- 1Natural Resources and Environmental Laboratory, Taza Polydisciplinary Faculty, Sidi Mohamed Ben Abdellah University, Fez, Morocco
- 2Microbial Biotechnology and Bioactive Molecules Laboratory, Sciences and Technology Faculty, Sidi Mohamed Ben Abdellah University, Fez, Morocco
- 3Department of Biological Science and Chemistry, College of Arts and Science, University of Nizwa, Nizwa, Oman
With climate change, the frequency of regions experiencing water scarcity is increasing annually, posing a significant challenge to crop yield. Barley, a staple crop consumed and cultivated globally, is particularly susceptible to the detrimental effects of drought stress, leading to reduced yield production. Water scarcity adversely affects multiple aspects of barley growth, including seed germination, biomass production, shoot and root characteristics, water and osmotic status, photosynthesis, and induces oxidative stress, resulting in considerable losses in grain yield and its components. In this context, the present review aims to underscore the importance of selecting drought-tolerant barley genotypes and utilizing bio-inoculants constructed from beneficial microorganisms as an agroecological approach to enhance barley growth and production resilience under varying environmental conditions. Selecting barley genotypes with robust physiological and agronomic tolerance can mitigate losses under diverse environmental conditions. Plant Growth Promoting Rhizobacteria (PGPR) play a crucial role in promoting plant growth through nutrient solubilization, nitrogen fixation, phytohormone production, exopolysaccharide secretion, enzyme activity enhancement, and many other mechanisms. Applying drought-tolerant genotypes with bio-inoculants containing PGPR, improves barley's drought tolerance thereby minimizing losses caused by water scarcity.
1 Introduction
Barley (Hordeum vulgare L.) comes in fourth place among most cereals cultivated around the world. Historically, barley is among the first domesticated crops (Geng et al., 2021). Now, it is widely used in food, animal feed, brewing, distillation, forage, and many other industrial uses (Bouhraoua et al., 2024). Ensuring barley supply is becoming increasingly challenging due to the continuous increase in the global human population, especially considering that the majority of individuals depend on agriculture for their livelihoods (Castaneda et al., 2016). Indeed, the risks associated with abiotic stresses such as drought, salinity, and heat are escalating annually due to climate change.
Drought is among the major stresses that affect barley crops and can cause more than 50% of yield losses (Kebede et al., 2020). Several studies showed ridiculous changes in barley plants subjected to drought stress when compared to the unstressed ones. Drought affects root and shoot length and weight (Abou-Elwafa, 2016), reduces relative water content (Hasanuzzaman et al., 2019), affects membrane steadiness (Ferioun et al., 2024), alters photosynthesis efficiency (Ghotbi-Ravandi et al., 2014), induces some metabolites accumulation, stimulates reactive oxygen species accumulation (Sallam et al., 2019) and many other physiological changes, which impacts the grain yield (Ferioun et al., 2024). Hence, increasing barley production by expanding land areas for cultivation is not a straightforward solution. Furthermore, relying solely on chemical fertilizers is not an agroecological solution.
Selecting high-performing genotypes is among the solutions that many studies have endeavoured to pursue with the aim of minimizing crop losses attributable to water scarcity. It is challenging to select the most high-yielding genotypes due to significant genotype x environment interactions (Boussakouran et al., 2021). Therefore, testing various genotypes in different environments is widely recommended to identify ideal genotypes that shown high yield production and are less affected by stresses (Boussakouran et al., 2021; Bouhraoua et al., 2023).
Investigating alternative strategies to increase barley output while reducing the negative environmental effects associated with traditional agricultural techniques has gained traction in recent years. A bio-inoculant based on Plant Growth Promoting Rhizobacteria (PGPR), mycorrhizae (Vafa et al., 2021; Jabborova et al., 2022; Vafa et al., 2024), and/or algae is considered as an agroecological solution that offers numerous benefits to various crops in both non-stressful and stressful conditions Ahluwalia et al., 2021; Al-Turki et al., 2023). The selection of microorganisms used for bio-inoculant production is mostly based on plant growth promoting traits such as nutrients solubilization, phytohormones production, nitrogen fixation, exopolysaccharides secretion, and enzymes activities (Etesami and Maheshwari, 2018; Kumar et al., 2019).
In barley growth, bio-inoculants have demonstrated a significant improvement in various environmental conditions. Barley plants inoculated with bio-inoculants, when compared to uninoculated ones, exhibited higher leaf relative water content, increased biomass production, improved grain quality and quantity, and lower sensitivity indices (Baris et al., 2014; Jodeh et al., 2015; Slimani et al., 2023a). Furthermore, the positive effects of bio-inoculants extend to improving soil health in the rhizosphere (Tirry et al., 2023). The utilization of bio-inoculants as fertilizers in the field is continuously progressing, although it remains limited compared to chemical fertilization practices (Bittencourt et al., 2023). In light of the numerous disadvantages associated with chemical fertilizers, bio-inoculants appear to offer a sustainable and agroecological solution for enhancing barley resilience to drought.
The present study aimed to investigate bio-inoculant advancements and breeding techniques to enhance barley tolerance to drought conditions. We also explored the potential synergistic approach of combining robust barley genotypes with biofertilizers for improved crop production in barley fields.
2 Drought stress in the context of global climate change
In recent decades, there has been growing concern about worsening global weather conditions and its impact on agriculture. The persistent build-up of greenhouse gases (GHGs) in the Earth's atmosphere is forecasted to intensify soon. This escalation is expected to elevate the average global temperature, alter precipitation patterns, and amplify the occurrence and intensity of extreme weather events. Consequently, this leads to the phenomenon known as climate change, marked by reduced rainfall, prolonged droughts, intense winds, or flooding that can damage crops and lead to losses after harvesting (Toulotte et al., 2022; Mutengwa et al., 2023). One of the biggest challenges is the increase in drought and water scarcity (Vila-Traver et al., 2021; Bogati and Walczak, 2022). The future prediction indicates that climate change will result in heightened occurrences of droughts in most parts of the world. The climate forecasts indicate a potential rise in average temperatures ranging between 0.5° to 5.6°C, varying according to different scenarios. Moreover, these projections suggest a continual decrease in rainfall between April to September, estimating a reduction of approximately 4% for every degree Celsius of global warming (Cramer et al., 2020). The affected area is anticipated to surge from 15.4% to 44% by the year 2100 and Africa is identified as the most vulnerable region (Idris et al., 2022). Irregular and inadequate rainfall has serious and detrimental effects on crop production and crop quality, especially in tropical regions where most of the world’s water is used for agriculture (Reynolds and Ortiz, 2010; Kebede et al., 2020). The impact of drought varies across time and space, from the local level to the regional level and then to the global level (Mishra et al., 2015). According to Cavicchioli et al. (2019); Toulotte et al. (2022), rising temperatures and drought significantly impact crop growth. Forecasts suggest that in areas affected by drought, the yields of major crops could decrease by over 50% by 2050 and nearly 90% by 2100 (Li et al., 2009). Multiple separate studies have highlighted the impact of elevated temperatures and water stress on agricultural yields. For instance, in Canada, severe events that occurred in 2001 and 2002, along with droughts and floods in 2010 and 2011, significantly devastated crop yields, resulting in reductions of up to 50% (Yang et al., 2020). Additionally, from 1980 to 2016, substantial disasters in the United States, each surpassing a billion dollars annually, underscore the significant agricultural losses exceeding $220 billion stemming from the combined effects of drought and heat (Lamaoui et al., 2018). Among various abiotic stresses, drought is considered as the most detrimental since it poses a major challenge to sustainable food production, as it can reduce the potential yield of different crops by up to 70% (Gosal et al., 2009). Drought can exert a significant toll on both human well-being and agricultural activities. They are estimated to impact around 55 million individuals annually worldwide, representing a major menace to livestock and crops on a global scale (Francini and Sebastiani, 2019; Elakhdar et al., 2022). Molénat et al. (2023) assert that climate change amplifies the challenges confronting Mediterranean agriculture, particularly in maintaining or improving production levels to meet the growing demands of an expanding population. The Mediterranean diet, comprising representative crops such as olives, grapes, fruits, cereals, and legumes (used as an alternative to animal protein), enjoys global recognition (Cramer et al., 2020). The agricultural sector in the Mediterranean region employs a significant workforce and holds economic importance. For example, in the Maghreb, agriculture engages 13–20% of the workforce and contributes 10–20% to the region's gross domestic product (Cramer et al., 2020). In developing nations, the impact of heat and drought is of paramount significance. In Morocco, where agriculture plays a substantial role in the economy, involving around 40% of the workforce, drought significantly reduces crop productivity. This directly affects farmers' livelihoods and has subsequent repercussions on the overall economy. Reports from West Africa indicate crop failures due to drought, leading to a 25% decrease in per capita food production (Gbegbelegbe et al., 2024). In the Mediterranean region, agriculture is the main user of water. For example, in countries of the Eastern Mediterranean, crop irrigation accounts for up to 79% of total water withdrawals (Hellal et al., 2019). This challenge coincides with the need to protect essential natural resources like water, soil, and biodiversity, which remain susceptible to both climate-related dangers and anthropogenic activities. The effective management of the limited water supply in a warmer and drier climate stands out as one of the most critical concerns confronting Mediterranean agriculture. Climate projections and crop modeling indicate that water scarcity and heat waves significantly restrict and will continue to limit crop production (Francini and Sebastiani, 2019; Molénat et al., 2023). Consequently, the viability of the Mediterranean food system hinges on the ability to sustain Mediterranean agriculture amidst water scarcity.
3 Barley and water stress
Barley (Hordeum vulgare L.) is the 13th most produced crop in the world and 4th in harvested area, with 1570000 Tons and 51.6 million ha, respectively, it stands as a highly important cereal crop cultivated across regions spanning Europe, the Middle East, North and South Africa, as well as Asia (Elakhdar et al., 2022; Talaat, 2023). In global production, Europe holds a share of 60% with 46% of the total global area dedicated to barley cultivation (Martínez-López et al., 2022). Spain leads in barley cultivation, producing approximately 11000 Tons across 2.75 million hectares, of which 0.36 million hectares are under irrigation (Martínez-López et al., 2022). Barley is a versatile crop with substantial economic impact, serving as a staple food for both humans and animals, as well as in industrial sectors such as brewing (Elakhdar et al., 2022; Toulotte et al., 2022). Drought is characterized as an extended duration of insufficient rainfall, leading to the depletion of soil moisture through transpiration or evaporation, thereby rendering it insufficient to meet the demands of crops (Lamaoui et al., 2018; Francini and Sebastiani, 2019; Toulotte et al., 2022). Several studies conducted globally have demonstrated that water shortage triggered multiple modifications in barley (Hasanuzzaman et al., 2019; Tabatabaei and Ansari, 2020; Talaat, 2023). Drought has been shown to reduce grain yield in barley, by 49–87% (Samarah, 2005; Kebede et al., 2020). Under such circumstances, it becomes increasingly important for farmers and agronomists to focus on enhancing barley's resilience to drought stress, all the while striving to maintain or even improve productivity in challenging climatic conditions.
4 Effect of drought stress on crucial barley plant processes
4.1 Seed germination
Seed germination is a crucial process for the growth, development, and successful establishment of a plant. It begins with the uptake of water, which activates the essential metabolic processes in the seed. This water uptake is also necessary for the activation of hydrolytic enzymes, which are responsible for the degradation of starch, solubilization, and transport of carbohydrates. However, the scarcity of soil moisture can significantly impact the successful germination and establishment of plants (Wijewardana et al., 2019). This is because of the reduced water uptake rate through the seed coat under stress conditions that can lead to a decrease in the percentage of seed germination (Reza Yousefi et al., 2020). Additionally, germinated seedlings in a moisture-deficit environment show a reduction in both seedling vigor and germination index (Sazegari et al., 2020; Tang et al., 2023). The average time required for seed germination is also extended under soil moisture deficit stress (Queiroz et al., 2019). Furthermore, the fresh weight as well as the dry weight of germinated seedlings decline in a moisture-deficit environment. This reduction in seed hydration can slow down the process of hydrolysing stored carbohydrate material, leading to a lesser and slower supply of hydrolysed material to the embryo axis. This, in turn, can affect the emergence of the radicle from the seed coat, leading to a delay in seed emergence and a reduction in seedling vigour (Channaoui et al., 2019).
4.2 Water & nutrients uptake and transport
In times of soil water deficit, the uptake capacity of roots becomes limited due to the reduction in the water potential gradient between the soil and the plant. This is because the root hydraulic conductivity decreases under drought conditions, which in turn limits the water uptake capacity of roots from the soil (Zhu et al., 2024). The expression of genes coding for aquaporin, which controls the root hydraulic conductivity, is generally downregulated under moisture deficit conditions. This downregulation ultimately lowers the root water uptake capacity (Mukarram et al., 2021). Moreover, under stress, the continuous loss of transpiration and lower soil moisture lead to an increase in xylem cavitation. This increase gradually reduces the hydraulic conductance in plants and blocks the movement of water within the plant. This further exacerbates the water stress conditions (Zhu et al., 2024).
In plants, nutrient uptake is a complex process that involves the absorption of nutrients from the soil through water. Water serves as a medium for nutrients to move within the soil matrix and from the soil to the plants. However, soil moisture deficit conditions can significantly decrease the nutrient uptake from the soil. This reduction in nutrient uptake may be attributed to a decrease in the nutrient supply through mineralization, as well as a reduction in the diffusion and mass flow of nutrients in the soil (Duman, 2006; Jorenush and Rajabi, 2015). The kinetics of nutrient uptake by the roots are also affected, reducing the rate of nutrient uptake under drought stress. This is further compounded by the reduced translocation of nutrients from the root to the shoot, which contributes to a reduction in the nutrient status of different plant parts (El-Denary and El-Shawy, 2014). In addition, the microbial growth in the rhizosphere is also affected under deficit soil moisture conditions, ultimately affecting the nutrient uptake by the roots (Jalal et al., 2014). To cope with drought, plants upregulate the expression of different types of transporter proteins to balance the nutrient uptake under stress conditions. Genes encoding for potassium transporter proteins are also upregulated under drought stress (Maleki Farahani et al., 2010; Abdel-Ghani et al., 2015). Bista et al. (2018) reported that the reductions in P uptake under drought conditions were correlated with decreases in P-uptake protein concentration and activity. However, reductions in nitrogen (N) uptake were only inversely related to N-uptake protein levels. Due to greater decreases in total protein per gram, the concentration of nutrient-uptake proteins per gram significantly declined, despite increases in total protein per gram. Therefore, it is plausible that decreases in the concentration of root nutrient-uptake proteins in both drought-tolerant and sensitive species contributed to at least some of the drought-related declines in nutrient concentration, particularly in %P. In conclusion, while drought stress can negatively affect nutrient uptake from the soil, plants also develop strategies to challenge stressful conditions through the synthesis of a greater number of transport proteins to fulfill their nutrient requirements.
4.3 Physiological responses
4.3.1 Growth
Severe stress conditions significantly impact various aspects of germination, seed vigour, and seedling characteristics in different cereals genotypes (Dhanda et al., 2004). This stress, characterized by rough conditions, leads to delays, reductions, or inhibitions in the germination process and the overall vigour of cereal seeds (Ghanifathi et al., 2011; Tabatabaei, 2013). Additionally, it influences the Germination Stress Tolerance Index (GSTI) (Moayedi et al., 2009; Barati et al., 2015). Under water-deficit conditions, the seed vigour index emerges as the most susceptible trait, followed by root and shoot length, and germination percentage (Moayedi et al., 2009). The repercussions of drought stress extend to the reduction of cell membrane stability, changes in relative water content (Geravandi et al., 2011), early maturity, diminished leaf area (Tabatabaei and Ansari, 2020), decreased dry weight, and alterations in the root–shoot ratio (Shi et al., 2014). Furthermore, the overall response of the whole plant, including the transpiration rate, to elevated atmospheric vapor pressure deficit is linked to drought tolerance in cereals (Schoppach et al., 2017).
4.3.2 Root and shoot characteristics
Morphological characteristics of barley affected by water deficiency encompass various leaf features, including shape, area, expansion, size, waxiness, pubescence, senescence, and cuticle tolerance. Additionally, root traits such as length, density, fresh and dry weight are influenced by water deficit (Hasanuzzaman et al., 2020). Assessing leaf water potential proves to be an effective and dependable method for gauging plants' response to water scarcity. Under water-limiting conditions, the gradual reduction in electron transport of photosystem II occurs, leading to an increase in non-photochemical quenching capacity. Consequently, this results in a decline in cereal leaf relative moisture content (Jouyban et al., 2015). Drought conditions contribute to a decrease in leaf water potential in barley plant, attributed to solute accumulation. However, there may be genotypic variations in the response to water potential, both under well-watered and drought conditions (Istanbuli et al., 2020). Leaf water potential also impacts various gas exchange characteristics, including stomatal conductance, net-photosynthetic rate, and transpiration (Hasanuzzaman et al., 2019) (Figure 1).
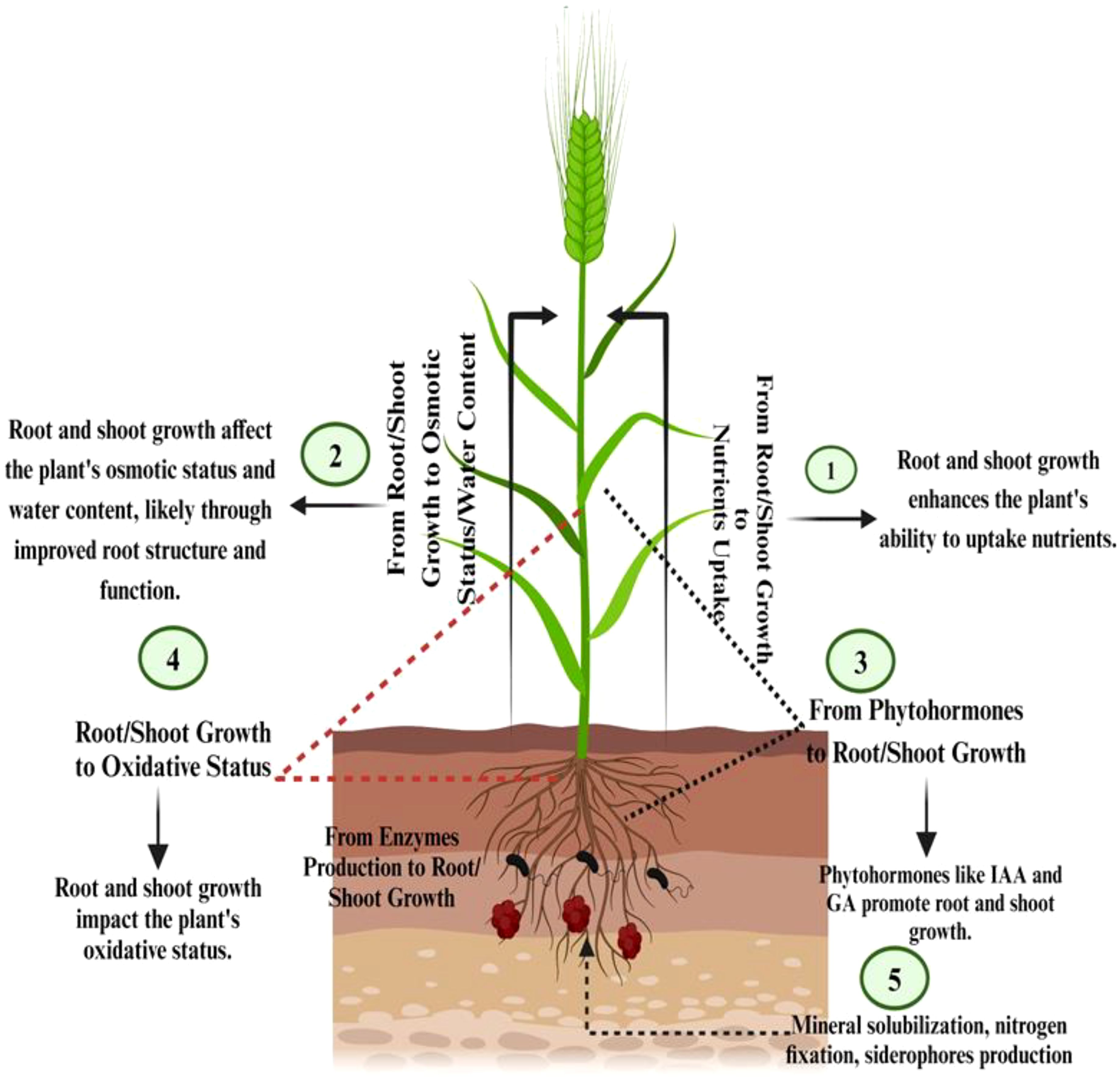
Figure 1. Mechanisms of barley drought tolerance: interaction between root/shoot growth, nutrient uptake, and physiological processes.
