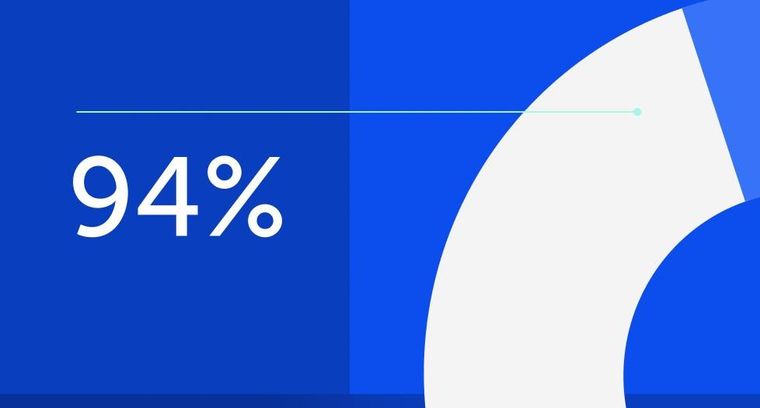
94% of researchers rate our articles as excellent or good
Learn more about the work of our research integrity team to safeguard the quality of each article we publish.
Find out more
ORIGINAL RESEARCH article
Front. Plant Sci., 05 April 2022
Sec. Plant Pathogen Interactions
Volume 13 - 2022 | https://doi.org/10.3389/fpls.2022.860170
This article is part of the Research TopicMolecular Interactions between Crops and Phytopathogens, Volume I: Wheat and MaizeView all 11 articles
Corn sheath blight, caused by AG1-IA, a fusion group of Rhizoctonia solani, which acts as a kind of necrotrophic fungal pathogen, poses a global threat to the production of Zea mays. Although cellulase plays a crucial role in R. solani infections, how plants respond to it is still poorly understood. In this study, we identified a gamma-aminobutyric acid transaminase (GABA-T), ZmGABA-T, in Z. mays that interacts with a cell wall–degrading enzyme (CWDE), EG1, in the cell membrane, using yeast two-hybrid assay, co-immunoprecipitation (Co-IP), and bimolecular fluorescence complementation assays. We found that the combination of EG1 and ZmGABA-T suppressed the allergic necrosis induced by EG1. We also found that the substrate of GABA-T–GABA, can inhibit the transcription of EG1. Transient expression of ZmGABA-T inhibited R. solani infection in Nicotiana benthamiana. The homolog in Oryza sativa, OsGABA-T, could also interact with EG1 to suppress the allergic necrosis induced by EG1. The OsGABA-T knocked out plants displayed enhanced susceptibility to R. solani and showed larger lesions. In conclusion, our results suggest that ZmGABA-T inhibits allergic necrosis induced by EG1 based on the combination with EG1, producing resistance to R. solani infection.
Plants are constantly invaded by pathogens during their development. As a result, both have evolved a complex immune interaction network to fight each other called the “Invasion Model” (Kanyuka and Rudd, 2019). Pathogens are regarded as invasive molecules (IMs), and host receptors are called invasion pattern receptors. IMs are recognized by invasion pattern receptors and respond with apoplast-initiated immune responses and cytosol-initiated immune responses. Pathogen-associated molecular patterns (PAMPs) are typical IMs that induce PAMP-triggered immunity (PTI) via pattern recognition receptors (PRRs), leading to the accumulation of reactive oxygen species (ROS), calcium ion (Ca2+) level elevation, the activation of defense-related genes, and hypersensitive responses (HRs) (Dangl and Jones, 2001; Desaki et al., 2006; Boller and Felix, 2009; Zipfel, 2009). Several studies have reported that some CWDEs, such as xyloglucanase (Ma Z. et al., 2015; Gui et al., 2017; Snarr et al., 2017; Mauff et al., 2019; Shen et al., 2020), endopolygalacturonases (Zhang et al., 2014), and cellulase (Ma Y. et al., 2015; Guo et al., 2021) can act as PAMPs and induce PTI, in which their PAMP activities can be independent of their enzymatic activities.
HRs defend against most pathogens, such as pathogenicity in biotrophic and hemibiotrophic fungi, preventing further development in early infection stages. They comprise a powerful protective mechanism for plants (Stergiopoulos and de Wit, 2009). However, some necrotrophic pathogens, such as Rhizoctonia solani, have been known to kill plants. Necrotrophic fungi kill host cells and obtain nutrients from dead plant tissues. Successful necrotrophic pathogens rely on the secretion of hydrolytic enzymes to macerate and digest plants for their development (Doehlemann et al., 2017). A large number of genes coding for CWDEs and other hydrolytic enzymes present in the genomes of necrotrophic fungi support this notion (Amselem et al., 2011). However, the infection process of necrotrophic pathogens is complex. The burst of ROS, which probably has multiple functions, mediates the life cycle of necrotrophic pathogens. Although they are considered to be a kind of defense response, they also serve as a virulence factor, at least in necrotrophic interactions (Doehlemann et al., 2017). For example, HRs facilitate the infection of plants by Botrytis cinerea (Govrin and Levine, 2000). During Sclerotinia sclerotiorum infection, oxalic acid induces increased ROS levels in plants, which correlates to cell death, thereby promoting infection (Kim et al., 2008).
To resist infection by diverse pathogens, plants have evolved various defense mechanisms. Salicylic acid (SA) contributes to plants’ resistance to biotrophic and hemibiotrophic pathogens, whereas SA, jasmonate (JA), and ethylene (ET) all promote resistance to necrotrophic fungi (Tsuda et al., 2009). The Arabidopsis Botrytis Susceptible1 Interactor (BOI) aids disease resistance through the suppression of necrotrophic pathogens–induced cell death (Luo et al., 2010). Inhibitors of apoptosis proteins (IAPs) have a similar structure and function as BOI in animals (Vaux and Silke, 2005). Transgenic plants expressing baculovirus IAPs suppress cell death and are resistant to necrotrophic fungi (Dickman et al., 2001). A transcriptional repressor of gibberellin (GA) signaling, DELLA protein, promotes resistance to necrotrophic pathogens by altering the relative strength of SA and JA signaling (Navarro et al., 2008). The Arabidopsis pentatricopeptide repeat protein, peptidoglycan (PGN), regulates the ROS dynamic equilibrium in mitochondria to resist B. cinerea infection (Laluk et al., 2011). In contrast, the Arabidopsis BOTRYTIS-SUSCEPTIBLE1 (BOS) mutant is more susceptible to necrotrophic fungi (Mengiste et al., 2003).
