- 1Key Laboratory of Plant Resource Conservation and Germplasm Innovation in Mountainous Region (Ministry of Education), College of Life Sciences, Guizhou University, Guiyang, Guizhou, China
- 2Center for Water and Environmental Studies, College of Agricultural and Food Sciences, King Faisal University, Al-Ahsa, Saudi Arabia
- 3Department of Botany, The Islamia University of Bahawalpur, Bahawalpur, Pakistan
- 4Laboratory of Green Pesticide and Agricultural Bioengineering, Ministry of Education, Guizhou University, Guiyang, Guizhou, China
- 5Department of Biology, College of Science, University of Lahore, Lahore, Pakistan
- 6Department of Plant Pathology, Faculty of Agriculture and Environment, The Islamia University of Bahawalpur, Bahawalpur, Pakistan
- 7College of Agriculture, Guizhou University/College of Life Sciences, Guiyang, China
- 8Key Laboratory for Tropical Plant Improvement and Sustainable Use, Xishuangbanna Tropical 20 Botanical Garden, Chinese Academy of Sciences, Menglun, China
Ensuring global food security and achieving sustainable agricultural productivity remains one of the foremost challenges of the contemporary era. The increasing impacts of climate change and environmental stressors like drought, salinity, and heavy metal (HM) toxicity threaten crop productivity worldwide. Addressing these challenges demands the development of innovative technologies that can increase food production, reduce environmental impacts, and bolster the resilience of agroecosystems against climate variation. Nanotechnology, particularly the application of nanoparticles (NPs), represents an innovative approach to strengthen crop resilience and enhance the sustainability of agriculture. NPs have special physicochemical properties, including a high surface-area-to-volume ratio and the ability to penetrate plant tissues, which enhances nutrient uptake, stress resistance, and photosynthetic efficiency. This review paper explores how abiotic stressors impact crops and the role of NPs in bolstering crop resistance to these challenges. The main emphasis is on the potential of NPs potential to boost plant stress tolerance by triggering the plant defense mechanisms, improving growth under stress, and increasing agricultural yield. NPs have demonstrated potential in addressing key agricultural challenges, such as nutrient leaching, declining soil fertility, and reduced crop yield due to poor water management. However, applying NPs must consider regulatory and environmental concerns, including soil accumulation, toxicity to non-target organisms, and consumer perceptions of NP-enhanced products. To mitigate land and water impacts, NPs should be integrated with precision agriculture technologies, allowing targeted application of nano-fertilizers and nano-pesticides. Although further research is necessary to assess their advantages and address concerns, NPs present a promising and cost-effective approach for enhancing food security in the future.
Introduction
Climate change and agriculture
Abrupt environmental changes are intensifying their negative impacts on the negative impacts on crop physiology, growth, development, and productivity. Environmental stressors such as drought, salt, temperature stress, and HM toxicity have become more frequent and severe, leading to a substantial decline in global agricultural output. This situation is further compounded by the growing global population, projected to exceed 9 billion by 2050, intensifying the demand for food systems to sustainably increase production.
Traditional chemical-based farming practices have been proven proven insufficient in meeting these demands. The extensive dependence on agrochemicals and pesticides has significant adverse effects, including soil degradation, a decline in biodiversity, and environmental pollution (Tripathi et al., 2020; Brunelle et al., 2024). For example, excessive use of nitrogen-based fertilizers, induces soil acidification, which reduces nutrient availability and disrupts the soil’s microbial ecosystem, ultimately lowering soil productivity. Pesticide use, particularly with organophosphates and carbamates, has reduced populations of beneficial soil microorganisms, weakening soil structure and fertility. In addition, traditional irrigation practices have exacerbated issues like water scarcity and soil salinity (Mohanavelu et al., 2021; Tarolli et al., 2024). These challenges highlight the urgent need for sustainable agricultural technologies to enhance crop resilience to environmental stressors and improve yield.
Impacts of abiotic stress on crop production
Agriculture is essential for maintaining global food security and supporting the livelihoods of a large segment of the global population. Therefore, protecting this vital sector from environmental stressors, particularly those intensified by climate change, is crucial. Abiotic stresses, such as drought, salinity, and HM toxicity, are responsible for 20-50% of annual global crop yield losses. Under adverse conditions, plants experience impaired physiological functions, including reduced photosynthesis, nutrient absorption, and water uptake, which can slow growth and, in extreme cases, lead to complete crop failure, especially in sensitive species like maize (Li et al., 2021b).
Abiotic stress encompasses external factors that disrupt normal plant processes and cause physiological and biochemical changes that impact agricultural yield. These stressors, such as severe climatic conditions, including drought, salinity, and temperature fluctuations, disrupt normal plant processes and lead to physiological and biochemical changes, ultimately impacting agricultural yield (Ghani et al., 2022; Kareem et al., 2022; Hassan et al., 2023). Drought stress, for instance, significantly decreases nitrogen uptake efficiency, diminishing the effectiveness of fertilization, while salinity stress imposes osmotic pressure on plants, increasing their vulnerability to other abiotic factors. These combined environmental stressors can significantly reduce the adaptive capacity of ecosystems to respond to fluctuating environmental conditions (Han et al., 2022; Anwar et al., 2023).
Climate change poses significant threats to agriculture in the modern world, impacting crop physiology, growth, development, and productivity.
Role of nanotechnology in agriculture
Nanotechnology, particularly the use of NPs, has emerged as a key player with significant potential to enhance crop resilience and productivity. NPs exhibit special physicochemical properties, including a large surface-area-to-volume ratio and the ability to cross biological membranes, facilitating targeted nutrient delivery and stress resistance (Singh et al., 2024). NPs can enhance nutrient uptake, improve stress resistance, and increase photosynthetic efficiency (Figure 1), resulting in better growth and yield even in challenging conditions (Singh et al., 2024).
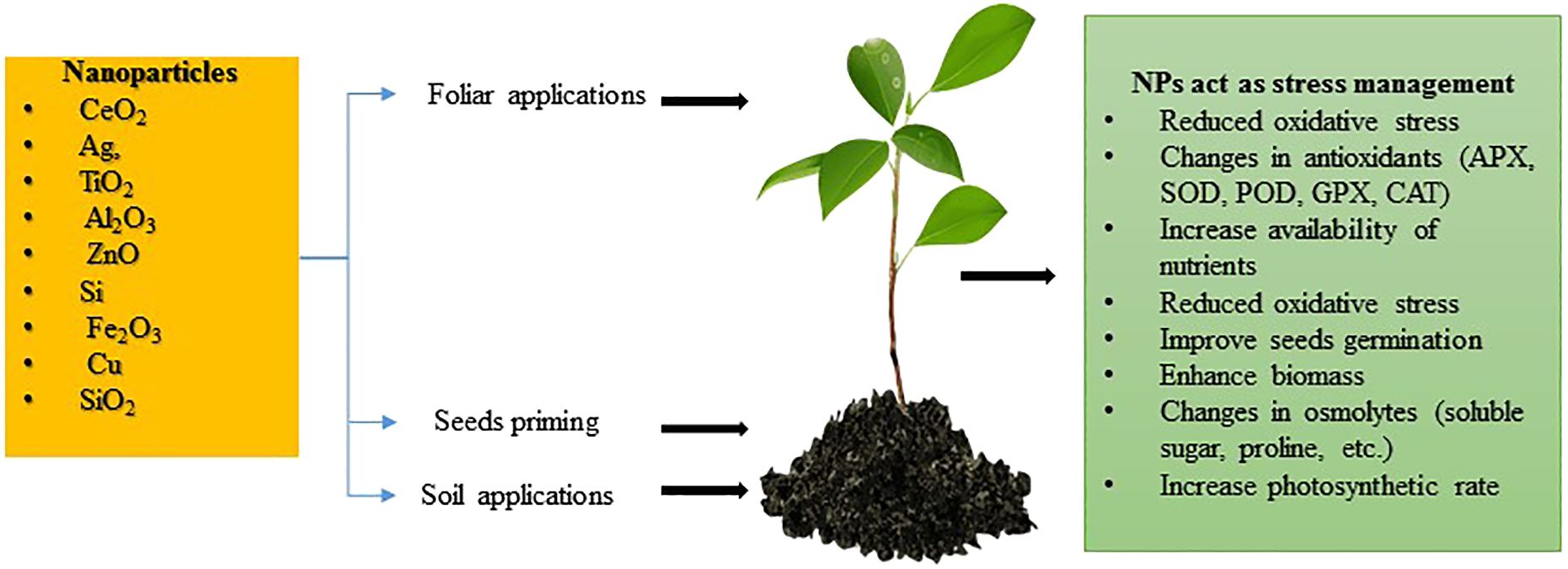
Figure 1. Effect of different NPs and their different applications on seed germination, performance and physiological parameters and photosynthetic rate.
Advancement in agriculture, particularly via the adoption of nanotechnology, is essential in the modern world. NPs possess exceptional chemical and physical properties that enable them to penetrate cellular membranes and interact effectively with biological systems. In agriculture, nanoscale products, such as nano-pesticides, nano-fungicides, nano-herbicides, and nano-fertilizers, offer significant benefits. They can improve plant growth and reduce the environmental footprint of conventional agricultural chemicals (Wahab et al., 2024). These nanoparticles are effective even at low concentrations, and can be delivered through various methods, including seed treatment, foliar spraying, and hydroponic delivery (Nile et al., 2022; Mawale et al., 2024). Unlike traditional fertilizers that often leach into water bodies and cause pollution, nanoparticles provide controlled, efficient nutrient release, ensuring plants receive the necessary elements over time (Easwaran et al., 2024; Haydar et al., 2024). For instance, they can improve the delivery of vital nutrients like nitrogen (N), phosphorus (P), and potassium (K), reducing environmental runoff and eutrophication (Sheikhalipour et al., 2021; Jakhar et al., 2022). Nanotechnology also offers potential in mitigating specific abiotic stresses, such as drought and salinity, by enhancing water-use efficiency and osmotic adjustment in plants. Although the application of NPs in agriculture is still in its developmental stages, it holds promise for increasing crop resilience against various stressors (Su et al., 2019; Haydar et al., 2024) (Table 1). An overview of studies investigating the impact of various NPs on different plant components is provided.
Challenges and considerations for nanotechnology use in agriculture
NPs are gaining attention in agriculture as a highly effective strategy for reducing abiotic stresses and serving as nano-fertilizers. They can stimulate plant growth, enhance nutrient uptake, and strengthen resilience to environmental stressors.
Despite their potential, the widespread adoption of nanoparticles in agriculture faces challenges. Concerns remain about the long-term effects of NPs on plant systems, soil microorganisms, and human health. For instance, while nanoparticles can enhance plant health, their impact on non-target organisms and overall ecosystem balance is not fully understood. Additionally, the cost-effectiveness of nanoparticle production is a critical factor that influences their feasibility for large-scale agricultural use. Addressing these concerns is vital for the responsible integration of NPs in agriculture. Ensuring the safety, environmental compatibility, and economic viability of nanotechnology will be essential to its sustainable implementation. This review aims to explore how nanoparticles can address agricultural challenges and identify environmentally friendly solutions. The main objectives are: (1) to analyze the adverse effects of abiotic stresses, such as drought, salinity, HM, and temperature changes, on crop yield and assess whether nanoparticles can mitigate these stressors and enhance yield; (2) to discuss the role of nanomaterials, including stable synthetic variants, in agriculture and evaluate their safety for humans and the environment; (3) to examine the cost-effectiveness of producing and utilizing nanoparticles in agricultural activities and determine their efficacy; and (4) to propose strategies for the safe incorporation of nanotechnology into agricultural practices and measures to optimize nanoparticle use.
Mechanisms of nanoparticles in enhancing crop resilience
Nanoparticles physicochemical properties
NPs, due to their distinct characteristics, are considered effective in alleviating abiotic stress in plants. Their large surface area relative to volume increases reactivity, facilitating more efficient interactions with plant cells (El-Saadony et al., 2022). This property allows NPs to dissolve readily and penetrate plant cell membranes, thereby enabling the targeted delivery of nutrients and stress-mitigating agents (Yadav et al., 2023). Once NPs penetrate plant tissues either through root uptake or foliar retention, they translocate throughout the plant using via both apoplastic and symplastic routes. This localization and movement within plant tissues contribute to increased resistance against various abiotic stress factors, ultimately stimulating plant growth under challenging conditions (Ali et al., 2021b). By reaching intracellular targets, NPs can directly influence metabolic processes directly, improving nutrient uptake and stress resistance. This capability is particularly beneficial in enhancing photosynthetic efficiency and promoting plant growth under adverse environmental conditions (Singh et al., 2021b).
Defense mechanism
Plants have developed various defense mechanisms to cope with environmental stressors, utilizing both biochemical and physiological pathways to minimize damage (Haile et al., 2020; Hassan et al., 2023). While ongoing research continues to unravel these complex protective processes, certain mechanisms have been identified as essential for plant resilience. NPs are emerging as a promising tool for enhancing these defenses, as they help to strengthen plant defense by boosting the antioxidant activity, promoting, osmolyte accumulation, and activating genes associated with stress tolerance (Ali et al., 2019; Ahmed et al., 2021).
One of the primary ways NPs aid plant defenses is by enhancing antioxidant systems, which are crucial for neutralizing reactive oxygen species (ROS). Under conditions of environmental stress, such as drought, salinity, and UV exposure plants produce higher levels of ROS, which can damage cellular components like lipids, proteins, and DNA. NPs, particularly those containing titanium dioxide (TiO₂) and silicon (SiO₂), have shown the ability to alleviate oxidative stress by increasing the activity of key antioxidant enzymes, including superoxide dismutase (SOD) and catalase (CAT) (Ye et al., 2019). For example, TiO₂ NPs can function as catalysts in redox reactions, helping to detoxify ROS and protect plant tissues from UV-induced damage. Similarly, SiO₂ NPs have been found to enhance antioxidant defenses in wheat, helping to mitigate the damage caused by UV-B radiation. This enhancement of antioxidant activity helps maintain cellular integrity under stress, enabling plants to better endure challenging conditions.
In addition, to bolstering antioxidant defenses, NPs stimulate the accumulation of osmolytes, small organic compounds such as trehalose, proline, and glycine betaine, that play a crucial role in osmoregulation. These compounds help plants retain water, stabilize proteins, and protect cell membranes during periods of water deficit and high salinity conditions (Sarkar et al., 2024). Proline, for instance, acts as an osmoprotectant by maintaining cell turgor and facilitating cellular functions in low-water conditions. Similarly, glycine betaine and trehalose support membrane stability and protein structure under stress. By promoting osmolyte buildup, NPs help plants manage osmotic stress, improving their resilience in arid and saline environments.
NPs also appear to enhance stress tolerance at the genetic level by activating the expression of certain stress-related genes. Research has shown that NPs like silicon (SiO₂) and TiO₂ can trigger the transcription of genes involved in stress responses, including those that encode heat-shock proteins (HSPs) and late embryogenesis abundant (LEA) proteins (Zulfiqar and Ashraf, 2021a). These proteins play a critical role in protecting cellular structures under extreme stress conditions, further enhancing the plants ability to survive in harsh environments. By influencing these molecular pathways, NPs not only improve immediate stress resistance but may also contribute to longer-term adaptation to adverse conditions.
In general, the application of NPs in agriculture presents a potential approach for increasing crop resilience by bolstering plant defenses by using their distinct physicochemical characteristics and capacity to trigger the plant defense response. This approach improves nutrient delivery, stress resistance, and supports sustainable agricultural productivity in the face of increasing environmental challenges.
UV radiation and oxidative stress in plants
Ultraviolet (UV) radiation is a significant abiotic stressor that induces oxidative stress in plants. Exposure to UV-B light leads to abnormal leaf structure and reduced photosynthetic efficiency, prompting plant cells to produce ROS. Additionally, UV-B exposure increases lipid peroxidation and the extracellular release of electrolytes through the generation of H2O2 and O2- radicals. The elevated levels of ROS may result from the inhibition of SOD and APX activities due to UV-B (Takshak and Agrawal, 2014). TiO2 nanoparticles operate as catalysts in an oxidation-reduction process that produces superoxide anion radicals and hydroxide when exposed to light (Zou et al., 2013). Si NPs boost antioxidant activity in wheat, helping to reduce oxidative damage induced by UV-B exposure (MS et al., 2021). This can be inferred that Si NPs provide protection to plants by triggering the antioxidant defense system and mitigating the photosynthetic damage caused by ROS. Si NPs are more effective than bulk silicon in reducing UV-B stress in wheat seedlings (Ayub et al., 2022).
Multi-walled carbon nanotubes (MWCNTs) protect the model plant Arabidopsis thaliana by alleviating paraquat-induced oxidative stress. MWCNTs enhance photosynthetic efficiency and stimulate plant lateral root growth, and mitigate the bioavailability of toxic substances (Fan et al., 2018; Igiebor et al., 2023). NPs have demonstrated beneficial effects in mitigating oxidative stress in plants, but it is essential to carefully design approaches to minimize their possible toxicity and optimize their properties. Factors such as size, shape, and surface characteristics should be tailored to ensure the optimal performance of NPs while minimizing environmental risks (Chen et al., 2023).
Optimizing nanoparticle properties for enhanced efficacy and reduced toxicity
NPs have shown the potential to mitigate oxidative stress in plants, improving their tolerance to environmental stressors (Thiruvengadam et al., 2024). However, careful design is crucial to minimize any risks associated with toxicity, ensuring NPs deliver their benefits without harmful side effects to plants or ecosystems. Therefore, safe and efficient production strategies should emphasize refining nanoparticle properties to maximize beneficial effects while minimizing adverse impacts (Iavicoli et al., 2017). In this regard, developing nanoparticles with optimized properties such as size, shape, and surface characteristics becomes essential for maximizing benefits while minimizing environmental risks. Smaller nanoparticles tend to be more efficiently absorbed and transported within plant tissues, and specific shapes, such as rod-like or tubular structures, can enhance interactions with plant cells (Zuverza-Mena et al., 2017; Wang et al., 2023). Additionally, surface modifications, such as applying biocompatible coatings, further improve nanoparticle stability, reduce aggregation, and lower toxicity by regulating how these particles interact with cell membranes (Mansouri et al., 2023). By carefully customizing these features, researchers can create nanoparticles that support plant health in a safer, more sustainable way, advancing eco-friendly applications of nanotechnology in agriculture (Yang et al., 2017a).