4.3.3 Water status
Relative water content (RWC) serves as a crucial indicator of water status in barley experiencing water deficit, in contrast to leaf water potential (Sallam et al., 2019). This parameter has proven instrumental in selecting drought-tolerant barley cultivars (Teulat et al., 2003). Notably, in cereals, the negative impact of drought intensifies when applied later in plant growth (beyond 6 weeks post-emergence), leading to more detrimental effects on water relations, nutrient uptake, growth, and yield, compared to early drought imposition (after 3 weeks of seedling emergence) (Ghotbi-Ravandi et al., 2014). Chlorophyll content, membrane stability, and RWC of barley cultivars during the flowering stage are significantly reduced under drought stress (Hebbache et al., 2021). A study found that drought stress caused a substantial 43% reduction in relative water content (from 88% to 45%) in four genotypes of wheat plant (Siddiqui et al., 2021). This reduction in RWC leads to stomatal closure, subsequently diminishing the rate of photosynthesis. Water scarcity hampers osmotic regulation, but alternating between drying and re-watering induces osmotic regulation, improving the plant's water use efficiency during drought conditions. A tolerance strategy to water deficit involves maintaining high relative moisture content, attributed to increased osmotic regulation (Alghabari and Ihsan, 2018). Drought-tolerant genotypes demonstrate the ability to sustain high turgor potential and relative water content, indicating minimal impact on their protoplasmic structure compared to sensitive genotypes. This correlation between RWC and photosynthetic rate underscores the importance of maintaining leaf turgor as an adaptive mechanism crucial for stomatal regulation and photosynthetic activities under water-deficit conditions (Elakhdar et al., 2022).
4.3.4 Osmotic status
Osmoregulation is the active process of lowering osmotic potential in plant cells through the accumulation of osmotically active compounds (Sallam et al., 2019). It is an indispensable and effective component of plant resistance to drought, in particular because it maintains the turgor pressure associated with a large number of metabolic and physiological processes for which the presence of cell turgor is crucial (Zivcak et al., 2016).
Plants employ various strategies to maintain their water balance when faced with drought. Three methods of osmotic regulation exist, intracellular water reduction, wherein plants decrease the amount of water inside their cells to reduce osmotic potential and conserve the free energy of water, cell volume reduction, where cells reduce in size to maintain osmotic pressure, enabling plants to better resist water stress, and increase in cell content, whereby plants elevate the concentration of solutes inside their cells (Sallam et al., 2019; Ahluwalia et al., 2021). This lowers osmotic potential and facilitates water uptake, even when external water potential is low (Wang et al., 2000). While these mechanisms coexist in plants, not all possess the same capacity for osmotic regulation (Osakabe et al., 2014) (Figure 1).
Ultimately, osmotic regulation is necessary to maintain the required turgor pressure for cell growth, even in water-stressed periods (Sanders and Arndt, 2012; Osakabe et al., 2014). Osmotic regulation is an important mechanism that helps maintain stomatal conductance and turgor pressure in plants when there is a moderate water deficit. This mechanism is crucial for keeping a high concentration of CO2 in the intercellular space of the mesophyll, which prevents or reduces the inhibition of photosynthesis (Osakabe et al., 2014). Osmotic regulation also plays a vital role in protecting various cellular processes like cell growth, stomatal opening, and photosynthesis under environmental stress (Sallam et al., 2019).
Certain compounds, such as amino acids, amino acid derivatives, trehalose, fructan, and mannitol, act as osmotic regulators and help control the osmotic pressure in the cytoplasm (Wang et al., 2000). These substances have low molecular weight, high solubility, and low toxicity, making them effective in maintaining normal osmotic pressure and preserving the activity of proteins and the integrity of the cell membrane structure (Sanders and Arndt, 2012; Osakabe et al., 2014; Sallam et al., 2019). Currently, a large number of studies are focusing on osmotic regulating substances such as proline, soluble sugars, glycine betaine, among others. Research has shown that proline accumulation represents a protective strategy adopted by plants to cope with drought stress (Yang et al., 2021).
4.3.5 Photosynthesis efficiency
One essential metabolic function greatly impacted by drought is photosynthesis. In response to water scarcity, roots produce abscisic acid (ABA), causing stomatal closure, which reduces gas exchange and inhibits photosynthesis (Sallam et al., 2019; Ahluwalia et al., 2021; Qiao et al., 2024). Drought affects various subcellular regions and organelles, slowing overall plant growth, preventing seed germination, and hindering cell elongation (Fadiji et al., 2022). Drought-stressed plants are shorter and have fewer leaves, which limits their ability to absorb photosynthetically active radiation (PAR) and consequently restricts both photosynthesis and yield (Fadiji et al., 2022). Additionally, the lack of moisture in the soil increases salt concentration, which lowers the water potential of plant cells and impedes growth (Mondini and Pagnotta, 2015; Mahboob et al., 2023; Nazim et al., 2024). As a result, drought disrupts the mass movement of essential water-soluble minerals, such as calcium, magnesium, and nitrate, all vital for plant development (ElSayed et al., 2022; Qiao et al., 2024. Ahluwalia et al., 2021). These changes highlight the critical importance of water availability for overall plant functioning and growth (Zargar et al., 2017; Fadiji et al., 2022; Benito-Verdugo et al., 2023; Iqbal et al., 2023; Kumar et al., 2024; Qiao et al., 2024).
Furthermore, stomatal closure decreases stomatal conductance, leading to increased photorespiration and further restricting photosynthesis (Fadiji et al., 2022). This inhibition likely results in plants absorbing more light energy than they can effectively utilize for carbon fixation (Jedmowski et al., 2013; Ghadirnezhad Shiade et al., 2023; Luqman et al., 2023). Key components limiting photosynthesis include reduced CO2 diffusion due to early stomatal closure, decreased activity of photosynthetic enzymes, biochemical changes related to triose-phosphate formation, and reduced photochemical efficiency of photosystem II (Ghadirnezhad Shiade et al., 2023; Li and Wang, 2023; Qiao et al., 2024). The imbalance between light capture and its utilization leads to the accumulation of reactive oxygen species (ROS) in the chloroplast and, subsequently, the disorganization of thylakoid membranes, decrease in Rubisco activity, loss of chloroplast membranes, degradation of chloroplast structure and photosynthetic apparatus, chlorophyll photo-oxidation, destruction of chlorophyll substrate, inhibition of chlorophyll biosynthesis, and the increase of chlorophyllase activity (Kirkby et al., 2023; Rodrigues et al., 2024; Sharma et al., 2024).
While reduced stomatal conductance minimizes water loss through leaves (Li and Wang, 2023; Qiao et al., 2024), it simultaneously limits photosynthesis by decreasing intercellular CO2 levels (Benito-Verdugo et al., 2023; Ghadirnezhad Shiade et al., 2023; Jahan et al., 2023). Consequently, these interconnected disturbances lead to reduced water use efficiency (WUE) in plants (Sallam et al., 2019; Kirkby et al., 2023; Li and Wang, 2023; Mahboob et al., 2023; Kumar et al., 2024; Qiao et al., 2024).
4.3.6 Oxidative status
The production of reactive oxygen species (ROS) in response to stress represents the primary reaction of plant cells to various environmental stresses. These ROS cause cellular damage by altering proteins, inactivating enzymes, disrupting gene expression and altering membranes (Amirjani and Mahdiyeh, 2013). In parallel, changes in ROS generation act as signals, influencing gene transcription and thus contributing to plant adaptation to abiotic stresses (Rajasekar et al., 2015). The key members of the ROS family, such as singlet oxygen (O), superoxide radicals (O2), hydrogen peroxide (H2O2) and hydroxyl radical (OH), are responsible for oxidative damage in plants (Sallam et al., 2019). Antioxidant enzymes regulate ROS at low levels under normal conditions, ensuring stability (Hasanuzzaman et al., 2020). However, a decrease in antioxidants or an increase in ROS production can upset this balance, resulting in oxidative stress. The latter results in damage to macromolecules and cell membranes, as well as increased lipid peroxidation (Ansari et al., 2019). Drought induces increased ROS production, leading to a rise in malondialdehyde (MDA) levels (Istanbuli et al., 2020). This compound is considered a reliable indicator of oxidative damage, particularly as a marker of membrane lipid peroxidation (Pakzad et al., 2019). Reduced membrane stability thus reflects the level of ROS-induced lipid peroxidation (Murtaza et al., 2016). Low MDA levels are linked to drought stress tolerance in cereals (Zhang et al., 2011).
The main ROS production sites in plants are located in the chloroplast, mitochondria and peroxisomes, while the endoplasmic reticulum, cell membrane, cell wall and apoplast act as secondary production centers (Sallam et al., 2019). To protect themselves against the damaging effects of ROS, plants have developed an effective antioxidant system, consisting of both enzymatic antioxidants, such as superoxide dismutase (SOD), catalase (CAT), ascorbate peroxidase (APX), guaiacol peroxidase (GPX), glutathione reductase (GR), mono dehydro-ascorbate reductase (MDHAR) and dehydro-ascorbate reductase (DHAR), as well as non-enzymatic antioxidants, including ascorbic acid, reduced glutathione (GSH), α-tocopherol, carotenoids, flavonoids and proline. These two antioxidant systems work in tandem to neutralize ROS (Das and Roychoudhury, 2014).
4.4 Agronomic traits
Drought stress hurts flowering and grain development processes during the reproductive stage, which reduces spikelet fertility, results in poor grain filling, and reduces grain weight (Thabet et al., 2020). Consequently, compared to non-stressed plants, barley plants in drought conditions frequently display decreased grain production, smaller grain size, and a poorer harvest index (Samarah et al., 2009; Khalili et al., 2016). The impact of drought is not solely determined by its duration but also by the timing of water scarcity (Dietz et al., 2021). When drought occurs during the germination stage, it results in significant losses in plant density (Farooq et al., 2012). Conversely, water shortage during the vegetative stage leads to reductions in biomass production, particularly affecting leaf area, especially the flag leaf (Biswal and Kohli, 2013; Tian et al., 2022), which in turn affects yield production. This relationship is supported by the strong association between leaf area and grain yield (Jabbari et al., 2019). Water scarcity during the flowering stage (anthesis) has a detrimental effect on fertility, leading to a reduction in the number of spikes per plant (Jamieson et al., 1995; Dietz et al., 2021). Post-anthesis drought impacts grain filling by diminishing seed weight, thereby reducing grain yield due to decreased carbohydrate reserves. Consequently, these effects extend to the subsequent generation (Dietz et al., 2021).
Drought stress can also change the protein content, starch composition, and malting properties of barley grains (Mahalingam, 2017), which can have an impact on the food and beverage sectors. Overall, the impact of drought stress on barley agronomic traits highlights the necessity of all-encompassing approaches, such as the creation of drought-resistant cultivars and the application of water-saving agronomic techniques, to improve drought tolerance and reduce yield losses in barley production.
5 Methods for mitigation of drought stress in barley plants
5.1 Selection of drought-tolerant genotypes
5.1.1 methods adopted for genotypes selection
Drought tolerance might be approached differently, making it very complicated. Successful plant breeding requires effective assays to select the drought-tolerant genotypes (Sallam et al., 2019). In literature, there are many methods for selecting barley drought-tolerant genotypes, each method is based on various traits and can be adopted in different growth phenological stages (Table 1). Many screening studies were effected at the germination stage by estimating the germination rate and speed (Barati et al., 2015; Hellal et al., 2018; Adriana, 2020). Drought stress decreased germination percentage and speed, with a higher effect on germination speed than germination percentage (Hellal et al., 2018). Autumn rainfall water deficit can slow down and inhibit germination, which affects yield production (Adriana, 2020). Based on this problematic, breeders think that the selection of barley genotypes that can preserve a high germination rate and speed under water shortage conditions might guarantee good barley plant growth in various environmental conditions (Khafagy et al., 2017).
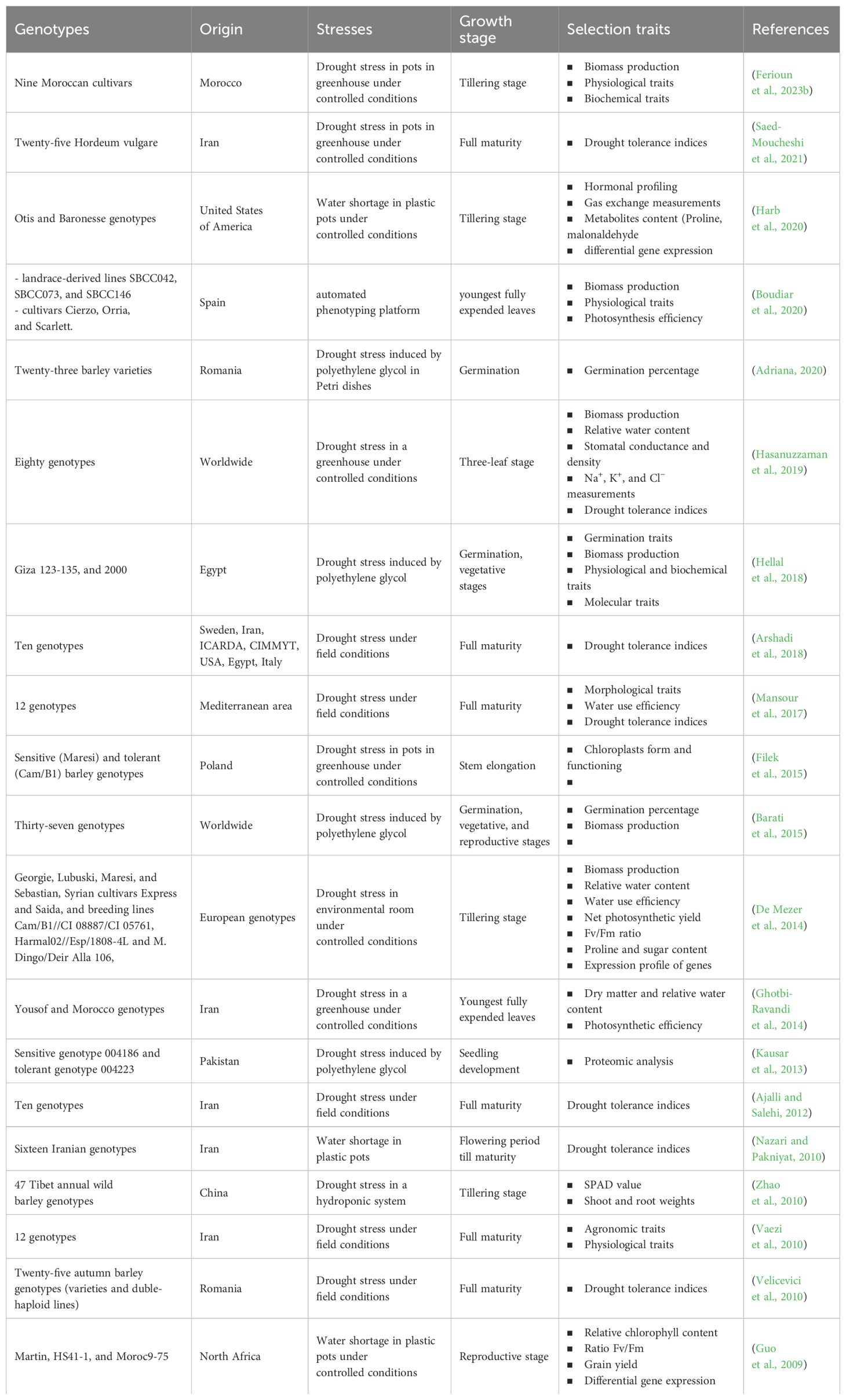
Table 1. Studies performed for selecting barley drought genotypes based on various physiological and agro-morphological traits.
The selection might be also in the vegetative stage, based on various traits. Selecting drought-tolerant cultivars in this stage is based on morphology, biomass production, physiology, and biochemistry (Figure 2).
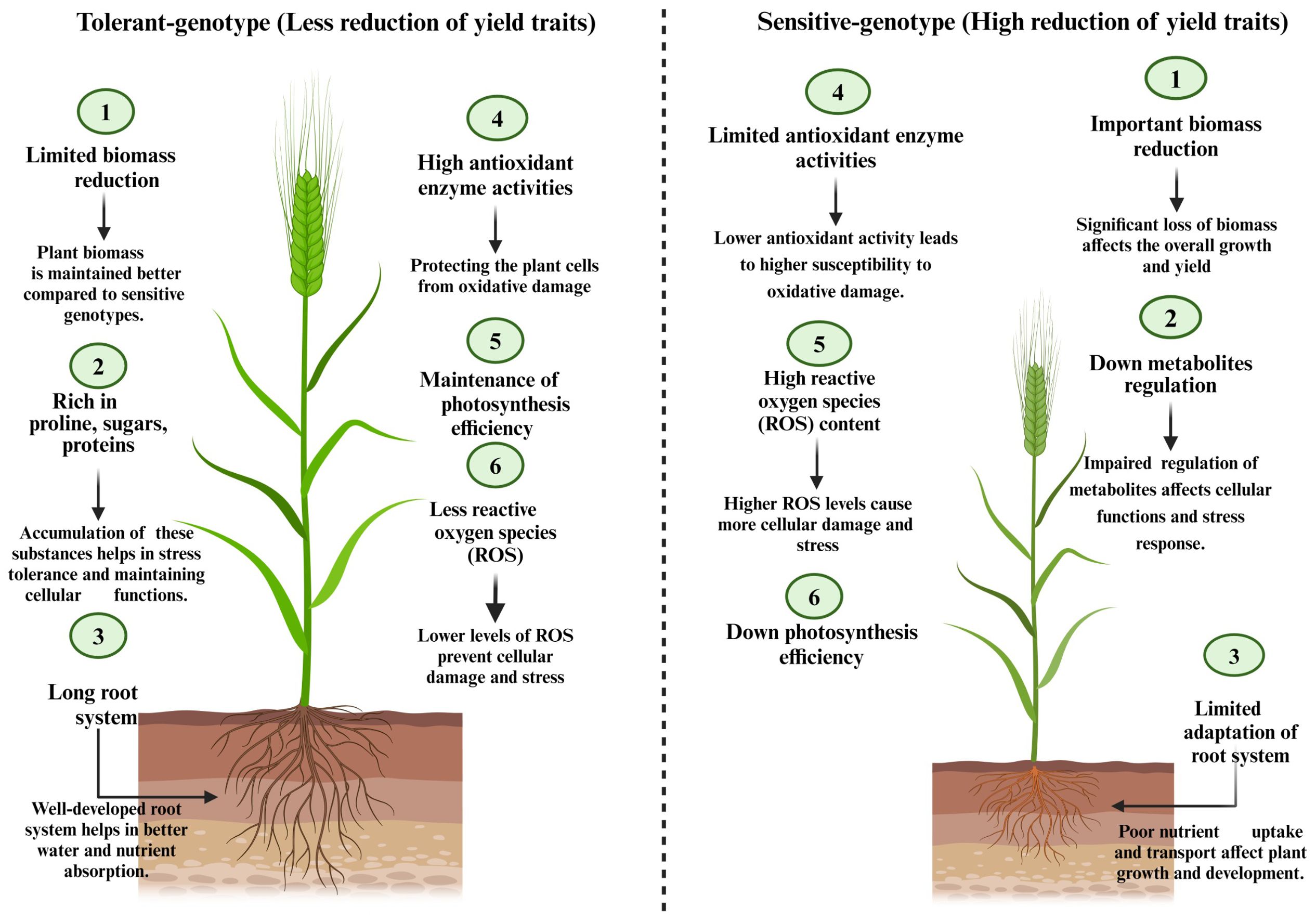
Figure 2. Essential physiological and biochemical differences between tolerant and sensitive barley genotypes.
