) proposed that an increase in can lead to a speed-accuracy trade-off in complex tasks with a faster response time and higher error incidence. Our study aimed to dissociate skin from core temperature effects by targeting testing at timepoints where skin temperature sharply deviated from core temperature (NC-HS and HC-CS). However, an increase in skin temperature by ~5°C and thermal comfort to ‘slightly uncomfortable’ (NC-HS condition) did not affect performance of complex cognitive tasks, nor did the skin cooling of ~4°C and dropping of thermal comfort back to baseline levels of “comfortable” (HC-CS) compared to the typically-studied thermal state of neutral core and skin (Base) or hot core and hot skin (HC-HS, thermal comfort “very uncomfortable”). These results are similar to previous findings testing executive function (inhibitory control) performance where there were no differences in task performance or P300 amplitude between hyperthermia (~∆ + 1.3°C) and hyperthermia with face cooling despite improvements in thermal comfort (Shibasaki et al., 2017). These studies contrast with our previous research using a similar skin/core dissociation protocol to study neuromuscular function, where we have shown that skin temperature is the dominant driver of isokinetic maximal force production with knee extension (Cheung and Sleivert, 2004b) and electromyographic amplitude during a dynamic position task (Coletta et al., 2018). Similarly, regional surface cooling with neck cold collars improved thermal comfort and were sufficient to increase voluntary exercise capacity in hot environments despite no core temperature changes (Tyler and Sunderland, 2011). Overall our data suggests that, unlike in the physiological realm, thermal perception changes driven by skin temperature do not impact cognitive function.
Carbon dioxide levels have a primary influence on CBF (Ainslie et al., 2005) and in physiological systems such as neuromuscular capacity in thermoneutral and hot environments (Ross et al., 2012; Hartley et al., 2016), which may have extended into a role for PetCO2 changes during passive heating on cognitive function. The ISO condition, which controlled PetCO2 to baseline levels throughout heating despite perturbations of skin or core temperature, demonstrated similar increases in psychomotor processing and executive function (set-shifting) reaction time without a speed accuracy trade-off with moderate hyperthermia (irrespective of skin temperature) as the POIKI condition with no differences between other tasks. These findings demonstrate that changes in PetCO2 or MCAv did not alter cognitive performance at moderate hyperthermia levels, and is in line with recent work demonstrating that restoration of PetCO2. Additionally, MCAv did not counter the decline in executive function (inhibitory control and spatial working memory) performance during high-intensity exercise (Komiyama et al., 2019) or psychomotor processing during passive hyperthermia at sea-level and altitude (Gibbons et al., 2020). Similar to our previous investigations using active hyperthermia (Wallace et al., 2017), we found no decrements in accuracy on executive function (inhibitory control, spatial working memory) or working memory task performance with a ∆ + 1.5°C increase in Tre. However, in the current study, we did demonstrate an increased psychomotor speed with hyperthermia (irrespective of skin temperature) indicating faster encoding which may have influenced higher-order cognitive function (Racinais et al., 2008). As PetCO2 has been demonstrated to improve neuromuscular capacity, future studies should determine if PetCO2 plays a role in complex motor based cognitive tasks (Piil et al., 2017).