Dose-dependent effects of nanoparticles on plant growth and toxicity
Previous research has demonstrated that the toxicity of NPs is highly dependent on their concentration, exposure time, and specific plant species (Ma et al., 2015; Cox et al., 2016; Khan et al., 2021). Certain NPs, such as TiO2, have demonstrated the ability to enhance plant development at low concentrations, while excessive accumulation at higher concentrations can lead to the overproduction of ROS, ultimately inhibiting growth. This dose-dependent response underscores the importance of carefully controlling NP exposure levels to achieve the desired beneficial effects while avoiding potential phytotoxicity (Yang et al., 2017b). TiO2 NPs were also demonstrated to increase SOD activity, with the influence increasing with NPs concentration. At 40 and 50 mg/mL concentrations, TiO2 NPs resulted in the lowest CAT and POD activity, whereas POD and amylase enzyme secretion were highest and lowest, respectively (Javed et al., 2022). The findings of these studies suggest that NPs are important for enhancing agricultural productivity, however, a full understanding of the right process and how NPs interact with plants at different levels is still evolving (Fincheira et al., 2020).
Jampílek and Kráľová (2022) investigation has shown that CuNPs exert an impact on the development of Oryza sativa (rice) and Lactuca sativa (lettuce). At low concentrations (0.8 to 798.9 mg/L), CuNPs promoted root growth. However, the phytotoxicity of this substance escalated with increasing concentration, leading to a reduction in the number of thylakoids and stomatal conductivity. This indicates that while low and medium doses are beneficial to plants, high doses can be toxic.
Various metal oxide nanoparticles, including those of copper, zinc, and silver, have been found to exhibit phytotoxic effects on plants. The magnitude of these hazards may vary across the crop, species, and cultivars (Xiong et al., 2017; Mahawar et al., 2024). Understanding the specific phytotoxic thresholds and mechanisms for each type of NP is crucial for developing safe and effective applications in agriculture (Tuga et al., 2023). CuNPs stressed Oryza sativa, resulting in a decrease in the rate of photosynthetic activity, the number of thylakoids in each granum, the rate of transpiration, and the conductivity of the stomatal cells (Aqeel et al., 2022).
ZnO NPs also exhibit phytotoxicity. Exposure to 100 and 1000 mg/L ZnO NPs in Salicornia persica resulted in a 50% reduction in shoot length, accompanied by increased levels of ROS production and lipid peroxidation compared to untreated plants. Similar effects on growth parameters were observed in Cajanus cajan seeds exposed to varying concentrations of ZnO NPs (Tortella et al., 2023). Additionally, the phytotoxicity of ZnO NPs is influenced by soil pH and plant species. Notably, acidic soils exhibited more pronounced toxic effects compared to alkaline soils, where the presence of Zn is restricted. The aforementioned observation suggests that environmental variables substantially influence the phytotoxic reaction to ZnO NPs (Fasake et al., 2021). It is crucial to emphasize that both soil pH and plant species significantly affect the phytotoxicity of ZnO NPs, as well as the damage caused by metal or metal oxide nanoparticles (Sturikova et al., 2018).
Cytotoxicity and phytotoxicity are also associated with silver nanoparticles (AgNPs). For instance, biogenic AgNPs synthesized using Aloe vera extract caused significant injury to Brassica seedlings in hydroponic systems. This stress resulted from oxidative effects that led to cell death and DNA damage. Although the toxicity of AgNPs was not as pronounced as that of silver nitrate, they still exhibited significant toxicity in certain concentrations (Feregrino-Pérez et al., 2023).
The surface characteristics of AgNPs significantly influence their phytotoxicity. NPs with a negative charge and Ag⁺ ions are particularly detrimental to plants (Sarkar et al., 2022). In contrast, titanium dioxide (TiO2NPs) are generally less phytotoxic than many other metal oxide nanoparticles, making them safer for plant applications (Irshad et al., 2021).
AgNPs with distinct properties can restrict the growth in model monocot and dicot plants to varying degrees. The synthesis of these AgNPs involved the use of trisodium citrate, tannic acid, and cysteamine hydrochloride, leading to the formation of nanoparticles with well-defined surface charges. Exposure to these silver nanoparticles adversely affected the roots and shoots of both monocot and dicot plants. Notably, negatively charged AgNPs and silver ions derived from silver nitrate (AgNO3) exhibited greater toxicity to plants (Cox et al., 2016).
Although TiO2 NPs have been assessed for their phytotoxic effects, they are generally less harmful to plants compared to metal or metal oxide NPs (Rastogi et al., 2017). The application of TiO2 NPs may pose less risk in terms of phytotoxicity, particularly if growth inhibition, alterations in root water transport, cell membrane damage, ROS generation, or chlorophyll synthesis inhibition are observed (Mir et al., 2021). Recent studies suggest that while TiO2 NPs can be phytotoxic, they may also exhibit hormetic effects, promoting shoot and root elongation as well as overall biomass growth at specific concentrations, such as 100 mg/L. However, these beneficial effects diminish at higher concentrations, particularly beyond 1000 mg/L (Maluin et al., 2021). However, there was a noticeable limitation at concentrations over 1000 mg/L. The use of NPs in plants has shown a number of favorable impacts. To avoid harm and unfavorable effects on non-target species, the dose must be calculated based on crop type, soil, and other considerations (Tortella et al., 2023).
Long-term impacts of nanoparticles on soil ecosystem
While the integration of nanoparticles (NPs) into agricultural practices offers promising benefits for crop resilience and productivity, understanding their long-term effects on soil ecosystems remains crucial. Research has shown that NPs can persist in the environment and accumulate in soil, potentially altering the soil’s chemical and biological properties. The interactions between NPs and soil microorganisms, which play a pivotal role in maintaining soil health and nutrient cycles, are particularly concerning. Changes in microbial community structures can affect soil fertility, organic matter decomposition, and nutrient availability, potentially leading to disruptions in ecosystem services over time (Bakshi et al., 2015; Donia and Carbone, 2019).
The behavior of NPs in soil is influenced by several factors, including their size, surface charge, and coating, as well as soil pH and organic matter content. Studies indicate that certain metal and metal oxide nanoparticles, such as zinc oxide (ZnO) and TiO2, can exert phytotoxic effects that may vary with soil type and plant species (Ghori et al., 2019). Additionally, the formation of a protein corona around NPs can affect their absorption and movement through soil matrices, potentially impacting the bioavailability of essential nutrients and toxic metals (Huang et al., 2017).
Understanding the cumulative effects of NPs on soil ecosystems is vital for assessing their long-term sustainability. Potential outcomes include reduced microbial diversity, shifts in enzymatic activity, and altered soil-plant interactions, which could compromise agricultural productivity in the long run. These impacts necessitate further investigation through long-term field studies that assess NP behavior, transport, and transformation in soil environments under different climatic conditions.
Management of abiotic stress from nanoparticles
Plants encounter numerous challenges from abiotic stressors, including drought, salinity, alkalinity, submersion, and deficiencies in minerals and metals. These environmental factors hinder plant growth and significantly reduce agricultural productivity (Ma et al., 2015; Li et al., 2021b; Kareem et al., 2022; Mahawar et al., 2024). Among these, salinity, drought, extreme temperatures, and heavy metals (HMs) are the predominant factors contributing to decreased agricultural productivity. Plants experience a range of abiotic stressors throughout their life cycle and have evolved sophisticated physiological processes to withstand these challenges (Ma et al., 2015; Ghani et al., 2022; Anwar et al., 2023). In response to these stressors, plants modulate gene expression to adapt and alleviate their impact, therefore facilitating their ability to handle unfavorable environmental conditions (Pathak et al., 2021).
Plants have to deal with several forms of abiotic stress throughout their life cycle and have developed various defense mechanisms to address them via various physiological pathways. Plants reduce and adapt to varied stressors by altering gene expressions. Experiments have demonstrated that nanoparticles help plants survive abiotic stresses by exerting a concentration-dependent result on plant growth. NPs enhance plants’ ability to withstand certain toxic metals, such as chromium (Cr), cadmium (Cd), iron (Fe), aluminum (Al), and manganese (Mn) (Faizan et al., 2023).
The absorption and movement of Cd, Mn, and Pb in plant tissues have also been shown to be decreased by the management of NPs. Furthermore, through increasing antioxidant activity, osmolyte accumulation, synthesis of free amino acids, and nutritional improvements, NPs help plants resist environmental challenges (Khalid et al., 2022). NPs are used to mitigate the harm caused by abiotic stress. Metal nanoparticles have several uses in plants. Si NPs encourage plant development and increase plant resilience to biotic stress (Yang et al., 2017b). The use of Cu, ZnO, and Se NPs as nano-fertilizers has shown great results. Additionally, nanoparticles have demonstrated the capacity to act as inducers of phytohormone production, controlling plant improvement and metabolism in response to abiotic stress (Zulfiqar and Ashraf, 2021b) Figure 2. illustrates abiotic stress management by modulating different morphological and physiological parameters.
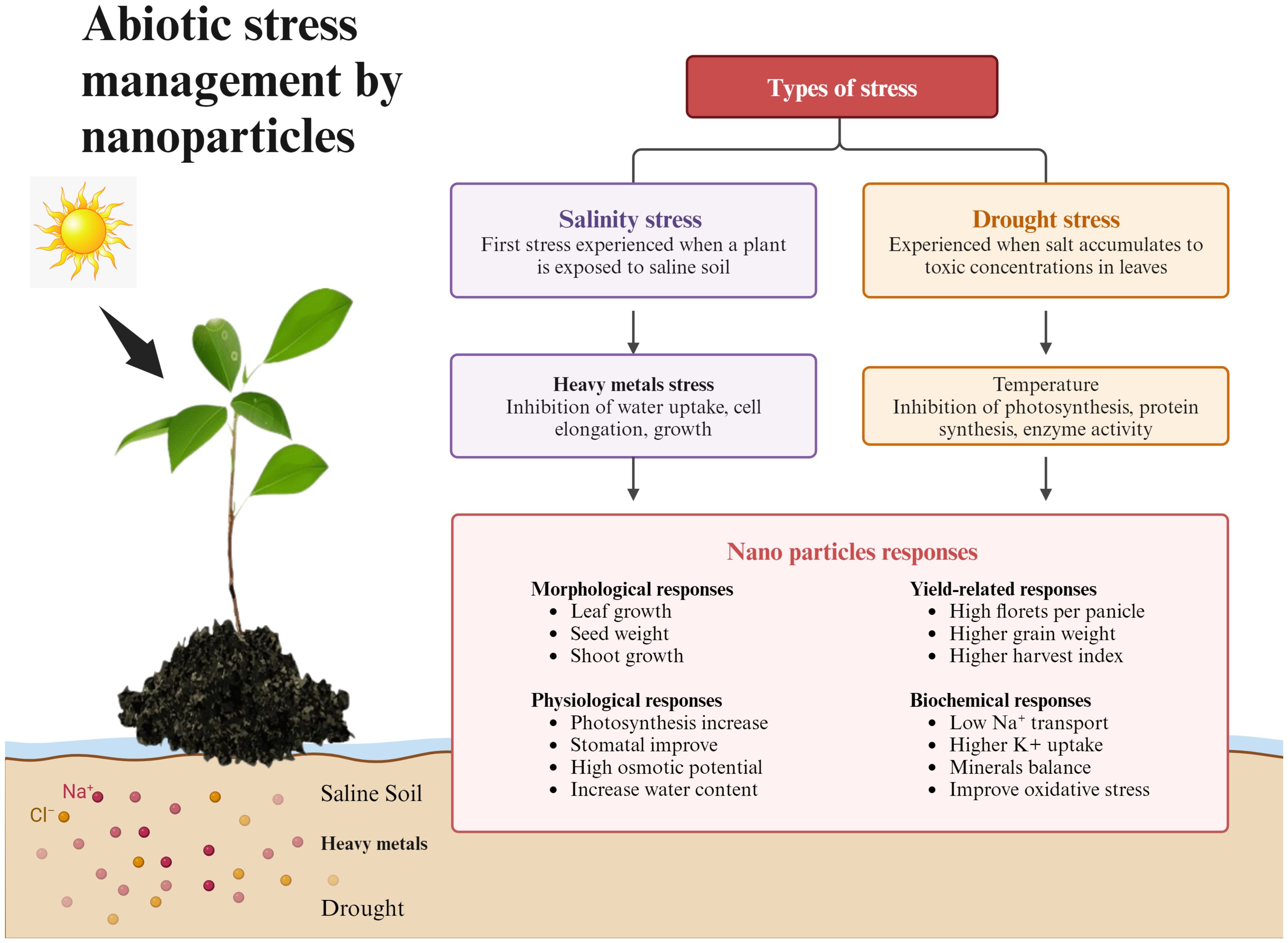
Figure 2. Flowchart demonstrating abiotic stress management by NPs and plants show response on different levels like morphological, yield related, physiological, and biochemical.
Drought stress
Drought is a significant challenge for agriculture, affecting farmers worldwide due to insufficient irrigation and reduced rainfall (Orimoloye et al., 2022). This natural phenomenon is complex and not limited to specific regions or timeframes, making it difficult to monitor and manage (Faiz et al., 2021). Drought stress is primarily caused by a decrease in precipitation, leading to prolonged dry periods. It manifests in four distinct forms: meteorological, hydrological, agricultural, and socio-economic (Haile et al., 2020). Dry weather conditions characterize meteorological drought, while hydrological drought is characterized by low water supply affecting surface and groundwater levels. Agricultural drought results from decreased soil moisture, leading to crop failures and impacting global food production. Socio-economic drought results from limited water supply, which affects economic activities (Swain et al., 2022).
Plant physiological, biochemical, molecular, and genetic responses to drought and salinity are quite similar (Abdelaal et al., 2021; Ghani et al., 2022). Because water intake becomes constrained when soluble solute levels rise due to a drop in water potential, drought conditions start to occur more often in high-salinity locations (Ahluwalia et al., 2021). Plants respond to these stresses by generating phytohormones, adjusting osmotically, reducing transpiration, and altering growth, photosynthesis, carbon absorption, and leaf turgor (Hamid et al., 2021). Figure 3 shows drought stress mitigation through different physiological and biochemical mechanisms.
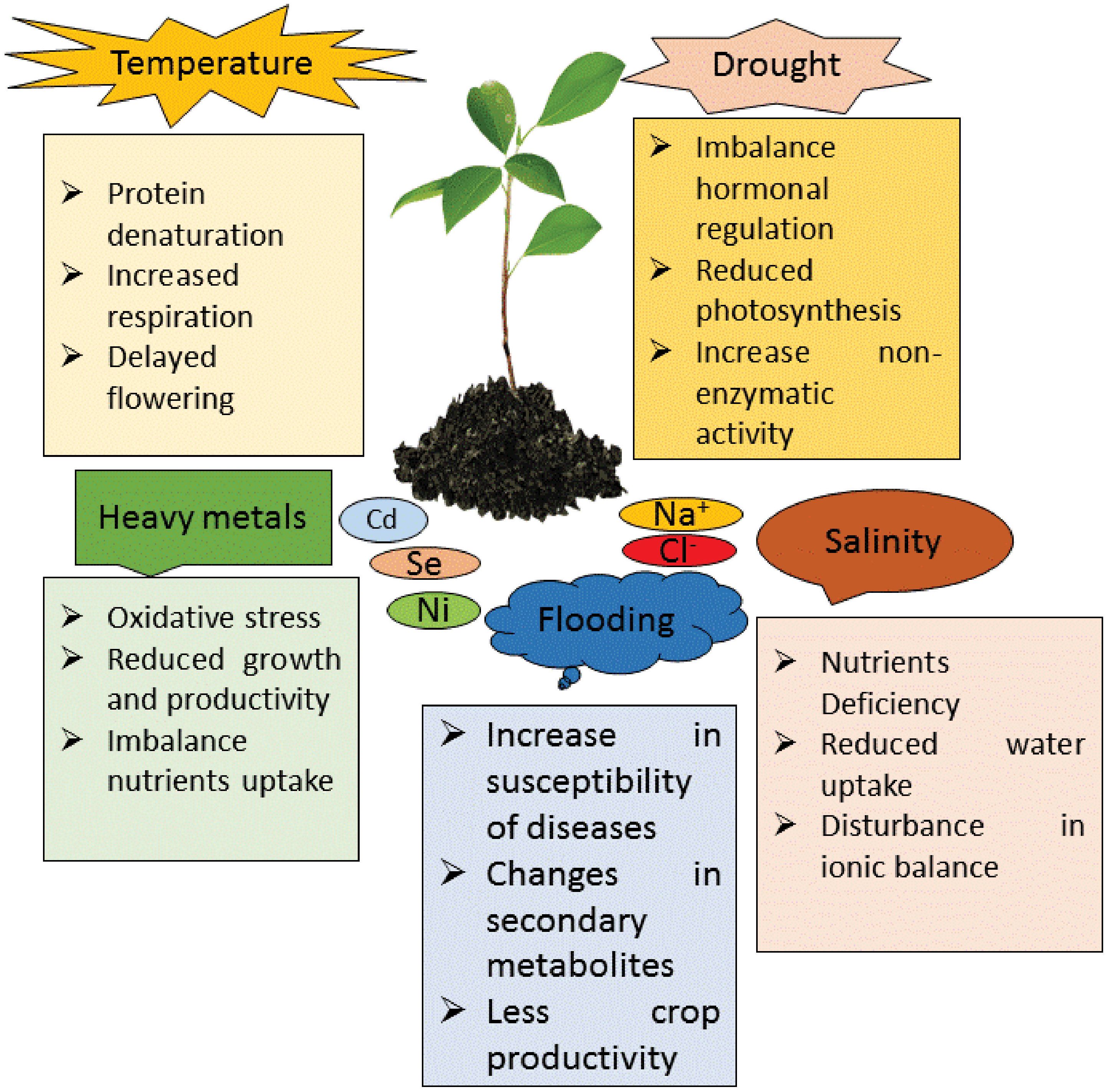
Figure 3. Impacts of drought stress and NPs on plants, showing morphological, physiological, and biochemical changes at different growth levels.
NPs like Si, Ag, Zn, TiO2, and Fe enhance photosynthetic rates, reduce malondialdehyde levels, raise relative water content, and strengthen root and shoot systems, promoting plant growth and development (Chandrashekar et al., 2023) (Table 2). Summarize recent research on the role of NPs in alleviating drought stress. Although the precise methods by which nanoparticles provide drought resistance are not completely known, research indicates that they influence aquaporins—water channel proteins responsible for water transport and seed germination. This mechanism increases the availability of water and nutrients, enhancing germination rates even under dry situations (Zia et al., 2021).
