- 1Institute of Neuroscience and Psychology, University of Glasgow, Glasgow, United Kingdom
- 2School of Psychology, University of Glasgow, Glasgow, United Kingdom
Transcranial electrical stimulation (tES) is being investigated as an experimental and clinical interventional technique in human participants. While promising, important limitations have been identified, including weak effect sizes and high inter- and intra-individual variability of outcomes. Here, we compared two “inhibitory” tES-techniques with supposedly different mechanisms of action as to their effects on performance in a visuospatial attention task, and report on a direct replication attempt. In two experiments, 2 × 20 healthy participants underwent tES in three separate sessions testing different protocols (10 min stimulation each) with a montage targeting right parietal cortex (right parietal–left frontal, electrode-sizes: 3cm × 3cm–7 cm × 5 cm), while performing a perceptual line bisection (landmark) task. The tES-protocols were compared as to their ability to modulate pseudoneglect (thought to be under right hemispheric control). In experiment 1, sham-tES was compared to transcranial alternating current stimulation at alpha frequency (10 Hz; α-tACS) (expected to entrain “inhibitory” alpha oscillations) and to cathodal transcranial direct current stimulation (c-tDCS) (shown to suppress neuronal spiking activity). In experiment 2, we attempted to replicate the findings of experiment 1, and establish frequency-specificity by adding a 45 Hz-tACS condition to α-tACS and sham. In experiment 1, right parietal α-tACS led to the expected changes in spatial attention bias, namely a rightward shift in subjective midpoint estimation (relative to sham). However, this was not confirmed in experiment 2 and in the complete sample. Right parietal c-tDCS and 45 Hz-tACS had no effect. These results highlight the importance of replication studies, adequate statistical power and optimizing tES-interventions for establishing the robustness and reliability of electrical stimulation effects, and best practice.
Introduction
Transcranial electrical stimulation (tES) is an umbrella term for non-invasive brain stimulation techniques that involve the application of weak electrical currents to the brain. These techniques are increasingly being employed as tools in cognitive neuroscience and investigated as to their potential for novel clinical interventions in various neurological and psychiatric disorders(Berlim et al., 2013; Baeken et al., 2016; Lefaucheur, 2016). However, while some results provide evidence of cognitive enhancement and therapeutic benefit through tES across a range of cognitive domains (see Santarnecchi et al., 2015 for a review of neuroenhancement in healthy samples and Brunoni et al., 2012; Fregni et al., 2015 for discussions of clinical research), there remains little understanding of tES mechanisms of action and questions have been raised about the reliability of its effects (Bestmann et al., 2015; Horvath et al., 2015a,b; Parkin et al., 2015). Large variability in tES-outcome has been shown within and across studies. This variability is likely to be explained by the large number of factors influencing outcome (Fertonani and Miniussi, 2016), including current strength/density (Teo et al., 2011; Moos et al., 2012; Bastani and Jaberzadeh, 2013; Batsikadze et al., 2013; Hoy et al., 2013; Benwell et al., 2015; Ho et al., 2016), electrode montage (Moliadze et al., 2010; Sehm et al., 2013; Scheldrup et al., 2014; Mehta et al., 2015), stimulation duration (Nitsche and Paulus, 2000; Stagg and Nitsche, 2011), stimulation frequency (Kanai et al., 2010; Feurra et al., 2011; Brignani et al., 2013; Wach et al., 2013; Cabral-Calderin et al., 2016), timing of stimulation relative to task engagement (Pirulli et al., 2013; Scheldrup et al., 2014; Bortoletto et al., 2015; Cabral-Calderin et al., 2016), baseline trait/task performance levels of participants (Dockery et al., 2009; Tseng et al., 2012; Hsu et al., 2014; Sarkar et al., 2014; Benwell et al., 2015; Learmonth et al., 2015; Li et al., 2015b; London and Slagter, 2015), task demands (Li et al., 2015a; Roe et al., 2016) and the initial excitatory/oscillatory state of the stimulated region (Feurra et al., 2013). In addition to the difficulty of understanding all of the potential contributors to tES outcome, its effects tend to be relatively small and difficult to replicate (Walsh, 2013).
Here, we sought to directly compare two different tES techniques as to their efficacy to change behavior within the same experimental setting (same participant, task, montage) and to establish reproducibility in a follow-up experiment. In the first experiment, we compared cathodal tDCS (c-tDCS) and tACS at 10 Hz, i.e., in the alpha-band (8–12Hz) (α-tACS) against sham tES as to their ability to modulate visuospatial attention. We chose these protocols because both have been associated with “inhibitory” effects on attention tasks, despite their supposedly different mechanisms of action, namely a decrease of cortical excitability through an alteration of the resting neuronal membrane threshold for c-tDCS (Bindman et al., 1964; Nitsche and Paulus, 2000; Nitsche et al., 2005) or the synchronization of oscillatory activity in the alpha-band for α-tACS (Zaehle et al., 2010; Antal and Paulus, 2013; Herrmann et al., 2013; Helfrich et al., 2014; Veniero et al., 2015). Alpha-band activity has been shown to inversely relate to visual cortex excitability (Romei et al., 2008) in line with its proposed inhibitory role (Klimesch et al., 2007; Jensen and Mazaheri, 2010).
In order to assess tES outcome on attention processes, we employed a perceptual variant of line bisection (the landmark task: Milner et al., 1992; McCourt and Olafson, 1997) measuring spatial attentional bias [usually biased toward the left in heathy participants, c.f. pseudoneglect (Bowers and Heilman, 1980; Jewell and McCourt, 2000)], and which is thought to be driven by a right hemisphere (RH) dominance (for neuroimaging evidence, see Fink et al., 2000; Weiss et al., 2000; Foxe et al., 2003; Cicek et al., 2009; Thiebaut de Schotten et al., 2011; Benwell et al., 2014a). We chose the landmark task because of its high test–retest reliability (Benwell et al., 2013b, 2015), and because simple experimental manipulations (e.g., time-on-task) can induce reproducible short-term changes in line bisection bias (Manly et al., 2005; Dufour et al., 2007; Benwell et al., 2013a). We reasoned that a combination of high test–retest reliability and amenability to experimental manipulation constitutes an important precondition when probing for potentially weak tES effects. Specifically, we hypothesized that both right parietal c-tDCS and right parietal α-tACS would cause an inhibition of right hemispheric attention processes and thus result in a similar behavioral outcome on attentional bias in the landmark task, namely canceling out the systematic group-level leftward bias (i.e., pseudoneglect) (Benwell et al., 2013a,b, 2014a,b). This is motivated by previous tDCS research in healthy participants showing that right parietal c-tDCS drives a rightward shift in subjective midpoint estimation (Giglia et al., 2011; Wright and Krekelberg, 2014; Benwell et al., 2015). Right parietal α-tACS, on the other hand, may influence attentional bias by up-regulating parieto-occipital alpha power (see Thut et al., 2006; Newman et al., 2013, 2017 implicating EEG alpha activity in visuospatial bias) and thereby downregulate RH contribution to landmark task processing and ameliorate pseudoneglect.
In the second experiment, we aimed to reproduce effects of active versus sham tES observed in the first experiment in an independent sample of participants. We also added an extra tACS condition (45 Hz, γ-tACS) to test for frequency-specificity of outcome, as γ-tACS is not expected to induce an inhibitory effect on attentional processes.
Materials and Methods
Participants
A total of 40 right-handed volunteers participated after having given their written informed consent. In the first experiment, 20 participants were recruited. One participant was excluded due to poor task performance [curve fitting procedures showed a low goodness of fit in one of the conditions (R2 = 0.5), see analysis below for details]. Data from 19 participants were therefore considered for the final analysis of experiment 1 (12 female, mean age: 23.6, SD = 3.8). In the second experiment, an independent group of 20 participants was enrolled (10 female, mean age: 23.8, SD = 2.8).
All participants had normal or corrected-to-normal vision and had no contraindication to brain stimulation according to the screening questionnaire described in Rossi et al. (2009) or reported any neurological, psychiatric, or other relevant medical problems. The protocol was performed in accordance with ethical standards and was approved by the Ethics Committee of the College of Science and Engineering (University of Glasgow).
Task and Stimuli
In both experiments, participants performed a modified version of the landmark task (Milner et al., 1992; Benwell et al., 2015) in which pre-transected black and white lines of 100% Michelson contrast are presented on a gray background (luminance = 179, hue = 160). Lines measured 24.3 cm by 0.5 cm and subtended 19.67° by 0.40° of visual angle at a viewing distance of 70 cm. Lines were transected at 17 different points ranging symmetrically ±4% of absolute line length from veridical center. This represented a range of -0.8 to 0.8° of visual angle relative to veridical center. Stimuli were presented on a CRT monitor with a 1280 pixel × 1024 pixel resolution using E-prime (Psychology Software Tools, Pittsburgh, PA, United States).
Each trial started with a central fixation cross (0.4 × 0.4° visual angle) displayed for 1 s, followed by the presentation of a transected line for 150 ms (Figure 1A). The transection mark was always aligned with the fixation cross, therefore preventing use of the fixation cross as a reference point for bisection judgments. The fixation cross then reappeared for the response period, during which participants indicated as fast and accurately as possible which end of the line the transection mark had appeared closest to, by pressing either a left or right response key with the index or middle finger of the right hand. Participants were instructed to keep their gaze on the fixation cross. The next trial started as soon as the response was made (Figure 1A). The location of the transector point was randomly selected in each trial.