Rhizoctonia solani acts as a necrotrophic fungus and harms gramineous crops, posing a severe threat to production. Necrotrophic fungi kill host cells and take nutrients from dead plant tissues to complete their life cycle. During this cycle, they secrete various cell necrosis–causing CWDEs and toxins to achieve a successful infection (Oliver and Solomon, 2010). There are many pathogenic factors in R. solani, such as the effector AGLIP1, which triggers cell death in plants and promotes disease development by inhibiting PTI, such as protection-related (PR) gene expression in Arabidopsis thaliana (Li et al., 2019). The RsRlpA effector is a protease inhibitor promoting the virulence of R. solani through suppression of the ROS burst and HR (Charova et al., 2020). The novel effector RsIA_NP8 in R. solani AG1-IA induces cell death and triggers defense responses in non-host plants (Wei et al., 2020). However, how plants interact with R. solani during the pathogenic infection process is not yet known. In this study, we identified a transaminase ZmGABA-T that interacts with EG1 and suppresses the allergic necrosis induced by it. We also found that the ZmGABA-T is located in the plasma membrane. GABA, the substrate of GABA-T, suppresses the transcription of EG1. Hence, we inferred that the GABA-T participates in the plant disease resistance process.
Escherichia coli T1 was used for cloning and nucleotide sequencing. Pichia pastoris GS115 and plasmid vector pPIC9K were used for stable expression. Agrobacterium GV3101, plasmid vectors pGR106 and pROKII were used for transient expression.
Maize (Zea mays) plants (Non-gda 108 from Shandong, China, the most widely cultured hybrid in China) and N. benthamiana were grown at 25°C with 16 h of light and 8 h of darkness with a relative humidity of 60–70%. Oryza sativa (ZH11) and knockout mutants were grown at 28°C during the daytime and 26°C at night with 16 h of light and 8 h of darkness.
Strains of R. solani were cultured in PDA medium (200 g potato infusion, 20 g dextrose, and 20 g agar/L). Different GABA concentrations (0, 30, 40, 50, 60, and 70 mM) were added to the PDA medium to explore the effect of concentrated GABA on the transcription of EG1.
Agrobacterium tumefaciens-mediated transient expression was performed. Plasmid constructs were introduced into the A. tumefaciens strain GV3101. After culturing for 18 h in a liquid Luria Bertani medium at 28°C, the bacterial cells were harvested by centrifugation and resuspended in an infiltration medium (10 mM 2-morpholinoethanesulfonic acid, 10 mM MgCl2, 150 μM acetosyringone, pH 5.6). The optical densities (ODs) of the cell suspensions in the infiltration medium were adjusted to OD600 = 0.6 (the final concentration for each strain in a mixture). Thereafter, the cells were incubated for 3 h at room temperature before infiltration into leaves of 4-week-old N. benthamiana plants or Nicotiana tabacum. The leaves were harvested for protein 48 h after infiltration.
Agrobacterium tumefaciens strain GV3101 was cultivated with 200 μM of acetosyringone for 12 h, and the bacterial cells were harvested by centrifugation and resuspended in a Murashige and Skoog (MS) liquid medium (with 200 μM acetosyringone). The ODs of the cell suspensions in the MS medium (with 200 μM acetosyringone) were adjusted to OD600 = 0.6 (the final concentration for each strain in a mixture). The inner epidermis of the onion was torn off and cultured in the MS medium (with 200 μM acetosyringone) for 24 h in the dark. We placed the onion’s pre-cultured inner epidermis in the MS liquid medium (with 200 μM acetosyringone) to resuspend the bacteria solution, soaked it for 30 min, picked up a corner of the epidermis, drained the bacteria solution slightly, and reapplied it to the MS medium (with 200 μM acetosyringone), with a photoperiod of 16–18 h, and a total of 48 h at 25°C.
After the co-cultivation, small pieces of the onion’s inner epidermis were taken out and washed with sterile water to remove the attached Agrobacterium. The epidermis was spread on a glass slide, observed, and photographed under a scanning microscope (LSM800, Zeiss). The GFP was detected with excitation at 488 nm and emission at 525 nm, and mCherry was detected with excitation at 552 nm and emission at 600 nm.
3,3-Diaminobenzidine (DAB, 5 mg/mL) was used to detect ROS accumulation in N. benthamiana. Three days after the infection, leaves immersed in DAB were vacuumed for 30 min and then lighted for 4 h. After that, the liquid was discarded and the leaves were washed with 95% ethanol and boiled in water at 95°C. The system was allowed to stand until all the chlorophyll had dissolved, then the leaves were transferred to 50% ethanol for preservation and photographed.
For enzyme activity determination, proteins expressed by N. benthamiana were extracted by phosphate buffer (1 × protein inhibitor cocktail, CWBIO). And for general purposes, proteins were extracted by lysis buffer (NP-40, Beyotime).
Leaves of N. benthamiana were co-infiltrated with A. tumefaciens carrying ZmGABA-T-HA and EG1-eGFP, OsGABA-T-HA and EG1-eGFP, NtGABA-T-HA and EG1-eGFP, and AtGABA-T-HA and EG1-eGFP. The infiltrated leaves were harvested after 2 days and then ground to powder in liquid nitrogen, and suspended in a lysis buffer (NP-40, Beyotime). Anti-GFP agarose bead suspension (AlpaLife by KanTi) was added to the protein supernatants. The mixture was incubated at 4°C for 8 h with constant end-over-end rotation. Then the beads were rinsed with washing buffer (10 mM Tris-HCl, pH 7.5, 0.5 mM EDTA, 150 mM NaCl), and the anti-GFP agarose mixtures were collected for Western blotting following SDS–PAGE by 2 × SDS loading buffer. Fusion proteins were detected by anti-HA and anti-GFP antibodies, respectively, according to the EasySee Western Blot Kit (Transgene, China) with the Chenpchemi series chemiluminescence/multicolor fluorescence/visible light gel imaging system.
Recombinant EG1 was purified from P. pastoris GS115, AtGABA-T-eGFP and NtGABA-T-eGFP were expressed by transient expression in N. benthamiana, and then extracted proteins were collected on anti-GFP beads (AlpaLife by KanTi). The EG1 proteins were mixed with the beads carrying AtGABA-T or NtGABA-T at a final concentration of 10 μM in a buffer (10 mM Tris-HCl, pH 7.5, 0.5 mM EDTA, 150 mM NaCl), and incubated for 1 h at 4°C. Western blotting was then performed as described above.
Contrast vectors EG1-cYFP and ZmGABA-T-nYFP were transformed into A. tumefaciens. Thereafter, A. tumefaciens was infiltrated onto N. benthamiana leaves. The leaves were observed under a confocal laser scanning microscope (LSM880, Zeiss) 48 h after the infiltration, and YFP was detected with excitation at 488 nm and emission at 515 nm.
Furthermore, pGR106 carried with EG1-eGFP and mCherry–ZmGABA-T were transformed into A. tumefaciens. Thereafter, A. tumefaciens were infiltrated onto N. benthamiana leaves. After 48 h infiltration. The leaves were observed under a confocal laser scanning microscope (LSM800, Zeiss) 48 h after the infiltration, and mCherry was detected with excitation at 552 nm and emission at 600 nm.
Enzyme activity was measured using the 3,5-dinitrosalicylic acid (DNS) method (Sumner, 1925; Miller, 1959). To explore the influence of ZmGABA-T on EG1 enzyme activity, EG1 expressed by P. pastoris GS115 was co-incubated with ZmGABA-T and pGR106 expressed by N. benthamiana at 50°C for 30 min. Next, 1% carboxymethylcellulose sodium (CMC–Na) was used as the enzyme substrate, and phosphate buffer (pH 5.0) was used as the buffer system. Then DNS was used to determine glucose content and measure absorbance at OD540.