NPs mediated molecular responses to drought stress in plants
NPs influence drought stress responses in plants by regulating specific genes and pathways involved in stress tolerance. The P5CS gene, essential for proline biosynthesis, enhances drought resistance by improving osmotic balance under water deficit conditions (Pérez-Labrada et al., 2020). Similarly, AREB/ABF transcription factors, regulated by abscisic acid, play a pivotal role in activating drought-responsive genes (Yoshida et al., 2015). Downregulation of the ZFHD gene alleviates drought stress by modulating the abscisic acid biosynthesis pathway, while TAS14 downregulation reduces osmotic pressure and increases solute aggregation, including potassium ions and sugars, enhancing drought resilience (Pérez-Labrada et al., 2020). These molecular mechanisms collectively strengthen plant tolerance to drought stress.
Temperature stress
Rising temperatures are among the most detrimental environmental stressors impacting agricultural plants (Fahad et al., 2017). Heat stress induces oxidative stress, producing ROS; these ROS drive the peroxidation of membrane lipids, disturb cellular homeostasis, and hinder several metabolic processes, finally manifesting as cell death (Fahad et al., 2017; Afzal et al., 2020). Additionally, heat stress adversely affects photosystem II, electron transport, carbon fixation, and chlorophyll stability, all of which are essential for the development and production of plants (Shang et al., 2019b).
The application of SeNPs has shown potential in enhancing the morphological characteristics of wheat under heat stress morphological features of wheat under heat stress (Omar et al., 2023). As nano-fertilizers, metallic NPs can enhance plant resilience by improving nutrient uptake and physiological responses. When applied in varying doses, NPs have been found to promote plant growth and hydration under heat-stress conditions. NPs exhibit antioxidative properties at low concentrations, while high concentrations may lead to oxidative stress (Ali et al., 2021a). Under heat stress, AgNPs dramatically improve the morphological features of wheat plants. Metallic NPs used as nano-fertilizers for sustainable agriculture may help plants withstand heat stress (Arora et al., 2022) (Table 3). Provided a detailed summary of NPs and their effects on heat stress.
Molecular mechanisms of nanoparticle-mediated heat stress tolerance in plants
Plants respond to heat stress by synthesizing heat shock proteins (HSPs) and molecular chaperones that stabilize proteins under extreme conditions (Rawat et al., 2021). When NPs were applied to plants in various doses to reduce the effects of heat stress, an enhancement in plant growth and hydration was observed. When NPs are delivered to plants in low concentrations, they exhibit antioxidant properties; however, at high concentrations, oxidative stress occurs. Numerous studies have documented that multiwall carbon nanotubes facilitate the production of HSPs, including HSP90, and augment the expression of heat shock genes (Khan et al., 2017; Zhao et al., 2023). Additionally, it has been demonstrated that cerium oxide nanoparticles (CeO2NPs) increase HSP70 levels in maize, contributing to improved heat stress tolerance. NPs also mitigate heat stress by regulating stomatal opening, thus enhancing transpiration and cooling (Ali et al., 2021a).
In soybean, ZnO NPs enhanced photosynthetic pigments, proline accumulation, and antioxidant enzyme activity by upregulating stress-related genes like HSF-34 and WRKY1 (Mirakhorli et al., 2021). Copper-based NPs modulate genes involved in oxidative stress, brassinosteroid biosynthesis, and root formation, while also influencing secondary metabolite production for signaling and defense (Ali et al., 2019). Similarly, silicon and lanthanum oxide (La₂O₃) NPs regulate aquaporin gene expression, improving water content and stress tolerance in wheat and maize (Yue et al., 2017; Ali et al., 2019).
Salinity stress
Global warming has exacerbated water shortages, leading to the increased reliance on saline water for irrigation in agricultural regions, which in turn increases soil salinity. This practice, however, contributes to rising soil salinity levels, posing a substantial challenge in modern agriculture. High salinity impairs plant growth by disrupting water and nutrient uptake, and prolonged exposure can ultimately result in plant mortality (Al-Khayri et al., 2023). When sodium chloride (NaCl) concentrations exceed 200 mM, salinity affects all stages of a plant’s life cycle, such as seed development, seedling growth, vegetative growth, and flowering (Yuan et al., 2019).
Salinity is a critical abiotic stressor that limits food production and adversely affects the quality of crops, hindering their ongoing development. It remains a major obstacle to achieving sustainable agricultural production, as recognized by the scientific community. Salinity stress affects 20% of all cultivated land globally, and the proportion is increasing on a daily basis (Kumar et al., 2022). The great majority of agricultural plant species are glycophytes, which are especially subject to salt stress and, hence, constitute the most significant environmental abiotic stress that may significantly affect crop yield (Munns and Tester, 2008). The majority of salinity issues are excessive sodium chloride, widely found in soils and water supplies in coastal and arid regions (Hailu and Mehari, 2021). Elevated NaCl concentrations present several challenges for higher plants. These include (i) it increases osmotic pressure in the external solution, necessitating osmotic adjustment by plant cells to prevent dehydration; (ii) Potential interference of excess sodium with the absorption and transportation of vital ions like potassium (K+) and calcium (Ca+2); and (iii) causing direct toxic effects on cell membranes and enzymes due to the presence of sodium (Na+) and chloride (Cl-) (Knop et al., 2023).
Salinity stress diminishes the soil’s osmotic potential, disrupts the nutritional equilibrium, and elevates ionic toxicity (Truscă et al., 2023). NPs play a significant role in mitigating salt stress in plants. They help regulate ion balance, reduce sodium ion toxicity, and enhance potassium ion absorption. Additionally, NPs activate antioxidant defense systems and improve pigment composition, solute levels, and stomatal conductance. For instance, magnetite NPs increase chlorophyll content and antioxidative enzyme activity, contributing to salinity resistance in wheat (Rawat et al., 2021). Similarly, nano-silicon dioxide (SiO2) has been demonstrated to enhance growth and antioxidant activities in Glycine max and improve seed germination and growth in wheat cultivars under salt stress (Dhakate et al., 2022).
ZnO NPs were applied to salt-stressed Brassica napus plants to mitigate the harmful effects of salinity by enhancing the antioxidative system, promoting osmolyte production, and regulating ionic balance (Zulfiqar and Ashraf, 2021b). Cu NPs foliage sprayed on Solanum lycopersicum boosted growth while reducing the impact of salt stress. Cu NPs increased levels of glutathione, polyphenols, and vitamin C, and altered the activity of APX, GPX, and SOD, contributing to improved overall plant growth and development (Fu et al., 2023). Seed priming with ZnO NPs minimized the adverse effects of NaCl treatment on Lupinus termss by increasing pigment levels, modulating osmoregulation, and lowering stress-related metabolite concentrations. According to another report, seed priming T. aestivum L. with AgNPs reduced salt stress (Hossain et al., 2021) (Table 4). represents the role of NPs in mitigating salinity stress.
Molecular modifications in plants mediated by NPs under salinity stress
Molecular processes in plants play a critical role in determining their biological functions, particularly under stress conditions (Figure 4). The impact of NPs on these processes, including gene expression and cellular activities, is evident and essential for their effectiveness. For example, salinity stress alters gene expression, which in turn affects plant growth and cellular functions. In NP-mediated root development, reduced miR164 expression influences auxin hormone signaling, while increased miR169 and decreased miR167 expression promote lateral root formation and accelerate flowering (Tolaymat et al., 2017). Foliar application of Zn NPs in rapeseed (Brassica napus L.) under salinity stress downregulated stress-related genes such as SKRD2, MYC, and MPK4, while upregulating ARP and MPK, which regulate hormonal and physiological responses (Hezaveh et al., 2019). Similarly, silicon nanoparticles (Si NPs) enhanced growth and molecular adaptations in Cannabis sativa L. under salinity stress (Guerriero et al., 2021). In tomato plants, proteomic studies revealed that Si NPs affected genes involved in light-harvesting complexes, cytochrome b6f (Cytb6f), and ATP synthesis (Guerriero et al., 2021).
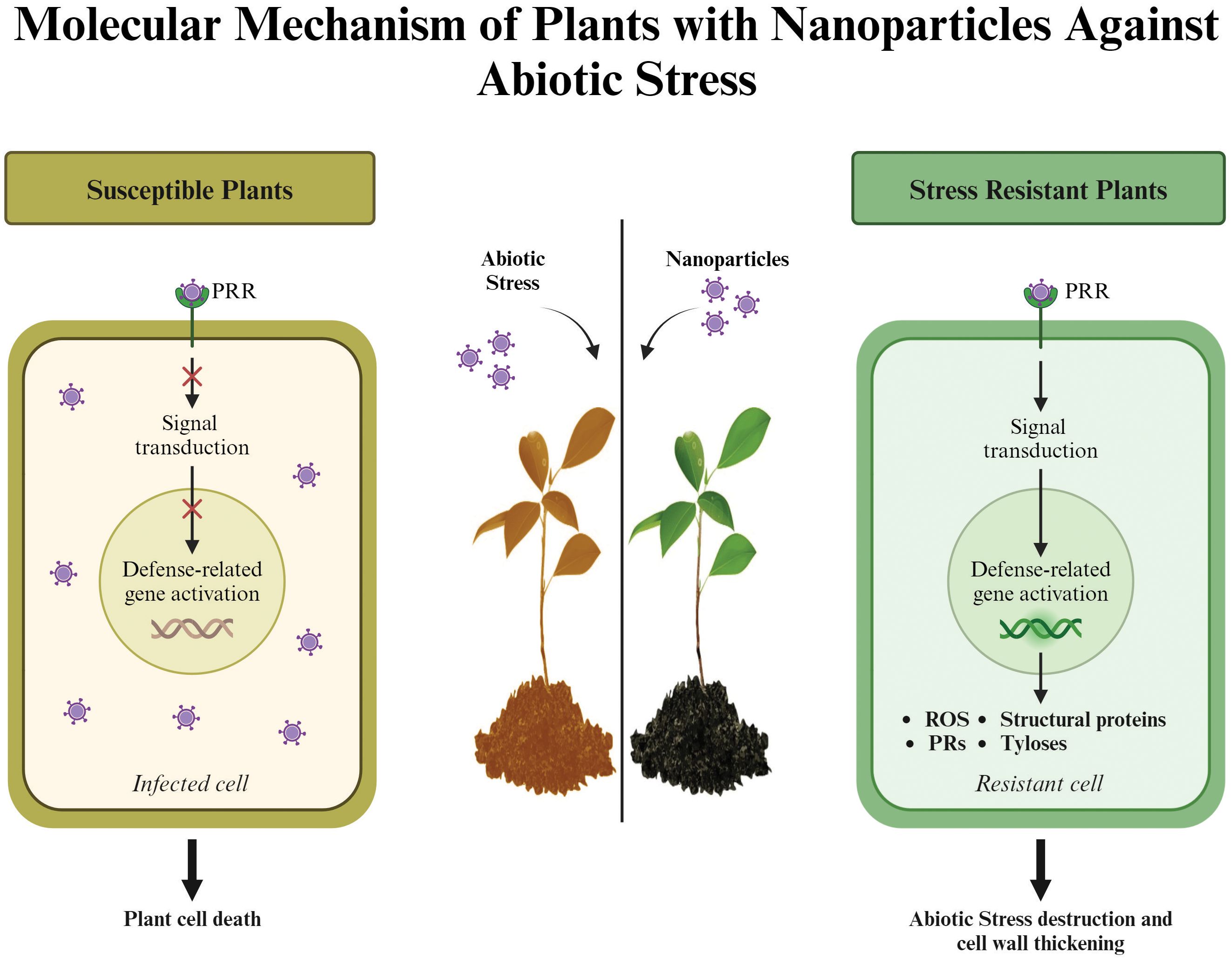
Figure 4. Different types of abiotic stresses like temperature, drought, heavy metals, salinity and flooding effects on plants and their response in various ways.
Role of NPs in modulating HM transport and stress alleviation in plants
Agriculture faces persistent issues with the buildup of HMs in the soil as a result of increasing industrialization, notably in mining and tanning industries (Sarwar et al., 2017). HM in soil is a contemporary issue since it is difficult to dissolve, readily translatable, and extremely hazardous to public health and the environment. HMs changes the natural composition of the soil (Yan et al., 2020; Thakare et al., 2021). The main contaminants that negatively impacting plant’s agro-biological systems are Cr, Cd, Ni, HG, Pb, and Cu (Yaashikaa et al., 2022). Due to their oxidative states, HM are very reactive and induce molecular and cellular alterations, such as changes in plant physiology with enzyme deactivation and protein denaturation, as well as substituting essential metals and damaging membranes (Ghori et al., 2019). These changes reduce photosynthesis and affect plant enzyme activity.
HM-induced oxidative stress causes plant HM resistance due to the high reactivity of HM, which drives at both molecular and cellular levels. HMs can disrupt plant physiology by inactivating enzymes, denaturing proteins, substituting essential metals, and damaging cellular membranes (Ghori et al., 2019). These disruptions lead to reduced photosynthesis and impaired enzyme activity, ultimately affecting plant growth and survival.
NPs present a great potential to improve plant tolerance to HM stress. NPs can be supplied to plants via foliar sprays or soil amendments. For example, selenium and silicon nanoparticles have been shown to alleviate Cd and lead (Pb) stress in Oryza sativa L. by reducing metal uptake and toxicity (Ahmad et al., 2022). By adding to being treated through the soil, NPs can also be applied to plants through the foliage. For example, selenium and silicon nanoparticles applied to leaves have been shown to reduce Cd and Pb stress in Oryza sativa L (Ahmad et al., 2023).
Plants have evolved mechanisms to maintain homeostasis in managing metal absorption, detoxification, and transport. NPs enhance these mechanisms by lowering the bioavailability of metal pollutants, influencing the regulation of genes responsible for metal transport, and strengthening the apoplastic barrier that prevents metals from entering the roots. The binding of NPs to HMs in plant cell walls primarily occurs through adsorption and complexation processes. Plant cell walls contain a variety of functional groups, including hydroxyl, carboxyl, and amino groups, which have a high affinity for binding metal ions (Zhao et al., 2012). When NPs are introduced, they interact with these functional groups and increase the cell wall’s capacity to immobilize HMs through ionic and covalent bonds (Ma et al., 2019). This binding reduces the bioavailability and mobility of metals within plant tissues, effectively sequestering them in a non-bioactive form (Wang et al., 2021). NPs can form stable complexes with metals like Cd, which minimizes their transport across cellular membranes and thus reduces their toxicity. This stabilization is crucial for the plant’s detoxification mechanisms, as it limits the potential of HMs to disrupt cellular functions (Li et al., 2023). By enhancing these metal-binding interactions within the cell wall matrix, NPs support the plant’s natural defense mechanisms against metal stress (Khan et al., 2021).
Although it does not completely block contaminants, the apoplastic barrier performs crucial protective activities in plant roots by managing the passage of ions, oxygen, and water (Fiol et al., 2021). The apoplastic barriers prevent HMs from entering plant roots, and their effectiveness can be increased by NPs (Singh et al., 2021a). By forming complexes with the HMs in the cell walls, NPs bind to them and render them inactive. Studying the many aspects of HM stress relief requires interaction between NPs and HMs. The application of NPs has been supported due to the decreased mobility and resulting bioavailability of metal pollutants in the soil. For instance, adding Si NPs and Fe3O4 NPs makes Cd more stable and reduces its mobility (Rahman et al., 2022). When NPs complexes are adsorbed, they become stationary and inhibit the movement of HMs inside plants, which lowers the biological activity of the plants (Alghanem et al., 2022). NPs improved the biosynthesis of these protective organic acids, as it was demonstrated when Si NPs were utilized to lessen the harm brought on by Cd (Mukarram et al., 2022). Additionally, NPs enhance the properties of soil; for instance, hydroxyapatite NPs can increase soil pH and releases phosphate, which lowers HM toxicity. NPs with a high surface-to-volume ratio, NPs can interact with specific cellular biomolecules and activate several biochemical pathways (Nel et al., 2009).
Similar outcomes were shown when Cd was remedied in coriander using nano-TiO2. These findings included decreased Cd concentration, decreased oxidative injuries brought on by Cd stress, and enhanced agronomic features. In soybeans, the application of nano-TiO2 enhanced photosynthetic rate and growth metrics. The use of Si NPs resulted in an increase in biomass due to a reduction in Cd stress (Memari-Tabrizi et al., 2021). To mitigate the effects of Cd on rice, graphite carbon nitride was generated; as a consequence, there was a large rise in plant biomass and a major decrease in Cd-induced toxicity (Hao et al., 2021).
NPs influence HM transport in plants through species-specific mechanisms that regulate stress responses. For example, silicon nanoparticles (Si NPs) reduce Cd toxicity in rice by downregulating Cd uptake and transport genes, such as low-affinity cation transporter (LCT1) and natural resistance-associated macrophage protein 5 (NRAMP5), while upregulating genes like HM ATPase 3 (HMA3) for Cd sequestration in vacuoles (Cong et al., 2019). Si NPs also enhance silicon uptake via the LSI1 gene, further mitigating Cd accumulation. Similarly, iron oxide (FeO) and hydrogel NPs reduce the expression of key Cd transporters, including OsHMA2, OsHMA3, and OsLCT1, lowering Cd translocation in rice (Ahmed et al., 2021). The NRAMP gene family, responsible for HM transport across species, is also influenced by NPs. Nanoscale zero-valent iron (nZVI) has been shown to alleviate HM buildup by downregulating metal uptake genes (e.g., IRT1, IRT2, YSL2, YSL15), promoting plant growth and reducing metal stress (Guha et al., 2020). (Table 5) summarizes the influence of NPs on alleviating HM stress.
Environmental risks of nanoparticles in agriculture: coping with abiotic stress
The potential for nanotechnology is to boost agricultural production and output, protect crops from environmental challenges, and lessen the release of chemicals into the environment. The distinct effects of nanoparticles (NPs) are significantly impacted by various factors, including their genotype or cultivar, size, dosage, composition, surface area, surface coatings, redox state, and the methods used for their application. However, some plant species, such as soybean, sorghum, broad bean, sweet basil, tomato, wheat, onion, and barley, have shown toxicity to NPs like CeO2, Se, TiO2, and ZnO (Vasseghian et al., 2022).