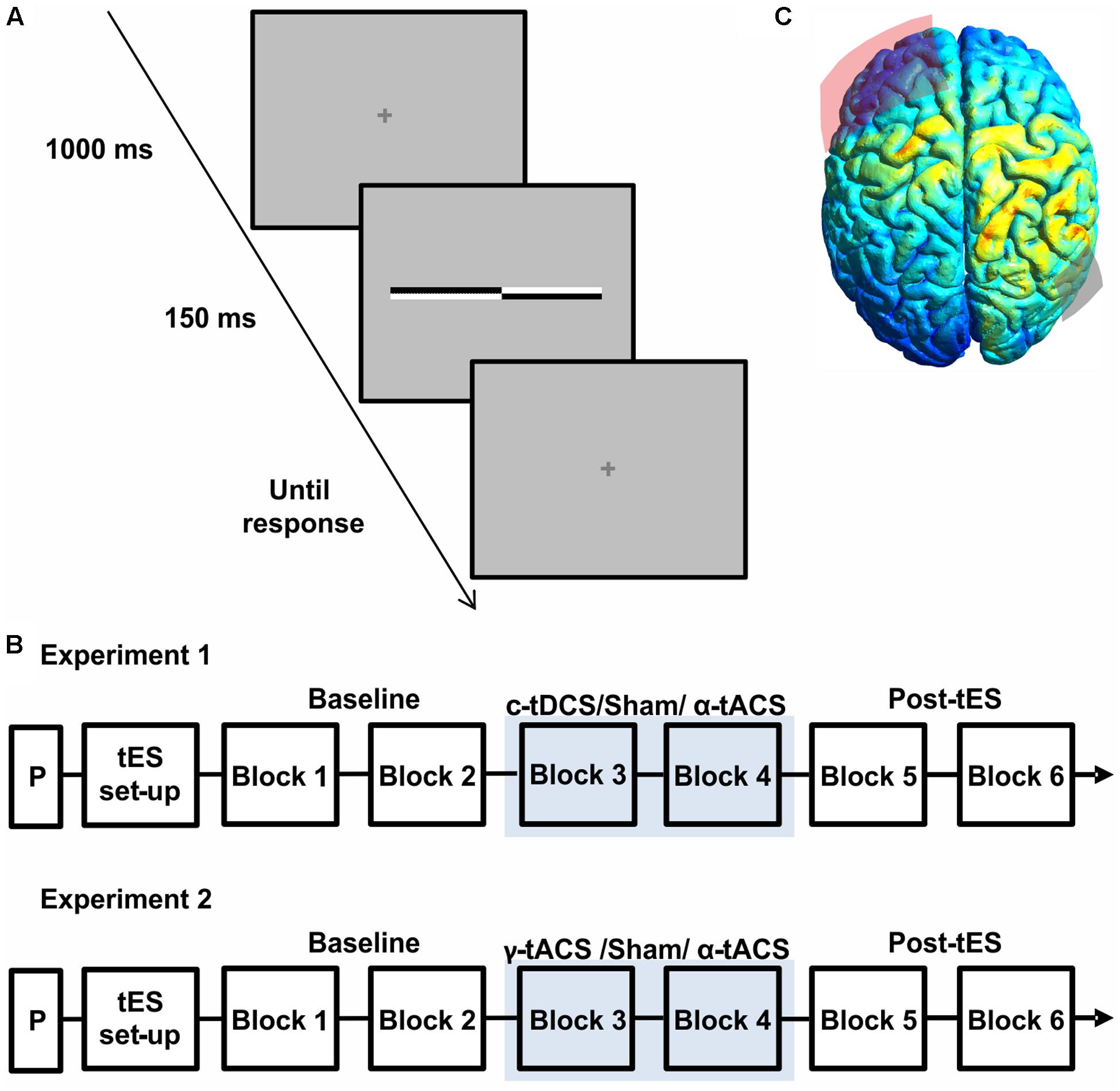
FIGURE 1. (A) Schematic representation of a single trial structure. A fixation cross was presented for 1000 ms, followed by a transected line flashed for 150 ms. The fixation cross reappeared for the response time period. (B) Schematic representation of the experimental procedure of both experiments. On each day, after a practice session (P) and transcranial electrical stimulation (tES) set up, participants were asked to complete six blocks of the landmark task. During block 3 and block 4 participants received active tES or sham in a counter-balanced order across days. (C) Results of electric field distribution modeling are shown together with the electrode position (F3 in red, P6 in gray). The electric field strength is scaled from 0 (blue) to maximum (red). The simulation indicates diffuse activation within the right parietal cortex together with a more restricted activation of a left frontal region.
Transcranial Electrical Stimulation
Transcranial electrical stimulation was delivered through a battery-driven, constant current stimulator (NeuroConn GmbH, Germany) using two surface electrodes placed in saline-soaked sponges kept in place by an elastic net. To optimize skin/electrode impedance a thin layer of electroconductive gel was applied to the sponges (Horvath et al., 2014; Fertonani et al., 2015) (for similar practices see, e.g., also Pirulli et al., 2013; Tecchio et al., 2013; Romero Lauro et al., 2014; Li et al., 2015a). Impedance values were maintained below 5 kΩ. The electrodes positions were individually located in accordance with the International 10–20 EEG system: the target electrode (3 cm × 3 cm) was placed over the right parietal cortex (P6), whereas the frontal electrode (7 cm × 5 cm) was centered over F3. The size of the frontal electrode was chosen to reduce current density and limit stimulation effects under its surface (Nitsche et al., 2007) relative to the parietal electrode, and the slightly more superior position, relative to the frequently used supra-orbital site (FP1), was chosen to limit phosphene perception. The F3 location was determined based on a pilot. In 3 participants (none of whom included in experiment 1 or 2), we assessed the amount of visual sensations induced by α-tACS under slightly different montages (moving the frontal electrode from FP1 to F3 while keeping the parietal electrode at P6). All participants reported visual sensations with the frontal electrode over FP1. When moving the frontal electrode to F3 (without lowering the stimulation intensity), phosphene perception was absent in these 3 participants. In the participants of the main experiments, phosphene perception was probed through a questionnaire asking about tES-induced sensation, which was given at the end of each session (see Procedure and Results section). Unilateral parietal stimulation (P6) was chosen (as opposed to bilateral parietal stimulation applied in some previous tDCS-studies, e.g., P6–P7) to be able to selectively interfere with right parietal cortex during both c-tDCS and α-tACS (bi-parietal α-tACS would have affected both hemispheres in the same way).
For all conditions, stimulation intensity was set at 1 mA, yielding an average current density of 0.11 and 0.03 mA/cm2 for the parietal and frontal electrode, respectively. The anatomical target of stimulation was verified by modeling the electric current field distribution induced with 1 mA intensity and the above montage using SimNIBS1 (Thielscher et al., 2015), adopting the standard head model provided by the software.
Each participant underwent three stimulation conditions, which consisted of c-tDCS, α-tACS and sham tES in experiment 1, and α-tACS, γ-tACS and sham tES in experiment 2. For tDCS, the polarity of the target electrode (P6) was cathodal (c-tDCS). For tACS, a sinusoidal electrical current waveform with no DC offset was delivered at 10 Hz (α-tACS) or 45 Hz (γ-tACS). The total stimulation time was 10 min, including 30 s ramping up/down except for the sham condition, during which the stimulator was turned off after 30 s (10 s ramping up/down).
For both experiments, the three sessions were separated by at least 48 h with the order of tES protocols counter-balanced across participants to control for learning and carry-over effects.
Procedure
Participants were seated in a comfortable armchair in a dimly lit room. The viewing distance (70 cm) was kept constant throughout the session using a chin rest. Each session started with a practice block (Figure 1B) including two trials for each transector location for a total of 34 trials. Participants were then prepared for the stimulation (tES setup, Figure 1B). During each session (experiment 1: c-tDCS, α-tACS and Sham; experiment 2: α-tACS, γ-tACS, and Sham), participants were asked to complete six blocks of landmark task performance, consisting of 136 trials (8 trials for 17 transector point), lasting around 5 min each. As show in Figure 1B, stimulation was applied during block 3 and 4, whereas the first and last two blocks were performed without stimulation and served as a baseline (blocks 1–2) and to test for potential after-effects (blocks 5–6) (Figure 1B). At the end of the blocks, participants were asked to complete a questionnaire implemented to evaluate sensations experienced during tES including phosphenes (Fertonani and Miniussi, 2016) on a scale of 0 (not experienced) to 4 (experienced very strongly). Data from this questionnaire were analyzed using a Friedman ANOVA to assess side-effects across sessions. At the end of all sessions, participants were asked to guess on which day they had received sham stimulation.
Analysis
To obtain an objective measure of perceived line midpoint for each block and session in each subject, psychometric functions (PFs) were derived using the method of constant stimuli. The dependent measure was the percentage of trials on which participants indicated that the transector had appeared closer to the left end of the line. Non-linear least-squares regression was used to fit a cumulative logistic function to the data for each block and stimulation condition in each subject. The cumulative logistic function is described by the equation:
where x represents the transection locations, μ corresponds to the estimated transector location with 50:50% “left”:“right” response rate and w is the estimated width of the psychometric curve (both measured in pixels on the x-axis). The 50% location is also known as the point of subjective equality (PSE) and represents an objective measure of perceived line midpoint. The width of the fitted PF provides a measure of the precision of the participants’ line midpoint judgments (visual discrimination sensitivity).
As PSE represented our dependent (outcome) measure of interest, we assessed its test–retest reliability within participants using robust correlation analysis between values obtained during the baseline blocks (average of blocks 1–2) in each session, i.e., across 3 days. This analysis was performed using Spearman’s rho and Shepherd’s Pi (Schwarzkopf et al., 2012). tES effects on PSE were analyzed using a repeated measure analysis of variance (ANOVA) testing the factors tES (Experiment 1: c-tDCS vs. α-tACS vs. Sham; Experiment 2: α-tACS vs. γ-tACS vs. Sham) and Block (B1–B6).
Finally, in order to quantify whether any effects observed in experiment 1 could be successfully replicated in experiment 2, we calculated the Bayes factor (B) which allows a quantification of how strong the evidence is for the alternative or the null hypothesis, here the presence or absence of stimulation effect. Despite the fact that evidence is continuous, B < 1/3 can be considered as strong evidence in favor of the null hypothesis, B > 3 as strong evidence in favor of the alternative hypothesis, whereas 1/3 < B < 3 indicates data insensitivity (support for neither hypothesis) (Dienes, 2014; Verhagen and Wagenmakers, 2014). We modeled the plausibility of different effects by a half normal distribution (Dienes and Mclatchie, 2017) and corrected for the degrees of freedom as in Dienes (2014).
Results
Electric Field Modeling
Figure 1C shows the results of the current distribution modeling. The electric field strength peaked within the right parietal cortex, suggesting that the chosen montage most strongly stimulates our target site. The modeling also indicated stimulation of a more spatially restricted part of the left frontal lobe, albeit at a weaker current.
Experiment 1
Sensations during tES
Transcranial electrical stimulation did not cause any of the participants to experience adverse effects. The results of the questionnaire indicated a low mean difference in sensation scoring when comparing the active with the sham condition (active tES minus sham ∼0.2). Three participants reported visual sensations at the beginning of α-tACS. A Friedman’s test used to compare the strength of the sensations in each session revealed no significant difference (p = 0.88), indicating that all protocols were similar in associated (low) discomfort. Eight participants correctly identified the sham session (42%). A chi square goodness of fit run to compare the percentage of correct guesses (42% = 8/11) with the expected (chance) occurrence (33%: 6/13) revealed no significant deviation from the expected value [X2(1) = 0.97; p = 0.32], thus confirming that the percentage of participants correctly identifying the sham condition was not different from chance.
Spatial Attention Bias: Test–Retest Reliability across Baseline Blocks
To inform the interpretation of potential tES effects on our variable of interest (subjective midpoint in line bisection/attentional bias), we first analyzed the data of the baseline blocks before tES (blocks 1–2) in terms of an overall deviation of subjective midpoint from the veridical midline (i.e., pseudoneglect), as well as its test–retest reliability within participants. We found a leftward bias at the group-level (pseudoneglect) across the three sessions (all baseline data collapsed) [one-sample t-test against zero, t(18) = -2.09, p = 0.05] and good test–retest reliability of individual baseline bias across the three (i.e., α-tACS, c-tDCS and sham) sessions (see Figure 2A for scatterplots and correlation analysis results). This confirms previous results (for pseudoneglect see, e.g., Jewell and McCourt, 2000 and for test–retest reliability see Benwell et al., 2013b, 2015; Varnava et al., 2013). It is of interest to note that while there is a leftward bias at the group level, and a rather low intra-individual variability across sessions (standard deviation ranged from 0.4 to 3.8 pixels), the inter-individual variability is relatively large (min = -11.3 pixels, max = +6.9, see Figure 2A), also in line with previous findings (Jewell and McCourt, 2000; Benwell et al., 2013b; Szczepanski and Kastner, 2013).