To understand the effect of GABA concentration on EG1 enzyme activity, GABA concentrations ranging from 30 to 70 mM were added into an enzymatic reaction system. EG1 expressed by P. pastoris GS115 was co-incubated with 1% CMC–Na and GABA at 50°C in a phosphate buffer (pH 5.0) for 30 min. Then DNS was used to determine glucose content and absorbance was measured at OD540.
Zea mays leaves injected with purified EG1 expressed by P. pastoris GS115 were harvested at different time points and ground in liquid nitrogen for RNA extraction with TRIzol. The first strand of cDNA was synthesized from 1 μg total RNA using TransScript All-in-One First Strand cDNA Synthesis SuperMix (Transgen, China). qRT-PCR was assayed by TransStart Top Green qPCR SuperMix (Transgen, China). Each PCR tube contained 10 μL of Top Green qPCR SuperMix, 25 ng of cDNA, and 0.2 μM of each primer. The thermal cycling conditions were 30 s at 94°C, followed by 40 cycles of 5 s at 94°C and 15 s at 55°C by Roche LightCycler 96.
Rhizoctonia solani was cultured in PDA with different GABA concentrations for 3 days and was collected in liquid nitrogen for RNA extraction and qRT-PCR as described above.
For biomass measurement, total DNA was extracted by the Plant Genomic DNA Kit (TIANGEN, China), and qPCR was assayed by the TransStart Top Green qPCR SuperMix (Transgen, China).
CRISPR/Cas9-mediated gene-editing vectors were constructed according to previous reports (Ma et al., 2016). Transgenic experiments were performed using A. tumefaciens–mediated callus transformation in the japonica variety ZH11.
To find the protein that interacts with EG1 in plants, we identified potential host protein targets using DUAL membrane yeast two-hybrid (Y2H) assays. We independently screened the EG1 forms as baits against a prey library from RNA prepared at 72 h postinoculation of Z. mays injected with EG1 protein. A total of 43 positive colonies were captured by the bait EG1 (Figures 1A,B).
Figure 1. EG1 interacts with ZmGABA-T. (A) Biological function pathways of candidate genes. There are 43 genes in total. And all the 43 genes were classified into 16 types according to their functions: Ubiquitin-mediated proteolysis, protein processing in the endoplasmic reticulum, oxidative phosphorylation, other glycan degradation, nucleotide excision repair, metabolic pathways, glycosphingolipid biosynthesis–globo series, ganglio series, glycosaminoglycan degradation, fatty acid metabolism, fatty acid biosynthesis, endocytosis, butanoate metabolism, biosynthesis of unsaturated fatty acids, amino sugar, and nucleotide sugar metabolism, and alanine, aspartate, and glutamate metabolism. (B) Gene Ontology (GO) analysis of candidate genes. The function of genes is divided into three parts: Biological process, cellular component, and molecular function. And the genes function of nutrient reservoir activity has the largest number. Enriched P-values less than 0.05 are marked with “*”. (C) Interactions between EG1 with ZmGABA-T in the DUAL membrane yeast 2 hybrid system. Yeast NMY32 cells cotransformed with bait and prey vectors were grown on QDO (SD/–Ade/–His/–Leu/–Trp) medium. The combination of pTSU2-APP and pNubG-Fe65 was used as a positive control, while the combination of pTSU2-APP and pPR3N was used as the negative control. (D) Interactions between EG1 with ZmGABA-T in Nicotiana benthamiana. pGR106 carried EG1-EGFP and ZmGABA-T-HA, respectively, were coexpressed in Nicotiana benthamiana. Around 48 h after infiltration, immunoprecipitates obtained from whole-cell extracts using anti-GFP trap beads were analyzed by immunoblotting with anti-HA and anti-GFP antibodies. This experiment was repeated three times with the same results. (E) BiFC assay in Nicotiana benthamiana. Bars = 20 μm.
According to the analysis of results of the 43 positive colonies, we found that the protein that was most likely to interact with EG1 was ZmGABA-T and chose to make it the focus of our study. We linked the EG1 without its signal peptide with a bait construct because the secreted protein EG1 owned the signal peptide. We also connected the ZmGABA-T to the prey construct. Point-to-point verification showed that all transformants grew on the SD/-Trp-Leu, but only yeasts containing both EG1 and ZmGABA-T could grow on the SD/-Trp-Leu-Ade-His media (Figure 1C).
To prove that EG1 can interact with ZmGABA-T in plants, co-immunoprecipitation (co-IP) assays were performed by transient expression in N. benthamiana. The proteins were pulled down with GFP beads and were all present in the relevant input samples except for ZmGABA-T-HA, which was co-immunoprecipitated in the presence of EG1-eGFP (Figure 1D).
Then EG1 and ZmGABA-T were fused to the construct with yellow fluorescent protein (YFP), which was divided into N-terminal and C-terminal to generate EG1-cYFP or ZmGABA-T-nYFP. In addition, the two empty constructs, nYFP and cYFP, were used as a negative control. Co-expression of EG1-cYFP and ZmGABA-T-nYFP in N. benthamiana resulted in YFP fluorescence signals located in the cytoplasmic membrane 72 h post-infiltration (hpi). The EG1-cYFP/nYFP and ZmGABA-T-nYFP/cYFP combinations failed to fluoresce, demonstrating that neither EG1 nor ZmGABA-T produced non-specific fluorescence in N. benthamiana (Figure 1E). All these results indicate that EG1 interacts with ZmGABA-T in plants.
We fused mCherry to the N-terminal of ZmGABA-T, with the transient expression of mCherry–ZmGABA-T in N. benthamiana. This resulted in red fluorescence signals in the cytoplasmic membrane 48 hpi. The plasmolysis of the onion epidermal cells returned similar results, the EG1-eGFP colocalized with mCherry–ZmGABA-T in the onions cell membrane (Figure 2).
Figure 2. Subcellular localization of ZmGABA-T and EG1 in Nicotiana benthamiana and onions. (A) Subcellular localization of ZmGABA-T. mCherry fused with ZmGABA-T were transiently expressed by A. tumefaciens in Nicotiana benthamiana 48 h after infiltration. (B) Co-localization of EG1 and ZmGABA-T. For coexpressed, EG1 fused with eGFP and mCherry fused with ZmGABA-T were coexpressed in onions 48 h after infiltration. After 48 h of co-cultivation on MS medium and plasmolysis with 1 M NaCl to induce cytoplasmic separation, both red and green fluorescent signals were found on the cell membrane. The infiltrated N. benthamiana leaves and onion’s epidermal cells were observed via fluorescence microscopy. The eGFP and mCherry were used as check samples. The merged image of two fluorescence signals is shown on the right. Bars = 20 μm.
Five days after the EG1 and ZmGABA-T were co-injected into the N. benthamiana, we found that the allergic necrosis caused by EG1 was weakened (Figure 3A). The ROS detected by 3,3-di-aminobenzidine (DAB) also showed weakened symptoms (Figure 3B).