An increasing amount of research indicates that NPs could potentially impact higher-level consumers, including humans. Recent studies are exploring not only the toxicity of NPs to plants but also their wider implications (Rajpal et al., 2023). E171 (TiO2 NPs-based food additive) has recently been demonstrated to enhance gastrointestinal tumor development and progression in a mouse model by altering systems such as inflammation, immunological responses, cancer signaling, and cell cycle (Li et al., 2021a). Plants suffer a range of adverse effects from NPs, such as, reduced seed germination and root elongation, biomass, growth inhibition, limited mineral uptake, ROS, generation of electron-hole pairs, damage to the cell wall and cell membrane, impairment of cellular structures, and NP aggregation, which leads to increased ROS levels and tissue toxicity (Ghori et al., 2019). However, observations showed that the toxicity of NPs is conditional on things like the plant species and genotype involved, the NPs’ concentration and size, and the length of time the plants are exposed to them (Zhou et al., 2023b).
Researchers in medicine and ecology have gained substantial insights into the protein corona, but its role in plants has only recently been clarified. The absorption, translocation, effects, and ultimate fate of NPs in plants are influenced by various factors, including the protein corona that surrounds them (Huang et al., 2017). The protein corona refers to a dynamic layer of proteins and other biomolecules that adsorb onto the surface of NPs when they enter biological environments, including plant systems. The formation of the protein corona is critical because it alters the physicochemical properties of NPs, such as their size, charge, and surface characteristics, which can impact how they are recognized and transported within plant tissues (Wang et al., 2020). In particular, the protein corona affects the uptake, translocation, and bioavailability of NPs in plants by modulating their interaction with cell walls and membranes (Figure 5). For instance, certain proteins in the corona may facilitate or inhibit NP entry into cells by interacting with membrane receptors or transporters, impacting the NP’s eventual localization within plant tissues (Kumar et al., 2022). Additionally, the protein corona can influence the biocompatibility and potential toxicity of NPs in plants, as specific proteins may reduce or increase the plant’s stress response to foreign particles (Petersen et al., 2016). Thus, understanding the formation and composition of the protein corona in plant systems provides valuable insights into the behavior and fate of NPs in agricultural and environmental contexts. This knowledge helps to better predict how NPs interact with plants at the cellular and molecular levels, which is essential for assessing their risks and benefits in agricultural applications.
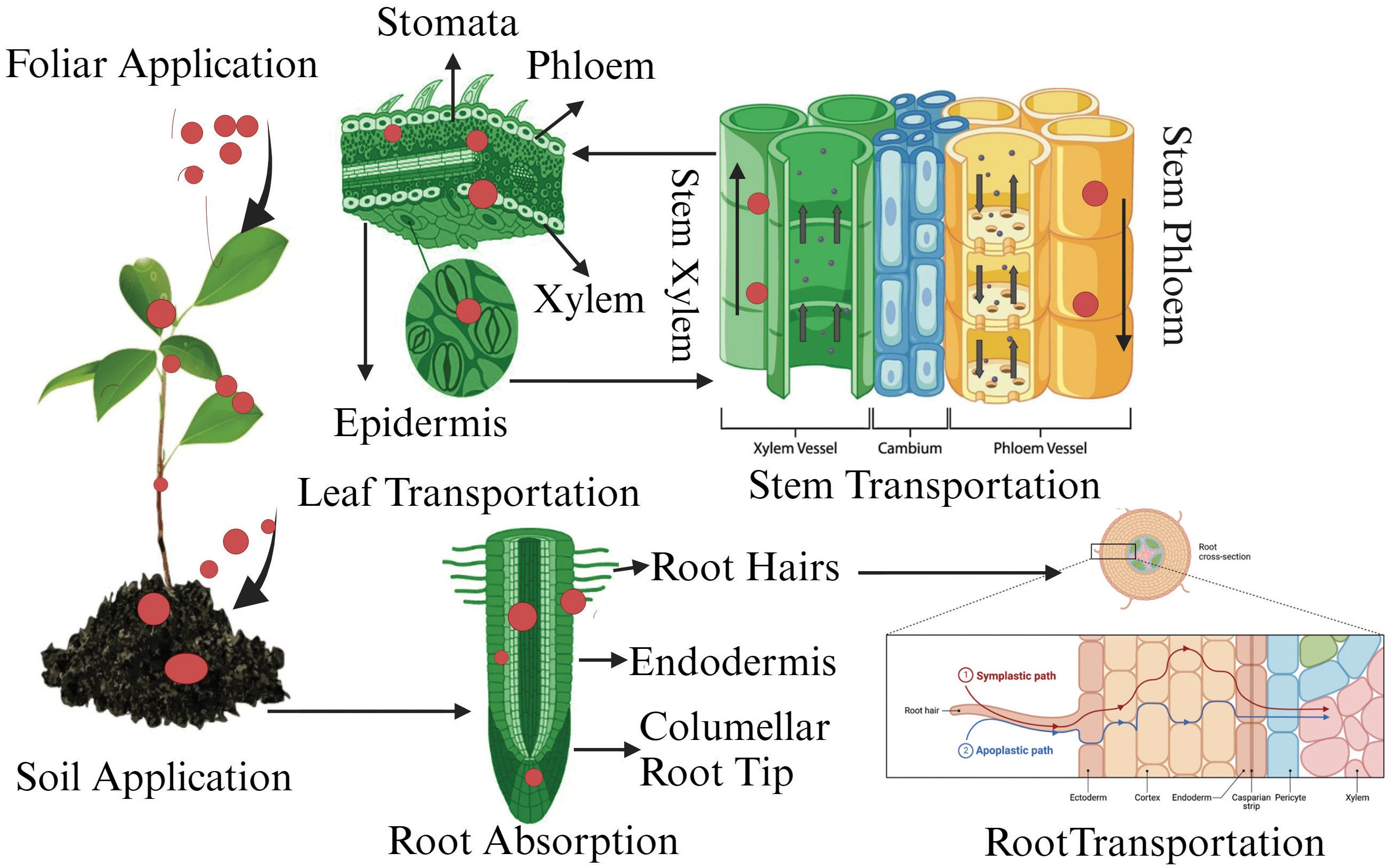
Figure 5. Pathways of nanoparticle uptake, transport, and distribution in plants: from root absorption to foliar application.
Before nanobiotechnology can be commercially applied, thorough research is essential, as cells respond differently to various aspects of the technology, such as its introduction, uptake, translocation, accumulation, concentration, surface activity, variability, and most importantly, the association of NPs with cellular organelles and their impact on cellular metabolism (Ali et al., 2021a). Given these limitations, future research efforts should investigate ways to make agricultural NP usage safer and more productive. To reduce the potential for ecotoxicological risks to humans and plants, greater research into nano-bio interaction and impact evaluations is needed. The safe disposal of NPs is another critical issue that requires the focus of the scientific community. If not burned, NPs should be disposed of in sealed containers in a specially designated place for hazardous waste. Unfortunately, NPs are being employed at several labs/institutions worldwide that do not have access to suitable technology. The usage and disposal of NPs require rigorous safety standards and regulations. The current evaluation stresses the need for precautionary measures while using NPs in the present setting (Donia and Carbone, 2019).
Future challenges
Environmental degradation poses a major risk to global food security, influenced by issues such as soil erosion, nutrient loss, pesticide pollution, and diminishing biodiversity. These obstacles, combined with the continuous reliance on conventional agricultural techniques, highlight the need for innovative approaches. Nanotechnology emerges as a viable solution, capable of bolstering crop resilience, improving nutrient distribution, and alleviating the effects of environmental challenges, thus supporting sustainable farming practices.in addition, abiotic stressors such as drought, salinity, and temperature extremes are becoming more frequent and severe. These stressors significantly reduce crop yield and compromise agricultural productivity. NPs have emerged as promising tools to alleviate plant abiotic stress by enhancing nutrient mobility, providing protection against damage, and improving stress tolerance. NPs are a promising new technology for improving agricultural sustainability. However, there are still some challenges that need to be addressed before NPs are widely adopted (Figure 6). These challenges include:
Regulatory and ethical aspects
Nanotechnology’s integration into agriculture, an emerging field, requires thorough attention to both regulatory and ethical concerns. Developing well-defined guidelines and regulations is essential to guarantee the safe application of NPs in agricultural systems. These measures are crucial for minimizing possible environmental and health risks related to NP applications. Ethical considerations include the impact of large-scale NP use on food safety, biodiversity, and ecosystem health. Collaboration among governments, regulatory bodies, and researchers is vital to determine safe NP concentration thresholds for various agricultural applications.
Integration with other agricultural technologies
Nanotechnology can be integrated with other emerging agricultural technologies, such as Precision Agriculture, to enhance efficiency. Technologies like drones, sensors, and satellite imagery facilitate targeted nutrient and pesticide delivery. However, there is ongoing debate about the environmental impact and efficiency of these technologies, partly due to NP concentrations. Precision Agriculture could benefit from NP-enhanced nutrients, allowing for more efficient and less polluting delivery systems. Additionally, smart fertilizers using NPs can provide variable delivery routes and amounts based on plant needs, promoting sustainability.
Integrating nanotechnology with other advanced agricultural technologies, particularly within the framework of Precision Agriculture, holds significant potential for improving efficiency and sustainability in farming. Precision Agriculture technologies, such as drones, soil sensors, and satellite imagery, enable real-time monitoring of crop health, soil quality, and environmental conditions. These tools facilitate targeted applications of fertilizers and pesticides, reducing waste and limiting environmental impact (Getahun et al., 2024). NPs could further enhance these technologies by improving the formulation of agrochemicals, enabling controlled-release fertilizers, and offering nano-based pesticides that are more precise and require lower doses than traditional formulations (Zain et al., 2023).
One promising application is the use of NP-enhanced nutrients, which could allow for more efficient delivery systems by controlling the release of nutrients directly into the root zones, minimizing runoff and reducing groundwater contamination (Shanmugavel et al., 2023). This approach aligns with sustainable practices by delivering nutrients in a manner that minimizes pollution. Additionally, integrating NPs with smart fertilizers that respond to environmental cues or plant needs could provide tailored nutrient delivery. For instance, NPs can be engineered to release nutrients in response to soil moisture levels or pH changes, thus ensuring that plants receive the right nutrients at the right time (Wang et al., 2020).
However, concerns regarding the long-term environmental and ecological impact of NPs persist, especially with respect to NP accumulation in soil and water systems. Research has indicated that while nanomaterials offer efficiency, their interactions with various environmental factors are complex and not fully understood, raising questions about their fate and effects in ecosystems (Lead et al., 2018). Ongoing studies aim to address these uncertainties by examining the biodegradability, persistence, and toxicity of NPs used in agricultural settings, aiming to establish guidelines for safe concentrations and applications.
Through strategic integration, nanotechnology could enhance the effectiveness of Precision Agriculture by ensuring that nutrients and pesticides are applied only where and when needed, promoting sustainable agricultural practices. This synergy between nanotechnology and digital farming tools could ultimately lead to a more environmentally conscious approach to food production, potentially mitigating some of the environmental pressures posed by conventional agricultural methods (Zhang et al., 2021).
Advance methodologies to track and observe the NPs delivery in plants
Advanced methodologies for tracking and observing NPs delivery within plants have significantly progressed, enabling detailed insights into NPs uptake, distribution, and transformation. Techniques such as confocal microscopy with intrinsically fluorescent or dye-labeled NPs (Demirer et al., 2020; Santana et al., 2020), provide real-time tracking of NPs, allowing visualization of their transport across cellular barriers and into specific plant structures, including roots and leaves (Santana et al., 2020; Jeon et al., 2024). Another powerful approach involves laser ablation coupled with inductively coupled plasma mass spectrometry (ICP-MS), which can quantitatively analyze elemental composition and map the distribution of metal-based NPs within different plant tissues, providing spatial resolution of NP presence in roots, stems, and leaves (Avellan et al., 2019).
Synchrotron-based X-ray fluorescence (XRF) imaging (Stegemeier et al., 2017) and X-ray absorption spectroscopy (López-Moreno et al., 2010) offer high-resolution elemental mapping, enabling researchers to observe the exact location and chemical state of NPs within plant cells and organelles. These techniques are especially useful for assessing NP translocation and transformations at cellular and subcellular levels (Lombi et al., 2016). For three-dimensional analysis, methods like X-ray tomography, magnetic resonance imaging (MRI), and advanced confocal microscopy allow for 3D reconstructions, which facilitate a comprehensive understanding of NP penetration pathways and localization within plant tissues (Staedler et al., 2013; Santana et al., 2020).
Additional techniques such as Fourier-transform infrared (FTIR) spectroscopy, Raman spectroscopy, and microparticle-induced X-ray emission (µ-Pixe) are essential for examining chemical changes in NP surfaces (Larue et al., 2014). These tools help to monitor how NPs interact with plant biomolecules, thus providing insight into the stability and transformations of NPs once inside the plant environment (Larue et al., 2014). Furthermore, single-particle ICP-MS is used to characterize individual NPs within plant tissues, offering data on size, distribution, and potential aggregation, which are critical for understanding NP behavior after uptake (Keller et al., 2018). Collectively, these advanced methodologies allow researchers to accurately track, quantify, and analyze NPs within plants, which is essential for optimizing nano-enabled delivery systems in agricultural applications.
These innovative tracking tools thus support a detailed understanding of NP delivery, behavior, and impact within plant systems, addressing essential concerns for the development of sustainable and effective NP applications in agriculture.
Public considerations
Public perception of NPs in agriculture is an important consideration. Concerns often stem from a deficiency of information and fears around the ingestion of NPs in food production. Educational campaigns and collaboration among researchers, policymakers, and the public are essential to foster informed and acceptable NP use in agriculture.
Long-term studies and environmental effects
Long term studies are crucial for understanding how NPs persist, move, and affect ecosystems within agricultural systems. While short-term studies provide insight into initial effects, only extended-duration research can reveal how NPs behave over time in complex agricultural systems and ecosystems. These studies are especially valuable for organic and sustainable farming systems, where minimal synthetic inputs are emphasized. In such contexts, the introduction of nanoparticles could have unexpected consequences for soil health, water quality, and crop quality.
Long-term field studies examining the environmental impacts of nanoparticles (NPs) indicate that NPs undergo complex transformations influenced by soil chemistry, redox potential, and interactions with plant and microbial metabolites, leading to significant environmental implications. For instance, metal-based NPs such as copper oxide (CuO) can be reduced in acidic, low-oxygen environments, forming stable compounds that persist in soil, thereby altering soil chemistry and affecting nutrient bioavailability over time (Shang et al., 2019a; Arshad et al., 2021). Additionally, transformations within plant systems vary with environmental factors like ionic strength and pH, impacting NP dissolution rates and, consequently, nutrient cycling in the surrounding soil and plant ecosystem (Lv et al., 2019; Zhang et al., 2020).
Studies reveal that these transformations affect the entire plant life cycle, with effects extending across generations. For example, nanoparticles such as silver (Ag) and cerium oxide (CeO₂) have been shown to enhance stress responses in plants, which can be inherited by subsequent generations (Zhao et al., 2022; Zhou et al., 2023a). However, the potential for bioaccumulation and biomagnification of NPs in food chains remains a significant concern, as these particles can migrate from soil to crops and subsequently into higher trophic levels, posing ecosystem health risks (Werlin et al., 2011; Wu et al., 2023).
Long-term environmental exposure studies in realistic soil systems underscore the complex interactions of NPs with soil microbiomes, groundwater, and higher organisms, emphasizing the need for sustainable NP application practices. For example, NPs can aggregate or bind with soil components, forming eco-coronas that mitigate toxicity, but complicate environmental fate and impact based on NP composition and environmental context (Kurepa et al., 2020; Borgatta et al., 2021). Understanding these transformations in agriculareral systems is thus crucial for safe and sustainable nano-enabled agricultural technologies.
Outstanding questions
1. Which plant developmental stages and species should be prioritized for nanoparticle research to better understand the mechanisms driving plant responses to abiotic stress? How do these responses change across different growth phases?
2. How can we improve the incorporation of nanoparticle methods to get a deeper awareness of the growth processes that control plant responses to severe abiotic stress conditions, hence improving the development of stress-resistant plants?
3. How can we develop advanced climate simulation systems that accurately reflect the impact of nanoparticles on crops in field conditions?
4. What are the environmental and safety risks of nanotechnology, and how may they reduced in order to accelerate agricultural development?
5. What are the long-term effects on stressed plants in changing climates, especially in practical farming environments, and how can these outcomes be predicted and managed?
6. How can we promote nanotechnology cooperation among plant scientists, geneticists, data experts, and agronomists to tackle the challenges involved in cultivating stress-resistant plants using nanotechnology?
7. What policies and strategies can be introduced at both national and international levels to encourage the adoption of nanotechnology in agriculture and contribute to achieving global food security goals, such as the ‘zero hunger’ initiative by the Food and Agriculture Organization (FAO)?
Conclusion
Scientists must design and implement sustainable solutions to minimize agricultural production losses due to abiotic stress. Nanotechnology is a novel and effective way to raise agricultural productivity and quality and manage global food demand. Several NPs are being researched for their potential role in minimizing abiotic stress-induced loss and enhancing plant development and agricultural output. NPs mitigate abiotic stress by triggering plant defense systems such as ROS production and phytotoxicity. NPs easily permeate plant tissues due to their tiny size, impacting plant morphological, physiological, and biochemical processes, enhancing plant development, and boosting crop output in plants exposed to various abiotic challenges.
Further research is necessary to maximize the benefits of NPs in agriculture and mitigate their potential negative impacts; key areas for investigation include: It is crucial to understand how NPs interact with plants at molecular and cellular stages. This includes studying their effects on metabolic processes and optimizing NPs size and concentration for effective field application. Research should focus on the potential toxic effects of NPs across different plant species. Understanding these effects is essential for developing safe application protocols. Exploring how NPs influence gene expression can provide insights into their role in enhancing plant stress tolerance and resilience.
While NPs offer promising solutions for enhancing agricultural sustainability and resilience, their implementation must be approached with caution. By addressing regulatory, ethical, and environmental considerations, nanotechnology can be safely and effectively integrated into agricultural practices, contributing to global food security and environmental health.