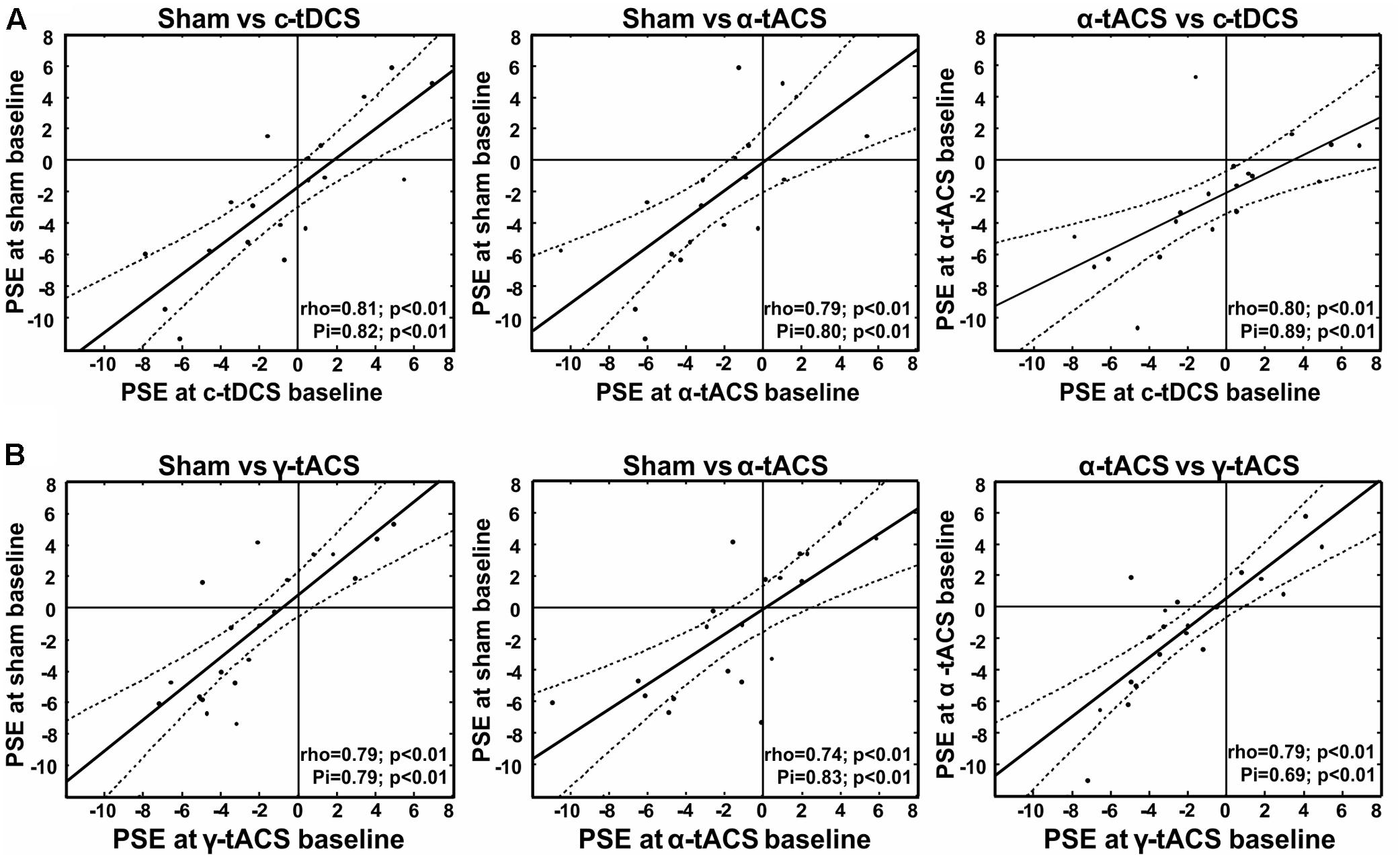
FIGURE 2. Test re-test reliability of spatial bias in perceptual line bisection (point of subjective equality, PSE) for experiment 1 (A) and experiment 2 (B). Test–retest reliability is significant across three measurement points collected before tES (baseline) at three different days, as indicated by both Spearman’s rho and Shepherd’s Pi values (see lower right corner of each graph). x- and y-values represent the distance from the veridical center in pixels (dashed lines represent 95% confidence intervals).
Spatial Attention Bias: Effects of α-tACS and c-tDCS
We next analyzed the influence of tES on attentional bias (see Figure 3A for the group-averaged data per condition). The ANOVA revealed an interaction between the factors tES (α-tACS vs. c-tDCS vs. Sham) and Blocks (B1–6) [F(10,180) = 2.51, p = 0.008, = 0.12] suggesting that active and sham tES differently affected subjective midline judgment over time. No main effects were observed [tES: F(2,36) = 0.96, p = 0.39, = 0.05, Block: F(5,90) = 1.17, p = 0.33, = 0.06]. Breaking down the interaction revealed a significant effect of tES (α-tACS vs. c-tDCS vs. Sham) in block 4 [F(2,36) = 3.81, p = 0.032, = 0.17], i.e., during the second half of the 10 min of tES, while there was no significant tES effect in any other block [F(2,36) = 0.13–2.49, p = 0.88–0.10]. By block 4, α-tACS had shifted spatial attention rightward (see Figure 3A, PSE significantly less negative than in the other two conditions), both in comparison to Sham [B4: F(1,18) = 7.00, p = 0.016, d = 0.38] and c-tDCS [B4: F(1,18) = 4.79, p = 0.042, d = 0.36]. c-tDCS did not differ from Sham [F(1,18) = 0.0001, p = 0.99, d = 0.00] at block 4. Overall, this suggests that as expected, α-tACS over right parietal cortex may have redirected attention to the ipsilateral, right hemifield. In contrast to our expectations, c-tDCS did not seem to affect task performance.
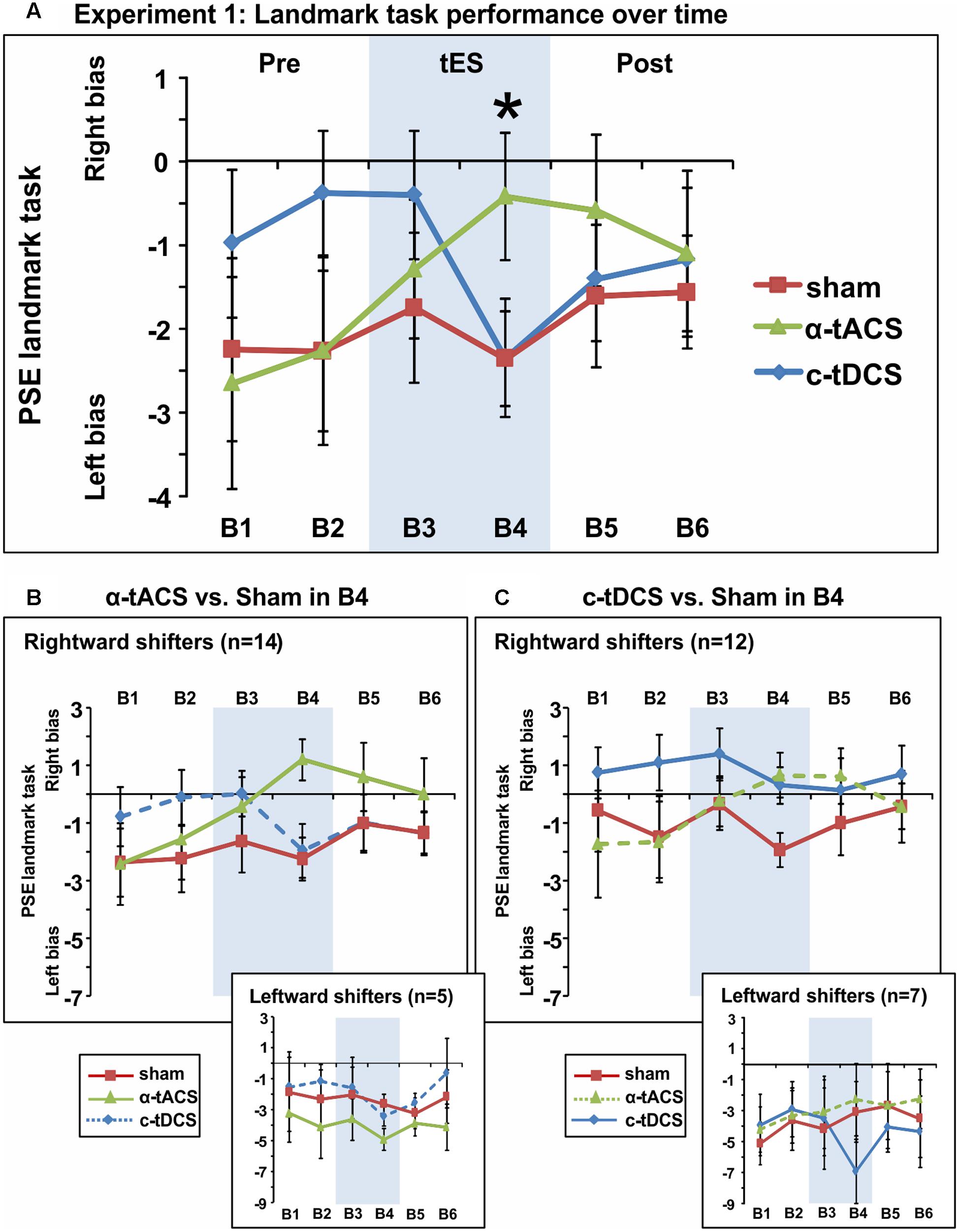
FIGURE 3. Spatial bias in perceptual line bisection (PSE during landmark task performance) over time (six blocks) in three experimental sessions varying in terms of the tES protocol used (experiment 1). tES was applied with a right parietal – left frontal montage for 10 min using either α-tACS, c-tDCS or sham tES during blocks 3–4 (B3–4, shaded area). (A) Overall group mean PSE values (19 participants, within-subject design) as a function of blocks (B1–6) and tES (line plots). During the second block of tES (block 4), α-tACS significantly differed from sham and c-tDCS. The asterisk indicates a significant difference between Sham and α-tACS (p < 0.05). (B) Performance split according to “rightward shifters” (upper main graph, 14/19 participants) and “leftward shifters” (lower inset, 5/19 participants) during α-tACS (as compared to sham in block 4). The pattern of results is consistent with α-tACS having shifted spatial bias rightward (reduced pseudoneglect) in the majority of participants. (C) Performance split according to “rightward shifters” (upper main graph, 12/19 participants) and “leftward shifters” (lower inset, 7/19 participants) during c-tDCS (as compared to sham in block 4). No effects of active stimulation (c-tDCS vs. sham) are discernible in this condition and population. The error bars represent 95% confidence interval corrected for a within subjects design (Cousineau, 2005).
In order to further explore the effect of tES and to test whether subgroups of tES-responders (either for α-tACS or c-tDCS) could be identified, we split participants for each stimulation condition into “rightward shifters” (responders as predicted by our hypothesis) and “leftward shifters” (non-responders) according to their difference in PSE between the active and sham conditions at block 4 (B4 α-tACS or B4 c-tDCS compared to B4 sham). Calculating the number of responders for α-tACS revealed a subgroup of 14 “rightward shifters” (illustrated in Figure 3B, upper large panel) and 5 “leftward shifters” (Figure 3B, lower small panel). The majority of participants responded over time to α-tACS with a progressive rightward shift away from sham performance (Figure 3B, upper large panel, green versus red line). Splitting the data in the same way for c-tDCS (B4 c-tDCS vs. B4 sham) revealed a different picture. While 12 versus 7 participants were more “rightward oriented” in block 4 (see Figure 3C, upper large panel versus lower small panel), there was no sign of a progressive rightward shift during tDCS away from sham performance over time as differences already pre-existed prior to stimulation blocks. By extension, there is therefore no evidence even for a subgroup of participants responding as expected to tDCS. This further confirms that c-tDCS did not affect task performance.