Figure 3. ZmGABA-T suppresses allergic necrosis induced by EG1 according to promoting the degradation of EG1, rather than inhibit the enzyme activity of EG1. (A) Transient expression of EG1 and ZmGABA-T in Nicotiana benthamiana. Transient expression was operated in Nicotiana benthamiana leaves injected with Agrobacterium carrying the indicated genes, pGR106, eGFP, EG1, and ZmGABA-T. eGFP and EG1 were coexpressed on the left of the leaf, and ZmGABA-T and EG1 were coexpressed on the right. Experiments were repeated three times at least with similar results. (B) ROS detection of EG1 and ZmGABA-T. The transient expression of Nicotiana benthamiana leaves inoculated with eGFP and EG1, ZmGABA-T and EG1 were dyed with 5 mg/mL DAB (3,3-diaminobenzidine). Transient expression was assessed in Nicotiana benthamiana leaves from 4-week-old plants 5 days after agroinfiltration, and pGR106 empty vector was used as a control. Experiments were repeated three times at least with similar results. (C) Enzyme activity determination of EG1 and ZmGABA-T. Co-incubation EG1 (1 μM) expressed by Pichia pastoris GS115 with pGR106 and ZmGABA-T expressed by Nicotiana benthamiana (2 mg/mL) separately at 50°C for 30 min, then dinitrosalicylic acid (DNS) was used to determine glucose content, and measured absorbance at OD540, thereby calculated enzyme activity of EG1. The results of the experiment were repeated three times at least. Lower-case letters indicate statistically significant differences (P < 0.05). (D) Co-expression of EG1-eGFP and ZmGABA-T-HA in Nicotiana benthamiana. The concentration of inoculation about ZmGABA-T was increased sequentially from OD600 = 0 to 0.9, while the concentration of EG1 was OD600 = 0.5. The numbers in the first row indicated the inoculation concentration of ZmGABA-T-HA. The numbers in the second row indicated the gray value of EG1. The results of the experiment were repeated three times at least.
To explore why the allergic necrosis was suppressed, the EG1 and ZmGABA-T were expressed by yeast and N. benthamiana, respectively. Then, proteins were used to detect the enzyme activity by DNS. After enzymatic reaction for 30 min at 50°C, there was no significant difference in the specific vitality of EG1 in the presence of ZmGABA-T. Therefore, we concluded that ZmGABA-T could not affect EG1 enzyme activity (Figure 3C).
Then the co-expressed proteins by N. benthamiana were extracted. A Western blotting analysis showed that the expression of EG1-eGFP was significantly reduced in the presence of ZmGABA-T–HA (Figure 1D). To prove the results, we increased the concentration of ZmGABA-T—-HA gradually and then co-expressed ZmGABA-T–HA and EG1-eGFP in N. benthamiana. The result showed that the expression of EG1-eGFP was reduced because of the increased concentration of ZmGABA-T–HA (Figure 3D). The above results proved that ZmGABA-T promotes EG1 degradation and suppresses the EG1-induced allergic necrosis in N. benthamiana.
γ-Aminobutyric acid (GABA) plays an important role in the metabolism of amino acids (Ludewig et al., 2008). We added different concentrations of GABA to the EG1 enzymatic reaction system and found that GABA did not affect EG1 enzyme activity (Figure 4A). Thereafter, we added GABA to the culture medium of R. solani, and the transcription of EG1 was detected by the qPCR. However, we were surprised to see that increased GABA concentration led to decreased EG1 transcription (Figure 4B). Based on this result, we concluded that GABA repressed EG1 transcription during R. solani infection. All these results prove that ZmGABA-T and GABA work together to inhibit allergic necrosis induced by EG1.
Figure 4. GABA does not affect the enzyme activity of EG1 but suppresses the transcription of EG1. (A) Enzyme activity determination of EG1 after adding different concentrations of GABA. Different concentrations of GABA were added to the enzymatic reaction system, and DNS was used to detect the content of glucose. The experiments were repeated three times with the same results. Lowercase letters indicate statistically insignificant differences (P > 0.05). (B) The effect of different concentrations of GABA on the transcription of EG1. Different concentrations of GABA were added to the Potato Dextrose Agar (PDA) medium, then Rhizoctonia solani was grown on PDA medium with GABA for 3 days. Total RNA was extracted 3 days after training. Then qRT-PCR was used to detect the transcription level of EG1. The results of the experiment were repeated three times at least. Lower-case letters indicate statistically significant differences (P < 0.05).
To explore the function of ZmGABA-T, the leaves of N. benthamiana, which have transiently expressed ZmGABA-T 36 h after, were inoculated with R. solani. Leaves that expressed pGR106 were used as a negative control. Infection lesion sizes were recorded for comparison 4 days after inoculation with R solani. We found that, compared with the negative control, leaves that expressed ZmGABA-T showed smaller lesions (Figures 5A,B). Measurement of fungal DNA by quantitative PCR was used to quantify fungal colonization of the host tissues. There was a fivefold reduction in the fungal colonization of the host in leaves that had transiently expressed ZmGABA-T (Figure 5C).
Figure 5. Expression of ZmGABA-T can inhibit Rhizoctonia solani infection. (A) Rhizoctonia solani infection on Nicotiana benthamiana. N. benthamiana leaves were inoculated by Rhizoctonia solani AG1-IA after transient expression of pGR106 vector and ZmGABA-T at 36 h, and photographed at 72 h post-inoculation. (B) Lesion diameters of infected Nicotiana benthamiana leaves. Lesion diameters were calculated from three independent biological replicates. Error bars represent ± SD of at least six leaves each. Lower-case letters indicate statistically significant differences (P < 0.05). (C) Quantitative PCR was performed for quantification of relative fungal growth on inoculated leaves 2(Ct_NbActin–Ct_Rsdna). The values represent the means ± SD of six independent tests. Lower-case letters indicate statistically significant differences (P < 0.05).
To explore whether EG1 interacts with ZmGABA-T specifically, we compared different kinds of GABA-T around Z. mays, O. sativa, Arabidopsis thaliana, and N. tabacum (Supplementary Figure 1A), all of which had a Pyridoxal-5′-phosphate–binding domain (Ser/Thr-X-X-Lys). Yeast two-hybrid assay showed that OsGABA-T can interact with EG1. Co-IP proved that OsGABA-T interacts with EG1 weakly in N. benthamiana (Figure 6), and pull-down assay showed that EG1 interacts with neither AtGABA-T nor NtGABA-T (Supplementary Figures 1B,C).
Figure 6. EG1 interacts with OsGABA-T. (A) Interactions between EG1 with OsGABA-T in the DUAL membrane yeast 2 hybrid system. Yeast NMY32 cells cotransformed with bait and prey vectors were grown on QDO (SD/–Ade/–His/–Leu/–Trp) medium. The combination of pTSU2-APP and pNubG-Fe65 was used as a positive control, while the combination of pTSU2-APP and pPR3N was used as the negative control. (B) Interactions between EG1 with OsGABA-T in Nicotiana benthamiana. pGR106 carried EG1-EGFP and OsGABA-T-HA were coexpressed in Nicotiana benthamiana; 48 h after infiltration, immunoprecipitates obtained from whole-cell extracts using anti-GFP trap beads were analyzed by immunoblotting with anti-HA and anti-GFP antibodies. This experiment was repeated three times with similar results.