i). Plant height, root length, root ramification, number of tillers per plant, number of leaves per plant, internode length, flag leaf area, and number of main stem nodes are among the morphological traits frequently investigated in barley plants (Jabbari et al., 2018). In barley as in other cereals, several research studies reported a significant association of these traits to the grain yield trait (Boussakouran et al., 2019), which makes morphological traits effective for barley breeding programs in various growth phenological stages. Here, under drought stress, genotypes that showed less morphology affection are considered more tolerant.
ii). Evaluating the functioning of certain physiological and biochemical traits is widely adopted in breeding studies for the selection of drought tolerant genotypes (Sallam et al., 2019). In this context, the traits evaluated are numerous. In relation with water relations, the relative water content (RWC) index is the most measured trait that reflect the ability of plant to keep cell turgor under stressful condition (Lugojan and Ciulca, 2011), in numerous studies, under drought condition, the trait correlated negatively with grain yield (Samarah, 2005; Gonzalez et al., 2010; Aboughadareh et al., 2013). Genotypes that less reduced RWC traits are considered more drought tolerant (Sallam et al., 2019). Water loss rate shows the balance between transpiration rate and the amount of water supplied to the leaf. Drought tolerant genotypes shows less water loss rate reduction when compared to the sensitive ones (Izanloo et al., 2008). It is widely recorded that drought stress induces strong reduction in plant macro and micro-nutrient uptake (Noman et al., 2018), which affect plant growth and leads to reduces yield production (Sardans et al., 2008). Based on this fundings, many barley breeding studies includes some nutrients uptake in the traits studied (Ayad et al., 2010; Ahmed et al., 2013), the genotypes showed less nutrient uptake reduction under drought stress were considered more tolerant. Every genotypes selection study based on physiological and biochemical traits must investigate the oxidative statue in plant cells (Sallam et al., 2019), which is released by the quantification of reactive oxygen species (ROS) and antioxidants enzyme activities. High ROS content such as H2O2, superoxide radicals (O2), and hydroxyl radicals (OH), resulting stressful environment affects the cellular redox potential which leads to the oxidation of proteins, nucleic acids, chlorophyll pigment, and many other compounds (Hasanuzzaman et al., 2018, 2020), which alters the function of various apparatus and affects plants productivity. When compared to the sensitive ones, drought-tolerant genotypes showed less ROS content increase under drought stress coupled with important increase in antioxidant enzyme activities such as catalase, peroxidases, superoxide dismutase, and reductases (Guan and Scandalios, 2000). The tolerant genotypes showed high ability to increase the activity of antioxidant enzymes in stressful conditions (Guan and Scandalios, 2000). However, this ability is less recorded in sensitive genotypes. ROS accumulation in plant cells induces the peroxidation of membrane lipids (Sharma et al., 2017), which can investigated by measuring malonaldehyde (MDA) content. Low MDA content is widely linked to the stress tolerance (Ferioun et al., 2023a). Under stressful conditions, plants accumulate organic and inorganic osmolytes in cell cytosol such as sugars, proteins, amino-acids, and polyols as a drought tolerance mechanism (Loutfy et al., 2012). Solutes accumulated play many roles especially in osmo-protection, osmotic regulation, ROS neutralization, and macro-molecules protection (Sallam et al., 2019). Under drought stress, proline accumulation in barley leaves is widely reported (Hellal et al., 2018; Ferioun et al., 2023a, b), the roles attributed to this amino-acid are numerous, the most recorded are in relation with osmo-regulation, scavenging free radicals, membranes steadiness (Marcińska et al., 2013). It is important to sign that very limited species and genotypes can accumulates enough proline under stressful conditions (Zali and Ehsanzadeh, 2018) which make this criteria very important to consider it in barley breeding studies. Given the effect of drought stress on photosynthesis traits in barley plants, the evaluation of photosynthesis apparatus becomes highly important in drought-tolerant genotypes selection. Photosynthesis efficiency might be investigated adopting various parameters such as measuring leaves chlorophyll content, photosystem II efficiency (chlorophyll fluorescence), CO2 incorporation, and stomatal conductance. Genotypes that can preserve high scores in photosynthesis indices under drought stress might be considered as an important choice for barley breeding program.
At full maturity stage, the evaluation of drought tolerance might be carried out based on agronomic traits such as grain yield (GY), thousand grain weight (TGW), number of grains per spike, spike number per plant, grains per square meter… (Kadam et al., 2014). Furthermore, many breeders use drought tolerance indices based on grain yield under non-stressful and stressful conditions such as susceptibility index (SSI) for the fraction of genotypic productivity (Fischer and Maurer, 1978), mean productivity index (MP) (Mohammadi, 2020), The tolerance index (TOL) (McCaig and Clarke, 1982), stress tolerance index (STI) (G. C. J. Fernandez, 1992), Harmonic mean productivity (HMP) (Jafari et al., 2009), and geometric mean productivity (GMP) (G. C. J. Fernandez, 1992). In this context, many studies in many countries have been carried out to describe the suitable indices for selecting barley drought- tolerant genotypes. The most cited indices were STI, MP, and GMP (Nazari and Pakniyat, 2010; Ajalli and Salehi, 2012; Arshadi et al., 2016; Saed-Moucheshi et al., 2021).
5.1.2 Genetic variation between drought-tolerant and drought-sensitive barley genotypes in response to drought stress
Barley genome sequencing has emerged in recent years, making it easier to understand the molecular mechanisms. Scanning the genetic patterns involved in drought tolerance in barley (Hordeum vulgare L.) will facilitate the control of molecular mechanisms of drought tolerance (Karunarathne et al., 2023). Up to date, several studies have been executed in this area, and have revealed different genes and motifs associated with drought resistance/tolerance in barley. Xiong et al. (2023) have compared the drought sensitivity/tolerance of 100 barley genotypes at the seedling period. Those genomes from International Barley Core Selected Collection (BCS), (BCS24 for tolerant genotypes and BCS8 for sensitive ones) have been treated under two planting conditions. Using Genome-Wide Association Study (GWAS) and RNA-Seq methods, this study led to the identification of numerous drought tolerance controlling markers. In this study, 20 SNPs and 41 candidate genes located on chromosomes 2, 5, and 6 have been identified using GWAS. In addition, RNA-seq analysis has confirmed the results of GWAS and revealed more differentially expressed genes in BCS8 and BCS24 (Zhao et al., 2010; Xiong et al., 2023).
Feng et al. (2020); Fu et al. (2022). Gao et al. (2022), and Pan et al. (2022) have revealed new drought-protecting genes, more than 60 genes involved in plant protection against drought stress have been made known thanks to the abovementioned studies. Another study was conducted on a collection of 218 worldwide spring barley genomes, 108 from Europe, 45 from West Asia and North Africa, 36 from East Asia, and 29 from the Americas, this study identified 26 genomic regions localized on chromosomes 1, 2, and 5 considered as the precursors of several candidate genes involved in drought tolerance. Among the 26 revealed genes, 9 adaptive genes are drought-specific or control-specific, and 17 constitutive genes are involved in the genetic variation of the traits studied under both control and induced drought. On chromosome 1, no control-specific genes were identified, while four of them are drought-specific and the remaining 9 are constitutive. The genes located on chromosome 2 are 8 adaptive and 3 constitutive, whereas those identified on chromosome 5 are strictly constitutive. Putative candidate genes in this study, especially those that are drought-specific, are poised to be used for a wider application in crop molecular breeding (Thabet et al., 2018). The majority of executed studies in this area have identified several common drought-controlling genes in barley (Table 2), some of those genes have been promisingly used in numerous editing studies trying to improve barley resistance against drought stress (Karunarathne et al., 2023).
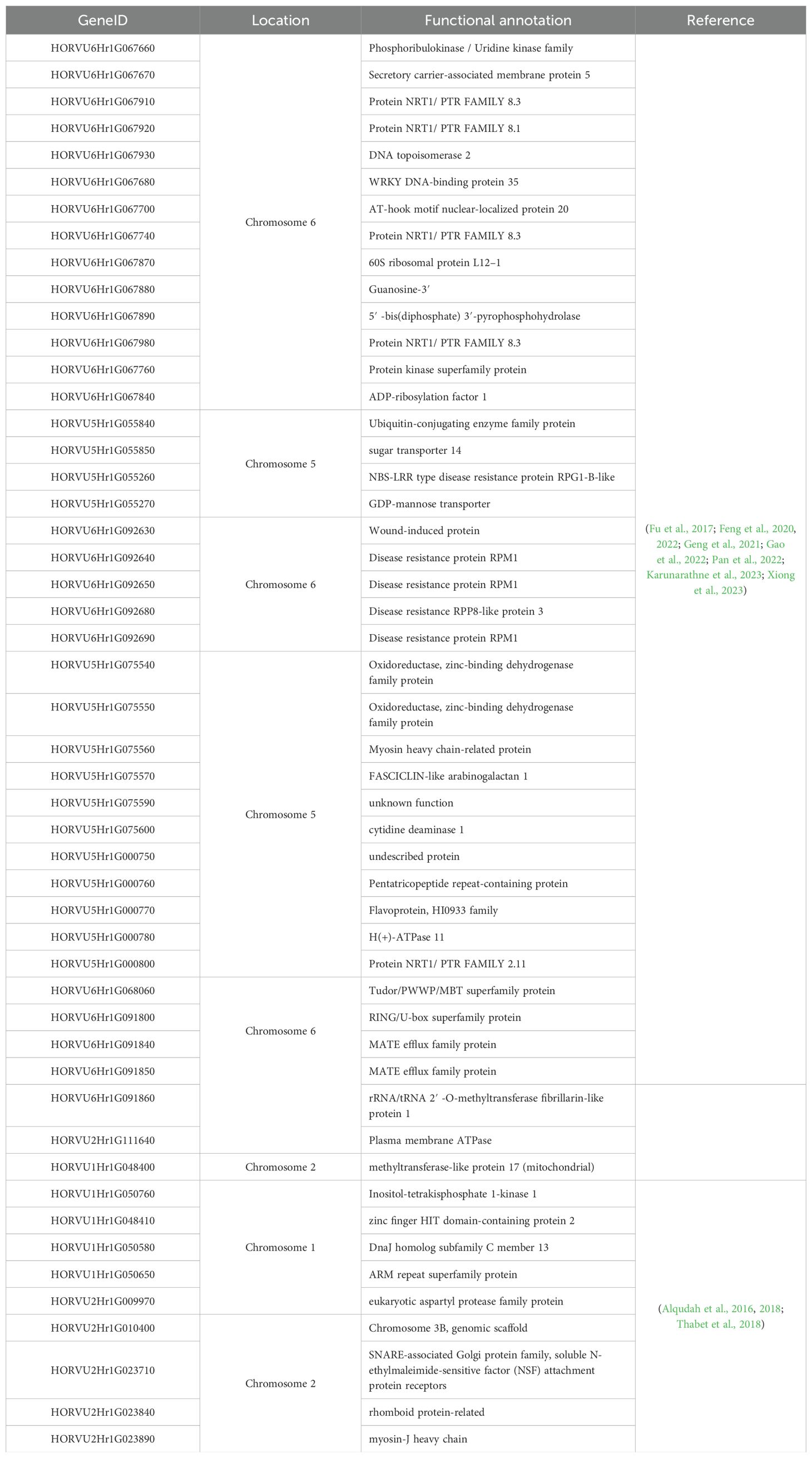
Table 2. Important genes involved in barley drought tolerance, their location, and functional annotation.
5.2 Bioinoculants
Microbial inoculants might be constructed using live microorganisms such as bacteria, fungi, and/or algae with important abilities of bio-stimulation and/or bio-protection (Lima et al., 2020; Nayana et al., 2023; Sagar et al., 2024). The application of these biofertilizers might be in the plant rhizosphere, through seed emergence in a biofertilizer solution, or by application to the plant surface (Bittencourt et al., 2023). Many species of these microbes have shown an important ability to improve plants tolerance to drought stress (Asghari et al., 2020; Ilyas et al., 2020; Hamid et al., 2021; Ashry et al., 2022; Mawar et al., 2023), which might be attributed to: (i) enhancing the content of assimilable nutrients via mineral solubilization, N2 fixation, organic compounds decomposition, and siderophores production, (ii) the secretion of hormones such as auxins and gibberellins, which ameliorate morphological and physiological status in plants and (iii) secretion of extracellular polymeric substances that helps retain moisture (Ilyas et al., 2020) and their role plant photosynthesis (Uarrota et al., 2022).
The attempts to commercialize bio-inoculants began for the first time in the twentieth century based on nodulating and non-nodulating bacteria and algae (Bittencourt et al., 2023). Ever since, the global adoption of bio-inoculants by farmers has been increasing annually (Santos et al., 2019; Kapadia et al., 2021). Gradually, several studies have described bio-inoculants formulated based on various microbes, demonstrating a remarkable enhancement in the growth of various plants under diverse environmental conditions (Etesami and Maheshwari, 2018; Rebi et al., 2022). In cereals, Rebelo Romão et al. (2022) reported the use of maize seeds encapsulated with xerotolerant microorganisms for the protection of maize plants against water shortage conditions. Kaboosi et al. (2023) reported the ability of Serendipita indica to enhance drought tolerance in maize. Bangash et al. (2021) reported the formulation of biofertilizer for improving wheat yield and growth in rainfall farming system.
The studies on bio-inoculants encompass not only the investigation of microbes but also the refinement of formulation techniques. Bio-inoculants formulation involves developing a homogenous combination of a chosen beneficial strain and an appropriate carrier that can stabilize and protect the strain throughout storage and transportation. Effectiveness, non-polluting nature, ease of biodegradation, high water retention capacity, and sufficient shelf life are all desirable qualities in a bioformulation. In literature, several types of formulation are recorded such as: solid, liquid, and polymeric formulations (Chaudhary et al., 2020).
5.3 Intercropping
Intercropping, also known as polyculture, refers to the simultaneous cultivation of two or more distinct crop species within a single field throughout a growing season (Elouattassi et al., 2023). It is widely embraced globally as a significant sustainable approach due to its beneficial impact on crop productivity, yield stability, as well as enhanced efficiency in utilizing nutrients and water resources (Fan et al., 2020; Hussain et al., 2023). The benefit of effective water use in intercropping arises due to variations in water needs among intercrops across spatiotemporal differences (Dong et al., 2018). Optimal irrigation amount and timing are crucial in aligning crop water needs with water availability, thereby enhancing the compatibility between the intercropped plants (Yin et al., 2020). Woldeamlak et al. (2006) conducted a drought experiment and discovered that the yields of wheat and barley mixtures remained notably consistent between the drought treatments and controls. These mixtures exhibited higher yields under both seedling and heading stage drought stress conditions. The authors hypothesized that this increased productivity in mixtures might be attributed to the later maturation of wheat, enabling it to utilize soil moisture more efficiently after the barley had matured, leading to a more comprehensive utilization of available moisture compared to the individual crop components. According to Yin et al. (2017), intercropping proves advantageous in elevating photosynthetic resources, such as leaf area index (LAI) and leaf area duration (LAD), while facilitating the transfer of photosynthetic substances from vegetative organs to grains. This process contributes to improving water use efficiency (WUE) by boosting crop yield. Ikram ul Haq et al. (2020) have shown that planting 8 rows of barley on beds alongside Egyptian clover grown in 120 cm irrigation furrows exhibited superior performance and emerged as the most promising approach concerning sustainability, profitability, and efficiency in utilizing irrigation water. Furthermore, a recent study done by (Liang et al., 2023) demonstrated that there was no significant difference in barley grain yield observed between barley monocropping and barley-pulse intercropping, particularly under conditions of limited irrigation. This suggests that barley-pulse intercropping systems might be well-suited for regions facing restricted irrigation resources or engaged in dryland farming. Cereal-pulse intercropping can enhance the use of soil moisture for crop growth and development by complimentary root growth coupled with improved water and nutrient uptake (Stomph et al., 2020; Gowtham et al., 2022; Rafique et al., 2023; Tanveer et al., 2023). Additionally, employing optimized tillage and mulching methods improves the harmony between water requirements for crop growth and the available water supply, thereby enhancing the water use efficiency (WUE) of intercropping (Yin et al., 2017; Khan et al., 2019; Jabborova et al., 2021; Najafi et al., 2021).
5.4 Other technologies
In recent years, there has been a remarkable increase in studies on the utilization of nanoparticles, such as TiO2, Ag, SiO2, and ZnO, to enhance plant growth under various environmental conditions. The mechanism of action of these nanoparticles is still unclear (Ahluwalia et al., 2021). However, it seems that the use of nanoparticles enhance germination, shoot and root growth, relative water content, nutrients uptake, osmotic status, oxidative status, and photosynthesis efficiency in several plants and under various environmental conditions (Arruda et al., 2015). In barley, the only study found in literature, by Moaveni et al. (2011), described a significant increase in yield in barley plants sprayed with Ti[O.sub.2] nanoparticle compared to those non-sprayed under field conditions. On the other hand, the use of these compounds with over dose can affect negatively plants growth. Indeed Martínez-Fernández et al. (2016) reported negative effects of nanoparticles on Helianthus annuus L., such us root dehydration and the up regulation of oxidative stress. Moulick et al. (2020), noted a possible long-term negative effect of these compounds on ecosystems.
Biochar, produced by the thermal treatment of biomass (wood, crop residues, or animal manure) under limited oxygen conditions, is widely cited in literature as fertilizer that can promote plants growth and enhance their tolerance against stressful conditions (Haider et al., 2022). In barley, Hafez et al. (2020) reported a considerable beneficial effect of biochar on antioxidant enzymes, anatomic characters, and osmotic status under drought stress. Nasiri et al. (2023) and Gul et al. (2023) recorded a significant increase in barley yield treated with biochar under drought stress. on the other hand, some disadvantages of biochar have been reported, such as soil alkalinization, unavailability (binding) of nutrients in the soil, binding of pesticides and herbicides, accumulation of heavy metals in soils treated with biochar, and affecting rhizosphere microorganisms (Ahluwalia et al., 2021).
Very recently, several studies have reported the utilization of hydrogels, a polymer capable of retaining a significant amount of water and nutrients. This involves the storage of water and nutrients for root plants, which can be utilized during periods of water scarcity (Zhang et al., 2021). However, the use of this technique is still limited in greenhouse setups.
6 Plant growth promoting rhizobacteria: An alternative for enhancing Hordeum vulgare productivity
Of different microbial populations present in the rhizosphere, bacteria are the most abundant microorganisms (Kaymak, 2010), and among these bacteria, we find Plant Growth-Promoting Rhizobacteria (PGPR). These represent a group of beneficial microorganisms inhabiting the rhizosphere and playing a crucial role in enhancing plant growth, yield, and crop quality (Figure 3) (Etesami and Maheshwari, 2018) under non-stress and stress conditions through various direct and indirect mechanisms (Mahmood et al., 2014).