Overall, experiment 1 therefore suggested that α-tACS had shifted spatial attention bias in the majority of participants (n = 14, 74%) in the expected direction, while there was no evidence of a c-tDCS effect. The average amplitude of the tACS-induced change in responders (i.e., the amplitude of the rightward shift) was in the order of the within-subject variability that can be observed across sessions (possibly due to state-changes). In line with this observation, we indeed observed a small effect size (Cohen’s d = 0.36–0.38). We therefore aimed to replicate and extend the result of experiment 1 in an independent sample of participants using α-tACS, with γ-tACS and sham-tES as control conditions. We also reasoned that adding 20 participants should strengthen the α-tACS effects in the whole sample if present (i.e., when the α-tACS and sham-tES conditions of experiment 1 and 2 are combined).
Experiment 2
Sensations during tES
As for experiment 1, tES did not cause any of the participants to experience adverse effects and the scores of the sensation questionnaires only minimally differed between active and sham tES conditions (active tES minus sham ∼0.1). The strength of the sensations in each session showed no significant difference (p = 0.39), with 35% of participants (7 out of 20) correctly identifying the sham session, which corresponds to chance-level, i.e., the number of participants who would correctly guess by chance. Five participants reported visual sensations at the beginning of α-tACS.
Spatial Attention Bias: Test–Retest Reliability across Baseline Blocks
As for experiment 1, we first tested whether we could find a leftward bias in subjective midpoint at the group-level (pseudoneglect) across the three baseline sessions (all baseline data collapsed) and whether we could replicate the high test–retest reliability of individual baseline bias across the three sessions. A one-sample t-test (against zero) again revealed a leftward bias [t(19) = -2, p = 0.06], and the correlation analysis confirmed consistency of PSE within participants as shown in Figure 2B.
Spatial Attention Bias: No Replication of α-tACS Effects
Figure 4A shows group-averaged data for each condition. The main ANOVA performed to assess tES effects (α-tACS vs. γ-tACS vs. Sham) over the six blocks (B1–6) revealed a significant main effect of tES [F(2,38) = 3.44, p = 0.04, = 0.15], explained by generally higher PSE values in the Sham compared to γ-tACS condition [F(1,19) = 6.05, p = 0.02, = 0.24], but no main effect of Block [F(5,95) = 1.75, p = 1.13, = 0.08]. Most importantly, the analysis revealed that there was no significant tES × Block interaction [F(10,190) = 0.73, p = 0.69, = 0.04], suggesting that we could not replicate the α-tACS induced rightward shift found in experiment 1. The lack of a significant interaction implied that in this second sample, α-tACS was unable to induce any modulation of PSE relative to sham at any time point. In order to quantify the number of participants showing the α-tACS-induced effect found in experiment 1, we again split our group into rightward and leftward attention shifters according to PSE values in block 4 (B4 α-tACS vs. B4 sham). As shown in Figure 4B, α-tACS modulated the attentional bias in the expected direction in only 9 out of the 20 participants, whereas the remaining 11 were classified as leftward shifters, further confirming the inconsistency of results in this experiment.
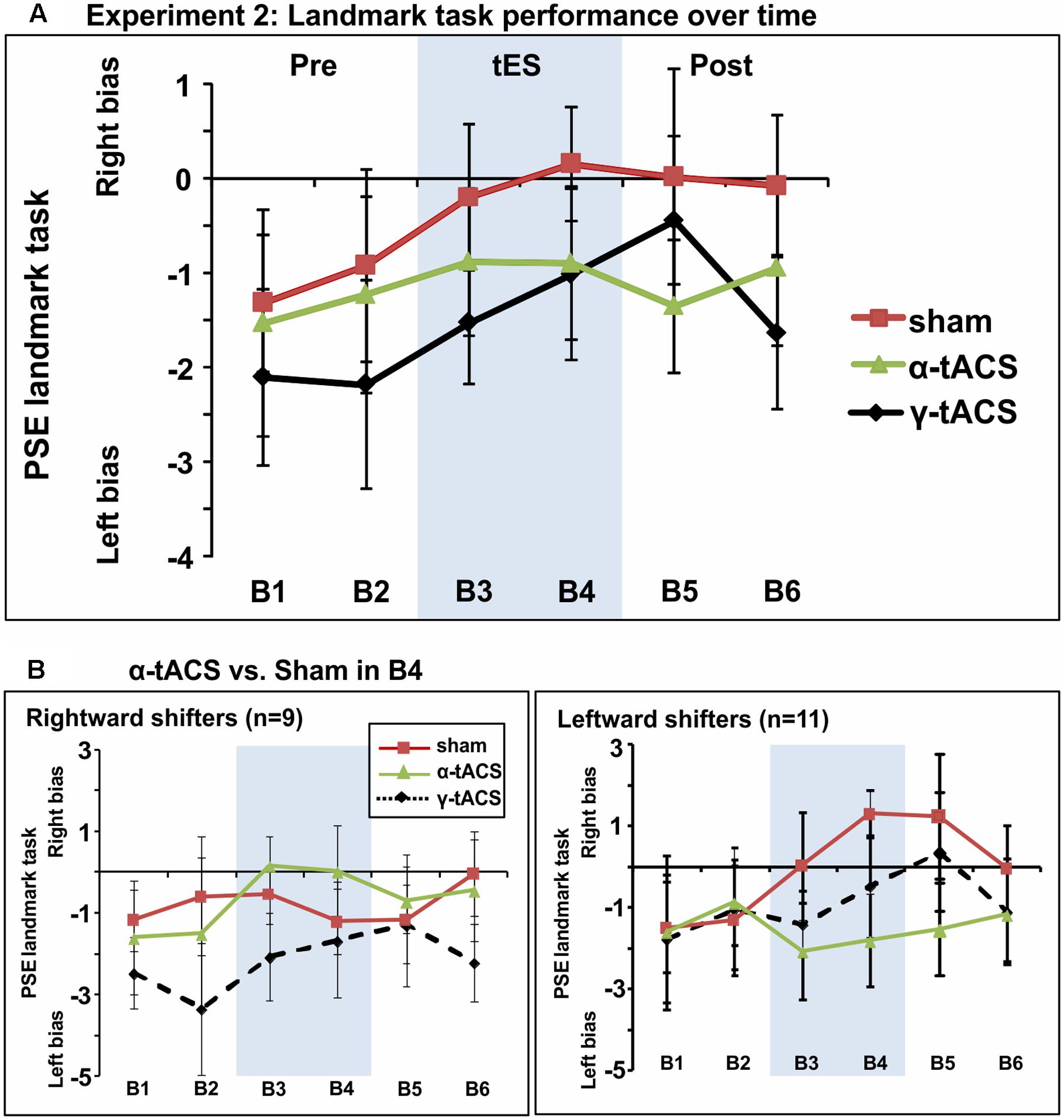
FIGURE 4. (A) Spatial bias in perceptual line bisection (PSE during landmark task performance) over time (six blocks) as a function of tES condition in experiment 2. The montage, intensity and total stimulation duration were identical to experiment 1. tES conditions consisted of α-tACS, 45 Hz (γ-tACS) or sham tES applied during blocks 3–4 (B3-4, shaded area). PSE values (20 participants, within-subject design) showed no significant difference across conditions in this population. Error bars represent 95% confidence interval corrected for a within subjects design. (B) Performance split according to “rightward shifters” (right panel, 9/20 participants) and “leftward shifters” (left panel, 11/20 participants) during α-tACS (as compared to sham in block 4). Unlike experiment 1, α-tACS reduced pseudoneglect in 45% of the population (inconsistent, random distribution).
In order to quantify more precisely the outcome of our replication attempt, we calculated the Bayes factor on the data from experiment 2. Our null hypothesis was that the effect of α-tACS and sham are equivalent and therefore the results of experiment 2 represent a failed replication of experiment 1. Our alternative hypothesis (H1) was that tACS stimulation differs from the sham stimulation, by an amount and direction informed by the first experiment. Hence, our measure of interest (α-tACS effect) was the magnitude of the α-tACS induced shift in PSE (B4 α-tACS minus B1 α-tACS) relative to the PSE shift in the sham condition (B4 Sham minus B1 Sham). The predictions of H1 were modeled as half-normal distribution (BH) with a SD equal to the effect obtained in the first experiment (rightward shift in the α-tACS relative to the sham condition = 2.22 pixels). The analysis revealed that B is <1[BH(0,2.22) = 0.26], thus providing substantial evidence in favor of the null hypothesis indicating a failed replication.
Data of Experiment 1 and 2 Collapsed
Spatial Attention Bias: Effect of α-tACS in Experiment 1 Dissolves in the Whole Sample
Based on the idea that inconsistency in tES outcome might be partly explained by low statistical power, given the small to intermediate effect sizes usually reported (see experiment 1 Cohen’s d and Horvath et al., 2016; Minarik et al., 2016; Schutter and Wischnewski, 2016), we collapsed the PSE data of both experiments for the two common conditions. The whole sample therefore included 39 participants who underwent α-tACS and sham stimulation. The repeated-measures ANOVA testing factors tES (Sham, α-tACS) and Blocks (B1–6) revealed no significant tES × Block interaction [F(5,190) = 0.24, p = 0.94, = 0.00; Figure 5]. In line with previous studies, we found a progressive left- to rightward shifts in line bisection judgment over time [time-on-task effect: main effect of Block: F(5,190) = 3.37, p = 0.006, = 0.08], regardless of the stimulation condition. By block 3, PSE was significantly less negative when compared to the first baseline block [mean B1 PSE = -1.9, mean B3 PSE = -1.08, mean B4 PSE = -0.89, mean B5 PSE = -0.80, mean B6 PSE = -0.88, all F(1,38) ≥ 3.99, all p ≤ 0.05, d ≥ 0.21].
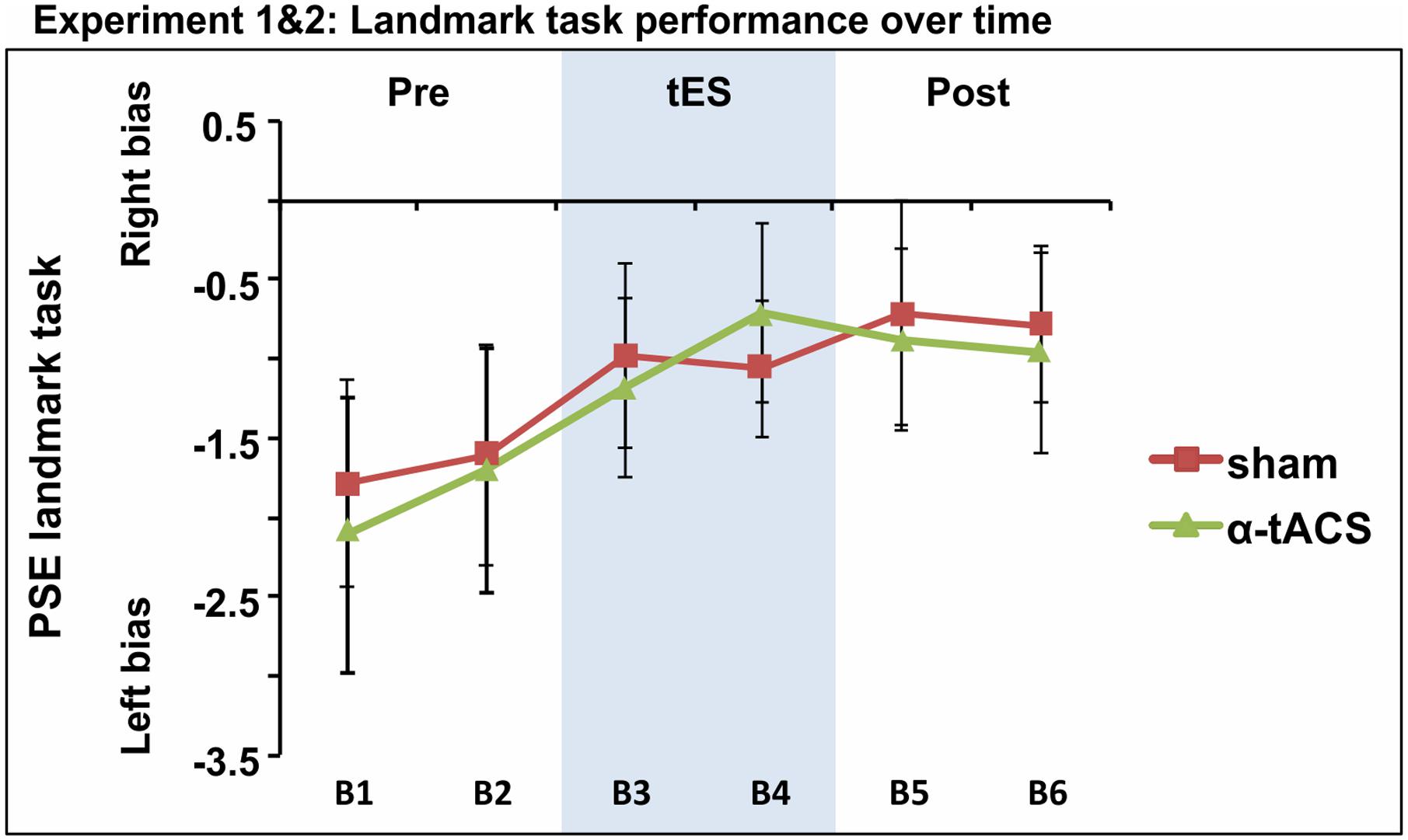
FIGURE 5. Spatial bias in perceptual line bisection (PSE) over time (six blocks) as a function of tES condition when data from experiment 1 and 2 were collapsed over common conditions (Sham, α-tACS). PSE values showed no difference across conditions in the overall population (39 participants). Error bars represent 95% confidence interval corrected for a within subjects design.