So we continued to explore if OsGABA-T has the same function as ZmGABA-T. Transient expression of OsGABA-T and EG1 showed that the allergic necrosis induced by EG1 was attenuated (Figure 7A). The ROS detected by DAB also showed an obvious weakening of the symptoms (Figure 7B).
Figure 7. OsGABA-T suppresses allergic necrosis induced by EG1. (A) Transient expression of EG1 and OsGABA-T in Nicotiana benthamiana. Transient expression was operated in Nicotiana benthamiana leaves injected Agrobacterium carrying the indicated genes, pGR106, eGFP, EG1, and OsGABA-T. eGFP and EG1 were coexpressed on the left of the leaf, OsGABA-T and EG1 were coexpressed on the right. Experiments were repeated three times at least with similar results. (B) ROS detection of EG1 and OsGABA-T. The transient expression of Nicotiana benthamiana leaves inoculated with eGFP and EG1, and OsGABA-T and EG1 were dyed with 5 mg/mL 3,3-diaminobenzidine (DAB). Transient expression was assessed in Nicotiana benthamiana leaves from 4-week-old plants 5 days after agroinfiltration, and pGR106 empty vector was used as a control. Experiments were repeated three times at least with similar results.
Two independent transgenic rice lines whose OsGABA-T were knocked out by CRISPR (Os-24 and Os-31) were tested for OsGABA-T expression. A cytosine (C) or guanine (G) residue was inserted into the putative U3-gRNA target site, and thymine (T) was inserted into the putative U6a-gRNA target site or a guanine–adenine–adenine (GAA) sequence was lost from the U6a-gRNA target site (Figure 8A). Both caused an 80% more reduction in OsGABA-T expression than the non-transformed line ZH11 wild type at 4 weeks (Figure 8B). Mutant plants exhibited reduced resistance against R. solani (Figure 8C). The measurement of fungal DNA by qPCR was used to quantify the fungal colonization of the host tissue. Compared with the wild type, the lesion length of Os-24 and Os-31 were significantly increased (Figure 8D). There was a 15-fold increase in the fungal colonization of the host in Os-24 and Os-31 plants (Figure 8E). All the results showed that mutant plants increased sensitivity to R. solani.
Figure 8. Knockout of OsGABA-T reduces rice disease resistance to Rhizoctonia solani. (A) Sequence of gRNA target of rice. Red color represents the target sequence. (B) OsGABA-T transcription levels in wild-type ZH11 and OsGABA-T knocked out rice lines, Os-24 and Os-31. Error bars represent ± SD of at least three independent tests. Lower-case letters indicate statistically significant differences (P < 0.05). (C) Inoculation of wild-type ZH11 and OsGABA-T knocked out lines Os-24 and Os-31 with Rhizoctonia solani AG1-IA. Images were taken 72 h after inoculation. (D) Lesion length of infected rice leaves. Lesion length was calculated from three independent biological replicates. And the red arrows indicated lesion length. Error bars represent ± SD of at least six leaves each. Lower-case letters indicate statistically significant differences (P < 0.05). (E) Quantitative PCR was performed for quantification of relative fungal growth on inoculated leaves 2(Ct_OsUbiq–Ct_RsDNA). Error bars represent ± SD of 6 independent tests. Lower-case letters indicate statistically significant differences (P < 0.05).
The HR refers to cell death induced by pathogenic infections and is a classical indicator of resistance. Cell death has markedly different functions in plant responses to necrotrophs and biotrophs. HR is thought to confine pathogens by abolishing nutrient supply, thereby limiting the growth of pathogens in biotrophs (Mengiste, 2012). However, cell death also occurs at the early stage of necrotrophic pathogens infection and promotes further infection (Govrin et al., 2006; van Kan, 2006). Necrotrophic fungi, including R. solani, kill the cells and tissues of the host plant and then draw nutrients from them. Successful necrotrophs rely on a large number of hydrolytic enzymes for the maceration and utilization of the plant as their source of nutrition (Doehlemann et al., 2017). As a countermeasure, plants develop surveillance systems to perceive necrotrophic fungi and activate their innate immunity, like the signal of chitin perception, the regulation of mitogen-activated protein kinase, and the modification of histones (McGrath et al., 2005; Veronese et al., 2006; Kemmerling et al., 2007; Berr et al., 2010; Lenz et al., 2011; Li et al., 2012; Zhang et al., 2012). In our previous study, we proved that the cellulase EG1 secreted by R. solani can act as a PAMP that participates in plant immune responses (Ma Y. et al., 2015; Guo et al., 2021). In this study, we identified ZmGABA-T, a GABA-T from Z. mays through Y2H screening, and the interaction between ZmGABA-T and EG1 was verified by Y2H, CoIP, and BiFC assays (Figure 1).
GABA-T catalyzes the breakdown of GABA to succinic semialdehyde (SSA), playing a key role in its life cycle and metabolism (Cauwenberghe and Shelp, 1999; Cauwenberghe et al., 2002). In A. thaliana, full-length AtGABA-T contains an N-terminal targeting pre-sequence that is 36 amino acids long and is both sufficient and necessary for targeting enzymes to mitochondria (Clark et al., 2009a). In the tomato plant Solanum lycopersicum L., three GABA-Ts owned highly similar sequences in their coding regions, except their N-terminal regions, leading to distinct subcellular localizations in the mitochondrion, plastid, or cytosol (Clark et al., 2009b). In O. sativa, four putative GABA-T genes exhibit a high amino acid identity (73–82%), but they differ in length at the N-terminal region, located in different organelles (Shimajiri et al., 2013). Our study revealed that ZmGABA-T in Z. mays is located in the cell membrane (Figure 2A).
In A. thaliana and Z. mays, plant elicitor peptides (PEPs) play a key role in plant immunity (Yamaguchi and Huffaker, 2011; Bartels and Boller, 2015). PEPs without N-terminal secretion signals can be released to the extra-plasma by unidentified mechanisms and identified by plant elicitor peptide receptors (PEPRs) on the plasma membrane (Yamaguchi et al., 2006; Yamaguchi et al., 2010; Yamaguchi and Huffaker, 2011). ZmGABA-T also lacks transmembrane structure and does not contain a signal peptide. During the infection of R. solani, ZmGABA-T can interact with EG1 in the cell membrane (Figure 2B). Therefore, we concluded that an unknown protein can interact with it in cells and anchor it to the cell membrane.
Research has shown that the regulatory actions of GABA-T are closely related to immune responses to pathogens (Wu et al., 2006). Our results showed that the transient expression of GABA-T in N. benthamiana decreased the level of ROS triggered by EG1 and further suppressed the allergic necrosis induced by EG1 (Figures 3A,B, 7). In addition, we proved that the reduced allergic necrosis and ROS can be attributed to the promotion of EG1 degradation by ZmGABA-T rather than the inhibition of EG1 activity in N. benthamiana (Figures 3C,D).