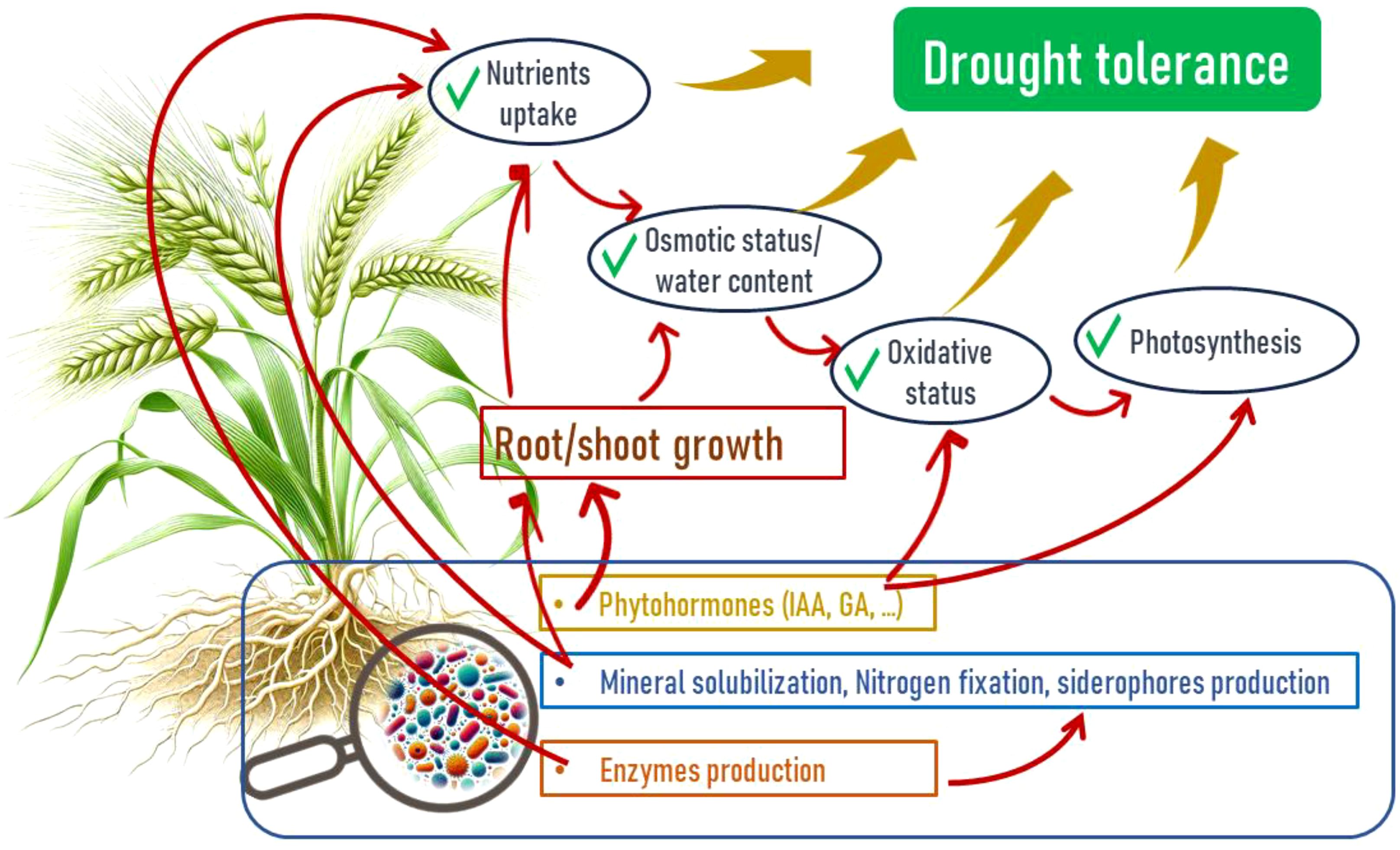
Figure 3. Mechanisms of barley drought tolerance enhancement by Plant Growth Promoting Rhizobacteria (PGPR) through direct and indirect effects.
Under normal and stressful conditions (abiotic (drought, salt, and heavy metal) and biotic (phytopathogenic species), PGPRs can affect plants directly by facilitating the uptake of certain nutrients by the plant through the synthesis of certain compounds by the bacteria and indirectly by reducing or preventing the undesirable effects of one or more phytopathogenic organisms (Kumar et al., 2019).
6.1 Direct mechanisms
Direct promotion is achieved through several mechanisms, including the production of phytohormones such as gibberellins, auxins (indole acetic acid), and cytokinin, which play an important role in cell elongation, cell division, tissue differentiation, and apical dominance (Kaymak, 2010). For example, the treatment of two selections of roses (Rosa canina and Rosa dumalis) and Pistacia vera with indole-3-butyric acid (IBA) improved the rooting rate and increased the number of lateral roots of the plants (Ercisli et al., 2004; Orhan et al., 2007).
Other direct mechanisms include nitrogen fixation and the solubilization of certain nutrients that limit plant growth. Physiological fixation of nitrogen by rhizobacteria makes it more accessible to plants, and can be symbiotic, forming nodules at plant roots, or non-symbiotic (Bellés-Sancho et al., 2023). To assimilate nitrogen, certain bacteria such as Azotobacter spp. and Azospirillum lipoferum have the capacity to synthesize the enzyme nitrogenase (Niewiadomska et al., 2018). Inoculation of Trifolium repens with Rhizobium and PGPR showed an increase in nodules, nitrogenase levels, and nitrogen content in roots and shoots compared with the control (Matse et al., 2020).
In the soil, phosphorus exists in two forms: inorganic and organic. Because of its low solubility, micro-organisms play a crucial role in the process of dissolving and mineralizing phosphorus, notably through the production of organic acids and phosphatases that catalyse the hydrolysis of phosphoric acid esters (Alori et al., 2017). In other words, phosphate solubilization is achieved through acidification, chelation, exchange reactions, and gluconic acid production (Hameeda et al., 2008). Phosphate solubilizing bacteria (PSB) are omnipresent (Gyaneshwar et al., 2002), where Bacillus, Enterobacter, Erwinia and Pseudomonas are among the most potent genera (Podile and Kishore, 2006). Mesorhizobium mediterraneum was able to effectively mobilize tricalcium phosphate and insoluble phosphates added to the soil and increase the P, N, K, Ca, Mg and dry matter content of barley and chickpea (Peix et al., 2001).
6.2 Indirect mechanisms
The indirect mechanisms comprise suppression of phytopathogens by the production of volatile HCN, siderophores, volatile metabolites, and ammonia, etc., induced systemic resistance in the host plant and competition with the pathogen for space and nutrients (Etesami and Adl, 2020).
The trivalent form of iron hydroxide (Fe3+) in the soil is difficult for plants to absorb, and to facilitate this uptake, bacteria produce low-molecular-weight molecules called siderophores. These siderophores act as iron chelators while making iron accessible to plants in case of deficiency (especially in neutral and alkaline soils) (Khan et al., 2018). Siderophores not only chelate iron but can also form stable complexes in the presence of other metals (Gu et al., 2020). Siderophores play a crucial role in plant disease management by depriving pathogens of iron, leading to their inhibition. For example, siderophores secreted by Pseudomonas are the main factors inhibiting the growth and development of fungal pathogens, including Colletotrichum dematium, Rhizoctonia solani and Sclerotium rolfsii (Sharma and Johri, 2003).
Volatile organic compounds (VOCs) secreted by rhizobacteria are low molecular weight compounds capable of diffusing through different matrices, including biological membranes, water, soil and air (Lemfack et al., 2014; Etminani et al., 2024) to provide inter- and intra-organism communication (Farag et al., 2013; Mhlongo et al., 2018). They play a crucial role in improving plant growth by modulating levels of phytohormones such as ethylene, auxin, and jasmonic acid and tolerance to abiotic stress (Song et al., 2008). In addition, a multitude of VOCs (benzothiazole, cyclohexanol, n-decanal, dimethyl trisulfide, 2-ethyl 1-hexanol, and nonanal) produced by bacteria in the rhizospheres of canola and soybean inhibit sclerotia and ascospore germination and mycelial growth of Sclerotinia sclerotiorum under both laboratory and field conditions (Fernando et al., 2005).
Hydrogen cyanide (HCN) is a toxic secondary metabolite that inhibits aerobic respiration activities (Williams et al., 2006). It is produced only in a few bacterial species, including P. aeruginosa (Neerincx et al., 2015). HCN produced by rhizobacteria plays an important role in the biological control of phytopathogens and pests (Suryadi et al., 2019). For example, HCN synthesised by Pseudomonas sp. inhibits certain pathogenic fungi (Suryadi et al., 2019), and that synthesised by P. chlororaphis O6 has shown nematocidal activity (Kang et al., 2018). Ammonia (NH3+) production helps to satisfy the nitrogen demand of host plants and in excess reduces plant colonization by pathogens. This NH3+ production is achieved by the hydrolysis of urea by nitrogenase into ammonia and carbon dioxide (Mbai et al., 2013), degradation of plant ACC, or deamination of amino acids (Etesami and Adl, 2020).
In 1990, a discovery revealed that certain non-pathogenic bacteria could prevent the metabolic changes caused by pathogen attacks by triggering a systemic response in the plant called induced systemic resistance (ISR) (Ali et al., 2022; Desai et al., 2023; Gowtham et al., 2024). This response was initially discovered in the plant model Arabidopsis thaliana and has since been observed in many plant species. In A. thaliana, the ISR response is mediated by ethylene and jasmonic acid as signal transducers. Additionally, the NPR1 protein is involved, which induces the expression of other proteins different from PRs. For example, P. fluorescens strain WCS417 was shown to be effective against Fusarium oxysporum f. sp. dianthi on carnations, acting protectively even when the bacteria remained confined to the root system of the plant (van Peer, 1991).
This phenomenon was observed with several PGPR strains applied to cucumber roots, providing protection against the anthracnose fungus Colletotrichum orbiculare during subsequent inoculations (Wei et al., 1991).
Through these mechanisms, the treatment of plants in general and barley specifically with PGPRs affects growth parameters positively and offers protection against abiotic and biotic stresses (Table 3).
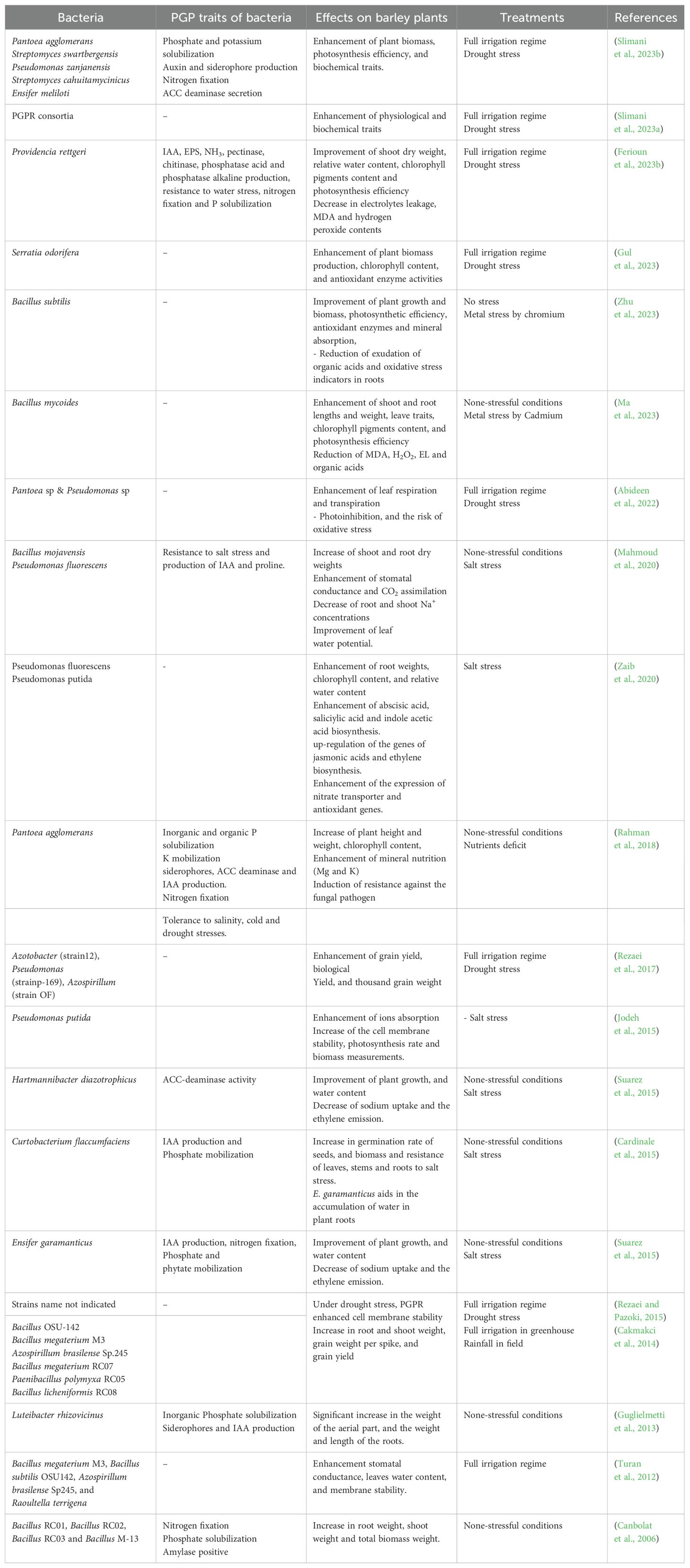
Table 3. Studies for the enhancement of barley drought tolerance employing bioinoculant based on plant growth promoting rhizobacteria (PGPR).
6.3 Root and shoot growth
The root system is the first part of the plant that is exposed to the PGPR influence. Generally, roots growth and architecture are affected by phytohormones such as auxins, gibberellin, and abscisic acid. The drought stress reduces barley plants length and biomass by affecting phytohormones balance (Barati et al., 2015; Bardehji et al., 2023). The bioinoculant based on PGPR showed a significant increase in various root traits in barley plant grown under drought stress. Ferioun et al. (2023b) described a significant increased effect of Providencia rettgeri on RDW. Slimani et al. (2023a) described four PGPR isolates that increased significantly roots length of barley plants under drought stress. In both last studies, PGPR strains were characterized as being able to produce high levels of indole-3-acetic acid (IAA) phytohormone. This last is widely reported in literature in the regulation of cells and tissues plant growth (Etesami and Adl, 2020). The majority of PGPR strains isolated were IAA producer, and many researches in other crops reported a significant relationship between phytohormones secretion and yield production (Ahluwalia et al., 2021) (Figure 1).
Several studies described a significant increase in shoot weight and length on barley plants inoculated with PGPR strains when compared to the uninoculated ones (Gul et al., 2023; Slimani et al., 2023a; Ferioun et al., 2023b). The last references described a higher significant positive correlation between shoot growth traits and root ones, which prove that shoot growth is linked to root development induced by PGPRs inoculation. Furthermore, the ability of PGPRs to fixate N2 and to solubilize some nutrients such as potassium and phosphate increases nutrient content in plants rhizosphere (Dhaked et al., 2022; Tirry et al., 2023). The increase in water and nutrients assimilation due to the root growth enhancement and nutrients assimilation lead to the improvement of photosynthesis efficiency and biomass production (Raklami et al., 2021). Moreover, the increase in shoot growth might be linked to the enhancement of ACC deaminase activity by PGPRs which promote physiological tolerance of plants under stressful conditions (Danish et al., 2020). This enzyme catalyzes the reaction of 1-aminocyclopropane-1-carboxylic acid (ACC) cleavage into ammonia and α-ketobutyrate, thereby inhibiting the formation of the stress hormone ethylene (Sagar et al., 2020; Naing et al., 2021). In barley, as in other cereals, the inoculation with ACC deaminase PGPR producer increased plant tolerance to drought stress (Chandra et al., 2019; Danish et al., 2020; Slimani et al., 2023b).
6.4 Water and osmotic status
Relative water content (RWC) is utilized in several studies as an indicator of plant water status. Generally, RWC is associated with the water taken by leaf tissue and the water lost through transpiration (Lugojan and Ciulca, 2011). High RWC value under stressful conditions reflects a strong ability of plant tissues to maintain their cell turgor pressure under stresses (Ferioun et al., 2023a). The inoculation of barley plants with PGPRs under drought stress increased RWC% when compared to the stressed uninoculated plants (Abideen et al., 2022; Ferioun et al., 2023b); aiding barley plants in mitigating damages associated with decreased cell turgor pressure. This effect is frequently linked to the effect of PGPRs on the opening/closure of stomates, root water absorption, and hydraulic conductivity (Ahluwalia et al., 2021).
Plant cells adjust their osmotic pressure to mitigate the damages caused by fluctuations in osmotic pressure (Sallam et al., 2019). The osmotic adjustment might be released by osmoregulation through solutes accumulation which leads to reduced osmotic pressure in plant cells (Ahluwalia et al., 2021). On the other hand, the osmo-protection might be involved by antioxidant system or by the accumulation of osmo-protectants such as polyamines, sugars, and proline (Zulfiqar et al., 2020; Eswaran et al., 2024). Askarnejad et al. (2021); Slimani et al. (2023a); Ferioun et al. (2023b), and Slimani et al. (2023b) reported a significant impact of PGPR inoculation on sugars and proline contents in barley plants under drought stress, which confirm the strong effect of PGPRs on the adjustment of osmotic pressure and on the enhancement of physiological tolerance.
6.5 Oxidative status
In barley plants inoculated with PGPRs, the contents of H2O2 and MDA under various environmental conditions is significantly fewer than uninoculated plants under drought stress (Zaib et al., 2020; Abideen et al., 2022; Slimani et al., 2023a; Ferioun et al., 2023b). The same references indicated a significant increase in antioxidant (scavenging) enzyme activities (catalase, peroxidase, superoxide dismutase) in barley plants inoculated with PGPRs when compared to the uninoculated ones. Indeed, several studies reported a negative correlation between antioxidant enzyme activities and H2O2 content (Zhanassova et al., 2021), which confirms that PGPRs reduce oxidative stress induced by water shortage via the up-regulation of scavenging enzyme activities.
6.6 Photosynthesis efficiency
In barley, under non-stressful conditions, under drought stress, and in the case of other abiotic stresses, PGPRs enhance photosynthesis efficiency, which improves biomass and organic matter production (Zaib et al., 2020; Ahluwalia et al., 2021; Abideen et al., 2022; Abbasi et al., 2023). PGPRs promote chlorophyll pigments synthesis pathways directly via hormones synthesis or indirectly via the enhancement of water and nutrients uptake (Ghanbarzadeh et al., 2020). This increase in chlorophyll content leads to the optimization of photosynthetic rate and photosystem II efficiency, and the functioning of the complex pigment-proteins (Abideen et al., 2022). This last study reported that, the inoculation of barley plants with Pantoea sp. & Pseudomonas sp. under drought stress optimized leaf transpiration, gas exchange, as well as stomatal conductance.
7 Prospects and challenges for the future
In the next few years, the need for increased outputs, better crop productivity, soil health, and ecologically sound farming is growing. Breeding genotypes resilient to drought has great potential to increase barley's ability to withstand water shortage in future years, but it also comes with a number of difficulties. Through the use of genome editing, genomic selection, and marker-assisted selection (MAS), drought-tolerant barley genotypes may be developed more quickly than ever before as a result of developments in molecular genetics, genomics, and phenotyping technology (Sallam et al., 2019). By using these methods, breeders may quickly accelerate the genetic development of drought tolerance characteristics by identifying and introducing advantageous alleles linked to drought tolerance from broad germplasm pools and wild barley relatives into top breeding lines. On the other hand, there are still a number of difficulties in selecting barley drought-tolerant genotypes despite these technical developments. Drought tolerance is a complex feature involving many interacting physiological, biochemical, and molecular systems, it is difficult to properly deconstruct and regulate these qualities. Moreover, Genotypes x Environment (G x E) interactions and polygenicity are common in the genetic architecture of drought tolerance traits, making multi-environment trials and strong statistical techniques necessary for precise trait assessment and selection. Furthermore, socioeconomic and legal obstacles, such as those pertaining to intellectual property rights, public acceptability, and farmer adoption, stand in the way of the broad distribution and adoption of drought-tolerant barley cultivars.
The strategic use of bio-inoculants to improve barley stress tolerance to drought has great potential in the upcoming years. PGPR bio-inoculants offer a sustainable and agroecological solution (Abbasi et al., 2023). The combination of these bio-inoculants with suitable breeding approach seems promising for developing barley genotypes with enhanced drought resilience indices. To fully realize the promise of bio-inoculants for improving barley's resistance to drought stress, several challenges must be overcome: (i) Field experiments and on-farm demonstrations are necessary to further investigate the efficacy and consistency of bio-inoculant performance under various environmental circumstances and agricultural techniques; (ii) Strong screening and selection processes that take into account genotype-microbiome interactions and compatibility with other agricultural inputs are necessary for the selection and optimization of appropriate bio-inoculant strains for particular barley cultivars and agroecosystems; (iii) It is necessary to address the flexibility, cost-effectiveness, and commercial feasibility of bio-inoculant manufacturing and application technologies in order to enable farmers and stakeholders to use them widely. To overcome these challenges and ensure food security in the face of water scarcity and climate change, interdisciplinary research collaborations, technological advancements, and policy support will be necessary to support the sustainable integration of bio-inoculants into barley cultivation systems.