To investigate whether participants’ spatial bias at baseline may have affected tES outcome (e.g., may have contributed to the negative findings), participants were split into two subgroups according to the median of PSE values at baseline (each individual value was calculated as the average of block 1 across α-tACS and Sham). A 2 × 6 × 2 mixed-design ANOVA was performed with tES (α-tACS vs. sham) and Blocks (B1–6) as within-subject factors and PSE at baseline (above or below group median) as a between-subject factor. The ANOVA confirmed the progressive left- to rightward shift over time (main effect of Block [F(5,185) = 3.38, p = 0.006, = 0.08]), but revealed no effect of the initial spatial bias on tES outcome [no 3-way interaction of PSE at baseline × tES × Block: F(5,185) = 2.04, p = 0.07, = 0.005].
In the same vein, we tested whether our results could be explained by differences in discrimination sensitivity at baseline, defined as the average PF curve width in block 1 across the two sessions (as we have previously found tDCS-outcome on line bisection to depend on this measure, Benwell et al., 2015). Participants were therefore divided into two groups, one including those displaying baseline PF curve width below the median and one including those displaying width values above the median. tES effects on PSE were then tested through a 2 × 6 × 2 mixed design ANOVA with tES (α-tACS vs. Sham) and Blocks (B1–6) as within-subject factors and Curve Width at baseline (above or below group median) as a between-subject factor. The results again revealed a significant effect of block on PSE [F(10,170) = 2.53, p = 0.007, = 0.13] but no effect of the discrimination sensitivity at baseline on tES outcome [curve width × tES × Block: F(5,185) = 0.98, p = 0.43, = 0.02].
Discussion
Across two experiments, we aimed to test to what extent previously reported parietal tES-effects on line bisection performance can be extended by introducing α-tACS and to compare its effects to c-tDCS, thereby providing new information on what could constitute promising stimulation parameters for interventions with existing, putatively “inhibitory” protocols. We also aimed to further address the tES replicability issue by attempting to replicate our own results in a second, independent sample. Our main findings were twofold. First, the results from experiment 1 showed no effect of c-tDCS on spatial attention bias, not replicating previously reported tDCS effects (Giglia et al., 2011; Benwell et al., 2015), whereas tACS delivered at 10 Hz caused a rightward shift in subjective midpoint estimation when compared to sham stimulation. This effect, however, was only present during stimulation and was characterized by a modest effect size. Second, when attempting to reproduce the α-tACS effect in an independent sample (experiment 2), we found no difference between α-tACS and sham stimulation. The null-effect was confirmed when testing the complete sample of participants (n = 39).
Discrepancies with Previous tDCS Studies
Parietal tDCS has previously been reported to modulate visuospatial attention in a number of different tasks depending on polarity and stimulated hemisphere (Sparing et al., 2009; Loftus and Nicholls, 2012; Wright and Krekelberg, 2014; Benwell et al., 2015) (see Laczo et al., 2012; Li et al., 2015a for negative findings). Also using a line bisection task, Giglia et al. (2011) and Benwell et al. (2015) found a rightward shift in subjective midpoint estimation when cathodal tDCS was applied over the right parietal cortex. The discrepant findings in our study may be explained by differences in stimulation parameters. In both previous studies, tDCS was applied in a dual-parietal configuration, with the cathode over the right parietal cortex and the anode over the homologous left parietal area, while here we used unilateral parietal tDCS (with a second electrode over frontal cortex to selectively target right parietal cortex using the same montage also with α-tACS). However, Giglia et al. (2011) also tested unilateral right parietal c-tDCS with the anodal electrode over the contralateral orbit (i.e., similar to our electrode configuration) and found tDCS to reduce pseudoneglect, albeit with weaker effects compared to the bilateral montage. Differences in stimulation duration could also account for the discrepant findings, as in Giglia et al. (2011) and Benwell et al. (2015) total stimulation time was 20 min, whereas in the current study it was reduced to 10 min. Regarding tACS, no previous study has investigated tACS effects on attentional bias as measured by landmark task performance.
Failed Replication Attempt: Points to Consider for Best Practice
Whilst the failed replication of previous tDCS effects may be accounted for by differences in task design, our failed direct internal replication of the tACS results is more unexpected.
A key point is that in the first experiment, we found statistically significant α-tACS effects, while the experiment was comparable in sample size to the majority of published tACS studies (we tested 19 participants; for previously used sample sizes see Schutter and Wischnewski, 2016, Table 1, average over 50 studies = 17 participants). It is therefore reasonable to assume that our first experiment would have been suitable for publication, although our results would have clearly overestimated the α-tACS effect if published stand-alone. These findings may cast doubts on the efficacy and reliability of tES (Wiethoff et al., 2014; Heroux et al., 2015; Horvath et al., 2015a) and/or point to a small to moderate effect size (see also Benwell et al., 2015; Hill et al., 2016; Mancuso et al., 2016; Minarik et al., 2016; Schutter and Wischnewski, 2016), which in combination with small sample sizes (Heroux et al., 2015; Grefkes and Fink, 2016; Minarik et al., 2016) increases the probability of false discoveries (Cappelleri et al., 1996; Ioannidis, 2005; Heroux et al., 2015; Vannorsdall et al., 2016) and the over- or underestimation of the effects of stimulation. It is possible that the α-tACS effect in experiment 1 may represent a false positive, as it was not confirmed in the second experiment (nor over the complete sample); or alternatively, our study may have been underpowered for revealing the true effect [although we merged the data from two experiments, n = 39; see Minarik et al. (2016) for a calculation of adequate sample size in tES studies]. However, while we would like to point out that our results may not be conclusive as to the efficacy of the present tES-protocols in modulating attentional bias, the inconsistent findings across our two studies clearly suggest that one should be cautious when interpreting results from single tES studies. Because testing large samples is not always realistic, internal replication attempts should be considered to establish tES efficacy before publication.
Limitations in Design of Our Study and Suggestions for Improvements
It is conceivable that our overall unsuccessful attempt to modulate attention bias is based on the wrong choice of stimulation parameters, but also on the high variability in individual responses to brain stimulation (tES: Kuo et al., 2013; Lopez-Alonso et al., 2014; Wiethoff et al., 2014; TMS: Fratello et al., 2006; Hamada et al., 2013). The identification of factors that might contribute to this variability is crucial in order for them to be controlled for in future research (for a review on individual differences influencing tES outcome see Krause and Cohen Kadosh, 2014). Although identifying these factors and suboptimal design parameters is difficult, we point out below some potentially important changes to the overall ineffective stimulation approach we used here for consideration in future studies.
Evidence supporting the idea that tACS might engage neural activity through entrainment of endogenous oscillations has come from animal models (Ozen et al., 2010), computational models (Ali et al., 2013; Herrmann et al., 2016) and human studies (Helfrich et al., 2014; Neuling et al., 2015). According to this framework, matching the stimulation frequency to the individual natural frequency increases the efficacy of the stimulation by facilitating the emergence of entrainment (Pikovsky et al., 2003). In the present study, tACS frequency was not individually adjusted. It is therefore possible that stimulation was effective only in a small subsample (when individual alpha frequency matched the stimulation frequency of 10 Hz) potentially explaining the difference between experimental groups (i.e., in case of a higher number of individuals with an individual alpha frequency close to 10 Hz in the participant group of the first experiment). Note that matching the stimulation frequency to endogenous oscillations for obtaining entrainment could be particularly relevant for electrical stimulation, since the need for an exact match is magnified with weak stimulation forces (see Arnold tongues in entrainment models in, e.g., Pikovsky et al., 2003, but also Frohlich, 2015; Thut et al., 2017). This might not be the case for transcranial magnetic stimulation, a technique that allows for the induction of electric fields strong enough to trigger action potentials, and which has been shown to influence the balance of attention allocation even with fixed 10 Hz stimulation of parietal cortex (Romei et al., 2010; Ruzzoli and Soto-Faraco, 2014). In light of our findings, we therefore recommend to attempt an exact match between stimulation and natural frequencies when aiming to interact with oscillatory activity by tACS.
A second reason for our overall unsuccessful attempt to modulate attention bias could be differences in individual anatomy. Despite the fact that the simulation of the current distribution did suggest an electric field maximum within the right parietal cortex, the modeling was not informed by individual anatomy and therefore did not take into consideration individual skull thickness and gyri configuration. In light of this limitation, it is therefore possible that the amount and distribution of current reaching the brain varied across participants. Moreover, variations in individual anatomy will also influence the direction of the induced current flow (normal vs. tangential components) (De Berker et al., 2013). We believe that optimization of tES interventions will ideally require more detailed planning of stimulation montages before experimentation.
Stimulation outcome has been shown to depend on individual trait factors and the state of the cortex (Feurra et al., 2013; Neuling et al., 2013; Benwell et al., 2015; Alagapan et al., 2016). Here, we performed additional post hoc analysis on the entire sample in the attempt to identify possible trait factors co-varying with tES outcome (taking into account the measures we had available, i.e., PSE and curve width at baseline), but found no evidence of any influence. However, it is conceivable, that other factors, such as alpha power at baseline could have better predicted the stimulation effect, in line with studies showing that tACS can engage endogenous rhythms depending on the pre-existing power of the targeted oscillation (Neuling et al., 2013; Alagapan et al., 2016). Large-scale studies with large sample sizes (ideally multi-center) would be required to screen for possible predictors of outcome, and to test their validity.