GABA is a four-carbon, ubiquitous, non-proteinogenic amino acid. In animals, it is a signaling molecule that functions as an inhibitory neurotransmitter. In plants, abiotic stress (hypoxia, heat, cold, drought, mechanical wounding) or biotic stress (wounding due to herbivory and infection) results in the rapid accumulation of GABA. In addition, GABA can also act as a signaling molecule in plant defense (Bown and Shelp, 2016). Our results showed that GABA can suppress the transcription of EG1 while not affecting its enzymatic activity (Figure 4). Thus, we conclude that GABA-T and GABA can directly or indirectly inhibit the expression of EG1, and therefore the production of ROS and allergic necrosis.
The transient expression of ZmGABA-T inhibited R. solani infection, and the size of lesions and the biomass of pathogenic fungi were reduced (Figure 5). When the homologous sequence of ZmGABA-T in O. sativa was knocked out, the resistance of knocked out plants to R. solani was significantly weakened (Figure 8). These results suggest that GABA-T may positively regulate disease resistance against R. solani in rice.
In plants, GABA-T is involved in the metabolism of GABA, which is a significant component of the free amino acid pool in most prokaryotic and eukaryotic organisms (Bown, 1997; Shelp et al., 1999). GABA is metabolized mainly via the GABA-shunt pathways. GABA production from glutamate is catalyzed by glutamate decarboxylase; whereas GABA-T catalyzes the conversion of GABA to SSA, which is then oxidized to succinate due to succinic semialdehyde dehydrogenase. Thus, the carbon skeleton of GABA enters the tricarboxylic acid cycle (Tuin and Shelp, 1994; Shelp et al., 2012). Other studies have shown that GABA-T (GABA shunt) may play a role in restricting the levels of allergic necrosis during host and pathogen interaction (Wu et al., 2006).
In summary, our study revealed that GABA-T in Z. mays can target the CWDE EG1 and act as a positive defense regulator that can suppress the accumulation of ROS and allergic necrosis caused by EG1. Future studies will focus on investigating the protein interactions with ZmGABA-T and the receptor that interacts with EG1 in the plasma membrane.
The datasets presented in this study can be found in online repositories. The names of the repository/repositories and accession number(s) can be found below: Sequence data from this article can be found in the GenBank/EMBL data libraries under the following accession numbers: ZmGABA-T (XM_008670668.1), OsGABA-T (AF297651.1), NtGABA-T (XM_016626518.1), and AtGABA-T (NM_113117.4).
XG and DL designed the experiments, analyzed the data, and contributed to the drafting and revision of the manuscript. XG, JC, MG, and DL performed the experiments. All authors read and approved the final manuscript.
This research was funded by the Ministry of Science and Technology of China (Grant Nos. 2015BAD15B05 and 2012AA10180402) and the National Nature Science Foundation of China (Grant No. 31571949).
The authors declare that the research was conducted in the absence of any commercial or financial relationships that could be construed as a potential conflict of interest.
All claims expressed in this article are solely those of the authors and do not necessarily represent those of their affiliated organizations, or those of the publisher, the editors and the reviewers. Any product that may be evaluated in this article, or claim that may be made by its manufacturer, is not guaranteed or endorsed by the publisher.
We are grateful for funding the work by the Ministry of Science and Technology of China and the National Natural Science Foundation of China.
The Supplementary Material for this article can be found online at: https://www.frontiersin.org/articles/10.3389/fpls.2022.860170/full#supplementary-material
Amselem, J., Cuomo, C. A., van Kan, J. A., Viaud, M., Benito, E. P., Couloux, A., et al. (2011). Genomic analysis of the necrotrophic fungal pathogens Sclerotinia sclerotiorum and Botrytis cinerea. PLoS Genet. 7:e1002230. doi: 10.1371/journal.pgen.1002230
Bartels, S., and Boller, T. (2015). Quo vadis, Pep? Plant elicitor peptides at the crossroads of immunity, stress, and development. J. Exp. Bot. 66, 5183–5193. doi: 10.1093/jxb/erv180
Berr, A., Mccallum, E. J., Alioua, A., Heintz, D., Heitz, T., and Shen, W. H. (2010). Arabidopsis histone methyltransferase SET DOMAIN GROUP8 mediates induction of the jasmonate/ethylene pathway genes in plant defense response to necrotrophic fungi. Plant Physiol. 154, 1403–1414. doi: 10.1104/pp.110.161497
Boller, T., and Felix, G. (2009). A renaissance of elicitors: perception of microbe-associated molecular patterns and danger signals by pattern-recognition receptors. Annu. Rev. Plant Biol. 60, 379–406. doi: 10.1146/annurev.arplant.57.032905.105346
Bown, A. W., and Shelp, B. J. (2016). Plant GABA: not just a metabolite. Trends Plant Sci. 21, 811–813. doi: 10.1016/j.tplants.2016.08.001
Cauwenberghe, O., and Shelp, B. J. (1999). Biochemical characterization of partially purified gaba:pyruvate transaminase from Nicotiana tabacum. Phytochemistry 52, 575–581. doi: 10.1016/s0031-9422(99)00301-5
Cauwenberghe, O. V., Makhmoudova, A., Mclean, M., Clark, S. M., and Shelp, B. J. (2002). Plant pyruvate-dependent gamma-aminobutyrate transaminase: identification of an Arabidopsis cDNA and its expression in Escherichia coli. Can. J. Bot. 80, 933–941. doi: 10.1139/b02-087
Charova, S. N., Dölfors, F., Holmquist, L., Moschou, P. N., Dixelius, C., and Tzelepis, G. (2020). The RsRlpA effector is a protease inhibitor promoting rhizoctonia solani virulence through suppression of the hypersensitive response. Int. J. Mol. Sci. 21:8070. doi: 10.3390/ijms21218070
Clark, S. M., Di Leo, R., Dhanoa, P. K., Van Cauwenberghe, O. R., Mullen, R. T., and Shelp, B. J. (2009a). Biochemical characterization, mitochondrial localization, expression, and potential functions for an Arabidopsis gamma-aminobutyrate transaminase that utilizes both pyruvate and glyoxylate. J. Exp. Bot. 60, 1743–1757. doi: 10.1093/jxb/erp044
Clark, S. M., Di Leo, R., Van Cauwenberghe, O. R., Mullen, R. T., and Shelp, B. J. (2009b). Subcellular localization and expression of multiple tomato gamma-aminobutyrate transaminases that utilize both pyruvate and glyoxylate. J. Exp. Bot. 60, 3255–3267. doi: 10.1093/jxb/erp161
Dangl, J. L., and Jones, J. D. (2001). Plant pathogens and integrated defence responses to infection. Nature 411, 826–833. doi: 10.1038/35081161
Desaki, Y., Miya, A., Venkatesh, B., Tsuyumu, S., Yamane, H., Kaku, H., et al. (2006). Bacterial lipopolysaccharides induce defense responses associated with programmed cell death in rice cells. Plant Cell Physiol. 47, 1530–1540. doi: 10.1093/pcp/pcl019
Dickman, M. B., Park, Y. K., Oltersdorf, T., Li, W., Clemente, T., and French, R. (2001). Abrogation of disease development in plants expressing animal antiapoptotic genes. Proc. Natl. Acad. Sci. U.S.A. 98, 6957–6962. doi: 10.1073/pnas.091108998
Doehlemann, G., Ökmen, B., Zhu, W., and Sharon, A. (2017). Plant pathogenic fungi. Microbiol. Spectr. 5:23.