8 Conclusion
Drought strongly influences barley plant physiology and productivity and lowers the economic incomes of barley crops. Genotype breeding is among the solutions widely studied to obtain robust and resilient barley genotypes, aiming to mitigate barley losses caused by water scarcity. Tolerant genotypes exhibit fewer physiological disturbances and maintain relatively high productivity even under stressful conditions. At the same time, bio-inoculants based on PGPRs are considered effective agroecological and sustainable solution. PGPRs used for bio-inoculant construction exhibit strong PGP traits including enhancing nutrients availability, phytohormones secretion, EPS production, and the stimulation of plant systemic defence mechanisms. Barley plants inoculated with these bio-inoculants showed higher physiological resistance and yield productivity. Based on the genotype x microbiome interaction, developing appropriate bio-inoculants for specific barley cultivars and agroecosystems is a highly effective solution that can maximize barley yield production under diverse conditions.
Author contributions
MF: Conceptualization, Data curation, Formal analysis, Investigation, Methodology, Software, Writing – original draft, Writing – review & editing. IZ: Writing – original draft. SB: Writing – original draft. YE: Writing – original draft. DB: Writing – original draft. AE: Writing – original draft. SL: Conceptualization, Data curation, Formal analysis, Funding acquisition, Investigation, Methodology, Project administration, Resources, Software, Supervision, Validation, Visualization, Writing – original draft, Writing – review & editing. RS: Visualization, Writing – review & editing. NE: Conceptualization, Data curation, Formal analysis, Funding acquisition, Investigation, Methodology, Project administration, Resources, Software, Supervision, Validation, Visualization, Writing – original draft, Writing – review & editing.
Funding
The author(s) declare that no financial support was received for the research, authorship, and/or publication of this article.
Conflict of interest
The authors declare that the research was conducted in the absence of any commercial or financial relationships that could be construed as a potential conflict of interest.
Publisher’s note
All claims expressed in this article are solely those of the authors and do not necessarily represent those of their affiliated organizations, or those of the publisher, the editors and the reviewers. Any product that may be evaluated in this article, or claim that may be made by its manufacturer, is not guaranteed or endorsed by the publisher.
Abbreviations
ABA, Abscisic acid; APX, Ascorbate peroxidase; BCS, Barley Core Selected Collection; CAT, Catalase; DHAR, Dehydro-ascorbate reductase; GPX, Guaiacol peroxidase; GR, Glutathione reductase; GY, Grain yield; GWAS, Genome-Wide Association Study; HMP, Harmonic mean productivity; IAA, Indole-3-acetic acid; IBA, indole-3-butyric; ISR, Induced systemic resistance; N, Nitrogen; MDA, Malondialdehyde; MDHAR, Mono dehydro-ascorbate reductase; MP, Mean productivity; P, Phosphate; PGPR, Plant Growth Promoting Rhizobacteria; ROS, Reactive oxygen species; RWC, Relative water content; SNP, Single nucleotide polymorphism; SOD, Superoxide dismutase; SSI, Susceptibility index; STI, Stress tolerance index; TGW, Thousand grain weight; TOL, Tolerance index.
References
Abbasi, A., Mehri, S., Solimanzadeh, H., Alipour, S. (2023). Growth, yield, and photosynthetic dry matter remobilization response of barley to plant growth promoting rhizobacteria (PGPR) and nitrogen. Philippine Agric. Sci. 106, 4. doi: 10.62550/HU082022
Abdel-Ghani, A. H., Neumann, K., Wabila, C., Sharma, R., Dhanagond, S., Owais, S. J., et al. (2015). Diversity of germination and seedling traits in a spring barley (Hordeum vulgare L.) collection under drought simulated conditions. Genet. Resour. Crop Evol. 62, 275−292. doi: 10.1007/s10722-014-0152-z
Abideen, Z., Cardinale, M., Zulfiqar, F., Koyro, H.-W., Rasool, S. G., Hessini, K., et al. (2022). Seed endophyte bacteria enhance drought stress tolerance in Hordeum vulgare by regulating, physiological characteristics, antioxidants and minerals uptake. Front. Plant Sci. 13, 980046. doi: 10.3389/fpls.2022.980046
Abou-Elwafa, S. F. (2016). Association mapping for drought tolerance in barley at the reproductive stage. Comptes rendus biol. 339, 51−59. doi: 10.1016/j.crvi.2015.12.002
Aboughadareh, A. P., Naghavi, M. R., Khalili, M. (2013). Water deficit stress tolerance in some of barley genotypes and landraces under field conditions. Notulae Sci. Biol. 5, 249−255. doi: 10.15835/nsb529066
Adriana, C. (2020). Evaluation of some barley varieties concerning seed germination under different drought stress. 24 (1), 78–84.
Ahluwalia, O., Singh, P. C., Bhatia, R. (2021). A review on drought stress in plants : Implications, mitigation and the role of plant growth promoting rhizobacteria. Res. Environ. Sustain. 5, 100032. doi: 10.1016/j.resenv.2021.100032
Ahmed, I. M., Dai, H., Zheng, W., Cao, F., Zhang, G., Sun, D., et al. (2013). Genotypic differences in physiological characteristics in the tolerance to drought and salinity combined stress between Tibetan wild and cultivated barley. Plant Physiol. Biochem. 63, 49−60. doi: 10.1016/j.plaphy.2012.11.004
Ajalli, J., Salehi, M. (2012). Evaluation of Drought Stress Indices in barley (Hordeum vulgare L.). Ann. Biol. Res. 2012, 5515−5520.
Alghabari, F., Ihsan, M. Z. (2018). Effects of drought stress on growth, grain filling duration, yield and quality attributes of barley (Hordeum vulgare L.). Bangladesh J. Bot. 47, 421−428. doi: 10.3329/bjb.v47i3.38679
Ali, S. A. M., Sayyed, R. Z., Mir, M. I., Hameeda, B., Khan, Y., Alkhanani, M. F., et al. (2022). Induction of Systemic Resistance and Antibiofilm activity of Surfactin from Bacillus velezensis MS20 and evaluation of its Induced. Front. Microbiol. 13. doi: 10.3389/fmicb.2022.879739
Alori, E. T., Glick, B. R., Babalola, O. O. (2017). Microbial phosphorus solubilization and its potential for use in sustainable agriculture. Front. Microbiol. 8. doi: 10.3389/fmicb.2017.00971
Alqudah, A. M., Koppolu, R., Wolde, G. M., Graner, A., Schnurbusch, T. (2016). The genetic architecture of barley plant stature. Front. Genet. 7, 203302. doi: 10.3389/fgene.2016.00117
Alqudah, A. M., Youssef, H. M., Graner, A., Schnurbusch, T. (2018). Natural variation and genetic make-up of leaf blade area in spring barley. Theor. Appl. Genet. 131, 873−886. doi: 10.1007/s00122-018-3053-2
Al-Turki, A., Murali, M., Omar, A. F., Rehan, M., Sayyed, R. Z. (2023). Exploring the recent advances in PGPR mediated resilience towards interactive effects of drought and salt stress in plants. Front. Microbiol. 14. doi: 10.3389/fmicb.2023.1214845
Amirjani, M. R., Mahdiyeh, M. (2013). Antioxidative and biochemical responses of wheat to drought stress. J. Agric. Biol. Sci. 8, 291−301.
Ansari, W. A., Atri, N., Pandey, M., Singh, A. K., Singh, B., Pandey, S. (2019). Influence of drought stress on morphological, physiological and biochemical attributes of plants : A review. Biosci. Biotechnol. Res. Asia 16, 697−709. doi: 10.13005/bbra/2785
Arruda, S. C. C., Silva, A. L. D., Galazzi, R. M., Azevedo, R. A., Arruda, M. A. Z. (2015). Nanoparticles applied to plant science : A review. Talanta 131, 693−705. doi: 10.1016/j.talanta.2014.08.050
Arshadi, A., Karami, E., Khateri, B., Rezabakhsh, P. (2016). Drought stress effects on the grain yield among different barley cultivars. Genetika 48, 1087−1100. doi: 10.2298/GENSR1603087A
Arshadi, A., Karami, E., Sartip, A., Zare, M., Rezabakhsh, P. (2018). Genotypes performance in relation to drought tolerance in barley using multi-environment trials. doi: 10.15159/AR.18.004
Asghari, B., Khademian, R., Sedaghati, B. (2020). Plant growth promoting rhizobacteria (PGPR) confer drought resistance and stimulate biosynthesis of secondary metabolites in pennyroyal (Mentha pulegium L.) under water shortage condition. Sci. Hortic. 263, 109132. doi: 10.1016/j.scienta.2019.109132
Ashry, N. M., Alaidaroos, B. A., Mohamed, S. A., Badr, O. A., El-Saadony, M. T., Esmael, A. (2022). Utilization of drought-tolerant bacterial strains isolated from harsh soils as a plant growth-promoting rhizobacteria (PGPR). Saudi J. Biol. Sci. 29, 1760−1769. doi: 10.1016/j.sjbs.2021.10.054
Askarnejad, M. R., Soleymani, A., Javanmard, H. R. (2021). Barley ( Hordeum vulgare L.) physiology including nutrient uptake affected by plant growth regulators under field drought conditions. J. Plant Nutr. 1−17, 2201–2217. doi: 10.1080/01904167.2021.1889593
Ayad, J. Y., Al-Abdallat, A. M., Saoub, H. M. (2010). Variation in root water and nitrogen uptake and their interactive effects on growth and yield of spring wheat and barley genotypes. Int. J. Bot. 6, 404−413. doi: 10.3923/ijb.2010.404.413
Bangash, N., Mahmood, S., Akhtar, S., Hayat, M. T., Gulzar, S., Khalid, A. (2021). Formulation of biofertilizer for improving growth and yield of wheat in rain dependent farming system. Environ. Technol. Innovation 24, 101806. doi: 10.1016/j.eti.2021.101806
Barati, M., Majidi, M. M., Mirlohi, A., Pirnajmodini, F., Sharif-Moghaddam, N. (2015). Response of cultivated and wild barley germplasm to drought stress at different developmental stages. Crop Sci. 55, 2668−2681. doi: 10.2135/cropsci2015.04.0229
Bardehji, S., Soltan, S., Eshghizadeh, H. R., Zahedi, M., Zare, S., Koçak, M. Z., et al. (2023). Responses of two-row and six-row barley genotypes to elevated carbon dioxide concentration and water stress. Agronomy 13, 2373. doi: 10.3390/agronomy13092373
Baris, O., Sahin, F., Turan, M., Orhan, F., Gulluce, M. (2014). Use of plant-growth-promoting rhizobacteria (PGPR) seed inoculation as alternative fertilizer inputs in wheat and barley production. Commun. Soil Sci. Plant Anal. 45, 2457−2467. doi: 10.1080/00103624.2014.912296
Bellés-Sancho, P., Beukes, C., James, E. K., Pessi, G. (2023). Nitrogen-fixing symbiotic paraburkholderia species : current knowledge and future perspectives. Nitrogen 4, 135−158. doi: 10.3390/nitrogen4010010
Benito-Verdugo, P., Martínez-Fernández, J., González-Zamora, Á., Almendra-Martín, L., Gaona, J., Herrero-Jiménez, C. M. (2023). Impact of agricultural drought on barley and wheat yield: a comparative case study of Spain and Germany. Agriculture 13, 2111. doi: 10.3390/agriculture13112111
Bista, D. R., Heckathorn, S. A., Jayawardena, D. M., Mishra, S., Boldt, J. K. (2018). Effects of drought on nutrient uptake and the levels of nutrient-uptake proteins in roots of drought-sensitive and-tolerant grasses. Plants 7, 28. doi: 10.3390/plants7020028
Biswal, A. K., Kohli, A. (2013). Cereal flag leaf adaptations for grain yield under drought : Knowledge status and gaps. Mol. Breed. 31, 749−766. doi: 10.1007/s11032-013-9847-7
Bittencourt, P. P., Alves, A. F., Ferreira, M. B., da Silva Irineu, L. E. S., Pinto, V. B., Olivares, F. L. (2023). Mechanisms and applications of bacterial inoculants in plant drought stress tolerance. Microorganisms 11, 502. doi: 10.3390/microorganisms11020502
Bogati, K., Walczak, M. (2022). The impact of drought stress on soil microbial community, enzyme activities and plants. Agronomy 12, 189. doi: 10.3390/agronomy12010189
Boudiar, R., Casas, A. M., Gioia, T., Fiorani, F., Nagel, K. A., Igartua, E. (2020). Effects of low water availability on root placement and shoot development in landraces and modern barley cultivars. Agronomy 10, 134. doi: 10.3390/agronomy10010134
Bouhraoua, S., Ferioun, M., Boussakouran, A., Belahcen, D., Srhiouar, N., Hammani, K., et al. (2024). Physio-biochemical responses and yield performance of North African barley genotypes submitted to moderate and severe salinity. Cereal Res. Commun. doi: 10.1007/s42976-024-00549-9
Bouhraoua, S., Ferioun, M., Nassira, S., Boussakouran, A., Akhazzane, M., Belahcen, D., et al. (2023). Biomass partitioning and physiological responses of four Moroccan barley varieties subjected to salt stress in a hydroponic system. J. Plant Biotechnol. 50, 115−126. doi: 10.5010/JPB.2023.50.015.115
Boussakouran, A., El Yamani, M., Sakar, E. H., Rharrabti, Y. (2021). Genetic advance and grain yield stability of moroccan durum wheats grown under rainfed and irrigated conditions. Int. J. Agron. 1–13, 5571501. doi: 10.1155/2021/5571501
Boussakouran, A., Sakar, E. H., El Yamani, M., Rharrabti, Y. (2019). Morphological traits associated with drought stress tolerance in six Moroccan durum wheat varieties released between 1984 and 2007. J. Crop Sci. Biotechnol. 22, 345−353. doi: 10.1007/s12892-019-0138-0
Cakmakci, R., Turan, M., Gulluce, M., Sahin, F. (2014). Rhizobacteria for reduced fertilizer inputs in wheat (Triticum aestivum spp. Vulgare) and barley (Hordeum vulgare) on Aridisols in Turkey. International J. Plant Prod. 8 (2), 163–180.
Canbolat, M. Y., Bilen, S., Çakmakçı, R., Şahin, F., Aydın, A. (2006). Effect of plant growth-promoting bacteria and soil compaction on barley seedling growth, nutrient uptake, soil properties and rhizosphere microflora. Biol. Fertil. Soils 42, 350−357. doi: 10.1007/s00374-005-0034-9
Cardinale, M., Ratering, S., Suarez, C., Zapata Montoya, A. M., Geissler-Plaum, R., Schnell, S. (2015). Paradox of plant growth promotion potential of rhizobacteria and their actual promotion effect on growth of barley (Hordeum vulgare L.) under salt stress. Microbiol. Res. 181, 22−32. doi: 10.1016/j.micres.2015.08.002
Castaneda, R., Doan, D., Newhouse, D. L., Nguyen, M., Uematsu, H., Azevedo, J. P. (2016). Who are the poor in the developing world? World Bank Policy Res. Work. Paper, 7844.
Cavicchioli, R., Ripple, W. J., Timmis, K. N., Azam, F., Bakken, L. R., Baylis, M., et al. (2019). Scientists’ warning to humanity : Microorganisms and climate change. Nat. Rev. Microbiol. 17, 569−586. doi: 10.1038/s41579-019-0222-5
Chandra, D., Srivastava, R., Gupta, V. V. S. R., Franco, C. M. M., Sharma, A. K. (2019). Evaluation of ACC-deaminase-producing rhizobacteria to alleviate water-stress impacts in wheat ( Triticum aestivum L.) plants. Can. J. Microbiol. 65, 387−403. doi: 10.1139/cjm-2018-0636
Channaoui, S., El Idrissi, I. S., Mazouz, H., Nabloussi, A. (2019). Reaction of some rapeseed (Brassica napus L.) genotypes to different drought stress levels during germination and seedling growth stages. OCL 26, 23. doi: 10.1051/ocl/2019020
Chaudhary, T., Dixit, M., Gera, R., Shukla, A. K., Prakash, A., Gupta, G., et al. (2020). Techniques for improving formulations of bioinoculants. 3 Biotech. 10, 199. doi: 10.1007/s13205-020-02182-9
Cramer, W., Guiot, J., Marini, K., Secretariat, M., Plan Bleu, F., Cramer, W., et al. (2020). Climate and environmental change in the Mediterranean Basin–current situation and risks for the future. First Mediterr. Assess. Rep.
Danish, S., Zafar-ul-Hye, M., Mohsin, F., Hussain, M. (2020). ACC-deaminase producing plant growth promoting rhizobacteria and biochar mitigate adverse effects of drought stress on maize growth. PloS One 15, e0230615. doi: 10.1371/journal.pone.0230615
Das, K., Roychoudhury, A. (2014). Reactive oxygen species (ROS) and response of antioxidants as ROS-scavengers during environmental stress in plants. Front. Environ. Sci. 2, 53. doi: 10.3389/fenvs.2014.00053
De Mezer, M., Turska-Taraska, A., Kaczmarek, Z., Glowacka, K., Swarcewicz, B., Rorat, T. (2014). Differential physiological and molecular response of barley genotypes to water deficit. Plant Physiol. Biochem. 80, 234−248. doi: 10.1016/j.plaphy.2014.03.025
Desai, A., Ruparelia, J., Jha, C. K., Sayyed, R. Z., Mitra, D., Priyadarshini, A., et al. (2023). Articulating Beneficial rhizobacteria mediated plant defenses through induced systemic resistances. Pedosphere 33, 557–567. doi: 10.1016/j.pedsph.2022.10.003
Dhaked, B. S., Koli, G. K., Triveni, S., Reddy, R. S., Jaiswal, A., Koli, D. K. (2022). Screening of potassium and zinc solubilizing bacteria for plant growth promoting properties (PGPR) from different rhizospheric soil. Pharma Innov. J. 11, 1315−1319.