Finally, it may be argued that the measure of spatial bias used here (PSE) is potentially confounded by response bias, because our participants were always asked to indicate which side of the stimulus appeared ‘shortest.’ This confound can be removed by alternating within participants across blocks in which they are requested to indicate the ‘shortest’ and ‘longest’ end of the line (Toraldo et al., 2004). However, several studies have previously shown baseline pseudoneglect to be a consistent bias in healthy, young individuals regardless of whether single and/or separate instructions were given (Jewell and McCourt, 2000; Schmitz et al., 2011; Benwell et al., 2013a,b, 2014a). For this reason, we believe that the leftward bias is not explained by a response bias. Additionally, potentially opposite α-tACS effects on perceptual and motor bias could explain the inconclusive results in the overall sample, but are unlikely to explain the significant effect of α-tACS in the first experiment.
Conclusion
Taken together, our results highlight the need to adopt caution in interpreting tES results from single-studies when characterized by small sample sizes and arbitrary choices of stimulation parameters (frequency, electrode position, intensity of stimulation). Because these characteristics may potentially come with a lack of statistical power and/or an inadequate use of the stimulation itself, they limit the conclusions about the efficacy of the studied tES protocol. The specificity and efficacy of tES may be improved by taking into account individual differences in anatomy and other endogenous factors to optimize design parameters (e.g., stimulation frequencies, electrode montages, intensities etc.). However, we also believe that larger scale studies and direct replications are needed to establish the robustness and reliability of electrical stimulation effects, and to identify potential predictors of outcome.
Author Contributions
DV, CB, and GT conceived the study and analyzed the data. DV, CB and MA collected the data. All authors wrote the manuscript.
Funding
This work was supported by a Wellcome Trust Award to Gregor Thut [grant number 098434].
Conflict of Interest Statement
The authors declare that the research was conducted in the absence of any commercial or financial relationships that could be construed as a potential conflict of interest.
Acknowledgments
We would like to thank Carolin Schmidt, Julia Blair and Laura Palomo Sarrias for their help in data collection, Roberto Cecere for help with Electric field modeling and Zoltan Dienes for his useful comments and suggestions about Bayesian analysis.
Footnotes
References
Alagapan, S., Schmidt, S. L., Lefebvre, J., Hadar, E., Shin, H. W., and Frhlich, F. (2016). Modulation of cortical oscillations by low-frequency direct cortical stimulation is state-dependent. PLoS Biol. 14:e1002424. doi: 10.1371/journal.pbio.1002424
Ali, M. M., Sellers, K. K., and Frohlich, F. (2013). Transcranial alternating current stimulation modulates large-scale cortical network activity by network resonance. J. Neurosci. 33, 11262–11275. doi: 10.1523/JNEUROSCI.5867-12.2013
Antal, A., and Paulus, W. (2013). Transcranial alternating current stimulation (tACS). Front. Hum. Neurosci. 7:317. doi: 10.3389/fnhum.2013.00317
Baeken, C., Brunelin, J., Duprat, R., and Vanderhasselt, M. A. (2016). The application of tDCS in psychiatric disorders: a brain imaging view. Socioaffect. Neurosci. Psychol. 6:29588. doi: 10.3402/snp.v6.29588
Bastani, A., and Jaberzadeh, S. (2013). Differential modulation of corticospinal excitability by different current densities of anodal transcranial direct current stimulation. PLoS ONE 8:e72254. doi: 10.1371/journal.pone.0072254
Batsikadze, G., Moliadze, V., Paulus, W., Kuo, M. F., and Nitsche, M. A. (2013). Partially non-linear stimulation intensity-dependent effects of direct current stimulation on motor cortex excitability in humans. J. Physiol. 591, 1987–2000. doi: 10.1113/jphysiol.2012.249730
Benwell, C. S., Harvey, M., Gardner, S., and Thut, G. (2013a). Stimulus- and state-dependence of systematic bias in spatial attention: additive effects of stimulus-size and time-on-task. Cortex 49, 827–836. doi: 10.1016/j.cortex.2011.12.007
Benwell, C. S., Harvey, M., and Thut, G. (2014a). On the neural origin of pseudoneglect: EEG-correlates of shifts in line bisection performance with manipulation of line length. Neuroimage 86, 370–380. doi: 10.1016/j.neuroimage.2013.10.014
Benwell, C. S., Learmonth, G., Miniussi, C., Harvey, M., and Thut, G. (2015). Non-linear effects of transcranial direct current stimulation as a function of individual baseline performance: evidence from biparietal tDCS influence on lateralized attention bias. Cortex 69, 152–165. doi: 10.1016/j.cortex.2015.05.007
Benwell, C. S., Thut, G., Grant, A., and Harvey, M. (2014b). A rightward shift in the visuospatial attention vector with healthy aging. Front. Aging Neurosci. 6:113. doi: 10.3389/fnagi.2014.00113
Benwell, C. S., Thut, G., Learmonth, G., and Harvey, M. (2013b). Spatial attention: differential shifts in pseudoneglect direction with time-on-task and initial bias support the idea of observer subtypes. Neuropsychologia 51, 2747–2756. doi: 10.1016/j.neuropsychologia.2013.09.030
Berlim, M. T., Van Den Eynde, F., and Daskalakis, Z. J. (2013). Clinical utility of transcranial direct current stimulation (tDCS) for treating major depression: a systematic review and meta-analysis of randomized, double-blind and sham-controlled trials. J. Psychiatr. Res. 47, 1–7. doi: 10.1016/j.jpsychires.2012.09.025
Bestmann, S., De Berker, A. O., and Bonaiuto, J. (2015). Understanding the behavioural consequences of noninvasive brain stimulation. Trends Cogn. Sci. 19, 13–20. doi: 10.1016/j.tics.2014.10.003
Bindman, L. J., Lippold, O. C., and Redfearn, J. W. (1964). The action of brief polarizing currents on the cerebral cortex of the rat (1) during current flow and (2) in the production of long-lasting after-effects. J. Physiol. 172, 369–382.
Bortoletto, M., Pellicciari, M. C., Rodella, C., and Miniussi, C. (2015). The interaction with task-induced activity is more important than polarization: a tDCS study. Brain Stimul. 8, 269–276. doi: 10.1016/j.brs.2014.11.006
Bowers, D., and Heilman, K. M. (1980). Pseudoneglect: effects of hemispace on a tactile line bisection task. Neuropsychologia 18, 491–498.
Brignani, D., Ruzzoli, M., Mauri, P., and Miniussi, C. (2013). Is transcranial alternating current stimulation effective in modulating brain oscillations? PLoS ONE 8:e56589. doi: 10.1371/journal.pone.0056589
Brunoni, A. R., Ferrucci, R., Fregni, F., Boggio, P. S., and Priori, A. (2012). Transcranial direct current stimulation for the treatment of major depressive disorder: a summary of preclinical, clinical and translational findings. Prog. Neuropsychopharmacol. Biol. Psychiatry 39, 9–16. doi: 10.1016/j.pnpbp.2012.05.016
Cabral-Calderin, Y., Williams, K. A., Opitz, A., Dechent, P., and Wilke, M. (2016). Transcranial alternating current stimulation modulates spontaneous low frequency fluctuations as measured with fMRI. Neuroimage 141, 88–107. doi: 10.1016/j.neuroimage.2016.07.005
Cappelleri, J. C., Ioannidis, J. P., Schmid, C. H., De Ferranti, S. D., Aubert, M., Chalmers, T. C., et al. (1996). Large trials vs meta-analysis of smaller trials: how do their results compare? JAMA 276, 1332–1338.
Cicek, M., Deouell, L. Y., and Knight, R. T. (2009). Brain activity during landmark and line bisection tasks. Front. Hum. Neurosci. 3:7. doi: 10.3389/neuro.09.007.2009
Cousineau, D. (2005). Confidence intervals in within-subject designs: a simpler solution to Loftus and Masson’s method. Tutor. Quant. Methods Psychol. 1, 42–45. doi: 10.20982/tqmp.01.1.p042
De Berker, A. O., Bikson, M., and Bestmann, S. (2013). Predicting the behavioral impact of transcranial direct current stimulation: issues and limitations. Front. Hum. Neurosci. 7:613. doi: 10.3389/fnhum.2013.00613
Dienes, Z. (2014). Using bayes to get the most out of non-significant results. Front. Psychol. 5:781. doi: 10.3389/fpsyg.2014.00781
Dienes, Z., and Mclatchie, N. (2017). Four reasons to prefer Bayesian analyses over significance testing. Psychon. Bull. Rev. doi: 10.3758/s13423-017-1266-z [Epub ahead of print].
Dockery, C. A., Hueckel-Weng, R., Birbaumer, N., and Plewnia, C. (2009). Enhancement of planning ability by transcranial direct current stimulation. J. Neurosci. 29, 7271–7277. doi: 10.1523/JNEUROSCI.0065-09.2009
Dufour, A., Touzalin, P., and Candas, V. (2007). Time-on-task effect in pseudoneglect. Exp. Brain Res. 176, 532–537.
Fertonani, A., Ferrari, C., and Miniussi, C. (2015). What do you feel if I apply transcranial electric stimulation? Safety, sensations and secondary induced effects. Clin. Neurophysiol. 126, 2181–2188. doi: 10.1016/j.clinph.2015.03.015
Fertonani, A., and Miniussi, C. (2016). Transcranial electrical stimulation: what we know and do not know about mechanisms. Neuroscientist doi: 10.1177/1073858416631966 [Epub ahead of print].
Feurra, M., Bianco, G., Santarnecchi, E., Del Testa, M., Rossi, A., and Rossi, S. (2011). Frequency-dependent tuning of the human motor system induced by transcranial oscillatory potentials. J. Neurosci. 31, 12165–12170. doi: 10.1523/JNEUROSCI.0978-11.2011
Feurra, M., Pasqualetti, P., Bianco, G., Santarnecchi, E., Rossi, A., and Rossi, S. (2013). State-dependent effects of transcranial oscillatory currents on the motor system: what you think matters. J. Neurosci. 33, 17483–17489. doi: 10.1523/JNEUROSCI.1414-13.2013
Fink, G. R., Marshall, J. C., Shah, N. J., Weiss, P. H., Halligan, P. W., Grosse-Ruyken, M., et al. (2000). Line bisection judgments implicate right parietal cortex and cerebellum as assessed by fMRI. Neurology 54, 1324–1331.
Foxe, J. J., Mccourt, M. E., and Javitt, D. C. (2003). Right hemisphere control of visuospatial attention: line-bisection judgments evaluated with high-density electrical mapping and source analysis. Neuroimage 19, 710–726.