Author contributions
YC: Writing – original draft, Writing – review & editing. KT: Validation, Writing – review & editing, Funding acquisition. NB: Writing – original draft, Writing – review & editing. AG: Writing – review & editing. NA: Writing – review & editing. MA: Writing – review & editing. RA: Writing – review & editing. MG: Conceptualization, Writing – review & editing. MA: Conceptualization, Writing – review & editing.
Funding
The author(s) declare that financial support was received for the research, authorship, and/or publication of this article. This work was supported financially by the National Natural Science Foundation of China (32160456), Guizhou Provincial Key Technology R&D Program ((2021) YiBan272), Guizhou Provincial Basic Research Program (Natural Science) ((2020) 1Z018), and the National Natural Science Foundation of China (32360486). The authors are grateful to the Deanship of Scientific Research (DSR), King Faisal University, Saudi Arabia, for financing the publishing of this study (Project number: KFU241740).
Conflict of interest
The authors declare that the research was conducted in the absence of any commercial or financial relationships that could be construed as a potential conflict of interest.
Generative AI statement
The author(s) declare that no Generative AI was used in the creation of this manuscript.
Publisher’s note
All claims expressed in this article are solely those of the authors and do not necessarily represent those of their affiliated organizations, or those of the publisher, the editors and the reviewers. Any product that may be evaluated in this article, or claim that may be made by its manufacturer, is not guaranteed or endorsed by the publisher.
References
Abdelaal, K., AlKahtani, M., Attia, K., Hafez, Y., Király, L., Künstler, A. (2021). The role of plant growth-promoting bacteria in alleviating the adverse effects of drought on plants. Biology 10, 520. doi: 10.3390/biology10060520
Afshari, M., Pazoki, A., Sadeghipour, O. (2021). Foliar-applied silicon and its nanoparticles stimulate physio-chemical changes to improve growth, yield and active constituents of coriander (Coriandrum sativum l.) essential oil under different irrigation regimes. Silicon 1-12. doi: 10.1007/s12633-021-01101-8
Afzal, I., Akram, M., Rehman, H., Rashid, S., Basra, S. (2020). Moringa leaf and sorghum water extracts and salicylic acid to alleviate impacts of heat stress in wheat. South Afr. J. Bot. 129, 169–174. doi: 10.1016/j.sajb.2019.04.009
Aghdam, M. T. B., Mohammadi, H., Ghorbanpour, M. (2016). Effects of nanoparticulate anatase titanium dioxide on physiological and biochemical performance of linum usitatissimum (Linaceae) under well-watered and drought stress conditions. Braz. J. Bot. 39, 139–146. doi: 10.1007/s40415-015-0227-x
Ahluwalia, O., Singh, P. C., Bhatia, R. (2021). A review on drought stress in plants: Implications, mitigation and the role of plant growth promoting rhizobacteria. Resources Environ. Sustainability 5, 100032. doi: 10.1016/j.resenv.2021.100032
Ahmad, A., Hashmi, S. S., Palma, J. M., Corpas, F. J. (2022). Influence of metallic, metallic oxide, and organic nanoparticles on plant physiology. Chemosphere 290, 133329. doi: 10.1016/j.chemosphere.2021.133329
Ahmad, S., Pathak, A., Minj, R., Chaudhary, S., Kumar, A., Chalotra, T., et al. (2023). A comprehensive review of nanoparticles induced stress and toxicity in plants. Universal J. Green Chem. 1, 18–43. doi: 10.37256/ujgc.1120231946
Ahmed, T., Noman, M., Manzoor, N., Shahid, M., Abdullah, M., Ali, L., et al. (2021). Nanoparticle-based amelioration of drought stress and cadmium toxicity in rice via triggering the stress responsive genetic mechanisms and nutrient acquisition. Ecotoxicology Environ. Saf. 209, 111829. doi: 10.1016/j.ecoenv.2020.111829
Al Jabri, H., Saleem, M. H., Rizwan, M., Hussain, I., Usman, K., Alsafran, M. (2022). Zinc oxide nanoparticles and their biosynthesis: overview. Life 12 (4), 594. doi: 10.3390/life12040594
Alghanem, S. M., Alhaithloul, H. A. S., Abdelhamid, M. T., Soliman, M. H. (2022). “Role of various nanoparticles in countering heavy metal, salt, and drought stress in plants,” in Sustainable agriculture reviews 53: nanoparticles: A new tool to enhance stress tolerance (Switzerland AG: Springer), 151–170.
Ali, S., Mehmood, A., Khan, N. (2021a). Uptake, translocation, and consequences of nanomaterials on plant growth and stress adaptation. J. Nanomaterials 2021, 1–17. doi: 10.1155/2021/6677616
Ali, S., Mehmood, A., Khan, N. (2021b). Uptake, translocation, and consequences of nanomaterials on plant growth and stress adaptation. J. Nanomaterials 2021, 6677616. doi: 10.1155/2021/6677616
Ali, S., Rizwan, M., Hussain, A., ur Rehman, M. Z., Ali, B., Yousaf, B., et al. (2019). Silicon nanoparticles enhanced the growth and reduced the cadmium accumulation in grains of wheat (Triticum aestivum L.). Plant Physiol. Biochem. 140, 1–8. doi: 10.1016/j.plaphy.2019.04.041
Alluqmani, S. M., Alabdallah, N. M. (2023). Exogenous application of carbon nanoparticles alleviates drought stress by regulating water status, chlorophyll fluorescence, osmoprotectants, and antioxidant enzyme activity in. Environ. Sci. pollut. Res. 30 (20), 57423–57433. doi: 10.1007/s11356-023-26606-0
Al-Khayri, J. M., Rashmi, R., Surya Ulhas, R., Sudheer, W. N., Banadka, A., Nagella, P., et al. (2023). The role of nanoparticles in response of plants to abiotic stress at physiological, biochemical, and molecular levels. Plants 12, 292. doi: 10.3390/plants12020292
Alsaeedi, A., Show, Y. (2019). Synthesis of nano-carbon by in-liquid plasma method and its application to a support material of pt catalyst for fuel cell. Nanomaterials nanotechnology 9, 1847980419853159. doi: 10.1177/184798041985315
Anwar, T., Qureshi, H., Fatimah, H., Siddiqi, E. H., Anwaar, S., Moussa, I. M., et al. (2023). Improvement of physio-biochemical attributes and mitigation of salinity stress by combined application of melatonin and silicon nanoparticles in Brassica oleracea var. botrytis. Scientia Hortic. 322, 112456. doi: 10.1016/j.scienta.2023.112456
Anwar, T., Qureshi, H., Fatimah, H., Siddiqi, E. H., Anwaar, S., Moussa, I. M., et al. (2023). Elucidating effect of ZnO-nanoparticles and melatonin on physiological adjustments and growth of solanum melongena under salinity stress. Scientia Hortic. 322, 112455. doi: 10.1016/j.scienta.2023.112455
Aparato, V. P. M., Suh, D. Y. (2022). The functions and applications of GDSL esterase/lipase proteins in agriculture: A review. JSFA Rep. 2 (7), 304–312. doi: 10.1002/jsf2.70
Aqeel, U., Aftab, T., Khan, M. M. A., Naeem, M., Khan, M. N. (2022). A comprehensive review of impacts of diverse nanoparticles on growth, development and physiological adjustments in plants under changing environment. Chemosphere 291, 132672. doi: 10.1016/j.chemosphere.2021.132672
Arikan, B., Alp, F. N., Ozfidan-Konakci, C., Balci, M., Elbasan, F., Yildiztugay, E., et al. (2022). Fe2O3-modified graphene oxide mitigates nanoplastic toxicity via regulating gas exchange, photosynthesis, and antioxidant system in triticum aestivum. Chemosphere 307, 136048. doi: 10.1016/j.chemosphere.2022.136048
Arora, S., Murmu, G., Mukherjee, K., Saha, S., Maity, D. (2022). A comprehensive overview of nanotechnology in sustainable agriculture. J. Biotechnol. 355, 21–41. doi: 10.1016/j.jbiotec.2022.06.007
Arshad, M., Nisar, S., Gul, I., Nawaz, U., Irum, S., Ahmad, S., et al. (2021). Multi-element uptake and growth responses of Rice (Oryza sativa L.) to TiO2 nanoparticles applied in different textured soils. Ecotoxicology Environ. Saf. 215, 112149. doi: 10.1016/j.ecoenv.2021.112149
Avellan, A., Yun, J., Zhang, Y., Spielman-Sun, E., Unrine, J. M., Thieme, J., et al. (2019). Nanoparticle size and coating chemistry control foliar uptake pathways, translocation, and leaf-to-rhizosphere transport in wheat. ACS nano 13, 5291–5305. doi: 10.1021/acsnano.8b09781
Avestan, S., Ghasemnezhad, M., Esfahani, M., Byrt, C. S. (2019). Application of nano-silicon dioxide improves salt stress tolerance in strawberry plants. Agronomy 9 (5), 246. doi: 10.3390/agronomy9050246
Ayub, M. A., Naeem, A., ur Rehman, M. Z., Farooqi, Z. U. R., Umar, W., Fatima, H., et al. (2022). Role of nanotechnology in enhancing crop production and produce quality. Sustain. Nanotechnology Environ. Remediation, 703–764. doi: 10.1016/B978-0-12-824547-7.00014-X
Azimi, R., Borzelabad, M. J., Feizi, H., Azimi, A. (2014). Interaction of SiO nanoparticles with seed prechilling on germination and early seedling growth of tall wheatgrass (Agropyron elongatum l.). Polish J. Chem. Technol. 16 (3), 25–29. doi: 10.2478/pjct-2014-0045
Azmat, A., Tanveer, Y., Yasmin, H., Hassan, M. N., Shahzad, A., Reddy, M., et al. (2022). Coactive role of zinc oxide nanoparticles and plant growth promoting rhizobacteria for mitigation of synchronized effects of heat and drought stress in wheat plants. Chemosphere 297, 133982. doi: 10.1016/j.chemosphere.2022.133982
Bakshi, S., He, Z. L., Harris, W. G. (2015). Natural nanoparticles: implications for environment and human health. Crit. Rev. Environ. Sci. Technol. 45, 861–904. doi: 10.1080/10643389.2014.921975
Baz, H., Creech, M., Chen, J., Gong, H., Bradford, K., Huo, H. (2020). Water-soluble carbon nanoparticles improve seed germination and post-germination growth of lettuce under salinity stress. Agronomy 10 (8), 1192. doi: 10.3390/agronomy10081192
Behboudi, F., Tahmasebi-Sarvestani, Z., Kassaee, M. Z., Modarres-Sanavy, S. A. M., Sorooshzadeh, A., Mokhtassi-Bidgoli, A. (2019). Evaluation of chitosan nanoparticles effects with two application methods on wheat under drought stress. J. Plant Nutr. 42 (13), 1439–1451. doi: 10.1080/01904167.2019.1617308
Bhattacharjee, R., Kumar, L., Mukerjee, N., Anand, U., Dhasmana, A., Preetam, S., et al. (2022). The emergence of metal oxide nanoparticles (NPs) as a phytomedicine: A two-facet role in plant growth, nano-toxicity and anti-phyto-microbial activity. Biomedicine Pharmacotherapy 155, 113658. doi: 10.1016/j.biopha.2022.113658
Bidi, H., Fallah, H., Niknejad, Y., Tari, D. B. (2021). Iron oxide nanoparticles alleviate arsenic phytotoxicity in rice by improving iron uptake, oxidative stress tolerance and diminishing arsenic accumulation. Plant Physiol. Biochem. 163, 348–357. doi: 10.1016/j.plaphy.2021.04.020
Borgatta, J. R., Lochbaum, C. A., Elmer, W. H., White, J. C., Pedersen, J. A., Hamers, R. J. (2021). Biomolecular corona formation on CuO nanoparticles in plant xylem fluid. Environ. Science: Nano 8, 1067–1080. doi: 10.1039/D1EN00140J
Brunelle, T., Chakir, R., Carpentier, A., Dorin, B., Goll, D., Guilpart, N., et al. (2024). Reducing chemical inputs in agriculture requires a system change. Commun. Earth Environ. 5, 369. doi: 10.1038/s43247-024-01533-1
Cao, Z., Rossi, L., Stowers, C., Zhang, W., Lombardini, L., Ma, X. (2018). The impact of cerium oxide nanoparticles on the physiology of soybean (Glycine max (L.) merr.) under different soil moisture conditions. Environ. Sci. pollut. Res. 25, 930–939. doi: 10.1007/s11356-017-0501-5
Chandrashekar, H. K., Singh, G., Kaniyassery, A., Thorat, S. A., Nayak, R., Murali, T. S., et al. (2023). Nanoparticle-mediated amelioration of drought stress in plants: a systematic review. 3 Biotech. 13, 336. doi: 10.1007/s13205-023-03751-4
Chen, J., Guo, Y., Zhang, X., Liu, J., Gong, P., Su, Z., et al. (2023). Emerging nanoparticles in food: sources, application, and safety. J. Agric. Food Chem. 71, 3564–3582. doi: 10.1021/acs.jafc.2c06740
Cong, W., Miao, Y., Xu, L., Zhang, Y., Yuan, C., Wang, J., et al. (2019). Transgenerational memory of gene expression changes induced by heavy metal stress in rice (Oryza sativa L.). BMC Plant Biol. 19, 1–14. doi: 10.1186/s12870-019-1887-7
Cox, A., Venkatachalam, P., Sahi, S., Sharma, N. (2016). Silver and titanium dioxide nanoparticle toxicity in plants: a review of current research. Plant Physiol. Biochem. 107, 147–163. doi: 10.1016/j.plaphy.2016.05.022
Demirer, G. S., Zhang, H., Goh, N. S., Pinals, R. L., Chang, R., Landry, M. P. (2020). Carbon nanocarriers deliver siRNA to intact plant cells for efficient gene knockdown. Sci. Adv. 6, eaaz0495. doi: 10.1126/sciadv.aaz0495
Dhakate, P., Kandhol, N., Raturi, G., Ray, P., Bhardwaj, A., Srivastava, A., et al. (2022). Silicon nanoforms in crop improvement and stress management. Chemosphere 305, 135165. doi: 10.1016/j.chemosphere.2022.135165
Djanaguiraman, M., Nair, R., Giraldo, J. P., Prasad, P. V. V. (2018). Cerium oxide nanoparticles decrease drought-induced oxidative damage in sorghum leading to higher photosynthesis and grain yield. ACS omega 3 (10), 14406–14416. doi: 10.1021/acsomega.8b01894
Donia, D., Carbone, M. (2019). Fate of the nanoparticles in environmental cycles. Int. J. Environ. Sci. Technol. 16, 583–600. doi: 10.1007/s13762-018-1960-z
Easwaran, C., Moorthy, G., Christopher, S. R., Mohan, P., Marimuthu, R., Koothan, V., et al. (2024). Nano hybrid fertilizers: A review on the state of the art in sustainable agriculture. Sci. Total Environ. 172533. doi: 10.1016/j.scitotenv.2024.172533
El-Gazzar, N., Almaary, K., Ismail, A., Polizzi, G. (2020). Influence of funneliformis mosseae enhanced with titanium dioxide nanoparticles (TiO2NPs) on phaseolus vulgaris l. under salinity stress. PloS One 15 (8), e0235355. doi: 10.1371/journal.pone.0235355
El-Saadony, M. T., Saad, A. M., Soliman, S. M., Salem, H. M., Desoky, E.-S. M., Babalghith, A. O., et al. (2022). Role of nanoparticles in enhancing crop tolerance to abiotic stress: A comprehensive review. Front. Plant Sci. 13, 946717. doi: 10.3389/fpls.2022.946717
El-Zohri, M., Al-Wadaani, N. A., Bafeel, S. O. (2021). Foliar sprayed green zinc oxide nanoparticles mitigate drought-induced oxidative stress in tomato. Plants 10 (11), 2400. doi: 10.3390/plants10112400
Elsheery, N. I., Helaly, M. N., El-Hoseiny, H. M., Alam-Eldein, S. M. (2020). Zinc oxide and silicone nanoparticles to improve the resistance mechanism and annual productivity of salt-stressed mango trees. Agronomy 10 (4), 558. doi: 10.3390/agronomy10040558
Etesami, H., Fatemi, H., Rizwan, M. (2021). Interactions of nanoparticles and salinity stress at physiological, biochemical and molecular levels in plants: A review. Ecotoxicology Environ. Saf. 225, 112769. doi: 10.1016/j.ecoenv.2021.112769
Fahad, S., Bajwa, A. A., Nazir, U., Anjum, S. A., Farooq, A., Zohaib, A., et al. (2017). Crop production under drought and heat stress: plant responses and management options. Front. Plant Sci. 8. doi: 10.3389/fpls.2017.01147
Faiz, M. A., Zhang, Y., Ma, N., Baig, F., Naz, F., Niaz, Y. (2021). Drought indices: aggregation is necessary or is it only the researcher’s choice? Water Supply. 21, 3987–4002. doi: 10.2166/ws.2021.163
Faizan, M., Alam, P., Rajput, V., Faraz, A., Afzal, S., Ahmed, S., et al. (2023). Nanoparticle mediated plant tolerance to heavy metal stress: what we know? Sustainability 15, p.1446. doi: 10.3390/su15021446
Faizan, M., Bhat, J. A., Chen, C., Alyemeni, M. N., Wijaya, L., Ahmad, P., et al. (2021). Zinc oxide nanoparticles (ZnO-NPs) induce salt tolerance by improving the antioxidant system and photosynthetic machinery in tomato. Plant Physiol. Biochem. 161, 122–130. doi: 10.1016/j.plaphy.2021.02.002
Fan, X., Xu, J., Lavoie, M., Peijnenburg, W., Zhu, Y., Lu, T., et al. (2018). Multiwall carbon nanotubes modulate paraquat toxicity in Arabidopsis thaliana. Environ. pollut. 233, 633–641. doi: 10.1016/j.envpol.2017.10.116
Faraji, J., Sepehri, A. (2019). Ameliorative effects of TiO 2 nanoparticles and sodium nitroprusside on seed germination and seedling growth of wheat under PEG-stimulated drought stress. J. Seed Sci. 41, 309–317. doi: 10.1590/2317-1545v41n3213139
Farouk, S., Elhindi, K. M., Alotaibi, M. A. (2020). Silicon supplementation mitigates salinity stress on ocimum basilicum l. via improving water balance, ion homeostasis, and antioxidant defense system. Ecotoxicology Environ. Saf. 206, 111396. doi: 10.1016/j.ecoenv.2020.111396
Fasake, V., Patil, N., Javed, Z., Mishra, M., Tripathi, G. D., Srivastava, A., et al. (2021). Effects of nanobionics in crop production: A review. Nanoscience Nanotechnology - Asia 11, 249–261. doi: 10.2174/2210681210999200602173303
Fatemi, H., Pour, B. E., Rizwan, M. (2021). Foliar application of silicon nanoparticles affected the growth, vitamin c, flavonoid, and antioxidant enzyme activities of coriander (Coriandrum sativum l.) plants grown in lead (Pb)-spiked soil. Environ. Sci. pollut. Res. Int. 28 (2), 1417–1425. doi: 10.1007/s11356-020-10549-x
Feregrino-Pérez, A. A., Meraz Dávila, S., Pérez García, C. E., Escobar Ortiz, A., Mendoza Jiménez, D., Piña Ramírez, J. E., et al. (2023). “Toxic effects of nanomaterials on plant cellular mechanisms,” in Nanomaterial interactions with plant cellular mechanisms and macromolecules and agricultural implications., vol. 171-209. (Cham: Springer).