Cerebral blood flow is tightly regulated by arterial CO2, pH, MAP, cerebral metabolism and autonomic nervous system function (for reviews see: Willie et al., 2014; Bain et al., 2015). We showed that moderate hyperthermia (HC-HS, ∆ + 1.5°C in Tre) conditions led to a hyperventilatory hypocapnic response (~∆−8 mmHg) with a decrease in MCAv by ~26% in POIKI. Although PetCO2 was successfully ‘clamped’ just above eucapnic levels leading to significantly higher MCAv compared to POIKI, the hyperventilatory response remained and MCAv was still reduced ~18% relative to thermoneutral baseline. These results are in line with previous investigations (Fujii et al., 2008; Brothers et al., 2009; Ross et al., 2012) but are in contrast to studies that successfully restored MCAv to baseline levels (Nelson et al., 2011; Bain et al., 2013). These discrepancies may be due to our ‘clamping’ PetCO2 continuously throughout heating versus its restoration only upon reaching hyperthermia (Ross et al., 2012; Bain et al., 2013), our moderate versus severe hyperthermia (Ross et al., 2012; Bain et al., 2013), or the semi-recumbent position used in the current study versus supine postures (Nelson et al., 2011; Bain et al., 2013; Favre et al., 2020). Additionally, ISO demonstrated an exacerbated response during both HC-HS and HC-CS time-points compared to POIKI. The response likely occurred due to the addition of inspiratory CO2 by the end-tidal forcing system increasing the overall inspiratory volume. Conversely, the trend for a higher (~1–2 breaths·min−1) at HC-HS with ISO may indicate that the additional CO2 increased minute ventilation by stimulating the respiratory centres in the brain (Nattie, 1999). There is the potential that the additional inspiratory volume may have interfered with neural processing and potentially influencing cognitive function during the ISO condition. However, there were no condition or condition x timepoint interactions between the ISO and POIKI for any of the cognitive variables. However, future research is needed to determine the role of PetCO2 and ventilation on neural processing (using such tools as electroencephalography) during heat stress. Lastly, this study is limited by not having a measure of intracranial pressure or cerebral perfusion, which are influenced by changes in MAP and vascular resistance (for review see: Numan et al., 2014). Recently, Shoemaker et al., (2019) determined that, under hypercapnia in thermoneutral conditions, CO2 is the primary driver for changes in MCAv as opposed to MAP changes that occur during acute mental stress caused by performing a subtraction task. In the current study, it is unlikely that there were differences in cerebral perfusion as MAP remained relatively unchanged throughout heating, as well as participants remained in the semi-recumbent posture throughout heating to reduce the impact of positional changes on MAP and cerebral perfusion (Nelson et al., 2011; Numan et al., 2014).
A strength of our research experiment was the use of an end-tidal forcing system that allowed us to accurately clamp PetCO2 (~1–4% from BASE) breath-by-breath for the entirety of the ISO trial (Figure 1). This method provides an accurate control of PetCO2 as an indirect measure of arterial PCO2, where PetCO2 has been demonstrated to accurately estimate arterial PCO2 throughout passive hyperthermia up to +2.0°C (Brothers et al., 2009; Ross et al., 2012; Bain et al., 2013). Breath-by-breath clamping of PetCO2 is more advantageous compared to CO2 bolus techniques because, once hypocapnic, the CBF responses is slower to respond to changes in CO2 (Poulin et al., 1998; Ross et al., 2012). Therefore, with use of the end-tidal forcing system, we prevented hypocapnia in order to control CBF. A limitation of our study was the use of TCD to measure MCAv as an index representation of CBF, which is a valid relationship if assuming the diameter of the MCA does not change (Ainslie and Hoiland, 2014). Direct measurement of the MCA using magnetic resonance imaging determined that the MCA dilates during 10 min of hypercapnia (~48 mmHg PetCO2) and constricts during hypocapnia (~31 mmHg PetCO2) in thermoneutral conditions (Al-Khazraji et al., 2019). We clamped PetCO2 throughout the ISO trial to BASE levels which may have minimized vasodilation of cerebrovascular vessels. Additionally, we cannot account for local CBF changes in the executive attention network and prefrontal cortex, which are important neural regions for the executive function and working memory during passive hyperthermia (Liu et al., 2013; Qian et al., 2013). Furthermore, a limitation of the study is there was no decrement in cognitive performance with manipulation of skin and core temperature. Future research is needed with a cognitive test battery where cognitive function is impaired in order to determine if PetCO2 can counter decline in performance. Lastly, the results of this study are limited to males as no females were tested to account for fluctuations in core temperature due to the menstrual cycle. Under thermoneutral conditions, the evidence is mixed on whether there are sex-differences in cerebrovascular reactivity (Favre et al., 2020). Future evidence is needed to determine if there are sex-related differences in cognition, changes in PetCO2, and CBF regulation during passive heat stress.