Fratello, F., Veniero, D., Curcio, G., Ferrara, M., Marzano, C., Moroni, F., et al. (2006). Modulation of corticospinal excitability by paired associative stimulation: reproducibility of effects and intraindividual reliability. Clin. Neurophysiol. 117, 2667–2674. doi: 10.1016/j.clinph.2006.07.315
Fregni, F., Nitsche, M. A., Loo, C. K., Brunoni, A. R., Marangolo, P., Leite, J., et al. (2015). Regulatory considerations for the clinical and research use of transcranial direct current stimulation (tDCS): review and recommendations from an expert panel. Clin. Res. Regul. Aff. 32, 22–35. doi: 10.3109/10601333.2015.980944
Frohlich, F. (2015). Experiments and models of cortical oscillations as a target for noninvasive brain stimulation. Prog. Brain Res. 222, 41–73. doi: 10.1016/bs.pbr.2015.07.025
Giglia, G., Mattaliano, P., Puma, A., Rizzo, S., Fierro, B., and Brighina, F. (2011). Neglect-like effects induced by tDCS modulation of posterior parietal cortices in healthy subjects. Brain Stimul. 4, 294–299. doi: 10.1016/j.brs.2011.01.003
Grefkes, C., and Fink, G. R. (2016). Noninvasive brain stimulation after stroke: it is time for large randomized controlled trials! Curr. Opin. Neurol. 29, 714–720. doi: 10.1097/WCO.0000000000000395
Hamada, M., Murase, N., Hasan, A., Balaratnam, M., and Rothwell, J. C. (2013). The role of interneuron networks in driving human motor cortical plasticity. Cereb. Cortex 23, 1593–1605. doi: 10.1093/cercor/bhs147
Helfrich, R. F., Schneider, T. R., Rach, S., Trautmann-Lengsfeld, S. A., Engel, A. K., and Herrmann, C. S. (2014). Entrainment of brain oscillations by transcranial alternating current stimulation. Curr. Biol. 24, 333–339. doi: 10.1016/j.cub.2013.12.041
Heroux, M. E., Taylor, J. L., and Gandevia, S. C. (2015). The use and abuse of transcranial magnetic stimulation to modulate corticospinal excitability in humans. PLoS ONE 10:e0144151. doi: 10.1371/journal.pone.0144151
Herrmann, C. S., Murray, M. M., Ionta, S., Hutt, A., and Lefebvre, J. (2016). Shaping intrinsic neural oscillations with periodic stimulation. J. Neurosci. 36, 5328–5337. doi: 10.1523/JNEUROSCI.0236-16.2016
Herrmann, C. S., Rach, S., Neuling, T., and Struber, D. (2013). Transcranial alternating current stimulation: a review of the underlying mechanisms and modulation of cognitive processes. Front. Hum. Neurosci. 7:279. doi: 10.3389/fnhum.2013.00279
Hill, A. T., Fitzgerald, P. B., and Hoy, K. E. (2016). Effects of anodal transcranial direct current stimulation on working memory: a systematic review and meta-analysis of findings from healthy and neuropsychiatric populations. Brain Stimul. 9, 197–208. doi: 10.1016/j.brs.2015.10.006
Ho, K. A., Taylor, J. L., Chew, T., Galvez, V., Alonzo, A., Bai, S., et al. (2016). The effect of transcranial direct current stimulation (tDCS) electrode size and current intensity on motor cortical excitability: evidence from single and repeated sessions. Brain Stimul. 9, 1–7. doi: 10.1016/j.brs.2015.08.003
Horvath, J. C., Carter, O., and Forte, J. D. (2014). Transcranial direct current stimulation: five important issues we aren’t discussing (but probably should be). Front. Syst. Neurosci. 8:2. doi: 10.3389/fnsys.2014.00002
Horvath, J. C., Forte, J. D., and Carter, O. (2015a). Evidence that transcranial direct current stimulation (tDCS) generates little-to-no reliable neurophysiologic effect beyond MEP amplitude modulation in healthy human subjects: a systematic review. Neuropsychologia 66, 213–236. doi: 10.1016/j.neuropsychologia.2014.11.021
Horvath, J. C., Forte, J. D., and Carter, O. (2015b). Quantitative review finds no evidence of cognitive effects in healthy populations from single-session transcranial direct current stimulation (tDCS). Brain Stimul. 8, 535–550. doi: 10.1016/j.brs.2015.01.400
Horvath, J. C., Vogrin, S. J., Carter, O., Cook, M. J., and Forte, J. D. (2016). Effects of a common transcranial direct current stimulation (tDCS) protocol on motor evoked potentials found to be highly variable within individuals over 9 testing sessions. Exp. Brain Res. 234, 2629–2642. doi: 10.1007/s00221-016-4667-8
Hoy, K. E., Emonson, M. R., Arnold, S. L., Thomson, R. H., Daskalakis, Z. J., and Fitzgerald, P. B. (2013). Testing the limits: investigating the effect of tDCS dose on working memory enhancement in healthy controls. Neuropsychologia 51, 1777–1784. doi: 10.1016/j.neuropsychologia.2013.05.018
Hsu, T. Y., Tseng, P., Liang, W. K., Cheng, S. K., and Juan, C. H. (2014). Transcranial direct current stimulation over right posterior parietal cortex changes prestimulus alpha oscillation in visual short-term memory task. Neuroimage 98, 306–313. doi: 10.1016/j.neuroimage.2014.04.069
Ioannidis, J. P. (2005). Why most published research findings are false. PLoS Med. 2:e124. doi: 10.1371/journal.pmed.0020124
Jensen, O., and Mazaheri, A. (2010). Shaping functional architecture by oscillatory alpha activity: gating by inhibition. Front. Hum. Neurosci. 4:186. doi: 10.3389/fnhum.2010.00186
Jewell, G., and McCourt, M. E. (2000). Pseudoneglect: a review and meta-analysis of performance factors in line bisection tasks. Neuropsychologia 38, 93–110.
Kanai, R., Paulus, W., and Walsh, V. (2010). Transcranial alternating current stimulation (tACS) modulates cortical excitability as assessed by TMS-induced phosphene thresholds. Clin. Neurophysiol. 121, 1551–1554. doi: 10.1016/j.clinph.2010.03.022
Klimesch, W., Sauseng, P., and Hanslmayr, S. (2007). EEG alpha oscillations: the inhibition-timing hypothesis. Brain Res. Rev. 53, 63–88. doi: 10.1016/j.brainresrev.2006.06.003
Krause, B., and Cohen Kadosh, R. (2014). Not all brains are created equal: the relevance of individual differences in responsiveness to transcranial electrical stimulation. Front. Syst. Neurosci. 8:25. doi: 10.3389/fnsys.2014.00025
Kuo, H. I., Bikson, M., Datta, A., Minhas, P., Paulus, W., Kuo, M. F., et al. (2013). Comparing cortical plasticity induced by conventional and high-definition 4 x 1 ring tDCS: a neurophysiological study. Brain Stimul. 6, 644–648. doi: 10.1016/j.brs.2012.09.010
Laczo, B., Antal, A., Niebergall, R., Treue, S., and Paulus, W. (2012). Transcranial alternating stimulation in a high gamma frequency range applied over V1 improves contrast perception but does not modulate spatial attention. Brain Stimul. 5, 484–491. doi: 10.1016/j.brs.2011.08.008
Learmonth, G., Gallagher, A., Gibson, J., Thut, G., and Harvey, M. (2015). Intra- and inter-task reliability of spatial attention measures in pseudoneglect. PLoS ONE 10:e0138379. doi: 10.1371/journal.pone.0138379
Lefaucheur, J. P. (2016). A comprehensive database of published tDCS clinical trials (2005-2016). Neurophysiol. Clin. 46, 319–398. doi: 10.1016/j.neucli.2016.10.002
Li, L. M., Leech, R., Scott, G., Malhotra, P., Seemungal, B., and Sharp, D. J. (2015a). The effect of oppositional parietal transcranial direct current stimulation on lateralized brain functions. Eur. J. Neurosci. 42, 2904–2914. doi: 10.1111/ejn.13086
Li, L. M., Uehara, K., and Hanakawa, T. (2015b). The contribution of interindividual factors to variability of response in transcranial direct current stimulation studies. Front. Cell Neurosci. 9:181. doi: 10.3389/fncel.2015.00181
Loftus, A. M., and Nicholls, M. E. (2012). Testing the activation-orientation account of spatial attentional asymmetries using transcranial direct current stimulation. Neuropsychologia 50, 2573–2576. doi: 10.1016/j.neuropsychologia.2012.07.003
London, R. E., and Slagter, H. A. (2015). Effects of transcranial direct current stimulation over left dorsolateral pFC on the attentional blink depend on individual baseline performance. J. Cogn. Neurosci. 27, 2382–2393. doi: 10.1162/jocn_a_00867
Lopez-Alonso, V., Cheeran, B., Rio-Rodriguez, D., and Fernandez-Del-Olmo, M. (2014). Inter-individual variability in response to non-invasive brain stimulation paradigms. Brain Stimul. 7, 372–380. doi: 10.1016/j.brs.2014.02.004
Mancuso, L. E., Ilieva, I. P., Hamilton, R. H., and Farah, M. J. (2016). Does transcranial direct current stimulation improve healthy working memory?: A meta-analytic review. J. Cogn. Neurosci. 28, 1063–1089. doi: 10.1162/jocn_a_00956
Manly, T., Dobler, V. B., Dodds, C. M., and George, M. A. (2005). Rightward shift in spatial awareness with declining alertness. Neuropsychologia 43, 1721–1728. doi: 10.1016/j.neuropsychologia.2005.02.009
McCourt, M. E., and Olafson, C. (1997). Cognitive and perceptual influences on visual line bisection: psychophysical and chronometric analyses of pseudoneglect. Neuropsychologia 35, 369–380.
Mehta, A. R., Pogosyan, A., Brown, P., and Brittain, J. S. (2015). Montage matters: the influence of transcranial alternating current stimulation on human physiological tremor. Brain Stimul. 8, 260–268. doi: 10.1016/j.brs.2014.11.003
Milner, A. D., Brechmann, M., and Pagliarini, L. (1992). To halve and to halve not: an analysis of line bisection judgements in normal subjects. Neuropsychologia 30, 515–526.
Minarik, T., Berger, B., Althaus, L., Bader, V., Biebl, B., Brotzeller, F., et al. (2016). the importance of sample size for reproducibility of tDCS effects. Front. Hum. Neurosci. 10:453. doi: 10.3389/fnhum.2016.00453
Moliadze, V., Antal, A., and Paulus, W. (2010). Electrode-distance dependent after-effects of transcranial direct and random noise stimulation with extracephalic reference electrodes. Clin. Neurophysiol. 121, 2165–2171. doi: 10.1016/j.clinph.2010.04.033
Moos, K., Vossel, S., Weidner, R., Sparing, R., and Fink, G. R. (2012). Modulation of top-down control of visual attention by cathodal tDCS over right IPS. J. Neurosci. 32, 16360–16368. doi: 10.1523/JNEUROSCI.6233-11.2012
Neuling, T., Rach, S., and Herrmann, C. S. (2013). Orchestrating neuronal networks: sustained after-effects of transcranial alternating current stimulation depend upon brain states. Front. Hum. Neurosci. 7:161. doi: 10.3389/fnhum.2013.00161
Neuling, T., Ruhnau, P., Fusca, M., Demarchi, G., Herrmann, C. S., and Weisz, N. (2015). Friends, not foes: magnetoencephalography as a tool to uncover brain dynamics during transcranial alternating current stimulation. Neuroimage 118, 406–413. doi: 10.1016/j.neuroimage.2015.06.026
Newman, D. P., Loughnane, G. M., Kelly, S. P., O’connell, R. G., and Bellgrove, M. A. (2017). Visuospatial asymmetries arise from differences in the onset time of perceptual evidence accumulation. J. Neurosci. 37, 3378–3385. doi: 10.1523/JNEUROSCI.3512-16.2017
Newman, D. P., O’connell, R. G., and Bellgrove, M. A. (2013). Linking time-on-task, spatial bias and hemispheric activation asymmetry: a neural correlate of rightward attention drift. Neuropsychologia 51, 1215–1223. doi: 10.1016/j.neuropsychologia.2013.03.027
Nitsche, M. A., Doemkes, S., Karakose, T., Antal, A., Liebetanz, D., Lang, N., et al. (2007). Shaping the effects of transcranial direct current stimulation of the human motor cortex. J. Neurophysiol. 97, 3109–3117. doi: 10.1152/jn.01312.2006
Nitsche, M. A., and Paulus, W. (2000). Excitability changes induced in the human motor cortex by weak transcranial direct current stimulation. J. Physiol. 527(Pt 3), 633–639.