Govrin, E. M., and Levine, A. (2000). The hypersensitive response facilitates plant infection by the necrotrophic pathogen Botrytis cinerea. Curr. Biol. 10, 751–757. doi: 10.1016/s0960-9822(00)00560-1
Govrin, E. M., Rachmilevitch, S., Tiwari, B. S., Solomon, M., and Levine, A. (2006). An Elicitor from Botrytis cinerea induces the hypersensitive response in Arabidopsis thaliana and other plants and promotes the gray mold disease. Phytopathology 96, 299–307. doi: 10.1094/PHYTO-96-0299
Gui, Y. J., Chen, J. Y., Zhang, D. D., Li, N. Y., Li, T. G., Zhang, W. Q., et al. (2017). Verticillium dahliae manipulates plant immunity by glycoside hydrolase 12 proteins in conjunction with carbohydrate-binding module 1. Environ. Microbiol. 19, 1914–1932. doi: 10.1111/1462-2920.13695
Guo, X., Liu, N., Zhang, Y., and Chen, J. (2021). Pathogen-associated molecular pattern active sites of GH45 endoglucanohydrolase from Rhizoctonia solani. Phytopathology 112, 355–363. doi: 10.1094/PHYTO-04-21-0164-R
Kanyuka, K., and Rudd, J. J. (2019). Cell surface immune receptors: the guardians of the plant’s extracellular spaces. Curr. Opin. Plant Biol. 50, 1–8. doi: 10.1016/j.pbi.2019.02.005
Kemmerling, B., Schwedt, A., Rodriguez, P., Mazzotta, S., Frank, M., Qamar, S. A., et al. (2007). The BRI1-associated kinase 1, BAK1, has a brassinolide-independent role in plant cell-death control. Curr. Biol. 17, 1116–1122. doi: 10.1016/j.cub.2007.05.046
Kim, K. S., Min, J. Y., and Dickman, M. B. (2008). Oxalic acid is an elicitor of plant programmed cell death during Sclerotinia sclerotiorum disease development. Mol. Plant Microbe Interact. 21, 605–612. doi: 10.1094/MPMI-21-5-0605
Laluk, K., Abuqamar, S., and Mengiste, T. (2011). The Arabidopsis mitochondria-localized pentatricopeptide repeat protein PGN functions in defense against necrotrophic fungi and abiotic stress tolerance. Plant Physiol. 156, 2053–2068. doi: 10.1104/pp.111.177501
Lenz, H. D., Haller, E., Melzer, E., Kober, K., and Nürnberger, T. (2011). Autophagy differentially controls plant basal immunity to biotrophic and necrotrophic pathogens. Plant J. 66, 818–830. doi: 10.1111/j.1365-313X.2011.04546.x
Li, G., Meng, X., Wang, R., Mao, G., Han, L., Liu, Y., et al. (2012). Dual-level regulation of ACC synthase activity by MPK3/MPK6 cascade and its downstream WRKY transcription factor during ethylene induction in Arabidopsis. PLoS Genet. 8:e1002767. doi: 10.1371/journal.pgen.1002767
Li, S., Peng, X., Wang, Y., Hua, K., Xing, F., Zheng, Y., et al. (2019). The effector AGLIP1 in Rhizoctonia solani AG1 IA triggers cell death in plants and promotes disease development through inhibiting PAMP-triggered immunity in Arabidopsis thaliana. Front. Microbiol. 10:2228. doi: 10.3389/fmicb.2019.02228
Ludewig, F., Hüser, A., Fromm, H., Beauclair, L., and Bouché, N. (2008). Mutants of GABA transaminase (POP2) suppress the severe phenotype of succinic semialdehyde dehydrogenase (ssadh) mutants in Arabidopsis. PLoS One 3:e3383. doi: 10.1371/journal.pone.0003383
Luo, H., Laluk, K., Lai, Z., Veronese, P., Song, F., and Mengiste, T. (2010). The Arabidopsis Botrytis susceptible1 interactor defines a subclass of RING E3 ligases that regulate pathogen and stress responses. Plant Physiol. 154, 1766–1782. doi: 10.1104/pp.110.163915
Ma, X., Zhu, Q., Chen, Y., and Liu, Y. G. (2016). CRISPR/Cas9 platforms for genome editing in plants: developments and applications. Mol. Plant 9, 961–974. doi: 10.1016/j.molp.2016.04.009
Ma, Y., Han, C., Chen, J., Li, H., He, K., Liu, A., et al. (2015). Fungal cellulase is an elicitor but its enzymatic activity is not required for its elicitor activity. Mol. Plant Pathol. 16, 14–26. doi: 10.1111/mpp.12156
Ma, Z., Song, T., Lin, Z., Ye, W., and Wang, Y. (2015). A Phytophthora sojae glycoside hydrolase 12 protein is a major virulence factor during soybean infection and is recognized as a PAMP. Plant Cell 27:2057. doi: 10.1105/tpc.15.00390
Mauff, F. L., Bamford, N. C., Alnabelseya, N., Zhang, Y., Baker, P., Robinson, H., et al. (2019). Molecular mechanism of Aspergillus fumigatus biofilm disruption by fungal and bacterial glycoside hydrolases. J. Biol. Chem. 294, 10760–10772. doi: 10.1074/jbc.RA119.008511
McGrath, K. C., Dombrecht, B., Manners, J. M., Schenk, P. M., Edgar, C. I., Maclean, D. J., et al. (2005). Repressor- and activator-type ethylene response factors functioning in jasmonate signaling and disease resistance identified via a genome-wide screen of Arabidopsis transcription factor gene expression. Plant Physiol. 139, 949–959. doi: 10.1104/pp.105.068544
Mengiste, T. (2012). Plant immunity to necrotrophs. Annu. Rev. Phytopathol. 50, 267–294. doi: 10.1146/annurev-phyto-081211-172955
Mengiste, T., Chen, X., Salmeron, J., and Dietrich, R. (2003). The BOTRYTIS SUSCEPTIBLE1 gene encodes an R2R3MYB transcription factor protein that is required for biotic and abiotic stress responses in Arabidopsis. Plant Cell 15, 2551–2565. doi: 10.1105/tpc.014167
Miller, G. L. (1959). Use of dinitrosalicylic acid reagent for determination of reducing sugar. Anal. Biochem. 31, 426–428. doi: 10.1021/ac60147a030
Navarro, L., Bari, R., Achard, P., Lisón, P., Nemri, A., Harberd, N. P., et al. (2008). DELLAs control plant immune responses by modulating the balance of jasmonic acid and salicylic acid signaling. Curr. Biol. 18, 650–655. doi: 10.1016/j.cub.2008.03.060
Oliver, R. P., and Solomon, P. S. (2010). New developments in pathogenicity and virulence of necrotrophs. Curr. Opin. Plant Biol. 13, 415–419. doi: 10.1016/j.pbi.2010.05.003
Shelp, B. J., Bown, A. W., and Mclean, M. D. (1999). Metabolism and functions of gamma-aminobutyric acid. Trends Plant Sci. 4, 446–452. doi: 10.1016/s1360-1385(99)01486-7