In summary, the results from our study showed that an increase in both skin and core temperature and thermal discomfort or reduction in MCAv did not impair cognitive function during a passive heating protocol when learning effect is controlled. However, the results did indicate that hyperthermia decreased reaction time on the detection task as well as the set-shifting task. In addition, our results showed a reduction in MCAv regardless of the maintenance of baseline PetCO2 during hyperthermia suggesting that hyperthermia-induced changes in CBF may have other controlling factors, such as cardiovascular control or skin temperature. Future research is needed to determine how longer exposure and/or different modes of heat stress and hyperthermia may affect cognition.
Data Availability Statement
The raw data supporting the conclusions of this article will be made available by the authors, without undue reservation.
Ethics Statement
The studies involving human participants were reviewed and approved by Brock University Biosciences Research Ethics Board. The patients/participants provided their written informed consent to participate in this study.
Author Contributions
RM, PW, GH, MT, and SC conceived and designed the study. RM, PW, JS, and SS collected the data. PW, RM, GH, JS, and SS reduced and analysed the data. PW, RM, and SC drafted the manuscript. JS, SS, GH, and MT edited the manuscript. All authors contributed to the article and approved the submitted version.
Funding
This study was supported by a Discovery grant from the Natural Science and Engineering Research Council (NSERC) of Canada (SSC, 2018–04077). RM was supported through an Ontario Graduate Scholarship and PW was supported through a NSERC Doctoral (PGS D) scholarship.
Conflict of Interest
MT is employed by N2M Consulting Inc.
The remaining authors declare that the research was conducted in the absence of any commercial or financial relationships that could be construed as a potential conflict of interest.
Publisher’s Note
All claims expressed in this article are solely those of the authors and do not necessarily represent those of their affiliated organizations, or those of the publisher, the editors and the reviewers. Any product that may be evaluated in this article, or claim that may be made by its manufacturer, is not guaranteed or endorsed by the publisher.
Acknowledgments
We thank the participants for volunteering their time and effort to take part in the study.
References
Ainslie, P. N., Ashmead, J. C., Ide, K., Morgan, B. J., and Poulin, M. J. (2005). Differential responses to CO2 and sympathetic stimulation in the cerebral and femoral circulations in humans: blood flow responses to sympathetic stimulation and CO2 in humans. J. Physiol. 566, 613–624. doi: 10.1113/jphysiol.2005.087320
Ainslie, P. N., and Hoiland, R. L. (2014). Transcranial Doppler ultrasound: valid, invalid, or both? J. Appl. Physiol. 117, 1081–1083. doi: 10.1152/japplphysiol.00854.2014
Al-Khazraji, B. K., Shoemaker, L. N., Gati, J. S., Szekeres, T., and Shoemaker, J. K. (2019). Reactivity of larger intracranial arteries using 7 T MRI in young adults. J. Cereb. Blood Flow Metab. 39, 1204–1214. doi: 10.1177/0271678X18762880
Bain, A. R., Nybo, L., and Ainslie, P. N. (2015). “Cerebral Vascular Control and Metabolism in Heat Stress,” in Comprehensive Physiology, ed. G. R. Terjung (Hoboken, NJ, USA: John Wiley & Sons, Inc.), 1345–1380. doi: 10.1002/cphy.c140066
Bain, A. R., Smith, K. J., Lewis, N. C., Foster, G. E., Wildfong, K. W., Willie, C. K., et al. (2013). Regional changes in brain blood flow during severe passive hyperthermia: effects of PaCO2 and extracranial blood flow. J. Appl. Physiol. 115, 653–659. doi: 10.1152/japplphysiol.00394.2013
Broadbent, D. E., Cooper, P. F., FitzGerald, P., and Parkes, K. R. (1982). The Cognitive Failures Questionnaire (CFQ) and its correlates. Br. J. Clin. Psychol. 21, 1–16. doi: 10.1111/j.2044-8260.1982.tb01421.x