Fincheira, P., Tortella, G., Duran, N., Seabra, A. B., Rubilar, O. (2020). Current applications of nanotechnology to develop plant growth inducer agents as an innovation strategy. Crit. Rev. Biotechnol. 40, 15–30. doi: 10.1080/07388551.2019.1681931
Fiol, D. F., Terrile, M. C., Frik, J., Mesas, F. A., Álvarez, V. A., Casalongué, C. A. (2021). Nanotechnology in plants: recent advances and challenges. J. Chem. Technol. Biotechnol. 96, 2095–2108. doi: 10.1002/jctb.6741
Fouda, A., Hassan, S. E.-D., Saied, E., Hamza, M. F. (2021). Photocatalytic degradation of real textile and tannery effluent using biosynthesized magnesium oxide nanoparticles (MgO-NPs), heavy metal adsorption, phytotoxicity, and antimicrobial activity. J. Environ. Chem. Eng. 9 (4), 105346. doi: 10.1016/j.jece.2021.105346
Fu, C., Khan, M. N., Yan, J., Hong, X., Zhao, F., Chen, L., et al. (2023). Mechanisms of nanomaterials for improving plant salt tolerance. Crop Environ. 2, 92–99. doi: 10.1016/j.crope.2023.03.002
Getahun, S., Kefale, H., Gelaye, Y. (2024). Application of precision agriculture technologies for sustainable crop production and environmental sustainability: A systematic review. Sci. World J. 2024, 2126734. doi: 10.1155/2024/2126734
Ghabel, V. K., Karamian, R. (2020). Effects of TiO2 nanoparticles and spermine on antioxidant responses of glycyrrhiza glabra l. @ to cold stress. Acta Botanica Croatica 79 (2), 137–147. doi: 10.37427/botcro-2020-025
Ghani, M. I., Saleem, S., Rather, S. A., Rehmani, M. S., Alamri, S., Rajput, V. D., et al. (2022). Foliar application of zinc oxide nanoparticles: An effective strategy to mitigate drought stress in cucumber seedling by modulating antioxidant defense system and osmolytes accumulation. Chemosphere 289, 133202. doi: 10.1016/j.chemosphere.2021.133202
Ghori, N.-U.-H., Ghori, T., Hayat, M., Imadi, S. R., Gul, A., Altay, V., et al. (2019). Heavy metal stress and responses in plants. Int. J. Environ. Sci. Technol. 16, 1807–1828. doi: 10.1007/s13762-019-02215-8
Giglou, M. T., Giglou, R. H., Esmaeilpour, B., Azarmi, R., Padash, A., Falakian, M., et al. (2022). A new method in mitigation of drought stress by chitosan-coated iron oxide nanoparticles and growth stimulant in peppermint. Ind. Crops Products 187, 115286. doi: 10.1016/j.indcrop.2022.115286
Guerriero, G., Sutera, F. M., Torabi-Pour, N., Renaut, J., Hausman, J.-F., Berni, R., et al. (2021). Phyto-courier, a silicon particle-based nano-biostimulant: evidence from Cannabis sativa exposed to salinity. ACS nano 15, 3061–3069. doi: 10.1021/acsnano.0c09488
Guha, T., Barman, S., Mukherjee, A., Kundu, R. (2020). Nano-scale zero valent iron modulates Fe/Cd transporters and immobilizes soil Cd for production of Cd free rice. Chemosphere 260, 127533. doi: 10.1016/j.chemosphere.2020.127533
Haghighi, M., Abolghasemi, R., da Silva, J. A. T. (2014). Low and high temperature stress affect the growth characteristics of tomato in hydroponic culture with se and nano-se amendment. Scientia Hortic. 178, 231–240. doi: 10.1016/j.scienta.2014.09.006
Haile, G. G., Tang, Q., Li, W., Liu, X., Zhang, X. (2020). Drought: Progress in broadening its understanding. Wiley Interdiscip. Reviews: Water 7, e1407. doi: 10.1002/wat2.1407
Hailu, B., Mehari, H. (2021). Impacts of soil salinity/sodicity on soil-water relations and plant growth in dry land areas: A review. J. Natural Sci. Res. 12, 1–10.
Hamid, B., Zaman, M., Farooq, S., Fatima, S., Sayyed, R., Baba, Z., et al. (2021). Bacterial plant biostimulants: A sustainable way towards improving growth, productivity, and health of crops. Sustainability 13, 2856. doi: 10.3390/su13052856
Han, M.-L., Lv, Q.-Y., Zhang, J., Wang, T., Zhang, C.-X., Tan, R.-J., et al. (2022). Decreasing nitrogen assimilation under drought stress by suppressing DST-mediated activation of Nitrate Reductase 1.2 in rice. Mol. Plant 15, 167–178. doi: 10.1016/j.molp.2021.09.005
Hao, Y., Lv, R., Ma, C., Adeel, M., Zhao, Z., Rao, Y., et al. (2021). Graphitic carbon nitride (g-C3N4) alleviates cadmium-induced phytotoxicity to rice (Oryza sativa L.). Environ. Sci. pollut. Res. 28, 21276–21284. doi: 10.1007/s11356-020-12027-w
Hassan, M. U., Kareem, H. A., Hussain, S., Guo, Z., Niu, J., Roy, M., et al. (2023). Enhanced salinity tolerance in Alfalfa through foliar nano-zinc oxide application: Mechanistic insights and potential agricultural applications. Rhizosphere 28, 100792. doi: 10.1016/j.rhisph.2023.100792
Hassan, N. S., Salah El Din, T. A., Hendawey, M. H., Borai, I. H., Mahdi, A. A. (2018). Magnetite and zinc oxide nanoparticles alleviated heat stress in wheat plants. Curr. nanomaterials 3 (1), 32–43. doi: 10.2174/2405461503666180619160923
Hasanpour, H., Maali-Amir, R., Zeinali, H. (2015). Effect of TiO 2 nanoparticles on metabolic limitations to photosynthesis under cold in chickpea. Russian J. Plant Physiol. 62, 779–787. doi: 10.1134/S1021443715060096
Haydar, M. S., Ghosh, D., Roy, S. (2024). Slow and controlled release nanofertilizers as an efficient tool for sustainable agriculture: Recent understanding and concerns. Plant Nano Biol., 100058. doi: 10.1016/j.plana.2024.100058
Hernández-Fuentes, A. D., López-Vargas, E. R., Pinedo-Espinoza, J. M., Campos-Montiel, R. G., Valdés-Reyna, J., Juárez-Maldonado, A. (2017). Postharvest behavior of bioactive compounds in tomato fruits treated with cu nanoparticles and NaCl stress. Appl. Sci. 7 (10), 980. doi: 10.3390/app7100980
Hezaveh, T. A., Pourakbar, L., Rahmani, F., Alipour, H. (2019). Interactive effects of salinity and ZnO nanoparticles on physiological and molecular parameters of rapeseed (Brassica napus L.). Commun. Soil Sci. Plant Anal. 50, 698–715. doi: 10.1080/00103624.2019.1589481
Hossain, A., Skalicky, M., Brestic, M., Maitra, S., Ashraful Alam, M., Syed, M. A., et al. (2021). Consequences and mitigation strategies of abiotic stresses in wheat (Triticum aestivum L.) under the changing climate. Agronomy 11, 241. doi: 10.3390/agronomy11020241
Hossain, Z., Mustafa, G., Sakata, K., Komatsu, S. (2016). Insights into the proteomic response of soybean towards Al2O3, ZnO, and ag nanoparticles stress. J. hazardous materials 304, 291–305. doi: 10.1016/j.jhazmat.2015.10.071
Huang, K., Boerhan, R., Liu, C., Jiang, G. (2017). Nanoparticles penetrate into the multicellular spheroid-on-chip: effect of surface charge, protein corona, and exterior flow. Mol. Pharmaceutics 14, 4618–4627. doi: 10.1021/acs.molpharmaceut.7b00726
Hussain, S., Shafiq, I., Chattha, M. S., Mumtaz, M., Brestic, M., Rastogi, A., et al. (2021). Effect of ti treatments on growth, photosynthesis, phosphorus uptake and yield of soybean (Glycine max l.) in maize-soybean relay strip intercropping. Environ. Exp. Bot. 187, 104476. doi: 10.1016/j.envexpbot.2021.104476
Hussein, M. M., Abou-Baker, N. H. (2018). The contribution of nano-zinc to alleviate salinity stress on cotton plants. R. Soc. Open Sci. 5 (8), 171809. doi: 10.1098/rsos.171809
Iavicoli, I., Leso, V., Beezhold, D. H., Shvedova, A. A. (2017). Nanotechnology in agriculture: Opportunities, toxicological implications, and occupational risks. Toxicol. Appl. Pharmacol. 329, 96–111. doi: 10.1016/j.taap.2017.05.025
Iqbal, M., Raja, N. I., Mashwani, Z.-U.-R., Hussain, M., Ejaz, M., Yasmeen, F. (2019). Effect of silver nanoparticles on growth of wheat under heat stress. Iranian J. Sci. Technology Trans. A: Sci. 43, 387–395. doi: 10.1007/s40995-017-0417-4
Iqbal, M. S., Singh, A. K., Singh, S. P., Ansari, M. I. (2020). Nanoparticles and plant interaction with respect to stress response. Nanomaterials Environ. Biotechnol. doi: 10.1007/978-3-030-34544-0_1
Igiebor, F. A., Ikhajiagbe, B., Asia, M. (2023). Green nanotechnology: A modern tool for sustainable agriculture in Nigeria–A review. Int. J. Hortic. Sci. Technol. 10, 269–286. doi: 10.22059/ijhst.2022.344790.571
Irshad, M. A., ur Rehman, M. Z., Anwar-ul-Haq, M., Rizwan, M., Nawaz, R., Shakoor, M. B., et al. (2021). Effect of green and chemically synthesized titanium dioxide nanoparticles on cadmium accumulation in wheat grains and potential dietary health risk: A field investigation. J. Hazardous Materials 415, 125585. doi: 10.1016/j.jhazmat.2021.125585
Ishtiaq, M., Mazhar, M. W., Maqbool, M., Hussain, T., Hussain, S. A., Casini, R., et al. (2023). Seed priming with the selenium nanoparticles maintains the redox status in the water stressed tomato plants by modulating the antioxidant defense enzymes. Plants 12 (7), 1556.
Jakhar, A. M., Aziz, I., Kaleri, A. R., Hasnain, M., Haider, G., Ma, J., et al. (2022). Nano-fertilizers: A sustainable technology for improving crop nutrition and food security. NanoImpact 27, 100411. doi: 10.1016/j.impact.2022.100411
Jampílek, J., Kráľová, K. (2022). “Impact of copper-based nanoparticles on economically important plants,” in Copper nanostructures: Next-generation of agrochemicals for sustainable agroecosystems (Elsevier), 293–339.
Javed, R., Gul, A., Arslan Ahmad, M., Guo, W., Ao, Q., Tian, S., et al. (2022). Diverse biotechnological applications of multifunctional titanium dioxide nanoparticles: An up-to-date review. IET nanobiotechnology 16, 171–189. doi: 10.1049/nbt2.12085
Jeon, S. J., Zhang, Y., Castillo, C., Nava, V., Ristroph, K., Therrien, B., et al. (2024). Targeted delivery of sucrose-coated nanocarriers with chemical cargoes to the plant vasculature enhances long-distance translocation. Small 20, 2304588. doi: 10.1002/smll.202304588
Kareem, H. A., Hassan, M. U., Zain, M., Irshad, A., Shakoor, N., Saleem, S., et al. (2022). Nanosized zinc oxide (n-ZnO) particles pretreatment to alfalfa seedlings alleviate heat-induced morpho-physiological and ultrastructural damages. Environ. pollut. 303, 119069. doi: 10.1016/j.envpol.2022.119069
Keller, A. A., Huang, Y., Nelson, J. (2018). Detection of nanoparticles in edible plant tissues exposed to nano-copper using single-particle ICP-MS. J. Nanoparticle Res. 20, 1–13. doi: 10.1007/s11051-018-4192-8
Khalid, M. F., Iqbal Khan, R., Jawaid, M. Z., Shafqat, W., Hussain, S., Ahmed, T., et al. (2022). Nanoparticles: the plant saviour under abiotic stresses. Nanomaterials 12, 3915. doi: 10.3390/nano12213915
Khan, M., Khan, M. S. A., Borah, K. K., Goswami, Y., Hakeem, K. R., Chakrabartty, I. (2021). The potential exposure and hazards of metal-based nanoparticles on plants and environment, with special emphasis on ZnO NPs, TiO2 NPs, and AgNPs: a review. Environ. Adv. 6, 100128. doi: 10.1016/j.envadv.2021.100128
Khan, M. N., Mobin, M., Abbas, Z. K., AlMutairi, K. A., Siddiqui, Z. H. (2017). Role of nanomaterials in plants under challenging environments. Plant Physiol. Biochem. 110, 194–209. doi: 10.1016/j.plaphy.2016.05.038
Khan, I., Awan, S. A., Rizwan, M., Akram, M. A., Zia-ur-Rehman, M., Wang, X., et al. (2023). Physiological and transcriptome analyses demonstrate the silver nanoparticles mediated alleviation of salt stress in pearl millet (Pennisetum glaucum l). Environ. pollut. 318, 120863. doi: 10.1016/j.envpol.2022.120863
Khan, Z. S., Rizwan, M., Hafeez, M., Ali, S., Adrees, M., Qayyum, M. F., et al. (2020). Effects of silicon nanoparticles on growth and physiology of wheat in cadmium contaminated soil under different soil moisture levels. Environ. Sci. pollut. Res. 27, 4958–4968. doi: 10.1007/s11356-019-06673-y
Knop, J.-M., Mukherjee, S., Jaworek, M. W., Kriegler, S., Manisegaran, M., Fetahaj, Z., et al. (2023). Life in multi-extreme environments: brines, osmotic and hydrostatic pressure─A physicochemical view. Chem. Rev. 123, 73–104. doi: 10.1021/acs.chemrev.2c00491
Kumar, A., Choudhary, A., Kaur, H., Guha, S., Mehta, S., Husen, A. (2022). Potential applications of engineered nanoparticles in plant disease management: A critical update. Chemosphere 295, 133798. doi: 10.1016/j.chemosphere.2022.133798
Kurepa, J., Shull, T. E., Smalle, J. A. (2020). Metabolomic analyses of the bio-corona formed on TiO 2 nanoparticles incubated with plant leaf tissues. J. Nanobiotechnology 18, 1–10. doi: 10.1186/s12951-020-00592-8
Kusiak, M., Oleszczuk, P., Jośko, I. (2022). Cross-examination of engineered nanomaterials in crop production: Application and related implications. J. Hazardous Materials 424, 127374. doi: 10.1016/j.jhazmat.2021.127374
Larue, C., Castillo-Michel, H., Sobanska, S., Trcera, N., Sorieul, S., Cécillon, L., et al. (2014). Fate of pristine TiO2 nanoparticles and aged paint-containing TiO2 nanoparticles in lettuce crop after foliar exposure. J. hazardous materials 273, 17–26. doi: 10.1016/j.jhazmat.2014.03.014
Lead, J. R., Batley, G. E., Alvarez, P. J., Croteau, M. N., Handy, R. D., McLaughlin, M. J., et al. (2018). Nanomaterials in the environment: behavior, fate, bioavailability, and effects—an updated review. Environ. Toxicol. Chem. 37, 2029–2063. doi: 10.1002/etc.v37.8
Li, Z., Su, X., Chen, Y., Fan, X., He, L., Guo, J., et al. (2021b). Melatonin improves drought resistance in maize seedlings by enhancing the antioxidant system and regulating abscisic acid metabolism to maintain stomatal opening under PEG-induced drought. J. Plant Biol. 64, 299–312. doi: 10.1007/s12374-021-09297-3
Li, Y., Xie, J., Um, W., You, D. G., Kwon, S., Zhang, L., et al. (2021a). Sono/photodynamic nanomedicine-elicited cancer immunotherapy. Advanced Funct. Materials 31, 2008061. doi: 10.1002/adfm.202008061
Li, Y., Hu, J., Qi, J., Zhao, F., Liu, J., Chen, L., et al. (2022). Improvement of leaf k+ retention is a shared mechanism behind CeO2 and Mn3O4 nanoparticles improved rapeseed salt tolerance. Stress Biol. 2 (1), 46. doi: 10.1007/s44154-022-00065-y
Li, Y., Xu, R., Ma, C., Yu, J., Lei, S., Han, Q., et al. (2023). Potential functions of engineered nanomaterials in cadmium remediation in soil-plant system: a review. Environ. pollut. 122340. doi: 10.1016/j.envpol.2023.122340
Linh, T. M., Mai, N. C., Hoe, P. T., Lien, L. Q., Ban, N. K., Hien, L. T. T., et al. (2020). Metal-based nanoparticles enhance drought tolerance in soybean. J. Nanomaterials 2020 (1), 4056563. doi: 10.1155/2020/4056563
Lian, J., Zhao, L., Wu, J., Xiong, H., Bao, Y., Zeb, A., et al. (2020). Foliar spray of TiO2 nanoparticles prevails over root application in reducing cd accumulation and mitigating cd-induced phytotoxicity in maize (Zea mays l.). Chemosphere 239, 124794. doi: 10.1016/j.chemosphere.2019.124794
López-Moreno, M. L., de la Rosa, G., Hernández-Viezcas, J. A., Peralta-Videa, J. R., Gardea-Torresdey, J. L. (2010). X-ray absorption spectroscopy (XAS) corroboration of the uptake and storage of CeO2 nanoparticles and assessment of their differential toxicity in four edible plant species. J. Agric. Food Chem. 58, 3689–3693. doi: 10.1021/jf904472e
Lv, J., Christie, P., Zhang, S. (2019). Uptake, translocation, and transformation of metal-based nanoparticles in plants: recent advances and methodological challenges. Environ. Science: Nano 6, 41–59. doi: 10.1039/C8EN00645H
Ma, C., White, J. C., Dhankher, O. P., Xing, B. (2015). Metal-based nanotoxicity and detoxification pathways in higher plants. Environ. Sci. Technol. 49, 7109–7122. doi: 10.1021/acs.est.5b00685
Ma, X., Sharifan, H., Dou, F., Sun, W. (2020). Simultaneous reduction of arsenic (As) and cadmium (Cd) accumulation in rice by zinc oxide nanoparticles. Chem. Eng. J. 384, 123802. doi: 10.1016/j.cej.2019.123802
Mahawar, L., Živčák, M., Barboricova, M., Kovár, M., Filaček, A., Ferencova, J., et al. (2024). Effect of copper oxide and zinc oxide nanoparticles on photosynthesis and physiology of Raphanus sativus L. under salinity stress. Plant Physiol. Biochem. 206, 108281. doi: 10.1016/j.plaphy.2023.108281
Malandrakis, A. A., Kavroulakis, N., Chrysikopoulos, C. V. (2019). Use of copper, silver and zinc nanoparticles against foliar and soil-borne plant pathogens. Sci. total Environ. 670, 292–299. doi: 10.1016/j.scitotenv.2019.03.210
Maluin, F. N., Hussein, M. Z., Nik Ibrahim, N. N. L., Wayayok, A., Hashim, N. (2021). Some emerging opportunities of nanotechnology development for soilless and microgreen farming. Agronomy 11, 1213. doi: 10.3390/agronomy11061213
Mansouri, E., Mesbahi, A., Hamishehkar, H., Montazersaheb, S., Hosseini, V., Rajabpour, S. (2023). The effect of nanoparticle coating on biological, chemical and biophysical parameters influencing radiosensitization in nanoparticle-aided radiation therapy. BMC Chem. 17, 180. doi: 10.1186/s13065-023-01099-7
Manzoor, N., Ahmed, T., Noman, M., Shahid, M., Nazir, M. M., Ali, L., et al. (2021). Iron oxide nanoparticles ameliorated the cadmium and salinity stresses in wheat plants, facilitating photosynthetic pigments and restricting cadmium uptake. Sci. Total Environ. 769, 145221. doi: 10.1016/j.scitotenv.2021.145221
Mawale, K. S., Nandini, B., Giridhar, P. (2024). Copper and silver nanoparticle seed priming and foliar spray modulate plant growth and thrips infestation in capsicum spp. ACS omega 9, 3430–3444. doi: 10.1021/acsomega.3c06961