Dhanda, S. S., Sethi, G. S., Behl, R. K. (2004). Indices of drought tolerance in wheat genotypes at early stages of plant growth. J. Agron. Crop Sci. 190, 6−12. doi: 10.1111/j.1439-037X.2004.00592.x
Dietz, K.-J., Zörb, C., Geilfus, C.-M. (2021). Drought and crop yield. Plant Biol. 23, 881−893. doi: 10.1111/plb.13304
Dong, N., Tang, M.-M., Zhang, W.-P., Bao, X.-G., Wang, Y., Christie, P., et al. (2018). Temporal differentiation of crop growth as one of the drivers of intercropping yield advantage. Sci. Rep. 8, 3110. doi: 10.1038/s41598-018-21414-w
Duman, I. (2006). Effects of seed priming with PEG or K3PO4 on germination and seedling growth in lettuce. Pakistan J. Biol. Sci. 9 (5), 923–928. doi: 10.5555/20063105795
Elakhdar, A., Solanki, S., Kubo, T., Abed, A., Elakhdar, I., Khedr, R., et al. (2022). Barley with improved drought tolerance : Challenges and perspectives. Environ. Exp. Bot. 201, 104965. doi: 10.1016/j.envexpbot.2022.104965
El-Denary, M. E., El-Shawy, E. E. (2014). Molecular and field analysis of some barley genotypes for water stress tolerance. Egypt J. Genet. Cytol 43, 187−198. doi: 10.21608/ejgc.2014.9940
Elouattassi, Y., Ferioun, M., El Ghachtouli, N., Derraz, K., Rachidi, F. (2023). Agroecological concepts and alternatives to the problems of contemporary agriculture : Monoculture and chemical fertilization in the context of climate change. J. Agric. Environ. Int. Dev. (JAEID) 117, 41−98. doi: 10.36253/jaeid-14672
ElSayed, A. I., Mohamed, A. H., Rafudeen, M. S., Omar, A. A., Awad, M. F., Mansour, E. (2022). Polyamines mitigate the destructive impacts of salinity stress by enhancing photosynthetic capacity, antioxidant defense system and upregulation of calvin cycle-related genes in rapeseed (Brassica napus L.). Saudi J. Biol. Sci. 29, 3675–3686. doi: 10.1016/j.sjbs.2022.02.053
Ercisli, S., Esitken, A., Sahin, F. (2004). Exogenous IBA and inoculation with Agrobacterium rubi stimulate adventitious root formation on hardwood stem cuttings of two rose genotypes. HortScience 39, 533−534. doi: 10.21273/hortsci.39.3.533
Eswaran, S. U. D., Sundaram, L., Perveen, K., Bukhari, N. A., Sayyed, R. Z. (2024). Osmolyte-producing microbial biostimulants regulate the growth of Arachis hypogaea L. under drought stress. BMC Microbiol. 24, 165. doi: 10.1186/s12866-024-03320-6
Etesami, H., Adl, S. M. (2020). Plant growth-promoting rhizobacteria (PGPR) and their action mechanisms in availability of nutrients to plants. Phyto-Microbiome Stress Regul. 10 (4), 147–203. doi: 10.1007/978-981-15-2576-6_9
Etesami, H., Maheshwari, D. K. (2018). Use of plant growth promoting rhizobacteria (PGPRs) with multiple plant growth promoting traits in stress agriculture : Action mechanisms and future prospects. Ecotoxicol. Environ. Saf. 156, 225−246. doi: 10.1016/j.ecoenv.2018.03.013
Etminani, F., Fazeli-Nasab, B., Gowtham, H. G., Mirzaei, A. R., Barasarathi, J., Sayyed, R. Z. (2024). Bioinformatics investigation of the effect of volatile and non-volatile compounds of rhizobacteria in inhibiting late embryogenesis abundant protein that induces drought tolerance. Open Agric. 9, 20220252. doi: 10.1515/opag-2022-0252
Fadiji, A. E., Orozco-Mosqueda, M., del, C., de los Santos-Villalobos, S., Santoyo, G., Babalola, O. O. (2022). Recent developments in the application of plant growth-promoting drought adaptive rhizobacteria for drought mitigation. Plants 11, 3090. doi: 10.3390/plants11223090
Fan, Y., Wang, Z., Liao, D., Raza, M. A., Wang, B., Zhang, J., et al. (2020). Uptake and utilization of nitrogen, phosphorus and potassium as related to yield advantage in maize-soybean intercropping under different row configurations. Sci. Rep. 10, 9504. doi: 10.1038/s41598-020-66459-y
Farag, M. A., Zhang, H., Ryu, C. M. (2013). Dynamic chemical communication between plants and bacteria through airborne signals : induced resistance by bacterial volatiles. J. Chem. Ecol. 39, 1007−1018. doi: 10.1007/s10886-013-0317-9
Farooq, M., Hussain, M., Wahid, A., Siddique, K. H. M. (2012). Drought stress in plants : An overview. Plant responses to drought stress: From morphol. to Mol. feat. 1−33. doi: 10.1007/978-3-642-32653-0_1
Feng, X., Liu, W., Cao, F., Wang, Y., Zhang, G., Chen, Z.-H., et al. (2020). Overexpression of HvAKT 1 improves drought tolerance in barley by regulating root ion homeostasis and ROS and NO signaling. J. Exp. Bot. 71, 6587−6600. doi: 10.1093/jxb/eraa354
Ferioun, M., Bouhraoua, S., Belahcen, D., Zouitane, I., Srhiouar, N., Louahlia, S., et al. (2024). PGPR consortia enhance growth and yield in barley cultivars subjected to severe drought stress and subsequent recovery. Rhizosphere, 100926. doi: 10.1016/j.rhisph.2024.100926
Ferioun, M., Bouhraoua, S., Srhiouar, N., Tirry, N., Belahcen, D., Siang, T. C., et al. (2023a). Optimized drought tolerance in barley (Hordeum vulgare L.) using plant growth-promoting rhizobacteria (PGPR). Biocatal. Agric. Biotechnol. 50, 102691. doi: 10.1016/j.bcab.2023.102691
Ferioun, M., Srhiouar, N., Bouhraoua, S., Elghachtouli, N., Louahlia, S. (2023b). Physiological and biochemical changes in Moroccan barley (Hordeum vulgare L.) cultivars submitted to drought stress. Heliyon 9 (2), e13643.
Fernando, W. G. D., Ramarathnam, R., Krishnamoorthy, A. S., Savchuk, S. C. (2005). Identification and use of potential bacterial organic antifungal volatiles in biocontrol. Soil Biol. Biochem. 37, 955−964. doi: 10.1016/j.soilbio.2004.10.021
Filek, M., Labanowska, M., Kościelniak, J., Biesaga-Kościelniak, J., Kurdziel, M., Szarejko, I., et al. (2015). Characterization of barley leaf tolerance to drought stress by chlorophyll fluorescence and electron paramagnetic resonance studies. J. Agron. Crop Sci. 201, 228−240. doi: 10.1111/jac.2015.201.issue-3
Fischer, R. A., Maurer, R. (1978). Drought resistance in spring wheat cultivars. I. Grain yield responses. Aust. J. Agric. Res. 29, 897−912. doi: 10.1071/ar9780897
Francini, A., Sebastiani, L. (2019). Abiotic stress effects on performance of horticultural crops. Horticulturae 5, 67. doi: 10.3390/horticulturae5040067
Fu, L., Penton, C. R., Ruan, Y., Shen, Z., Xue, C., Li, R., et al. (2017). Inducing the rhizosphere microbiome by biofertilizer application to suppress banana Fusarium wilt disease. Soil Biol. Biochem. 104, 39−48. doi: 10.1016/j.soilbio.2016.10.008
Fu, L., Wu, D., Zhang, X., Xu, Y., Kuang, L., Cai, S., et al. (2022). Vacuolar H+-pyrophosphatase HVP10 enhances salt tolerance via promoting Na+ translocation into root vacuoles. Plant Physiol. 188, 1248−1263. doi: 10.1093/plphys/kiab538
Gao, Y., Quan, S., Lyu, B., Tian, T., Liu, Z., Nie, Z., et al. (2022). Barley transcription factor HvNLP2 mediates nitrate signaling and affects nitrogen use efficiency. J. Exp. Bot. 73, 770–783. doi: 10.1093/jxb/erab245
Gbegbelegbe, S., Alene, A., Swamikannu, N., Frija, A. (2024). Multi-dimensional impact assessment for priority setting of agricultural technologies: An application of TOPSIS for the drylands of sub-Saharan Africa and South Asia. PloS one 19 (11), e0314007.
G. C. J. Fernandez. Adaptation of food crops to temperature and water stress: proceedings of an international symposium, Taiwan, 13-18 August 1992., 1993, 257-270 ref. 22 Adaptation of food crops to temperature and water stress: proceedings of an international symposium, Taiwan, 13-18 August 1992. C. G. Kuo Department of Agricultural Economics, University of Nevada, Reno, NV 89557-0107, USA.
Geng, L., Li, M., Xie, S., Wu, D., Ye, L., Zhang, G. (2021). Identification of genetic loci and candidate genes related to β-glucan content in barley grain by genome-wide association study in International Barley Core Selected Collection. Mol. Breed. 41, 6. doi: 10.1007/s11032-020-01199-5
Geravandi, M., Farshadfar, E., Kahrizi, D. (2011). Evaluation of some physiological traits as indicators of drought tolerance in bread wheat genotypes. Russian J. Plant Physiol. 58, 69−75. doi: 10.1134/S1021443711010067
Ghadirnezhad Shiade, S. R., Fathi, A., Taghavi Ghasemkheili, F., Amiri, E., Pessarakli, M. (2023). Plants’ responses under drought stress conditions: Effects of strategic management approaches—A review. J. Plant Nutr. 46, 2198–2230. doi: 10.1080/01904167.2022.2105720
Ghanbarzadeh, Z., Mohsenzadeh, S., Rowshan, V., Zarei, M. (2020). Mitigation of water deficit stress in Dracocephalum moldavica by symbiotic association with soil microorganisms. Sci. Hortic. 272, 109549. doi: 10.1016/j.scienta.2020.109549
Ghanifathi, T., Valizadeh, M., Shahryari, R., Shahbazi, H., Mollasadeghi, V. (2011). Effect of drought stress on germination indices and seedling growth of 12 bread wheat genotypes. Adv. Environ. Biol. 5, 1034−1039.
Ghotbi-Ravandi, A. A., Shahbazi, M., Shariati, M., Mulo, P. (2014). Effects of mild and severe drought stress on photosynthetic efficiency in tolerant and susceptible barley (Hordeum vulgare L.) genotypes. J. Agron. Crop Sci. 200, 403–415. doi: 10.1111/jac.12062
Gonzalez, A., Bermejo, V., Gimeno, B. S. (2010). Effect of different physiological traits on grain yield in barley grown under irrigated and terminal water deficit conditions. J. Agric. Sci. 148, 319−328. doi: 10.1017/S0021859610000031
Gosal, S. S., Wani, S. H., Kang, M. S. (2009). Biotechnology and drought tolerance. J. Crop Improve. 23, 19−54. doi: 10.1080/15427520802418251
Gowtham, H. G., Murali, M., Shilpa, N., Amruthesh, K. N., Gafur, A., Antonius S and Sayyed, R. Z. (2024). Harnessing abiotic elicitors to bolster plant’s resistance against bacterial pathogens. Plant Stress 11, 100371. doi: 10.1016/j.stress.2024.100371
Gowtham, H. G., Singh, S. B., Shilpa, N., Aiyaz, M., Nataraj, K., Udayashankar, A. C., et al. (2022). Insight into Recent Progress and Perspectives in Improvement of Antioxidant Machinery upon PGPR Augmentation in Plants under Drought Stress : A Review. Antioxidants 11, 9. doi: 10.3390/antiox11091763
Gu, S., Wei, Z., Shao, Z., Friman, V. P., Cao, K., Yang, T., et al. (2020). Competition for iron drives phytopathogen control by natural rhizosphere microbiomes. Nat. Microbiol. 5, 1002−1010. doi: 10.1038/s41564-020-0719-8
Guan, L. M., Scandalios, J. G. (2000). Hydrogen peroxide-mediated catalase gene expression in response to wounding. Free Radical Biol. Med. 28, 1182−1190. doi: 10.1016/S0891-5849(00)00212-4
Guglielmetti, S., Basilico, R., Taverniti, V., Arioli, S., Piagnani, C., Bernacchi, A. (2013). Luteibacter rhizovicinus MIMR1 promotes root development in barley (Hordeum vulgare L.) under laboratory conditions. World J. Microbiol. Biotechnol. 29, 2025−2032. doi: 10.1007/s11274-013-1365-6
Gul, F., Khan, I. U., Rutherford, S., Dai, Z.-C., Li, G., Du, D.-L. (2023). Plant growth promoting rhizobacteria and biochar production from Parthenium hysterophorus enhance seed germination and productivity in barley under drought stress. Front. Plant Sci. 14, 1175097. doi: 10.3389/fpls.2023.1175097
Guo, P., Baum, M., Grando, S., Ceccarelli, S., Bai, G., Li, R., et al. (2009). Differentially expressed genes between drought-tolerant and drought-sensitive barley genotypes in response to drought stress during the reproductive stage. J. Exp. Bot. 60, 3531−3544. doi: 10.1093/jxb/erp194
Gyaneshwar, P., Naresh Kumar, G., Parekh, L. J., Poole, P. S. (2002). Role of soil microorganisms in improving P nutrition of plants. Plant Soil 245, 83−93. doi: 10.1023/A:1020663916259
Hafez, Y., Attia, K., Alamery, S., Ghazy, A., Al-Doss, A., Ibrahim, E., et al. (2020). Beneficial effects of biochar and chitosan on antioxidative capacity, osmolytes accumulation, and anatomical characters of water-stressed barley plants. Agronomy 10, 630. doi: 10.3390/agronomy10050630
Haider, F. U., Coulter, J. A., Liqun, C. A. I., Hussain, S., Cheema, S. A., Jun, W. U., et al. (2022). An overview on biochar production, its implications, and mechanisms of biochar-induced amelioration of soil and plant characteristics. Pedosphere 32, 107−130. doi: 10.1016/S1002-0160(20)60094-7
Hameeda, B., Harini, G., Rupela, O. P., Wani, S. P., Reddy, G. (2008). Growth promotion of maize by phosphate-solubilizing bacteria isolated from composts and macrofauna. Microbiol. Res. 163, 234−242. doi: 10.1016/j.micres.2006.05.009
Hamid, B., Zaman, M., Farooq, S., Fatima, S., Sayyed, R. Z., Baba, Z. A., et al. (2021). Bacterial plant biostimulants : A sustainable way towards improving growth, productivity, and health of crops. Sustainability 13, 2856. doi: 10.3390/su13052856
Harb, A., Simpson, C., Guo, W., Govindan, G., Kakani, V. G., Sunkar, R. (2020). The effect of drought on transcriptome and hormonal profiles in barley genotypes with contrasting drought tolerance. Front. Plant Sci. 11. doi: 10.3389/fpls.2020.618491
Hasanuzzaman, M., Bhuyan, M. B., Zulfiqar, F., Raza, A., Mohsin, S. M., Mahmud, J. A., et al. (2020). Reactive oxygen species and antioxidant defense in plants under abiotic stress : Revisiting the crucial role of a universal defense regulator. Antioxidants 9, 681. doi: 10.3390/antiox9080681
Hasanuzzaman, M., Nahar, K., Anee, T. I., Khan, M. I. R., Fujita, M. (2018). Silicon-mediated regulation of antioxidant defense and glyoxalase systems confers drought stress tolerance in Brassica napus L. South Afr. J. Bot. 115, 50−57. doi: 10.1016/j.sajb.2017.12.006
Hasanuzzaman, M., Shabala, L., Brodribb, T. J., Zhou, M., Shabala, S. (2019). Understanding physiological and morphological traits contributing to drought tolerance in barley. J. Agron. Crop Sci. 205, 129−140. doi: 10.1111/jac.2019.205.issue-2
Hebbache, H., Benkherbache, N., Bouchakour, M., Mefti, M. (2021). Effect of water deficit stress on physiological traits of some Algerian barley genotypes. J. Cent. Eur. Agric. 22, 295−304. doi: 10.5513/JCEA01/22.2.3073
Hellal, F., Abdel-Hady, M., Khatab, I., El-Sayed, S., Abdelly, C. (2019). Yield characterization of Mediterranean barley under drought stress condition. AIMS Agric. Food 4, 518−533. doi: 10.3934/agrfood.2019.3.518
Hellal, F. A., El-Shabrawi, H. M., Abd El-Hady, M., Khatab, I. A., El-Sayed, S. A. A., Abdelly, C. (2018). Influence of PEG induced drought stress on molecular and biochemical constituents and seedling growth of Egyptian barley cultivars. J. Genet. Eng. Biotechnol. 16, 203−212. doi: 10.1016/j.jgeb.2017.10.009
Hussain, S., Wang, J., Naseer, M. A., Saqib, M., Siddiqui, M. H., Ihsan, F., et al. (2023). Water stress memory in wheat/maize intercropping regulated photosynthetic and antioxidative responses under rainfed conditions. Sci. Rep. 13, 13688. doi: 10.1038/s41598-023-40644-1
Idris, O. A., Opute, P., Orimoloye, I. R., Maboeta, M. S. (2022). Climate change in Africa and vegetation response : A bibliometric and spatially based information assessment. Sustainability 14, 4974. doi: 10.3390/su14094974
Ikram ul Haq, M., Maqbool, M. M., Ali, A., Farooq, S., Khan, S., Saddiq, M. S., et al. (2020). Optimizing planting geometry for barley-Egyptian clover intercropping system in semi-arid sub-tropical climate. PloS One 15, e0233171. doi: 10.1371/journal.pone.0233171
Ilyas, N., Mumtaz, K., Akhtar, N., Yasmin, H., Sayyed, R. Z., Khan, W., et al. (2020). Exopolysaccharides producing bacteria for the amelioration of drought stress in wheat. Sustainability 12, 8876. doi: 10.3390/su12218876
Iqbal, B., Li, G., Alabbosh, K. F., Hussain, H., Khan, I., Tariq, M., et al. (2023). Advancing environmental sustainability through microbial reprogramming in growth improvement, stress alleviation, and phytoremediation. Plant Stress, 100283. doi: 10.1016/j.stress.2023.100283
Istanbuli, T., Baum, M., Touchan, H., Hamwieh, A. (2020). Evaluation of morpho-physiological traits under drought stress conditions in barley (Hordeum vulgare L.). Photosynthetica 58, 1059−1067. doi: 10.32615/ps.2020.041
Izanloo, A., Condon, A. G., Langridge, P., Tester, M., Schnurbusch, T. (2008). Different mechanisms of adaptation to cyclic water stress in two South Australian bread wheat cultivars. J. Exp. Bot. 59, 3327−3346. doi: 10.1093/jxb/ern199
Jabbari, M., Fakheri, B. A., Aghnoum, R., Mahdi Nezhad, N., Ataei, R. (2018). GWAS analysis in spring barley (Hordeum vulgare L.) for morphological traits exposed to drought. PloS One 13, e0204952. doi: 10.1371/journal.pone.0204952
Jabbari, M., Fakheri, B. A., Aghnoum, R., Nezhad, N. M., Ataei, R., Koochakpour, Z. (2019). Association mapping of morphological and physiological traits of flag leaf related to drought tolerance in barley. Rev. Agricult. Neotrop. 6, 7−18. doi: 10.32404/rean.v6i2.3323
Jabborova, D., Annapurna, K., Azimov, A., Tyagi, S., Pengani, K. R., Sharma, S., et al. (2022). Co-inoculation of biochar and arbuscular mycorrhizae for growth promotion and nutrient fortification in soybean under drought conditions. Front. Plant Sci. 13. doi: 10.3389/fpls.2022.947547
Jabborova, D., KanNepalli, A., Davranov, K., Narimanov, A., Enakiev, Y., Syed, A., et al. (2021). Co-inoculation of rhizobacteria promotes growth, yield, and nutrient contents in soybean and improves soil enzymes and nutrients under drought conditions. Sci. Rep. 11, 22081. doi: 10.1038/s41598-021-01337-9
Jafari, A., Paknejad, F., JAMI, A. M. (2009). Evaluation of selection indices for drought tolerance of corn (Zea mays L.) hybrids. 3 (4), 33–38.
Jahan, E., Sharwood, R. E., Tissue, D. T. (2023). Effects of leaf age during drought and recovery on photosynthesis, mesophyll conductance and leaf anatomy in wheat leaves. Front. Plant Sci. 14, 1091418. doi: 10.3389/fpls.2023.1091418
Jalal, J., Razieh, K., Edris, K. (2014). Improving of barley seedling growth by seed priming under water deficit stress. J. Stress Physiol. Biochem. 10, 125−134.
Jamieson, P. D., Martin, R. J., Francis, G. S. (1995). Drought influences on grain yield of barley, wheat, and maize. New Z. J. Crop Hortic. Sci. 23, 55−66. doi: 10.1080/01140671.1995.9513868
Jedmowski, C., Ashoub, A., Brüggemann, W. (2013). Reactions of Egyptian landraces of Hordeum vulgare and Sorghum bicolor to drought stress, evaluated by the OJIP fluorescence transient analysis. Acta Physiol. Plant. 35, 345−354. doi: 10.1007/s11738-012-1077-9
Jodeh, S., Alkowni, R., Hamed, R., Samhan, S. (2015). The study of electrolyte leakage from barley (Hordeum vulgare L) and pearlmillet using plant growth promotion (PGPR) and reverse osmosis. J. Food Nutr. Res. 3, 422−429. doi: 10.12691/jfnr-3-7-3
Jorenush, M. H., Rajabi, M. (2015). Effect of drought and salinity tensions on germination and seedling growth of Artichoke (Cynara scolymus L.). Int. J. Adv. Biol. Biom. Res. 3, 297−302.
Jouyban, A., Give, H. S., Noryan, M. (2015). Relationship between agronomic and morphological traits in barley varieties under drought stress condition. Intl. Res. J. Appl. Basic. Sci. 9, 1507−1511.