Brothers, R. M., Wingo, J. E., Hubing, K. A., and Crandall, C. G. (2009). The effects of reduced end-tidal carbon dioxide tension on cerebral blood flow during heat stress: cerebrovascular blood flow during heat stress. J. Physiol. 587, 3921–3927. doi: 10.1113/jphysiol.2009.172023
Cheung, S. S., and Sleivert, G. G. (2004a). Multiple triggers for hyperthermic fatigue and exhaustion. Exerc. Sport Sci. Rev. 32, 100–106. doi: 10.1097/00003677-200407000-00005
Cheung, S. S., and Sleivert, G. G. (2004b). Lowering of skin temperature decreases isokinetic maximal force production independent of core temperature. Eur. J. Appl. Physiol. 91, 723–728. doi: 10.1007/s00421-004-1062-0
Cheung, S. S., Westwood, D. A., and Knox, M. K. (2007). Mild body cooling impairs attention via distraction from skin cooling. Ergonomics 50, 275–288. doi: 10.1080/00140130601068683
Coletta, N. A., Mallette, M. M., Gabriel, D. A., Tyler, C. J., and Cheung, S. S. (2018). Core and skin temperature influences on the surface electromyographic responses to an isometric force and position task. PLoS One 13:e0195219. doi: 10.1371/journal.pone.0195219
Favre, M. E., Lim, V., Falvo, M. J., and Serrador, J. M. (2020). Cerebrovascular reactivity and cerebral autoregulation are improved in the supine posture compared to upright in healthy men and women ed. Mogi M. PLOS ONE 15:e0229049. doi: 10.1371/journal.pone.0229049
Friend, A. T., Balanos, G. M., and Lucas, S. J. E. (2019). Isolating the independent effects of hypoxia and hyperventilation-induced hypocapnia on cerebral haemodynamics and cognitive function. Exp Physiol EP087602. 104, 1482–1493. doi: 10.1113/EP087602
Fujii, N., Honda, Y., Hayashi, K., Kondo, N., Koga, S., and Nishiyasu, T. (2008). Effects of chemoreflexes on hyperthermic hyperventilation and cerebral blood velocity in resting heated humans: Hyperthermic hyperpnoea and chemoreflex drive, cerebral circulation. Exp. Physiol. 93, 994–1001. doi: 10.1113/expphysiol.2008.042143
Gagge, A. P., Stolwijk, J. A. J., and Hardy, J. D. (1967). Comfort and thermal sensations and associated physiological responses at various ambient temperatures. Environ. Res. 1, 1–20. doi: 10.1016/0013-9351(67)90002-3
Gaoua, N., Grantham, J., Racinais, S., and El Massioui, F. (2012). Sensory displeasure reduces complex cognitive performance in the heat. J. Environ. Psychol. 32, 158–163. doi: 10.1016/j.jenvp.2012.01.002
Gaoua, N., Racinais, S., Grantham, J., and Massioui, F. E. (2011). Alterations in cognitive performance during passive hyperthermia are task dependent. Int. J. Hyperth. 27, 1–9. doi: 10.3109/02656736.2010.516305
Gibbons, T. D., Tymko, M. M., Thomas, K. N., Wilson, L. C., Stembridge, M., Caldwell, H. G., et al. (2020). Global REACH 2018: The influence of acute and chronic hypoxia on cerebral haemodynamics and related functional outcomes during cold and heat stress. J. Physiol. 598, 265–284. doi: 10.1113/JP278917
Hancock, P. A., Ross, J. M., and Szalma, J. L. (2007). A meta-analysis of performance response Under thermal stressors. Hum Factors J Hum Factors Ergon Soc 49, 851–877. doi: 10.1518/001872007X230226
Hancock, P. A., and Vasmatzidis, I. (1998). Human occupational and performance limits under stress: the thermal environment as a prototypical example. Ergonomics 41, 1169–1191. doi: 10.1080/001401398186469
Hartley, G. L., Watson, C. L., Ainslie, P. N., Tokuno, C. D., Greenway, M. J., Gabriel, D. A., et al. (2016). Corticospinal excitability is associated with hypocapnia but not changes in cerebral blood flow: cerebral blood flow and hypocapnia on corticospinal excitability. J. Physiol. 594, 3423–3437. doi: 10.1113/JP271914
Komiyama, T., Tanoue, Y., Sudo, M., Costello, J. T., Uehara, Y., Higaki, Y., et al. (2019). Cognitive impairment during high-intensity exercise: influence of cerebral blood flow. Med. Sci. Sports Exerc. 1. doi: 10.1249/MSS.0000000000002183