Memari-Tabrizi, E. F., Yousefpour-Dokhanieh, A., Babashpour-Asl, M. (2021). Foliar-applied silicon nanoparticles mitigate cadmium stress through physio-chemical changes to improve growth, antioxidant capacity, and essential oil profile of summer savory (Satureja hortensis L.). Plant Physiol. Biochem. 165, 71–79. doi: 10.1016/j.plaphy.2021.04.040
Mir, A., Pichtel, J., Hayat, S. (2021). Copper: uptake, toxicity and tolerance in plants and management of Cu-contaminated soil. BioMetals 34, 737–759. doi: 10.1007/s10534-021-00306-z
Mirakhorli, T., Ardebili, Z. O., Ladan-Moghadam, A., Danaee, E. (2021). Bulk and nanoparticles of zinc oxide exerted their beneficial effects by conferring modifications in transcription factors, histone deacetylase, carbon and nitrogen assimilation, antioxidant biomarkers, and secondary metabolism in soybean. PloS One 16, e0256905. doi: 10.1371/journal.pone.0256905
Mogazy, A. M., Hanafy, R. S. (2022). Foliar spray of biosynthesized zinc oxide nanoparticles alleviate salinity stress effect on vicia faba plants. J. Soil Sci. Plant Nutr. 22 (2), 2647–2662. doi: 10.1007/s42729-022-00833-9
Mohanavelu, A., Naganna, S. R., Al-Ansari, N. (2021). Irrigation induced salinity and sodicity hazards on soil and groundwater: An overview of its causes, impacts and mitigation strategies. Agriculture 11, 983. doi: 10.3390/agriculture11100983
Moradbeygi, H., Jamei, R., Heidari, R., Darvishzadeh, R. (2020). Investigating the enzymatic and non-enzymatic antioxidant defense by applying iron oxide nanoparticles in dracocephalum moldavica l. plant under salinity stress. Scientia Hortic. 272, 109537. doi: 10.1016/j.scienta.2020.109537
MS, A., Sridharan, K., Puthur, J. T., Dhankher, O. P. (2021). Priming with nanoscale materials for boosting abiotic stress tolerance in crop plants. J. Agric. Food Chem. 69, 10017–10035. doi: 10.1021/acs.jafc.1c03673
Mukarram, M., Petrik, P., Mushtaq, Z., Khan, M. M. A., Gulfishan, M., Lux, A. (2022). Silicon nanoparticles in higher plants: Uptake, action, stress tolerance, and crosstalk with phytohormones, antioxidants, and other signalling molecules. Environ. pollut. 310, 119855. doi: 10.1016/j.envpol.2022.119855
Munns, R., Tester, M. (2008). Mechanisms of salinity tolerance. Annu. Rev. Plant Biol. 59, 651–681. doi: 10.1146/annurev.arplant.59.032607.092911
Naeem, M. A., Abdullah, M., Imran, M., Shahid, M., Abbas, G., Amjad, M., et al. (2022). Iron oxide nanoparticles doped biochar ameliorates trace elements induced phytotoxicity in tomato by modulation of physiological and biochemical responses: implications for human health risk. Chemosphere 289, 133203. doi: 10.1016/j.chemosphere.2021.133203
Nawaz, S., Maqsood, I., Batool, F., Sandhu, Z. Y., Hassan, S., Akram, F., et al. (2023). Improvement of abiotic stress tolerance in plants with the application of nanoparticles. Abiotic Stress Plants-Adaptations to Climate Change. doi: 10.5772/intechopen.110201
Nel, A. E., Mädler, L., Velegol, D., Xia, T., Hoek, E. M., Somasundaran, P., et al. (2009). Understanding biophysicochemical interactions at the nano-bio interface. Nat. Mater 8, 543–557. doi: 10.1038/nmat2442
Nile, S. H., Thiruvengadam, M., Wang, Y., Samynathan, R., Shariati, M. A., Rebezov, M., et al. (2022). Nano-priming as emerging seed priming technology for sustainable agriculture—recent developments and future perspectives. J. nanobiotechnology 20, 254. doi: 10.1186/s12951-022-01423-8
Noman, M., Shahid, M., Ahmed, T., Tahir, M., Naqqash, T., Muhammad, S., et al. (2020). Green copper nanoparticles from a native klebsiella pneumoniae strain alleviated oxidative stress impairment of wheat plants by reducing the chromium bioavailability and increasing the growth. Ecotoxicology Environ. Saf. 192, 110303. doi: 10.1016/j.ecoenv.2020.110303
Noohpisheh, Z., Amiri, H., Mohammadi, A., Farhadi, S. (2021). Effect of the foliar application of zinc oxide nanoparticles on some biochemical and physiological parameters of trigonella foenum-graecum under salinity stress. Plant Biosystems-An Int. J. Dealing all Aspects Plant Biol. 155 (2), 267–280. doi: 10.1080/11263504.2020.1739160
Omar, A. A., Heikal, Y. M., Zayed, E. M., Shamseldin, S. A., Salama, Y. E., Amer, K. E., et al. (2023). Conferring of drought and heat stress tolerance in wheat (Triticum aestivum L.) genotypes and their response to selenium nanoparticles application. Nanomaterials 13, 998. doi: 10.3390/nano13060998
Orimoloye, I., Belle, J., Orimoloye, Y., Olusola, A., Ololade, O. (2022). Drought: A common environmental disaster. Atmosphere. 13, 111. doi: 10.3390/atmos13010111
Pallavi, Mehta, C. M., Srivastava, R., Arora, S., Sharma, A. K. (2016). Impact assessment of silver nanoparticles on plant growth and soil bacterial diversity. 3 Biotech. 6, 1–10. doi: 10.1007/s13205-016-0567-7
Pathak, H., Kumar, M., Molla, K. A., Chakraborty, K. (2021). Abiotic stresses in rice production: Impacts and management. Oryza 58, 103–125. doi: 10.35709/ory.2019.56.2
Pérez-Labrada, F., López-Vargas, E. R., Ortega-Ortiz, H., Cadenas-Pliego, G., Benavides-Mendoza, A., Juárez-Maldonado, A. (2019). Responses of tomato plants under saline stress to foliar application of copper nanoparticles. Plants 8 (6), 151. doi: 10.3390/plants8060151
Pérez-Labrada, F., Hernández-Hernández, H., López-Pérez, M., González-Morales, S., Benavides-Mendoza, A., Juárez-Maldonado, A. (2020). “Nanoparticles in plants: morphophysiological, biochemical, and molecular responses,” in Plant life under changing environment (Academic Press).
Potter, M., Deakin, J., Cartwright, A., Hortin, J., Sparks, D., Anderson, A. J., et al. (2021). Absence of nanoparticle-induced drought tolerance in nutrient sufficient wheat seedlings. Environ. Sci. Technol. 55 (20), 13541–13550. doi: 10.1021/acs.est.1c00453
Ragab, S. M., Turoop, L., Runo, S., Nyanjom, S. (2022). The effect of foliar application of zinc oxide nanoparticles and moringa oleifera leaf extract on growth, biochemical parameters and in promoting salt stress tolerance in faba bean. Afr. J. Biotechnol. 21 (6), 252–266. doi: 10.5897/AJB2022.17485
Rahman, S. U., Wang, X., Shahzad, M., Bashir, O., Li, Y., Cheng, H. (2022). A review of the influence of nanoparticles on the physiological and biochemical attributes of plants with a focus on the absorption and translocation of toxic trace elements. Environ. pollut., 119916. doi: 10.1016/j.envpol.2022.119916
Rajpal, V., Chaurasia, S., Mehta, S., Minkina, T., Rajput, V., Deswal, R. (2023). A comprehensive review on mitigating abiotic stresses in plants by metallic nanomaterials: prospects and concerns. Clean Technol. Environ. Policy. 25, 1–39. doi: 10.1007/s10098-023-02561-9
Rastogi, A., Zivcak, M., Sytar, O., Kalaji, H. M., He, X., Mbarki, S., et al. (2017). Impact of metal and metal oxide nanoparticles on plant: A critical review. Front. Chem. 5. doi: 10.3389/fchem.2017.00078
Rawat, N., Singla-Pareek, S. L., Pareek, A. (2021). Membrane dynamics during individual and combined abiotic stresses in plants and tools to study the same. Physiologia Plantarum 171, 653–676. doi: 10.1111/ppl.v171.4
Rizwan, M., Ali, S., ur Rehman, M. Z., Malik, S., Adrees, M., Qayyum, M. F., et al. (2019). Effect of foliar applications of silicon and titanium dioxide nanoparticles on growth, oxidative stress, and cadmium accumulation by rice (Oryza sativa). Acta physiologiae plantarum 41, 1–12. doi: 10.1007/s11738-019-2828-7
Rui, M., Ma, C., Hao, Y., Guo, J., Rui, Y., Tang, X., et al. (2016). Iron oxide nanoparticles as a potential iron fertilizer for peanut (Arachis hypogaea). Front. Plant Sci. 7. doi: 10.3389/fpls.2016.00815
Salachna, P., Byczyńska, A., Zawadzińska, A., Piechocki, R., Mizielińska, M. (2019). Stimulatory effect of silver nanoparticles on the growth and flowering of potted oriental lilies. Agronomy 9 (10), 610. doi: 10.3390/agronomy9100610
Santana, I., Wu, H., Hu, P., Giraldo, J. P. (2020). Targeted delivery of nanomaterials with chemical cargoes in plants enabled by a biorecognition motif. Nat. Commun. 11, 2045. doi: 10.1038/s41467-020-15731-w
Sardar, R., Ahmed, S., Yasin, N. A. (2022). Titanium dioxide nanoparticles mitigate cadmium toxicity in coriandrum sativum l. through modulating antioxidant system, stress markers and reducing cadmium uptake. Environ. pollut. 292, 118373. doi: 10.1016/j.envpol.2021.118373
Sarkar, M., Rashid, H. O., Rahman, A., Kafi, M. A., Hosen, I. (2022). Recent advances in nanomaterials based sustainable agriculture: An overview. Environ. Nanotechnology Monit. Manage. 18, 100687. doi: 10.1016/j.enmm.2022.100687
Sarkar, M. M., Rudra, P., Paul, P., Dua, T. K., Roy, S. (2024). Enhanced adaptation to salinity stress in lentil seedlings through the use of trehalose-functionalized silica nanoparticles (TSiNPs): Exploring silica-sugar absorption and oxidative balance. Plant Physiol. Biochem. 206, 108309. doi: 10.1016/j.plaphy.2023.108309
Sarkar, M. M., Mukherjee, S., Mathur, P., Roy, S. (2022). Exogenous nano-silicon application improves ion homeostasis, osmolyte accumulation and palliates oxidative stress in lens culinaris under NaCl stress. Plant Physiol. Biochem. 192, 143–161. doi: 10.1016/j.plaphy.2022.10.001
Sarwar, N., Imran, M., Shaheen, M., Ishaque, W., Kamran, A., Matloob, A., et al. (2017). Phytoremediation strategies for soils contaminated with heavy metals: Modifications and future perspectives. Chemosphere 171, 710–721. doi: 10.1016/j.chemosphere.2016.12.116
Seleiman, M. F., Al-Selwey, W. A., Ibrahim, A. A., Shady, M., Alsadon, A. A. (2023). Foliar applications of ZnO and SiO2 nanoparticles mitigate water deficit and enhance potato yield and quality traits. Agronomy 13 (2), 466. doi: 10.3390/agronomy13020466
Semida, W. M., Abdelkhalik, A., Mohamed, G. F., Abd El-Mageed, T. A., Abd El-Mageed, S. A., Rady, M. M., et al. (2021). Foliar application of zinc oxide nanoparticles promotes drought stress tolerance in eggplant (Solanum melongena l.). Plants 10 (2), 421. doi: 10.3390/plants10020421
Shabnam, N., Kim, M., Kim, H. (2019). Iron (III) oxide nanoparticles alleviate arsenic induced stunting in vigna radiata. Ecotoxicology Environ. Saf. 183, 109496. doi: 10.1016/j.ecoenv.2019.109496
Shah, A. A., Ahmed, S., Malik, A., Naheed, K., Hussain, S., Yasin, N. A., et al. (2022). Potassium silicate and zinc oxide nanoparticles modulate antioxidant system, membranous h+-ATPase and nitric oxide content in faba bean (Vicia faba) seedlings exposed to arsenic toxicity. Funct. Plant Biol. doi: 10.1071/FP21301
Shang, H., Guo, H., Ma, C., Li, C., Chefetz, B., Polubesova, T., et al. (2019a). Maize (Zea mays L.) root exudates modify the surface chemistry of CuO nanoparticles: Altered aggregation, dissolution and toxicity. Sci. Total Environ. 690, 502–510. doi: 10.1016/j.scitotenv.2019.07.017
Shang, Y., Hasan, M. K., Ahammed, G. J., Li, M., Yin, H., Zhou, J. (2019b). Applications of nanotechnology in plant growth and crop protection: A review. Molecules 24, 2558. doi: 10.3390/molecules24142558
Shanmugavel, D., Rusyn, I., Solorza-Feria, O., Kamaraj, S.-K. (2023). Sustainable SMART fertilizers in agriculture systems: A review on fundamentals to in-field applications. Sci. Total Environ. 904, 166729. doi: 10.1016/j.scitotenv.2023.166729
Sheikhalipour, M., Esmaielpour, B., Gohari, G., Haghighi, M., Jafari, H., Farhadi, H., et al. (2021). Salt Stress Mitigation via the Foliar Application of Chitosan-Functionalized Selenium and Anatase Titanium Dioxide Nanoparticles in Stevia (Stevia rebaudiana Bertoni). Molecules 26, 4090. doi: 10.3390/molecules26134090
Shenashen, M., Derbalah, A., Hamza, A., Mohamed, A., El Safty, S. (2017). Antifungal activity of fabricated mesoporous alumina nanoparticles against root rot disease of tomato caused by fusarium oxysporium. Pest Manage. Sci. 73 (6), 1121–1126. doi: 10.1002/ps.4420
Singh, A., Sharma, A., Singh, O., Rajput, V. D., Movsesyan, H. S., Minkina, T., et al. (2024). In-depth exploration of nanoparticles for enhanced nutrient use efficiency and abiotic stresses management: present insights and future horizons. Plant Stress, 100576. doi: 10.1016/j.stress.2024.100576
Singh, A., Tiwari, S., Pandey, J., Lata, C., Singh, I. (2021a). Role of nanoparticles in crop improvement and abiotic stress management. J. Biotechnol. 337, 57–70. doi: 10.1016/j.jbiotec.2021.06.022
Singh, A., Tiwari, S., Pandey, J., Lata, C., Singh, I. K. (2021b). Role of nanoparticles in crop improvement and abiotic stress management. J. Biotechnol. 337, 57–70. doi: 10.1016/j.jbiotec.2021.06.022
Song, Y., Jiang, M., Zhang, H., Li, R. (2021). Zinc oxide nanoparticles alleviate chilling stress in rice (Oryza sativa l.) by regulating antioxidative system and chilling response transcription factors. Molecules 26 (8), 2196. doi: 10.3390/molecules26082196
Srivastav, A., Shukla, A., Singhal, R. K., Srivastav, S., Ganjewala, D., Shrivastava, M. (2023). Soil and plant enzymes responses to zinc oxide nanoparticles in submerged rice (Oryza sativa l.) ecosystem. Trends Sci. 20 (9), 5558–5558. doi: 10.48048/tis.2023.5558
Staedler, Y. M., Masson, D., Schönenberger, J. (2013). Plant tissues in 3D via X-ray tomography: simple contrasting methods allow high resolution imaging. PloS One 8, e75295. doi: 10.1371/journal.pone.0075295
Stegemeier, J. P., Colman, B. P., Schwab, F., Wiesner, M. R., Lowry, G. V. (2017). Uptake and distribution of silver in the aquatic plant Landoltia punctata (duckweed) exposed to silver and silver sulfide nanoparticles. Environ. Sci. Technol. 51, 4936–4943. doi: 10.1021/acs.est.6b06491
Sturikova, H., Krystofova, O., Huska, D., Adam, V. (2018). Zinc, zinc nanoparticles and plants. J. Hazard Mater 349, 101–110. doi: 10.1016/j.jhazmat.2018.01.040
Su, Y., Ashworth, V., Kim, C., Adeleye, A. S., Rolshausen, P., Roper, C., et al. (2019). Delivery, uptake, fate, and transport of engineered nanoparticles in plants: a critical review and data analysis. Environ. Science: Nano 6, 2311–2331. doi: 10.1039/C9EN00461K
Sun, L., Song, F., Guo, J., Zhu, X., Liu, S., Liu, F., et al. (2020). Nano-ZnO-induced drought tolerance is associated with melatonin synthesis and metabolism in maize. Int. J. Mol. Sci. 21 (3), 782. doi: 10.3390/ijms21030782
Sutulienė, R., Ragelienė, L., Samuolienė, G., Brazaitytė, A., Urbutis, M., Miliauskienė, J. (2021). The response of antioxidant system of drought-stressed green pea (Pisum sativum l.) affected by watering and foliar spray with silica nanoparticles. Horticulturae 8 (1), 35. doi: 10.3390/horticulturae8010035
Swain, S., Mishra, S., Pandey, A., Kalura, P. (2022). Inclusion of groundwater and socio-economic factors for assessing comprehensive drought vulnerability over Narmada River Basin, India: A geospatial approach. Appl. Water Sci. 12, 14. doi: 10.1007/s13201-021-01529-8
Takshak, S., Agrawal, S. (2014). Effect of ultraviolet-B radiation on biomass production, lipid peroxidation, reactive oxygen species, and antioxidants in Withania somnifera. Biol. plantarum 58, 328–334. doi: 10.1007/s10535-014-0390-0
Tarolli, P., Luo, J., Park, E., Barcaccia, G., Masin, R. (2024). Soil salinization in agriculture: Mitigation and adaptation strategies combining nature-based solutions and bioengineering. Iscience 27. doi: 10.1016/j.isci.2024.108830
Thakare, M., Sarma, H., Datar, S., Roy, A., Pawar, P., Kanupriya, et al. (2021). Understanding the holistic approach to plant-microbe remediation technologies for removing heavy metals and radionuclides from soil. Curr. Res. Biotechnol. 3, 84–98. doi: 10.1016/j.crbiot.2021.02.004
Thind, S., Hussain, I., Ali, S., Rasheed, R., Ashraf, M. A. (2021). Silicon application modulates growth, physio-chemicals, and antioxidants in wheat (Triticum aestivum l.) exposed to different cadmium regimes. Dose-Response 19 (2), 15593258211014646. doi: 10.1177/15593258211014646
Thiruvengadam, M., Chi, H. Y., Kim, S.-H. (2024). Impact of nanopollution on plant growth, photosynthesis, toxicity, and metabolism in the agricultural sector: An updated review. Plant Physiol. Biochem., 108370. doi: 10.1016/j.plaphy.2024.108370
Tolaymat, T., Genaidy, A., Abdelraheem, W., Dionysiou, D., Andersen, C. (2017). The effects of metallic engineered nanoparticles upon plant systems: An analytic examination of scientific evidence. Sci. Total Environ. 579, 93–106. doi: 10.1016/j.scitotenv.2016.10.229
Tortella, G., Rubilar, O., Pieretti, J. C., Fincheira, P., de Melo Santana, B., Fernández-Baldo, M. A., et al. (2023). Nanoparticles as a promising strategy to mitigate biotic stress in agriculture. Antibiotics 12, 338. doi: 10.3390/antibiotics12020338
Tripathi, S., Srivastava, P., Devi, R. S., Bhadouria, R. (2020). “Influence of synthetic fertilizers and pesticides on soil health and soil microbiology,” in Agrochemicals detection, treatment and remediation (Butterworth-Heinemann: Elsevier), 25–54.