Nitsche, M. A., Seeber, A., Frommann, K., Klein, C. C., Rochford, C., Nitsche, M. S., et al. (2005). Modulating parameters of excitability during and after transcranial direct current stimulation of the human motor cortex. J. Physiol. 568, 291–303. doi: 10.1113/jphysiol.2005.092429
Ozen, S., Sirota, A., Belluscio, M. A., Anastassiou, C. A., Stark, E., Koch, C., et al. (2010). Transcranial electric stimulation entrains cortical neuronal populations in rats. J. Neurosci. 30, 11476–11485. doi: 10.1523/JNEUROSCI.5252-09.2010
Parkin, B. L., Ekhtiari, H., and Walsh, V. F. (2015). Non-invasive human brain stimulation in cognitive neuroscience: a primer. Neuron 87, 932–945. doi: 10.1016/j.neuron.2015.07.032
Pikovsky, A., Rosenblum, M., and Kurths, J. (2003). Synchronization: A Universal Concept in Nonlinear Sciences. Cambridge: Cambridge University Press.
Pirulli, C., Fertonani, A., and Miniussi, C. (2013). The role of timing in the induction of neuromodulation in perceptual learning by transcranial electric stimulation. Brain Stimul. 6, 683–689. doi: 10.1016/j.brs.2012.12.005
Roe, J. M., Nesheim, M., Mathiesen, N. C., Moberget, T., Alnaes, D., and Sneve, M. H. (2016). The effects of tDCS upon sustained visual attention are dependent on cognitive load. Neuropsychologia 80, 1–8. doi: 10.1016/j.neuropsychologia.2015.11.005
Romei, V., Brodbeck, V., Michel, C., Amedi, A., Pascual-Leone, A., and Thut, G. (2008). Spontaneous fluctuations in posterior alpha-band EEG activity reflect variability in excitability of human visual areas. Cereb. Cortex 18, 2010–2018. doi: 10.1093/cercor/bhm229
Romei, V., Gross, J., and Thut, G. (2010). On the role of prestimulus alpha rhythms over occipito-parietal areas in visual input regulation: correlation or causation? J. Neurosci. 30, 8692–8697. doi: 10.1523/JNEUROSCI.0160-10.2010
Romero Lauro, L. J., Rosanova, M., Mattavelli, G., Convento, S., Pisoni, A., Opitz, A., et al. (2014). TDCS increases cortical excitability: direct evidence from TMS-EEG. Cortex 58, 99–111. doi: 10.1016/j.cortex.2014.05.003
Rossi, S., Hallett, M., Rossini, P. M., and Pascual-Leone, A. (2009). Safety, ethical considerations, and application guidelines for the use of transcranial magnetic stimulation in clinical practice and research. Clin. Neurophysiol. 120, 2008–2039. doi: 10.1016/j.clinph.2009.08.016
Ruzzoli, M., and Soto-Faraco, S. (2014). Alpha stimulation of the human parietal cortex attunes tactile perception to external space. Curr. Biol. 24, 329–332. doi: 10.1016/j.cub.2013.12.029
Santarnecchi, E., Brem, A. K., Levenbaum, E., Thompson, T., Cohen Kadosh, R., and Pascual-Leone, A. (2015). Enhancing cognition using transcranial electrical stimulation. Curr. Opin. Behav. Sci. 4, 171–178. doi: 10.1016/j.cobeha.2015.06.003
Sarkar, A., Dowker, A., and Cohen Kadosh, R. (2014). Cognitive enhancement or cognitive cost: trait-specific outcomes of brain stimulation in the case of mathematics anxiety. J. Neurosci. 34, 16605–16610. doi: 10.1523/JNEUROSCI.3129-14.2014
Scheldrup, M., Greenwood, P. M., Mckendrick, R., Strohl, J., Bikson, M., Alam, M., et al. (2014). Transcranial direct current stimulation facilitates cognitive multi-task performance differentially depending on anode location and subtask. Front. Hum. Neurosci. 8:665. doi: 10.3389/fnhum.2014.00665
Schmitz, R., Deliens, G., Mary, A., Urbain, C., and Peigneux, P. (2011). Selective modulations of attentional asymmetries after sleep deprivation. Neuropsychologia 49, 3351–3360. doi: 10.1016/j.neuropsychologia.2011.08.009
Schutter, D. J., and Wischnewski, M. (2016). A meta-analytic study of exogenous oscillatory electric potentials in neuroenhancement. Neuropsychologia 86, 110–118. doi: 10.1016/j.neuropsychologia.2016.04.011
Schwarzkopf, D. S., De Haas, B., and Rees, G. (2012). Better ways to improve standards in brain-behavior correlation analysis. Front. Hum. Neurosci. 6:200. doi: 10.3389/fnhum.2012.00200
Sehm, B., Kipping, J., Schafer, A., Villringer, A., and Ragert, P. (2013). A comparison between Uni- and bilateral tDCS effects on functional connectivity of the human motor cortex. Front. Hum. Neurosci. 7:183. doi: 10.3389/fnhum.2013.00183
Sparing, R., Thimm, M., Hesse, M. D., Kust, J., Karbe, H., and Fink, G. R. (2009). Bidirectional alterations of interhemispheric parietal balance by non-invasive cortical stimulation. Brain 132, 3011–3020. doi: 10.1093/brain/awp154
Stagg, C. J., and Nitsche, M. A. (2011). Physiological basis of transcranial direct current stimulation. Neuroscientist 17, 37–53. doi: 10.1177/1073858410386614
Szczepanski, S. M., and Kastner, S. (2013). Shifting attentional priorities: control of spatial attention through hemispheric competition. J. Neurosci. 33, 5411–5421. doi: 10.1523/JNEUROSCI.4089-12.2013
Tecchio, F., Cancelli, A., Cottone, C., Tomasevic, L., Devigus, B., Zito, G., et al. (2013). Regional personalized electrodes to select transcranial current stimulation target. Front. Hum. Neurosci. 22:131. doi: 10.3389/fnhum.2013.00131
Teo, F., Hoy, K. E., Daskalakis, Z. J., and Fitzgerald, P. B. (2011). Investigating the role of current strength in tDCS modulation of working memory performance in healthy controls. Front. Psychiatry 2:45. doi: 10.3389/fpsyt.2011.00045
Thiebaut de Schotten, M., Dell’acqua, F., Forkel, S. J., Simmons, A., Vergani, F., Murphy, D. G., et al. (2011). A lateralized brain network for visuospatial attention. Nat. Neurosci. 14, 1245–1246. doi: 10.1038/nn.2905
Thielscher, A., Antunes, A., and Saturnino, G. B. (2015). Field modeling for transcranial magnetic stimulation: a useful tool to understand the physiological effects of TMS? Conf. Proc. IEEE Eng. Med. Biol. Soc. 2015, 222–225. doi: 10.1109/EMBC.2015.7318340
Thut, G., Bergmann, T. O., Frohlich, F., Soekadar, S. R., Brittain, J. S., Valero-Cabre, A., et al. (2017). Guiding transcranial brain stimulation by EEG/MEG to interact with ongoing brain activity and associated functions: a position paper. Clin. Neurophysiol. 28, 843–857. doi: 10.1016/j.clinph.2017.01.003
Thut, G., Nietzel, A., Brandt, S. A., and Pascual-Leone, A. (2006). Alpha-band electroencephalographic activity over occipital cortex indexes visuospatial attention bias and predicts visual target detection. J. Neurosci. 26, 9494–9502. doi: 10.1523/JNEUROSCI.0875-06.2006
Toraldo, A., McIntosh, R. D., Dijkerman, H. C., and Milner, A. D. (2004). A revised method for analysing neglect using the landmark task. Cortex 40, 415–431.
Tseng, P., Hsu, T. Y., Chang, C. F., Tzeng, O. J., Hung, D. L., Muggleton, N. G., et al. (2012). Unleashing potential: transcranial direct current stimulation over the right posterior parietal cortex improves change detection in low-performing individuals. J. Neurosci. 32, 10554–10561. doi: 10.1523/JNEUROSCI.0362-12.2012
Vannorsdall, T. D., Van Steenburgh, J. J., Schretlen, D. J., Jayatillake, R., Skolasky, R. L., and Gordon, B. (2016). Reproducibility of tDCS results in a randomized trial: failure to replicate findings of tDCS-induced enhancement of verbal fluency. Cogn. Behav. Neurol. 29, 11–17. doi: 10.1097/WNN.0000000000000086
Varnava, A., Dervinis, M., and Chambers, C. D. (2013). The predictive nature of pseudoneglect for visual neglect: evidence from parietal theta burst stimulation. PLoS ONE 8:e65851. doi: 10.1371/journal.pone.0065851
Veniero, D., Vossen, A., Gross, J., and Thut, G. (2015). Lasting EEG/MEG aftereffects of rhythmic transcranial brain stimulation: level of control over oscillatory network activity. Front. Cell Neurosci. 9:477. doi: 10.3389/fncel.2015.00477
Verhagen, J., and Wagenmakers, E. J. (2014). Bayesian tests to quantify the result of a replication attempt. J. Exp. Psychol. Gen. 143, 1457–1475. doi: 10.1037/a0036731
Wach, C., Krause, V., Moliadze, V., Paulus, W., Schnitzler, A., and Pollok, B. (2013). Effects of 10 Hz and 20 Hz transcranial alternating current stimulation (tACS) on motor functions and motor cortical excitability. Behav. Brain Res. 241, 1–6. doi: 10.1016/j.bbr.2012.11.038
Walsh, V. Q. (2013). Ethics and social risks in brain stimulation. Brain Stimul. 6, 715–717. doi: 10.1016/j.brs.2013.08.001
Weiss, P. H., Marshall, J. C., Wunderlich, G., Tellmann, L., Halligan, P. W., Freund, H. J., et al. (2000). Neural consequences of acting in near versus far space: a physiological basis for clinical dissociations. Brain 123(Pt 12), 2531–2541.
Wiethoff, S., Hamada, M., and Rothwell, J. C. (2014). Variability in response to transcranial direct current stimulation of the motor cortex. Brain Stimul. 7, 468–475. doi: 10.1016/j.brs.2014.02.003
Wright, J. M., and Krekelberg, B. (2014). Transcranial direct current stimulation over posterior parietal cortex modulates visuospatial localization. J. Vis. 14:5. doi: 10.1167/14.9.5
Keywords: tACS, tDCS, landmark task, replication, tES reliability
Citation: Veniero D, Benwell CSY, Ahrens MM and Thut G (2017) Inconsistent Effects of Parietal α-tACS on Pseudoneglect across Two Experiments: A Failed Internal Replication. Front. Psychol. 8:952. doi: 10.3389/fpsyg.2017.00952
Received: 28 March 2017; Accepted: 23 May 2017;
Published: 08 June 2017.
Edited by:
Joachim Lange, Heinrich Heine Universität Düsseldorf, GermanyReviewed by:
Til Ole Bergmann, University of Tübingen, GermanyPhilipp Ruhnau, Otto von Guericke University Magdeburg, Germany
Copyright © 2017 Veniero, Benwell, Ahrens and Thut. This is an open-access article distributed under the terms of the Creative Commons Attribution License (CC BY). The use, distribution or reproduction in other forums is permitted, provided the original author(s) or licensor are credited and that the original publication in this journal is cited, in accordance with accepted academic practice. No use, distribution or reproduction is permitted which does not comply with these terms.
*Correspondence: Domenica Veniero, ZG9tZW5pY2EudmVuaWVyb0BnbGFzZ293LmFjLnVr Gregor Thut, Z3JlZ29yLnRodXRAZ2xhc2dvdy5hYy51aw==
†These authors have contributed equally to this work.