Liu, K., Sun, G., Li, B., Jiang, Q., Yang, X., Li, M., et al. (2013). The impact of passive hyperthermia on human attention networks: An fMRI study. Behav. Brain Res. 243, 220–230. doi: 10.1016/j.bbr.2013.01.013
Malcolm, R. A., Cooper, S., Folland, J. P., Tyler, C. J., and Sunderland, C. (2018). Passive heat exposure alters perception and executive function. Front. Physiol. 9. doi: 10.3389/fphys.2018.00585
McCabe, D. P., Roediger, H. L., McDaniel, M. A., Balota, D. A., and Hambrick, D. Z. (2010). The relationship between working memory capacity and executive functioning: evidence for a common executive attention construct. Neuropsychology 24, 222–243. doi: 10.1037/a0017619
Nattie, E. (1999). CO2, brainstem chemoreceptors and breathing. Prog. Neurobiol. 59, 299–331. doi: 10.1016/S0301-0082(99)00008-8
Nelson, M. D., Haykowsky, M. J., Stickland, M. K., Altamirano-Diaz, L. A., Willie, C. K., Smith, K. J., et al. (2011). Reductions in cerebral blood flow during passive heat stress in humans: partitioning the mechanisms: Cerebral blood flow during passive heat stress. J. Physiol. 589, 4053–4064. doi: 10.1113/jphysiol.2011.212118
Numan, T., Bain, A. R., Hoiland, R. L., Smirl, J. D., Lewis, N. C., and Ainslie, P. N. (2014). Static autoregulation in humans: a review and reanalysis. Med. Eng. Phys. 36, 1487–1495. doi: 10.1016/j.medengphy.2014.08.001
Piil, J. F., Lundbye-Jensen, J., Trangmar, S. J., and Nybo, L. (2017). Performance in complex motor tasks deteriorates in hyperthermic humans. Temperature 4, 420–428. doi: 10.1080/23328940.2017.1368877
Poulin, M. J., Liang, P.-J., and Robbins, P. A. (1998). Fast and slow components of cerebral blood flow response to step decreases in end-tidal PaCO2 in humans. J. Appl. Physiol. 85, 388–397. doi: 10.1152/jappl.1998.85.2.388
Qian, S., Sun, G., Jiang, Q., Liu, K., Li, B., Li, M., et al. (2013). Altered topological patterns of large-scale brain functional networks during passive hyperthermia. Brain Cogn. 83, 121–131. doi: 10.1016/j.bandc.2013.07.013
Racinais, S., Gaoua, N., and Grantham, J. (2008). Hyperthermia impairs short-term memory and peripheral motor drive transmission. J. Physiol. 586, 4751–4762. doi: 10.1113/jphysiol.2008.157420
Ross, E. Z., Cotter, J. D., Wilson, L., Fan, J.-L., Lucas, S. J. E., and Ainslie, P. N. (2012). Cerebrovascular and corticomotor function during progressive passive hyperthermia in humans. J. Appl. Physiol. 112, 748–758. doi: 10.1152/japplphysiol.00988.2011
Schlader, Z. J., Lucas, R. A. I., Pearson, J., and Crandall, C. G. (2013). Hyperthermia does not alter the increase in cerebral perfusion during cognitive activation: cerebral perfusion during cognitive activation. Exp. Physiol. 98, 1597–1607. doi: 10.1113/expphysiol.2013.074104
Schmit, C., Hausswirth, C., Le Meur, Y., and Duffield, R. (2017). Cognitive functioning and heat strain: performance responses and protective strategies. Sports Med. 47, 1289–1302. doi: 10.1007/s40279-016-0657-z
Shibasaki, M., Namba, M., Oshiro, M., Kakigi, R., and Nakata, H. (2017). Suppression of Cognitive Function in Hyperthermia; From the viewpoint of executive and inhibitive cognitive processing. Sci. Rep. 7. doi: 10.1038/srep43528
Shoemaker, L. N., Wilson, L. C., Lucas, S. J. E., Machado, L., and Cotter, J. D. (2019). Cerebrovascular regulation is not blunted during mental stress. Exp. Physiol. 104, 1678–1687. doi: 10.1113/EP087832
Taylor, L., Watkins, S. L., Marshall, H., Dascombe, B. J., and Foster, J. (2016). The impact of different environmental conditions on cognitive function: A focused review. Front. Physiol. 6. doi: 10.3389/fphys.2015.00372
Tikuisis, P., and Keefe, A. A. (2007). Effects of cold strain on simulated sentry duty and marksmanship. Aviat. Space Environ. Med. 78, 399–407.