Tripathi, D. K., Singh, V. P., Prasad, S. M., Chauhan, D. K., Dubey, N. K. (2015). Silicon nanoparticles (SiNp) alleviate chromium (VI) phytotoxicity in pisum sativum (L.) seedlings. Plant Physiol. Biochem. 96, 189–198. doi: 10.1016/j.plaphy.2015.07.026
Truscă, M., Gâdea, s., Vidican, R., Stoian, V., Vâtcă, A., Balint, C., et al. (2023). Exploring the research challenges and perspectives in ecophysiology of plants affected by salinity stress. Agriculture 13, 734. doi: 10.3390/agriculture13030734
Tuga, B., O’Keefe, T., Deng, C., Ligocki, A. T., White, J. C., Haynes, C. L. (2023). Designing nanoparticles for sustainable agricultural applications. Trends Chem. 5, 814–826. doi: 10.1016/j.trechm.2023.07.004
Vasseghian, Y., Arunkumar, P., Joo, S.-W., Gnanasekaran, L., Kamyab, H., Rajendran, S., et al. (2022). Metal-organic framework-enabled pesticides are an emerging tool for sustainable cleaner production and environmental hazard reduction. J. Cleaner Production 373, 133966. doi: 10.1016/j.jclepro.2022.133966
Verma, V., Al-Dossari, M., Singh, J., Rawat, M., Kordy, M. G. M., Shaban, M. (2022). A review on green synthesis of TiO2 NPs: photocatalysis and antimicrobial applications. Polymers 14 (7), 1444. doi: 10.3390/polym14071444
Wahab, A., Muhammad, M., Ullah, S., Abdi, G., Shah, G. M., Zaman, W., et al. (2024). Agriculture and environmental management through nanotechnology: Eco-friendly nanomaterial synthesis for soil-plant systems, food safety, and sustainability. Sci. Total Environ., 171862. doi: 10.1016/j.scitotenv.2024.171862
Wahid, I., Rani, P., Kumari, S., Ahmad, R., Hussain, S. J., Alamri, S., et al. (2022). Biosynthesized gold nanoparticles maintained nitrogen metabolism, nitric oxide synthesis, ions balance, and stabilizes the defense systems to improve salt stress tolerance in wheat. Chemosphere 287, 132142. doi: 10.1016/j.chemosphere.2021.132142
Wang, X., Xie, H., Wang, P., Yin, H. (2023). Nanoparticles in plants: uptake, transport and physiological activity in leaf and root. Materials 16, 3097. doi: 10.3390/ma16083097
Wang, Z., Yue, L., Dhankher, O. P., Xing, B. (2020). Nano-enabled improvements of growth and nutritional quality in food plants driven by rhizosphere processes. Environ. Int. 142, 105831. doi: 10.1016/j.envint.2020.105831
Wang, S., Fu, Y., Zheng, S., Xu, Y., Sun, Y. (2022). Phytotoxicity and accumulation of copper-based nanoparticles in brassica under cadmium stress. Nanomaterials 12 (9), 1497. doi: 10.3390/nano12091497
Wang, L., Wu, W.-M., Bolan, N. S., Tsang, D. C. W., Li, Y., Qin, M., et al. (2021). Environmental fate, toxicity and risk management strategies of nanoplastics in the environment: Current status and future perspectives. J. hazardous materials 401, 123415. doi: 10.1016/j.jhazmat.2020.123415
Wani, M. Y., Ganie, N. A., Dar, K. A., Dar, S. Q., Khan, A. H., Khan, N. A., et al. (2023). Nanotechnology future in food using carbohydrate macromolecules: A state-of-the-art review. Int. J. Biol. Macromolecules 239, 124350. doi: 10.1016/j.ijbiomac.2023.124350
Werlin, R., Priester, J., Mielke, R., Krämer, S., Jackson, S., Stoimenov, P., et al. (2011). Biomagnification of cadmium selenide quantum dots in a simple experimental microbial food chain. Nat. nanotechnology 6, 65–71. doi: 10.1038/nnano.2010.251
Wu, J., Sun, J., Bosker, T., Vijver, M. G., Peijnenburg, W. J. (2023). Toxicokinetics and particle number-based trophic transfer of a metallic nanoparticle mixture in a terrestrial food chain. Environ. Sci. Technol. 57, 2792–2803. doi: 10.1021/acs.est.2c07660
Xiong, T., Dumat, C., Dappe, V., Vezin, H., Schreck, E., Shahid, M., et al. (2017). Copper oxide nanoparticle foliar uptake, phytotoxicity, and consequences for sustainable urban agriculture. Environ. Sci. Technol. 51, 5242–5251. doi: 10.1021/acs.est.6b05546
Yaashikaa, P., Kumar, P. S., Jeevanantham, S., Saravanan, R. (2022). A review on bioremediation approach for heavy metal detoxification and accumulation in plants. Environ. pollut. 301, 119035. doi: 10.1016/j.envpol.2022.119035
Yadav, A., Yadav, K., Ahmad, R., Abd-Elsalam, K. A. (2023). Emerging frontiers in nanotechnology for precision agriculture: advancements, hurdles and prospects. Agrochemicals 2, 220–256. doi: 10.3390/agrochemicals2020016
Yan, A., Wang, Y., Tan, S. N., Mohd Yusof, M. L., Ghosh, S., Chen, Z. (2020). Phytoremediation: A promising approach for revegetation of heavy metal-polluted land. Front. Plant Sci. 11. doi: 10.3389/fpls.2020.00359
Yan, S., Wu, F., Zhou, S., Yang, J., Tang, X., Ye, W. (2021). Zinc oxide nanoparticles alleviate the arsenic toxicity and decrease the accumulation of arsenic in rice (Oryza sativa l.). BMC Plant Biol. 21, 1–11. doi: 10.1186/s12870-021-02929-3
Yang, J., Cao, W., Rui, Y. (2017a). Interactions between nanoparticles and plants: phytotoxicity and defense mechanisms. J. Plant Interact. 12, 158–169. doi: 10.1080/17429145.2017.1310944
Yang, J., Cao, W., Yukui, R. (2017b). Interactions between nanoparticles and plants: phytotoxicity and defense mechanisms. J. Plant Interact. 12, 158–169. doi: 10.1080/17429145.2017.1310944
Yang, R., Diao, Y., Abayneh, B. (2018). Removal of Hg0 from simulated flue gas over silver-loaded rice husk gasification char. R. Soc. Open Sci. 5 (9), 180248.
Ye, Y., Medina Velo, I., Cota Ruiz, K., Moreno Olivas, F., Gardea-Torresdey, J. (2019). Can abiotic stresses in plants be alleviated by manganese nanoparticles or compounds? Ecotoxicology Environ. Saf. 184, 109671. doi: 10.1016/j.ecoenv.2019.109671
Yosefi, A., Mozafari, A. A., Javadi, T. (2022). In vitro assessment of strawberry (Fragaria× ananassa duch.) plant responses to water shortage stress under nano-iron application. In Vitro Cell. Dev. Biology-Plant 58 (4), 499–510. doi: 10.1007/s11627-022-10255-y
Yoshida, T., Fujita, Y., Maruyama, K., Mogami, J., Todaka, D., Shinozaki, K., et al. (2015). Four A rabidopsis AREB/ABF transcription factors function predominantly in gene expression downstream of SnRK2 kinases in abscisic acid signalling in response to osmotic stress. Plant Cell Environ. 38, 35–49. doi: 10.1111/pce.2015.38.issue-1
Yuan, F., Guo, J., Shabala, S., Wang, B. (2019). Reproductive physiology of halophytes: current standing. Front. Plant Sci. 9. doi: 10.3389/fpls.2018.01954
Yue, L., Ma, C., Zhan, X., White, J. C., Xing, B. (2017). Molecular mechanisms of maize seedling response to La 2 O 3 NP exposure: water uptake, aquaporin gene expression and signal transduction. Environ. Science: Nano 4, 843–855. doi: 10.1039/C6EN00487C
Zahedi, S. M., Moharrami, F., Sarikhani, S., Padervand, M. (2020). Selenium and silica nanostructure-based recovery of strawberry plants subjected to drought stress. Sci. Rep. 10 (1), 17672. doi: 10.1016/j.ecoenv.2020.111396
Zain, M., Ma, H., Chaudhary, S., Nuruzaman, M., Azeem, I., Mehmood, F., et al. (2023). Nanotechnology in precision agriculture: Advancing towards sustainable crop production. Plant Physiol. Biochem., 108244. doi: 10.2139/ssrn.4663507
Zhang, P., Guo, Z., Ullah, S., Melagraki, G., Afantitis, A., Lynch, I. (2021). Nanotechnology and artificial intelligence to enable sustainable and precision agriculture. Nat. Plants 7, 864–876. doi: 10.1038/s41477-021-00946-6
Zhang, P., Guo, Z., Zhang, Z., Fu, H., White, J. C., Lynch, I. (2020). Nanomaterial transformation in the soil–plant system: implications for food safety and application in agriculture. Small 16, 2000705. doi: 10.1002/smll.202000705
Zhao, L., Bai, T., Wei, H., Gardea-Torresdey, J. L., Keller, A., White, J. C. (2022). Nanobiotechnology-based strategies for enhanced crop stress resilience. Nat. Food 3, 829–836. doi: 10.1038/s43016-022-00596-7
Zhao, W., Wu, Z., Amde, M., Zhu, G., Wei, Y., Zhou, P., et al. (2023). Nanoenabled enhancement of plant tolerance to heat and drought stress on molecular response. J. Agric. Food Chem. 71, 20405–20418. doi: 10.1021/acs.jafc.3c04838
Zhao, L., Peng, B., Hernandez-Viezcas, J. A., Rico, C., Sun, Y., Peralta-Videa, J. R., et al. (2012). Stress response and tolerance of zea mays to CeO2 nanoparticles: cross talk among H2O2, heat shock protein, and lipid peroxidation. ACS nano 6 (11), 9615–9622. doi: 10.1021/nn302975u
Zhou, P., Jiang, Y., Adeel, M., Shakoor, N., Zhao, W., Liu, Y., et al. (2023a). Nickel oxide nanoparticles improve soybean yield and enhance nitrogen assimilation. Environ. Sci. Technol. 57, 7547–7558. doi: 10.1021/acs.est.3c00959
Zhou, P., Wang, L., Gao, J., Jiang, Y., Adeel, M., Hou, D. (2023b). Nanoplastic–plant interaction and implications for soil health. Soil Use Manage. 39, 13–42. doi: 10.1111/sum.12868
Zhou, P., Adeel, M., Shakoor, N., Guo, M., Hao, Y., Azeem, I., et al. (2020). Application of nanoparticles alleviates heavy metals stress and promotes plant growth: An overview. Nanomaterials 11 (1), 26. doi: 10.3390/nano11010026
Zia, R., Nawaz, M. S., Siddique, M. J., Hakim, S., Imran, A. (2021). Plant survival under drought stress: Implications, adaptive responses, and integrated rhizosphere management strategy for stress mitigation. Microbiological Res. 242, 126626. doi: 10.1016/j.micres.2020.126626
Zou, X.-Y., Xu, B., Yu, C.-P., Zhang, H.-W. (2013). Imbalance between oxidative and antioxidative systems: toward an understanding of visible light-induced titanium dioxide nanoparticles toxicity. Chemosphere 93, 2451–2457. doi: 10.1016/j.chemosphere.2013.08.076
Zulfiqar, F., Ashraf, M. (2021a). Nanoparticles potentially mediate salt stress tolerance in plants. Plant Physiol. Biochem. 160, 257–268. doi: 10.1016/j.plaphy.2021.01.028
Zulfiqar, F., Ashraf, M. (2021b). Nanoparticles potentially mediate salt stress tolerance in plants. Plant Physiol. biochemistry: PPB 160, 257–268. doi: 10.1016/j.plaphy.2021.01.028
Zuverza-Mena, N., Martínez-Fernández, D., Du, W., Hernandez-Viezcas, J. A., Bonilla-Bird, N., López-Moreno, M. L., et al. (2017). Exposure of engineered nanomaterials to plants: Insights into the physiological and biochemical responses-A review. Plant Physiol. Biochem. 110, 236–264. doi: 10.1016/j.plaphy.2016.05.037
Keywords: climate change, nanotechnology, abiotic stress, nanoparticles, sustainable agriculture, plant stress mitigation
Citation: Cao Y, Turk K, Bibi N, Ghafoor A, Ahmed N, Azmat M, Ahmed R, Ghani MI and Ahanger MA (2025) Nanoparticles as catalysts of agricultural revolution: enhancing crop tolerance to abiotic stress: a review. Front. Plant Sci. 15:1510482. doi: 10.3389/fpls.2024.1510482
Received: 13 October 2024; Accepted: 10 December 2024;
Published: 17 January 2025.
Edited by:
Muhammad Shoaib Rana, South China Agricultural University, ChinaReviewed by:
Subhash Babu, ICAR-Indian Agricultural Research Institute, IndiaMuhammad Tahir, University of Minnesota Twin Cities, United States
Afef Sai, University of Gafsa, Tunisia
Ansar Ali, The University of Texas at Austin, United States
Copyright © 2025 Cao, Turk, Bibi, Ghafoor, Ahmed, Azmat, Ahmed, Ghani and Ahanger. This is an open-access article distributed under the terms of the Creative Commons Attribution License (CC BY). The use, distribution or reproduction in other forums is permitted, provided the original author(s) and the copyright owner(s) are credited and that the original publication in this journal is cited, in accordance with accepted academic practice. No use, distribution or reproduction is permitted which does not comply with these terms.
*Correspondence: Khalid Turk, a3R1cmtAa2Z1LmVkdS5zYQ==; Muhammad Imran Ghani, SW1yYW5fcGFrQG53c3VhZi5lZHUuY24=; Muhammad Abass Ahanger, YWhhbmdlcm1hQGdtYWlsLmNvbQ==
†These authors have contributed equally to this work