Shelp, B. J., Mullen, R. T., and Waller, J. C. (2012). Compartmentation of GABA metabolism raises intriguing questions. Trends Plant Sci. 17, 57–59. doi: 10.1016/j.tplants.2011.12.006
Shen, D., Wang, J., Dong, Y., Zhang, M., and Xia, A. (2020). The glycoside hydrolase 18 family chitinases are associated with development and virulence in the mosquito pathogen Pythium guiyangense. Fungal Genet. Biol. 135:103290. doi: 10.1016/j.fgb.2019.103290
Shimajiri, Y., Ozaki, K., Kainou, K., and Akama, K. (2013). Differential subcellular localization, enzymatic properties and expression patterns of γ-aminobutyric acid transaminases (GABA-Ts) in rice (Oryza sativa). J. Plant Physiol. 170, 196–201. doi: 10.1016/j.jplph.2012.09.007
Snarr, B. D., Baker, P., Bamford, N. C., Sato, Y., Liu, H., Lehoux, M., et al. (2017). Microbial glycoside hydrolases as antibiofilm agents with cross-kingdom activity. Proc. Natl. Acad. Sci. U.S.A. 114, 7124–7129. doi: 10.1073/pnas.1702798114
Stergiopoulos, I., and de Wit, P. J. (2009). Fungal effector proteins. Annu. Rev. Phytopathol. 47, 233–263. doi: 10.1146/annurev.phyto.112408.132637
Sumner, J. B. (1925). A more specific reagent for the determination of sugar in urine. J. Biol. Chem. 65, 393–395. doi: 10.1016/s0021-9258(18)84848-7
Tsuda, K., Sato, M., Stoddard, T., Glazebrook, J., and Katagiri, F. (2009). Network properties of robust immunity in plants. PLoS Genet. 5:e1000772. doi: 10.1371/journal.pgen.1000772
Tuin, L. G., and Shelp, B. J. (1994). In situ [14C]glutamate metabolism by developing soybean cotyledons I. metabolic routes. J. Plant Physiol. 143, 1–7. doi: 10.1016/s0176-1617(11)82089-4
van Kan, J. A. (2006). Licensed to kill: the lifestyle of a necrotrophic plant pathogen. Trends Plant Sci. 11, 247–253. doi: 10.1016/j.tplants.2006.03.005
Vaux, D. L., and Silke, J. (2005). IAPs, RINGs and ubiquitylation. Nat. Rev. Mol. Cell Biol. 6, 287–297. doi: 10.1038/nrm1621
Veronese, P., Nakagami, H., Bluhm, B., Abuqamar, S., Chen, X., Salmeron, J., et al. (2006). The membrane-anchored BOTRYTIS-INDUCED KINASE1 plays distinct roles in Arabidopsis resistance to necrotrophic and biotrophic pathogens. Plant Cell 18, 257–273. doi: 10.1105/tpc.105.035576
Wei, M., Wang, A., Liu, Y., Ma, L., Niu, X., and Zheng, A. (2020). Identification of the novel effector RsIA_NP8 in Rhizoctonia solani AG1 IA that induces cell death and triggers defense responses in non-host plants. Front. Microbiol. 11:1115. doi: 10.3389/fmicb.2020.01115
Wu, C., Zhou, S., Zhang, Q., Zhao, W., and Peng, Y. (2006). Molecular cloning and differential expression of an gamma-aminobutyrate transaminase gene, OsGABA-T, in rice (Oryza sativa) leaves infected with blast fungus. J. Plant Res. 119, 663–669. doi: 10.1007/s10265-006-0018-3
Yamaguchi, Y., and Huffaker, A. (2011). Endogenous peptide elicitors in higher plants. Curr. Opin. Plant Biol. 14, 351–357. doi: 10.1016/j.pbi.2011.05.001
Yamaguchi, Y., Huffaker, A., Bryan, A. C., Tax, F. E., and Ryan, C. A. (2010). PEPR2 is a second receptor for the Pep1 and Pep2 peptides and contributes to defense responses in Arabidopsis. Plant Cell 22, 508–522. doi: 10.1105/tpc.109.068874
Yamaguchi, Y., Pearce, G., and Ryan, C. A. (2006). The cell surface leucine-rich repeat receptor for AtPep1, an endogenous peptide elicitor in Arabidopsis, is functional in transgenic tobacco cells. Proc. Natl. Acad. Sci. U.S.A. 103, 10104–10109. doi: 10.1073/pnas.0603729103
Zhang, L., Kars, I., Essenstam, B., Liebrand, T. W., Wagemakers, L., Elberse, J., et al. (2014). Fungal endopolygalacturonases are recognized as microbe-associated molecular patterns by the Arabidopsis receptor-like protein responsiveness to botrytis polygalacturonases1. Plant Physiol. 164, 352–364. doi: 10.1104/pp.113.230698
Zhang, X., Wang, C., Zhang, Y., Sun, Y., and Mou, Z. (2012). The Arabidopsis mediator complex subunit16 positively regulates salicylate-mediated systemic acquired resistance and jasmonate/ethylene-induced defense pathways. Plant Cell 24, 4294–4309. doi: 10.1105/tpc.112.103317
Keywords: Rhizoctonia solani AG1-IA, EG1, gamma aminobutyric acid transaminase, allergic necrosis, disease resistance
Citation: Guo X, Chen J, Gao M and Li D (2022) An Aminobutyric Acid Transaminase in Zea mays Interacts With Rhizoctonia solani Cellulase to Participate in Disease Resistance. Front. Plant Sci. 13:860170. doi: 10.3389/fpls.2022.860170
Received: 22 January 2022; Accepted: 04 March 2022;
Published: 05 April 2022.
Edited by:
Xiaodong Wang, Agricultural University of Hebei, ChinaReviewed by:
Saeed Tarighi, Ferdowsi University of Mashhad, IranCopyright © 2022 Guo, Chen, Gao and Li. This is an open-access article distributed under the terms of the Creative Commons Attribution License (CC BY). The use, distribution or reproduction in other forums is permitted, provided the original author(s) and the copyright owner(s) are credited and that the original publication in this journal is cited, in accordance with accepted academic practice. No use, distribution or reproduction is permitted which does not comply with these terms.
*Correspondence: Duochuan Li, bGlkYzIwQHNkYXUuZWR1LmNu
Disclaimer: All claims expressed in this article are solely those of the authors and do not necessarily represent those of their affiliated organizations, or those of the publisher, the editors and the reviewers. Any product that may be evaluated in this article or claim that may be made by its manufacturer is not guaranteed or endorsed by the publisher.
Research integrity at Frontiers
Learn more about the work of our research integrity team to safeguard the quality of each article we publish.