Tyler, C. J., and Sunderland, C. (2011). Neck cooling and running performance in the heat: single versus repeated application. Med. Sci. Sports Exerc. 43, 2388–2395. doi: 10.1249/MSS.0b013e318222ef72
van den Heuvel, A. M. J., Haberley, B. J., Hoyle, D. J. R., Taylor, N. A. S., and Croft, R. J. (2017). The independent influences of heat strain and dehydration upon cognition. Eur. J. Appl. Physiol. 117, 1025–1037. doi: 10.1007/s00421-017-3592-2
Van Diest, I., Stegen, K., Van de Woestijne, K. P., Schippers, N., and Van den Bergh, O. (2000). Hyperventilation and attention: effects of hypocapnia on performance in a Stroop task. Biol. Psychol. 53, 233–252. doi: 10.1016/S0301-0511(00)00045-4
Wallace, P. J., Martins, R. S., Scott, J. S., Steele, S. W., Greenway, M., and Cheung, S. S. (2021). The effects of acute dopamine reuptake inhibition on cognitive function during passive hyperthermia. Appl. Physiol. Nutr. Metab. apnm. 46, 511–520. doi: 10.1139/apnm-2020-0869
Wallace, P. J., McKinlay, B. J., Coletta, N. A., Vlaar, J. I., Taber, M. J., Wilson, P. M., et al. (2017). Effects of motivational self-talk on endurance and cognitive performance in the heat. Med. Sci. Sports Exerc. 49, 191–199. doi: 10.1249/MSS.0000000000001087
Willie, C. K., Colino, F. L., Bailey, D. M., Tzeng, Y. C., Binsted, G., Jones, L. W., et al. (2011). Utility of transcranial Doppler ultrasound for the integrative assessment of cerebrovascular function. J. Neurosci. Methods 196, 221–237. doi: 10.1016/j.jneumeth.2011.01.011
Keywords: passive hyperthermia, cognitive function, isocapnia, end-tidal carbon dioxide, clamping, middle cerebral artery velocity, executive function, working memory
Citation: Schultz Martins R, Wallace PJ, Steele SW, Scott JS, Taber MJ, Hartley GL and Cheung SS (2021) The Clamping of End-Tidal Carbon Dioxide Does Not Influence Cognitive Function Performance During Moderate Hyperthermia With or Without Skin Temperature Manipulation. Front. Psychol. 12:788027. doi: 10.3389/fpsyg.2021.788027
Edited by:
Antonio Hernández-Mendo, University of Malaga, SpainReviewed by:
Tomomi Fujimoto, Niigata University of Health and Welfare, JapanJose María Carames Tejedor, University of Edinburgh, United Kingdom
Copyright © 2021 Schultz Martins, Wallace, Steele, Scott, Taber, Hartley and Cheung. This is an open-access article distributed under the terms of the Creative Commons Attribution License (CC BY). The use, distribution or reproduction in other forums is permitted, provided the original author(s) and the copyright owner(s) are credited and that the original publication in this journal is cited, in accordance with accepted academic practice. No use, distribution or reproduction is permitted which does not comply with these terms.
*Correspondence: Stephen S. Cheung, scheung@brocku.ca
†These authors have contributed equally to this work and share first authorship