Kaboosi, E., Rahimi, A., Abdoli, M., Ghabooli, M. (2023). Comparison of serendipita indica inoculums and a commercial biofertilizer effects on physiological characteristics and antioxidant capacity of maize under drought stress. J. Soil Sci. Plant Nutr. 23, 900−911. doi: 10.1007/s42729-022-01091-5
Kadam, N. N., Xiao, G., Melgar, R. J., Bahuguna, R. N., Quinones, C., Tamilselvan, A., et al. (2014). Agronomic and physiological responses to high temperature, drought, and elevated CO2 interactions in cereals. Adv. Agron. 127, 111−156. doi: 10.1016/B978-0-12-800131-8.00003-0
Kang, B. R., Anderson, A. J., Kim, Y. C. (2018). Hydrogen cyanide produced by pseudomonas chlororaphis O6 exhibits nematicidal activity against meloidogyne hapla. Plant Pathol. J. 34, 35−43. doi: 10.5423/PPJ.OA.06.2017.0115
Kapadia, C., Sayyed, R. Z., El Enshasy, H. A., Vaidya, H., Sharma, D., Patel, N., et al. (2021). Halotolerant microbial consortia for sustainable mitigation of salinity stress, growth promotion, and mineral uptake in tomato plants and soil nutrient enrichment. Sustainability 13, 8369. doi: 10.3390/su13158369
Karunarathne, S., Walker, E., Sharma, D., Li, C., Han, Y. (2023). Genetic resources and precise gene editing for targeted improvement of barley abiotic stress tolerance. J. Zhejiang University-SCIENCE B 24, 1069−1092. doi: 10.1631/jzus.B2200552
Kausar, R., Arshad, M., Shahzad, A., Komatsu, S. (2013). Proteomics analysis of sensitive and tolerant barley genotypes under drought stress. Amino Acids 44, 345−359. doi: 10.1007/s00726-012-1338-3
Kaymak, H. C. (2010). “Potential of PGPR in agricultural innovations,” in Plant Growth and Health Promoting Bacteria. Ed. Maheshwari, D. K. (Springer, Berlin, Heidelberg: Springer-V). doi: 10.1007/978-3-642-13612-2
Kebede, H., Liu, X., Jin, J., Xing, F. (2020). Current status of major mycotoxins contamination in food and feed in Africa. Food Control 110, 106975. doi: 10.1016/j.foodcont.2019.106975
Khafagy, M. A., Mohamed, Z. A. A., Farouk, S., Amrajaa, H. K. (2017). Effect of pre-treatment of barley grain on germination and seedling growth under drought stress. Adv. Appl. Sci. 2, 33−42. doi: 10.11648/j.aas.20170203.12
Khalili, M., Pour-Aboughadareh, A., Naghavi, M. R. (2016). Assessment of drought tolerance in barley : Integrated selection criterion and drought tolerance indices. Environ. Exp. Biol. 14.
Khan, A., Singh, P., Srivastava, A. (2018). Synthesis, nature and utility of universal iron chelator – Siderophore : A review. Microbiol. Res. 212−213, 103−111. doi: 10.1016/j.micres.2017.10.012
Khan, M. N., Zhang, J., Luo, T., Liu, J., Ni, F., Rizwan, M., et al. (2019). Morpho-physiological and biochemical responses of tolerant and sensitive rapeseed cultivars to drought stress during early seedling growth stage. Acta Physiol. Plant. 41, 1−13. doi: 10.1007/s11738-019-2812-2
Kirkby, E. A., Nikolic, M., White, P. J., Xu, G. (2023). “Mineral nutrition, yield, and source–sink relationships,” in Marschner’s mineral nutrition of plants (Netherlands: Elsevier), 131–200.
Kumar, A., Naroju, S. P., Kumari, N., Arsey, S., Kumar, D., Gubre, D. F., et al. (2024). The role of drought response genes and plant growth promoting bacteria on plant growth promotion under sustainable agriculture: A review. Microbiol. Res., 127827. doi: 10.1016/j.micres.2024.127827
Kumar, A., Patel, J. S., Meena, V. S., Srivastava, R. (2019). Recent advances of PGPR based approaches for stress tolerance in plants for sustainable agriculture. Biocatal. Agric. Biotechnol. 20, 101271. doi: 10.1016/j.bcab.2019.101271
Lamaoui, M., Jemo, M., Datla, R., Bekkaoui, F. (2018). Heat and drought stresses in crops and approaches for their mitigation. Front. Chem. 6, 26. doi: 10.3389/fchem.2018.00026
Lemfack, M. C., Nickel, J., Dunkel, M., Preissner, R., Piechulla, B. (2014). MVOC: A database of microbial volatiles. Nucleic Acids Res. 42, 744−748. doi: 10.1093/nar/gkt1250
Li, L., Wang, Y. (2023). Independent and combined influence of drought stress and nitrogen deficiency on physiological and proteomic changes of barley leaves. Environ. Exp. Bot. 210, 105346. doi: 10.1016/j.envexpbot.2023.105346
Li, Y., Ye, W., Wang, M., Yan, X. (2009). Climate change and drought : A risk assessment of crop-yield impacts. Climate Res. 39, 31−46. doi: 10.3354/cr00797
Liang, X., Kayler, Z., Von Rein, I., Shaber, J., Sapkota, B. (2023). Barley–pulse intercropping improves resource use efficiency and soil health. Crops Soils 56, 10−13. doi: 10.1002/crso.20324
Lima, J. V., Tinoco, R. S., Olivares, F. L., de Moraes, A. J. G., Chia, G. S., da Silva, G. B. (2020). Hormonal imbalance triggered by rhizobacteria enhance nutrient use efficiency and biomass in oil palm. Sci. Hortic. 264, 109161. doi: 10.1016/j.scienta.2019.109161
Loutfy, N., El-Tayeb, M. A., Hassanen, A. M., Moustafa, M. F. M., Sakuma, Y., Inouhe, M. (2012). Changes in the water status and osmotic solute contents in response to drought and salicylic acid treatments in four different cultivars of wheat (Triticum aestivum). J. Plant Res. 125, 173−184. doi: 10.1007/s10265-011-0419-9
Lugojan, C., Ciulca, S. (2011). Evaluation of relative water content in winter wheat. J. Horticult. Forest. Biotechnol. 15, 173−177.
Luqman, M., Shahbaz, M., Maqsood, M. F., Farhat, F., Zulfiqar, U., Siddiqui, M. H., et al. (2023). Effect of strigolactone on growth, photosynthetic efficiency, antioxidant activity, and osmolytes accumulation in different maize (Zea mays L.) hybrids grown under drought stress. Plant Signaling Behav. 18, 2262795. doi: 10.1080/15592324.2023.2262795
Ma, J., Li, Y., Chen, F., Sun, Y., Zhu, Y., Wang, L. (2023). Bacillus mycoides PM35 in combination with titanium dioxide (TiO2)-nanoparticles enhanced morpho-physio-biochemical attributes in Barley (Hordeum vulgare L.) under cadmium stress. Chemosphere 323, 138224. doi: 10.1016/j.chemosphere.2023.138224
Mahalingam, R. (2017). Phenotypic, physiological and malt quality analyses of US barley varieties subjected to short periods of heat and drought stress. J. Cereal Sci. 76, 199−205. doi: 10.1016/j.jcs.2017.06.007
Mahboob, W., Rizwan, M., Irfan, M., Hafeez, O. B. A., Sarwar, N., Akhtar, M., et al. (2023). Salinity tolerance in wheat: responses, mechanisms and adaptation approaches. Appl. Ecol. Environ. Res. 21, 5299–5328. doi: 10.15666/aeer/2106_52995328
Mahmood, N. S., Ahmad, M., Zahir, Z. A., Javaid, A., Ashraf, M. (2014). The role of mycorrhizae and plant growth promoting rhizobacteria (PGPR) in improving crop productivity under stressful environments. Biotechnol. Adv., 429−448. doi: 10.1016/j.biotechadv.2013.12.005
Mahmoud, O. M., Hidri, R., Taamalli, W., Abdelly, C. (2020). Auxin and proline producing rhizobacteria mitigate salt-induced growth inhibition of barley plants by enhancing water and nutrient status. South Afr. J. Bot. 128, 209−217. doi: 10.1016/j.sajb.2019.10.023
Maleki Farahani, S., Mazaheri, D., Chaichi, M., Tavakkol Afshari, R., Savaghebi, G. (2010). Effect of seed vigour on stress tolerance of barley (Hordeum vulgare) seed at germination stage. Seed Sci. Technol. 38, 494–507. doi: 10.15258/sst.2010.38.2.21
Mansour, E., Abdul-Hamid, M. I., Yasin, M. T., Qabil, N., Attia, A. (2017). Identifying drought-tolerant genotypes of barley and their responses to various irrigation levels in a Mediterranean environment. Agric. Water Manage. 194, 58−67. doi: 10.1016/j.agwat.2017.08.021
Marcińska, I., Czyczyło-Mysza, I., Skrzypek, E., Filek, M., Grzesiak, S., Grzesiak, M. T., et al. (2013). Impact of osmotic stress on physiological and biochemical characteristics in drought-susceptible and drought-resistant wheat genotypes. Acta Physiol. Plant. 35, 451−461. doi: 10.1007/s11738-012-1088-6
Martínez-Fernández, D., Barroso, D., Komárek, M. (2016). Root water transport of Helianthus annuus L. under iron oxide nanoparticle exposure. Environ. Sci. pollut. Res. 23, 1732−1741. doi: 10.1007/s11356-015-5423-5
Martínez-López, J. A., López-Urrea, R., Martínez-Romero, Á., Pardo, J. J., Montero, J., Domínguez, A. (2022). Sustainable production of barley in a water-scarce mediterranean agroecosystem. Agronomy 12, 1358. doi: 10.3390/agronomy12061358
Matse, D. T., Huang, C. H., Huang, Y. M., Yen, M. Y. (2020). Effects of coinoculation of Rhizobium with plant growth promoting rhizobacteria on the nitrogen fixation and nutrient uptake of Trifolium repens in low phosphorus soil. J. Plant Nutr. 43, 739−752. doi: 10.1080/01904167.2019.1702205
Mawar, R., Ranawat, M., Ram, L., Sayyed, R. Z. (2023). “Harnessing Drought-Tolerant PGPM in Arid Agroecosystem for Plant Disease Management and Soil Amelioration,” in Plant Growth Promoting Microorganisms of Arid Region. Eds. Mawar, R., Sayyed, R. Z., Sharma, S. K., Sattiraju, K. S. (Springer, Singapore: Springer Nature), 27−43. doi: 10.1007/978-981-19-4124-5_2
Mbai, F., Magiri, E., Matiru, V. (2013). “Isolation and Characterisation of Bacterial Root Endophytes with Potential to Enhance Plant Growth from Kenyan Basmati Rice,” in Aijcrnet.Com. 3 (4), 25–40.
McCaig, T. N., Clarke, J. M. (1982). Seasonal changes in nonstructural carbohydrate levels of wheat and oats grown in a semiarid environment1. Crop Sci. 22. doi: 10.2135/cropsci1982.0011183X002200050016x
Mhlongo, M. I., Piater, L. A., Madala, N. E., Labuschagne, N., Dubery, I. A. (2018). The chemistry of plant–microbe interactions in the rhizosphere and the potential for metabolomics to reveal signaling related to defense priming and induced systemic resistance. Front. Plant Sci. 9. doi: 10.3389/fpls.2018.00112
Mishra, V., Ganguly, A. R., Nijssen, B., Lettenmaier, D. P. (2015). Changes in observed climate extremes in global urban areas. Environ. Res. Lett. 10, 024005. doi: 10.1088/1748-9326/10/2/024005
Moaveni, P., Talebi, A., Farahani, H. A., Maroufi, K. (2011). Study of Ti [O. sub. 2] nano particles spraying effect on the some physiological parameters in barley (Hordem vulgare L.). Adv. Environ. Biol., 1663−1668.
Moayedi, A. A., Boyce, A. N., Barakbah, S. S. (2009). Study on osmotic stress tolerance in promising durum wheat genotypes using drought stress indices. Res. J. Agric. Biol. Sci. 5, 603−607.
Mohammadi, R. (2020). The use of a combination scoring index to improve durum productivity under drought stress. Exp. Agric. 56, 161–170. doi: 10.1017/S0014479719000231
Molénat, J., Barkaoui, K., Benyoussef, S., Mekki, I., Zitouna, R., Jacob, F. (2023). Diversification from field to landscape to adapt Mediterranean rainfed agriculture to water scarcity in climate change context. Curr. Opin. Environ. Sustain. 65, 101336. doi: 10.1016/j.cosust.2023.101336
Mondini, L., Pagnotta, M. A. (2015). “Drought and salt stress in cereals,” in Sustainable agriculture reviews, vol. 1−31. (Cham: Springer).
Moulick, R. G., Das, S., Debnath, N., Bandyopadhyay, K. (2020). Potential use of nanotechnology in sustainable and ‘smart’ agriculture : Advancements made in the last decade. Plant Biotechnol. Rep. 14, 505−513. doi: 10.1007/s11816-020-00636-3
Mukarram, M., Choudhary, S., Kurjak, D., Petek, A., Khan, M. M. A. (2021). Drought : Sensing, signalling, effects and tolerance in higher plants. Physiol. Plant. 172, 1291−1300. doi: 10.1111/ppl.13423
Murtaza, G., Rasool, F., Habib, R., Javed, T., Sardar, K., Ayub, M. M., et al. (2016). A review of morphological, physiological and biochemical responses of plants under drought stress conditions. Imp. J. Interdiscip. Res. 2, 1600−1606.
Mutengwa, C. S., Mnkeni, P., Kondwakwenda, A. (2023). Climate-smart agriculture and food security in Southern Africa : A review of the vulnerability of smallholder agriculture and food security to climate change. Sustainability 15, 2882. doi: 10.3390/su15042882
Naing, A. H., Maung, T., Kim, C. K. (2021). The ACC deaminase-producing plant growth-promoting bacteria : Influences of bacterial strains and ACC deaminase activities in plant tolerance to abiotic stress. Physiol. Plant. 173, 1992−2012. doi: 10.1111/ppl.13545
Najafi, S., Nazari Nasi, H., Tuncturk, R., Tuncturk, M., Sayyed, R. Z., Amirnia, R. (2021). Biofertilizer Application Enhances Drought Stress Tolerance and Alters the Antioxidant Enzymes in Medicinal Pumpkin (Cucurbita pepo convar. Pepo var. Styriaca). Horticulturae 7, 588. doi: 10.3390/horticulturae7120588
Nasiri, S., Andalibi, B., Tavakoli, A., Delavar, M. A., Van Zwieten, L. (2023). The effect of biochar and methyl Jasmonate on biochemical alterations, yield and yield components of barley (Hordeum vulgare L.) under drought stress. Environ. Stresses Crop Sci. doi: 10.22077/escs.2023.5520.2154
Nayana, R. U. K., Nakkeeran, S., Saranya, N., Saravanan, R., Mahendra, K., Ashraf, S., et al. (2023). Triamcinolone Acetonide Produced by Bacillus velezensis YEBBR6 Exerts Antagonistic Activity Against Fusarium oxysporum f. sp. Cubense : A Computational Analysis. Mol. Biotechnol. doi: 10.1007/s12033-023-00797-w
Nazari, L., Pakniyat, H. (2010). Assessment of drought tolerance in barley genotypes. J. Appl. Sci. 10, 151−156. doi: 10.3923/jas.2010.151.156
Nazim, M., Fatima, M., Hussain, A., Ali, M., Mathpal, B., Alwahibi, M. S. (2024). Salt stress effects on growth, physiology, and ionic concentrations in hydroponically grown barley genotypes. J. King Saud University-Science 36, 103448. doi: 10.1016/j.jksus.2024.103448
Neerincx, A. H., Mandon, J., Van Ingen, J., Arslanov, D. D., Mouton, J. W., Harren, F. J. M., et al. (2015). Real-time monitoring of hydrogen cyanide (HCN) and ammonia (NH3) emitted by Pseudomonas aeruginosa. J. Breath Res. 9. doi: 10.1088/1752-7155/9/2/027102
Niewiadomska, A., Sulewska, H., Wolna-Maruwka, A., Ratajczak, K., Głuchowska, K., Waraczewska, Z., et al. (2018). An assessment of the influence of co-inoculation with endophytic bacteria and rhizobia, and the influence of PRP SOL and PRP EBV fertilisers on the microbial parameters of soil and nitrogenase activity in yellow lupine (Lupinus luteus L.) cultivation. Polish J. Environ. Stud. 27, 2687−2702. doi: 10.15244/pjoes/78890
Noman, A., Ali, Q., Naseem, J., Javed, M. T., Kanwal, H., Islam, W., et al. (2018). Sugar beet extract acts as a natural bio-stimulant for physio-biochemical attributes in water stressed wheat (Triticum aestivum L.). Acta Physiol. Plant. 40, 110. doi: 10.1007/s11738-018-2681-0
Orhan, E., Esitken, A., Ercisli, S., Sahin, F. (2007). Effects of indole-3-butyric acid (IBA), bacteria and radicle tip- cutting on lateral root induction in Pistacia vera. J. Hortic. Sci. Biotechnol. 82, 2−4. doi: 10.1080/14620316.2007.11512190
Osakabe, Y., Osakabe, K., Shinozaki, K., Tran, L.-S. P. (2014). Response of plants to water stress. Front. Plant Sci. 5, 76566. doi: 10.3389/fpls.2014.00086
Pakzad, R., Fatehi, F., Kalantar, M., Maleki, M. (2019). Evaluating the antioxidant enzymes activities, lipid peroxidation and proteomic profile changing in UCB-1 pistachio rootstock leaf under drought stress. Sci. Hortic. 256, 108617. doi: 10.1016/j.scienta.2019.108617
Pan, R., Ding, M., Feng, Z., Zeng, F., Medison, M. B., Hu, H., et al. (2022). HvGST4 enhances tolerance to multiple abiotic stresses in barley : Evidence from integrated meta-analysis to functional verification. Plant Physiol. Biochem. 188, 47−59. doi: 10.1016/j.plaphy.2022.07.027
Peix, A., Rivas-Boyero, A. A., Mateos, P. F., Rodriguez-Barrueco, C., Martínez-Molina, E., Velazquez, E. (2001). Growth promotion of chickpea and barley by a phosphate solubilizing strain of Mesorhizobium mediterraneum under growth chamber conditions. Soil Biol. Biochem. 33, 103−110. doi: 10.1016/S0038-0717(00)00120-6
Podile, A., Kishore, G. (2006). Plant growth-promoting rhizobacteria. Plant-associated bacteria, 195−230. doi: 10.1007/978-1-4020-4538-7_6
Qiao, M., Hong, C., Jiao, Y., Hou, S., Gao, H. (2024). Impacts of drought on photosynthesis in major food crops and the related mechanisms of plant responses to drought. Plants 13, 1808. doi: 10.3390/plants13131808
Queiroz, M. S., Oliveira, C. E., Steiner, F., Zuffo, A. M., Zoz, T., Vendruscolo, E. P., et al. (2019). Drought stresses on seed germination and early growth of maize and sorghum. J. Agric. Sci. 11, 310. doi: 10.5539/jas.v11n2p310
Rafique, N., Ilyas, N., Aqeel, M., Raja, N. I., Shabbir, G., Ajaib, M., et al. (2023). Interactive effects of melatonin and salicylic acid on Brassica napus under drought condition. Plant Soil. doi: 10.1007/s11104-023-05942-7
Rahman, M. M., Flory, E., Koyro, H. W., Abideen, Z., Schikora, A., Suarez, C., et al. (2018). Consistent associations with beneficial bacteria in the seed endosphere of barley (Hordeum vulgare L.). System. Appl. Microbiol. 41, 386−398. doi: 10.1016/j.syapm.2018.02.003
Rajasekar, M., Rabert, G. A., Manivannan, P. (2015). Triazole induced changes on biochemical and antioxidant metabolism of Zea mays L.(Maize) under drought stress. J. Plant Stress Physiol. 1, 35−42. doi: 10.5455/jpsp.2015-08-024
Raklami, A., Tahiri, A., Bechtaoui, N., Pajuelo, E., Baslam, M., Meddich, A., et al. (2021). Restoring the plant productivity of heavy metal-contaminated soil using phosphate sludge, marble waste, and beneficial microorganisms. J. Environ. Sci. 99, 210−221. doi: 10.1016/j.jes.2020.06.032
Rebelo Romão, I., Rodrigues dos Santos, A. S., Velasco, L., Martínez-Ferri, E., Vilchez, J. I., Manzanera, M. (2022). Seed-encapsulation of desiccation-tolerant microorganisms for the protection of maize from drought : phenotyping effects of a new dry bioformulation. Plants 11, 1024. doi: 10.3390/plants11081024
Rebi, A., Kashif, H. M., Chaudhry, U. F., Zaib, M., Shahid, M. Z., Safdar, M., et al. (2022). Phosphorus availability in soil and uptake by maize from rock phosphate inoculated with PGPR : A review. Nveo-Natural Volatiles Essential Oils J. 9 (1), 341–355.
Reynolds, M. P., Ortiz, R. (2010). Adapting crops to climate change : A summary. Climate Change Crop product. 1, 1. doi: 10.1079/9781845936334.0000
Rezaei, E., Miri, M. R., Shamsaee, H. R., Sepehri, M. H., Golzardi, F. (2017). Effect of cumulative application of nitrogen and PGPR on the various traits of barley (Hordeum vulgare L.) under drought stress conditions. J. Exp. Biol. Agric. Sci. 5 (1), 302−308. doi: 10.18006/2017.5(3).302.308
Rezaei, E., Pazoki, A. (2015). Study the effect of nitrogen fertilizers and Plant Growth Promoting Rhizobacteria (PGPR) for protein yield, relative water content, cell membrane stability and chlorophyll content in barley under drought stress. Adv. Biomed. Res. 6, 119−122.
Reza Yousefi, A., Rashidi, S., Moradi, P., Mastinu, A. (2020). Germination and Seedling Growth Responses of Zygophyllum fabago, Salsola kali L. and Atriplex canescens to PEG-Induced Drought Stress. Environments 7, 107. doi: 10.3390/environments7120107
Rodrigues, A. P., Pais, I. P., Leitão, A. E., Dubberstein, D., Lidon, F. C., Marques, I., et al. (2024). Uncovering the wide protective responses in Coffea spp. leaves to single and superimposed exposure of warming and severe water deficit. Front. Plant Sci. 14, 1320552. doi: 10.3389/fpls.2023.1320552
Saed-Moucheshi, A., Pessarakli, M., Mozafari, A., Sohrabi, F., Moradi, M., Marvasti, F. (2021). Screening barley varieties tolerant to drought stress based on tolerant indices. J. Plant Nutr. 1−12, 739–750. doi: 10.1080/01904167.2021.1963773
Sagar, A., Rai, S., Sharma, S., Perveen, K., Bukhari, N. A., Sayyed, R. Z., et al. (2024). Molecular characterization reveals biodiversity and biopotential of rhizobacterial isolates of bacillus spp. Microb. Ecol. 87, 83. doi: 10.1007/s00248-024-02397-w
Sagar, A., Sayyed, R. Z., Ramteke, P. W., Sharma, S., Marraiki, N., Elgorban AM and Syed, A. (2020). ACC deaminase and antioxidant enzymes producing halophilic Enterobacter sp. PR14 promotes the growth of rice and millets under salinity stress. Physiol. Mol. Biol. Plants. 26, 1847–1854. doi: 10.1007/s12298-020-00852-9
Sallam, A., Alqudah, A. M., Dawood, M. F., Baenziger, P. S., Börner, A. (2019). Drought stress tolerance in wheat and barley : Advances in physiology, breeding and genetics research. Int. J. Mol. Sci. 20, 3137. doi: 10.3390/ijms20133137
Samarah, N. H. (2005). Effects of drought stress on growth and yield of barley. Agron. Sustain. Dev. 25, 145−149. doi: 10.1051/agro:2004064
Samarah, N., Alqudah, A., Amayreh, J., GM, M. (2009). The effect of late-terminal drought stress on yield components of four barley cultivars. J. Agron. Crop Sci. 195, 427−441. doi: 10.1111/j.1439-037X.2009.00387.x
Sanders, G. J., Arndt, S. K. (2012). “Osmotic Adjustment Under Drought Conditions,” in Plant Responses to Drought Stress. Ed. Aroca, R. (Berlin Heidelberg: Springer ), 199−229. doi: 10.1007/978-3-642-32653-0_8
Santos, M. S., Nogueira, M. A., Hungria, M. (2019). Microbial inoculants : Reviewing the past, discussing the present and previewing an outstanding future for the use of beneficial bacteria in agriculture. AMB Express 9, 205. doi: 10.1186/s13568-019-0932-0
Sardans, J., Peñuelas, J., Ogaya, R. (2008). Drought’s impact on Ca, Fe, Mg, Mo and S concentration and accumulation patterns in the plants and soil of a Mediterranean evergreen Quercus ilex forest. Biogeochemistry 87, 49–69. doi: 10.1007/s10533-007-9167-2
Sazegari, S., Zinati, Z., Tahmasebi, A. (2020). Dynamic transcriptomic analysis uncovers key genes and mechanisms involved in seed priming-induced tolerance to drought in barley. Gene Rep. 21, 100941. doi: 10.1016/j.genrep.2020.100941
Schoppach, R., Fleury, D., Sinclair, T. R., Sadok, W. (2017). Transpiration sensitivity to evaporative demand across 120 years of breeding of Australian wheat cultivars. J. Agron. Crop Sci. 203, 219−226. doi: 10.1111/jac.12193
Sharma, P., Jha, A. B., Dubey, R. S. (2024). Addressing lanthanum toxicity in plants: Sources, uptake, accumulation, and mitigation strategies. Sci. Total Environ., 172560. doi: 10.1016/j.scitotenv.2024.172560
Sharma, A., Johri, B. N. (2003). Growth promoting influence of siderophore-producing Pseudomonas strains GRP3A and PRS9 in maize (Zea mays L.) under iron limiting conditions. Microbiol. Res. 158, 243−248. doi: 10.1078/0944-5013-00197
Sharma, P., Sareen, S., Saini, M. (2017). Assessing genetic variation for heat stress tolerance in Indian bread wheat genotypes using morpho-physiological traits and molecular markers. Plant Genet. Resour. 15, 539−547. doi: 10.1017/S1479262116000241
Shi, J., Yasuor, H., Yermiyahu, U., Zuo, Q., Ben-Gal, A. (2014). Dynamic responses of wheat to drought and nitrogen stresses during re-watering cycles. Agric. Water Manage. 146, 163−172. doi: 10.1016/j.agwat.2014.08.006
Siddiqui, M. N., Léon, J., Naz, A. A., Ballvora, A. (2021). Genetics and genomics of root system variation in adaptation to drought stress in cereal crops. J. Exp. Bot. 72, 1007−1019. doi: 10.1093/jxb/eraa487
Slimani, A., Oufdou, K., Meddich, A. (2023a). Intercropping and co-inoculation of beneficial microorganisms of soils improve drought tolerance in barley and alfalfa plants. Gesunde Pflanzen. doi: 10.1007/s10343-023-00949-7
Slimani, A., Raklami, A., Oufdou, K., Meddich, A. (2023b). Isolation and characterization of PGPR and their potenzial for drought alleviation in barley plants. Gesunde Pflanzen 75, 377−391. doi: 10.1007/s10343-022-00709-z
Song, M. C., Beom, R. K., Song, H. H., Anderson, A. J., Park, J. Y., Lee, Y. H., et al. (2008). 2R,3R-butanediol, a bacterial volatile produced by Pseudomonas chlororaphis O6, is involved in induction of systemic tolerance to drought in Arabidopsis thaliana. Mol. Plant-Microbe Interact. 21, 1067−1075. doi: 10.1094/MPMI-21-8-1067
Stomph, T., Dordas, C., Baranger, A., de Rijk, J., Dong, B., Evers, J., et al. (2020). Designing intercrops for high yield, yield stability and efficient use of resources : Are there principles? Adv. Agron. 160, 1−50. doi: 10.1016/bs.agron.2019.10.002
Suarez, C., Cardinale, M., Ratering, S., Steffens, D., Jung, S., Montoya, A. M. Z., et al. (2015). Plant growth-promoting effects of Hartmannibacter diazotrophicus on summer barley (Hordeum vulgare L.) under salt stress. Appl. Soil Ecol. 95, 23−30. doi: 10.1016/j.apsoil.2015.04.017
Suryadi, Y., Susilowati, D. N., Fauziah, F. (2019). “Management of plant diseases by PGPR-mediated induced resistance with special reference to tea and rice crops,” in Plant growth Promoting Rhizobacteria for Sustainable Stress Management, vol. 2 . Ed. Sayyed, R. Z. (Singapore: Springer), 56−110.
Tabatabaei, S. A. (2013). Effect of osmo-priming on germination and enzyme activity in barley (Hordeum vulgare L.) seeds under drought stress conditions. J. Stress Physiol. Biochem. 9, 25−31.
Tabatabaei, S. A., Ansari, O. (2020). The effect of priming on germination characteristics of barley seeds under drought stress conditions. Available online at: https://repository.uaiasi.ro/handle/20.500.12811/75.
Talaat, N. B. (2023). Drought stress alleviator melatonin reconfigures water-stressed barley (Hordeum vulgare L.) plants’ Photosynthetic efficiency, antioxidant capacity, and endogenous phytohormone profile. Int. J. Mol. Sci. 24, 16228. doi: 10.3390/ijms242216228
Tang, L., Shi, Y., Zhang, Y., Yang, D., Guo, C. (2023). Effects of plant-growth-promoting rhizobacteria on soil bacterial community, soil physicochemical properties, and soil enzyme activities in the rhizosphere of alfalfa under field conditions. Diversity 15, 537. doi: 10.3390/d15040537
Tanveer, S., Akhtar, N., Ilyas, N., Sayyed, R. Z., Fitriatin, B. N., Perveen, K., et al. (2023). Interactive effects of Pseudomonas putida and salicylic acid for mitigating drought tolerance in canola (Brassica napus L.). Heliyon 9. Available at: https://www.cell.com/heliyon/fulltext/S2405-8440(23)01400-7.
Teulat, B., Zoumarou-Wallis, N., Rotter, B., Ben Salem, M., Bahri, H., This, D. (2003). QTL for relative water content in field-grown barley and their stability across Mediterranean environments. Theor. Appl. Genet. 108, 181–188. doi: 10.1007/s00122-003-1417-7
Thabet, S. G., Moursi, Y. S., Karam, M. A., Börner, A., Alqudah, A. M. (2020). Natural variation uncovers candidate genes for barley spikelet number and grain yield under drought stress. Genes 11, 533. doi: 10.3390/genes11050533
Thabet, S. G., Moursi, Y. S., Karam, M. A., Graner, A., Alqudah, A. M. (2018). Genetic basis of drought tolerance during seed germination in barley. PloS One 13, e0206682. doi: 10.1371/journal.pone.0206682
Tian, H., Zhou, Q., Liu, W., Zhang, J., Chen, Y., Jia, Z., et al. (2022). Responses of photosynthetic characteristics of oat flag leaf and spike to drought stress. Front. Plant Sci. 13, 917528. doi: 10.3389/fpls.2022.917528
Tirry, N., Ferioun, M., Kouchou, A., Laghmari, G., Asri, M., Zouitane, I., et al. (2023). Plant growth-promoting rhizobacteria’s (PGPR) effects on Medicago sativa growth, arbuscular mycorrhizal colonisation, and soil enzyme activities. Int. J. Environ. Stud. 81 (3), 1190–1208. doi: 10.1080/00207233.2023.2216606
Toulotte, J. M., Pantazopoulou, C. K., Sanclemente, M. A., Voesenek, L. A. C. J., Sasidharan, R. (2022). Water stress resilient cereal crops : Lessons from wild relatives. J. Integr. Plant Biol. 64, 412−430. doi: 10.1111/jipb.13222
Turan, M., Gulluce, M., Şahin, F. (2012). Effects of plant-growth-promoting rhizobacteria on yield, growth, and some physiological characteristics of wheat and barley plants. Commun. Soil Sci. Plant Anal. 43, 1658−1673. doi: 10.1080/00103624.2012.681739
Uarrota, V. G., Sayyed, R. Z., Pedreschi, R. (2022). “The Role of PGPR-Secondary Metabolites on Plant Photosynthesis,” in Secondary Metabolites and Volatiles of PGPR in Plant-growth Promotion. Eds. Sayyed, R. Z., Uarrota, V. G. (Springer, Cham), 45–57. doi: 10.1007/978-3-031-07559-9_3
Vaezi, B., Bavei, V., Shiran, B. (2010). Screening of barley genotypes for drought tolerance by agro-physiological traits in field condition. Afr. J. Agric. Res. 5, 881−892.
Vafa, Z. N., Sohrabi, Y., Heidari, G., Rizwan M and Sayyed, R. Z. (2024). Wheat growth and yield response are regulated by mycorrhizae application and supplemental irrigation. Chemosphere 364, 143068. doi: 10.1016/j.chemosphere.2024.143068
Vafa, Z. N., Sohrabi, Y., Sayyed, R. Z., Suriani, N. L., Datta, R. (2021). Effects of combinations of Rhizobacteria, mycorrhizae, and seaweeds on growth and yields in wheat cultivars under the influence of supplementary irrigation. Plants. Available at: https://www.mdpi.com/2223-7747/10/4/811/htm.
van Peer, R. (1991). Induced resistance and phytoalexin accumulation in biological control of fusarium wilt of carnation by pseudomonas sp. Strain WCS417r. Phytopathology 81, 728. doi: 10.1094/phyto-81-728
Velicevici, G., Madosa, E., Ciulca, S., Ciulca, A., Petolescu, C., Bitea, N. (2010). Assessment of drought tolerance in some barley genotypes cultivated in West part of Romania. J. Horticult. Forest. Biotechnol. 14, 114−118.
Vila-Traver, J., Aguilera, E., Infante-Amate, J., de Molina, M. G. (2021). Climate change and industrialization as the main drivers of Spanish agriculture water stress. Sci. Total Environ. 760, 143399. doi: 10.1016/j.scitotenv.2020.143399
Wang, W. X., Vinocur, B., Shoseyov, O., Altman, A. (2000). Biotechnology of plant osmotic stress tolerance physiological and molecular considerations. IV Int. Symp. In Vitro Cult. Hortic. Breed. 560, 285−292. Available at: https://www.actahort.org/books/560/560_54.htm.
Wei, G., Kloepper, J. W., Tuzun, S. (1991). Induced systemic resistance of cucucmber to colletotrichum orbiculare by plant growth promoting Rhizobacteria. 81 (11), 1508–1512. doi: 10.1094/Phyto-81-1508
Wijewardana, C., Reddy, K. R., Bellaloui, N. (2019). Soybean seed physiology, quality, and chemical composition under soil moisture stress. Food Chem. 278, 92−100. doi: 10.1016/j.foodchem.2018.11.035
Williams, H. D., Zlosnik, J. E. A., Ryall, B. (2006). “Oxygen, Cyanide and Energy Generation in the Cystic Fibrosis Pathogen Pseudomonas aeruginosa,” in Advances in Microbial Physiology. Ed(s): Poole, R. K.. 52, 1–71. doi: 10.1016/S0065-2911(06)52001-6
Woldeamlak, A., Kropff, M. J., Struik, P. C. (2006). Effect of drought stress on barley-wheat intercropping. Afr. Crop Sci. J. 14, 185−195.
Xiong, J., Chen, D., Chen, Y., Wu, D., Zhang, G. (2023). Genome-wide association mapping and transcriptomic analysis reveal key drought-responding genes in barley seedlings. Curr. Plant Biol. 33, 100277. doi: 10.1016/j.cpb.2023.100277
Yang, X., Lu, M., Wang, Y., Wang, Y., Liu, Z., Chen, S. (2021). Response mechanism of plants to drought stress. Horticulturae 7, 50. doi: 10.3390/horticulturae7030050
Yang, Y., Thian, Y. G., Xuezhi, T. (2020). Spatiotemporal changes of drought characteristics and their dynamic drivers in Canada. Atmos. Res. 232, 104695. doi: 10.1016/j.atmosres.2019.104695
Yin, W., Chai, Q., Zhao, C., Yu, A., Fan, Z., Hu, F., et al. (2020). Water utilization in intercropping : A review. Agric. Water Manage. 241, 106335. doi: 10.1016/j.agwat.2020.106335
Yin, W., Chen, G., Feng, F., Guo, Y., Hu, F., Chen, G., et al. (2017). Straw retention combined with plastic mulching improves compensation of intercropped maize in arid environment. Field Crops Res. 204, 42−51. doi: 10.1016/j.fcr.2017.01.005
Zaib, S., Ahmad, I., Shakeel, S. N. (2020). ). Modulation of barley (Hordeum vulgare) defense and hormonal pathways by Pseudomonas species accounted for salinity tolerance. Pakistan J. Agric. Sci. 57.
Zali, A. G., Ehsanzadeh, P. (2018). Exogenous proline improves osmoregulation, physiological functions, essential oil, and seed yield of fennel. Ind. Crops Prod. 111, 133−140. doi: 10.1016/j.indcrop.2017.10.020
Zargar, S. M., Gupta, N., Nazir, M., Mahajan, R., Malik, F. A., Sofi, N. R., et al. (2017). Impact of drought on photosynthesis : Molecular perspective. Plant Gene 11, 154−159. doi: 10.1016/j.plgene.2017.04.003
Zhanassova, K., Kurmanbayeva, A., Gadilgereyeva, B., Yermukhambetova, R., Iksat, N., Amanbayeva, U., et al. (2021). ROS status and antioxidant enzyme activities in response to combined temperature and drought stresses in barley. Acta Physiol. Plant. 43, 114. doi: 10.1007/s11738-021-03281-7
Zhang, Z., Rod, M., Hosseinian, F. (2021). A comprehensive review on sustainable industrial vertical farming using film farming technology. Sustain. Agric. Res. 10, 46−53. doi: 10.5539/sar.v10n1p46
Zhang, Y.-J., Yang, J.-S., Guo, S.-J., Meng, J.-J., Zhang, Y.-L., Wan, S.-B., et al. (2011). Over-expression of the Arabidopsis CBF1 gene improves resistance of tomato leaves to low temperature under low irradiance. Plant Biol. 13, 362−367. doi: 10.1111/j.1438-8677.2010.00365.x
Zhao, J., Sun, H., Dai, H., Zhang, G., Wu, F. (2010). Difference in response to drought stress among Tibet wild barley genotypes. Euphytica 172, 395−403. doi: 10.1007/s10681-009-0064-8
Zhu, Y., Wang, L., Ma, J., Li, Y., Chen, F., Peijnenburg, W. (2023). Comparative physiological and metabolomics analyses using Ag-NPs and HAS31 (PGPR) to alleviate Cr stress in barley (Hordeum vulgare L.). Environ. pollut. 333, 122010. doi: 10.1016/j.envpol.2023.122010
Zhu, W., Zhao, D., Di, N., Li, D., Zhou, O., Sun, Y., et al. (2024). Matching root water uptake patterns to fine root and soil water distributions. Plant Soil 495, 499−516. doi: 10.1007/s11104-023-06349-0
Zivcak, M., Brestic, M., Sytar, O. (2016). “Osmotic Adjustment and Plant Adaptation to Drought Stress,” in Drought Stress Tolerance in Plants, vol. 1 . Eds. Hossain, M. A., Wani, S. H., Bhattacharjee, S., Burritt, D. J., Tran, L.-S. P. (Springer, Cham: Springer International Publishing), 105−143. doi: 10.1007/978-3-319-28899-4_5
Keywords: Bio-inoculant; drought stress, genotypes, Hordeum vulgare, microbial biostimulants, PGPR
Citation: Ferioun M, Zouitane I, Bouhraoua S, Elouattassi Y, Belahcen D, Errabbani A, Louahlia S, Sayyed R and El Ghachtouli N (2025) Applying microbial biostimulants and drought-tolerant genotypes to enhance barley growth and yield under drought stress. Front. Plant Sci. 15:1494987. doi: 10.3389/fpls.2024.1494987
Received: 11 September 2024; Accepted: 13 December 2024;
Published: 07 January 2025.
Edited by:
Fernanda Fidalgo, University of Porto, PortugalCopyright © 2025 Ferioun, Zouitane, Bouhraoua, Elouattassi, Belahcen, Errabbani, Louahlia, Sayyed and El Ghachtouli. This is an open-access article distributed under the terms of the Creative Commons Attribution License (CC BY). The use, distribution or reproduction in other forums is permitted, provided the original author(s) and the copyright owner(s) are credited and that the original publication in this journal is cited, in accordance with accepted academic practice. No use, distribution or reproduction is permitted which does not comply with these terms.
*Correspondence: Mohamed Ferioun, bW9oYW1lZC5mZXJpb3VuQHVzbWJhLmFjLm1h; Naïma El Ghachtouli, bmFpbWEuZWxnaGFjaHRvdWxpQHVzbWJhLmFjLm1h