- 1Department of Biochemistry, Cell and Molecular Biology of Plants, Estación Experimental del Zaidín, Consejo Superior de Investigaciones Científicas, Granada, Spain
- 2Department of Molecular Plant Biology, University of Turku, Turku, Finland
The sessile nature of plants forces them to face an ever-changing environment instead of escape from hostile conditions as animals do. In order to overcome this survival challenge, a fine monitoring and controlling of the status of the photosynthetic electron transport chain and the general metabolism is vital for these organisms. Frequently, evolutionary plant adaptation has consisted in the appearance of multigenic families, comprising an array of enzymes, structural components, or sensing, and signaling elements, in numerous occasions with highly conserved primary sequences that sometimes make it difficult to discern between redundancy and specificity among the members of a same family. However, all this gene diversity is aimed to sort environment-derived plant signals to efficiently channel the external incoming information inducing a right physiological answer. Oxygenic photosynthesis is a powerful source of reactive oxygen species (ROS), molecules with a dual oxidative/signaling nature. In response to ROS, one of the most frequent post-translational modifications occurring in redox signaling proteins is the formation of disulfide bridges (from Cys oxidation). This review is focused on the role of plastid thioredoxins (pTRXs), proteins containing two Cys in their active site and largely known as part of the plant redox-signaling network. Several pTRXs types have been described so far, namely, TRX f, m, x, y, and z. In recent years, improvements in proteomic techniques and the study of loss-of-function mutants have enabled us to grasp the importance of TRXs for the plastid physiology. We will analyze the specific signaling function of each TRX type and discuss about the emerging role in non-photosynthetic plastids of these redox switchers.
Introduction
Plant H2O photolysis provides electrons (and protons) to feed the photosynthetic electron transport chain (PETC) to allow NADPH and ATP synthesis for CO2 fixation. During the process, O2 and O2-derived by-products, called reactive oxygen species (ROS), are also released. However, ROS molecules (1O2, , H2O2, and •OH) are even more oxidant than O2 itself (Noctor and Foyer, 1998). ROS-exposed cellular components (proteins, lipids, polysaccharides, and DNA) can be damaged, especially under environmental conditions leading to oxidative stress. Recent works also point to NO as an emerging oxidative compound (Lamotte et al., 2005; Grun et al., 2006; Neill et al., 2008a,b; Wilson et al., 2008) The NO-derived species are called reactive nitrogen species (RNS) and S-nitrosylation is the post-translational change they promote. ROS and RNS levels are controlled by thiol peroxidase-like plastid peroxiredoxins (PRX). Nevertheless, despite their toxicity at high concentrations, ROS and RNS play an important role as central signaling molecules (Laloi et al., 2004, 2007; Bellin et al., 2013).
Thiol groups (-SH) of cysteine residues are susceptible to ROS oxidation, provoking post-translational changes leading to enzyme inactivation. Upon oxidation, new chemical species can be generated from -SH, which can be gradually oxidized to sulphenic (-SOH), sulphinic (-SOOH), and sulphonic (-SOOOH) acids, the latter being an irreversible oxidation state. When a -SOH group is in the proximity of an -SH group, a disulphide bridge can form (-S-S-). This chemical reaction is of great biological relevance because thiol/disulphide inter-conversion operates as a molecular on/off switch for redox-regulated enzymes (Couturier et al., 2013). Plants, like other organisms, have developed sensing systems to monitor and maintain optimal redox conditions to avoid metabolic collapse. These sensing and signaling mechanisms (König et al., 2012) could be considered to be true “redox eyes”.
Glutathione (GSH) and ascorbate (Asc), the most abundant antioxidant compounds in plant cells (Noctor and Foyer, 1998), are considered to be unspecific reducer molecules because of their small molecular masses. However, plants have complex enzymatic antioxidant systems composed of thioredoxins (TRX) and glutaredoxins (GRX), known under the name of redoxins (RX). On the contrary to GSH and Asc, surface topology of RX allows specific target interactions (Wangensteen et al., 2001; Barranco-Medina et al., 2009; Arsova et al., 2010). Among the first RX targets identified at the beginning of proteomic era were PRX (Baier and Dietz, 1999a,b, 2005; Dietz et al., 2006), which are involved in ROS/RNS signaling (König et al., 2012) and, as mentioned above, key ROS detoxifying enzymes. RX are highly diversified in plants and display a conserved tertiary structure (TRX folding) holding one or two Cys at their active sites. Useful reviews are available on plant GRX and their cross-talk with TRX (Rouhier et al., 2006; Xing et al., 2006; Meyer et al., 2009, 2012; Zaffagnini et al., 2012). TRX are low-redox-potential proteins (<-270 mV) of approximately 10–12 kDa with the conserved active site WC(G/P)PC (classical TRX). The Cys residues of the TRX active site switch from a reduced (sulphydryl groups) to an oxidized form (disulphide bridge) as part of the enzymatic mechanism resulting in the reduction of a target protein.
Plants are sessile eukaryotic photosynthetic organisms that have colonized multitude of terrestrial environments with fluctuating light intensities, water availability, temperature variations, and other environmental factors continuously challenging plant life. Success of this adaptation lies partially in the versatile redox signaling and regulation exerted by TRX (König et al., 2012). With the arrival of the genomics era and massive sequencing projects, many plant species have been already sequenced (e.g., Arabidopsis thaliana and Oryza sativa). The knowledge of full genomes offered the possibility of discovering tissue-specific or faintly expressed TRX, elusive before genomics. At present, the number of TRX, TRX-like proteins, or proteins with TRX-domains in Arabidopsis have risen to 44 members (Meyer et al., 2012), many of them without any assigned function. TRX are classified, according to their subcellular location and sequence similarity, into 15 subgroups (Meyer et al., 2012). While classical TRX h and o are located in cytosol/nucleus and mitochondria, respectively, five typical TRX exist in plastids, namely, TRX f, m, x, y, and z. TRX receive electrons from two compartment-specific and well-defined systems: the ferredoxin-thioredoxin system (FTS), which reduces plastid TRX with electrons coming from ferredoxin through the action of ferredoxin-thioredoxin reductase (FTR); and the NADP-thioredoxin system (NTS), involving the NADPH-thioredoxin reductase (NTR) to furnish electrons from NADPH to TRX h and o. Apart from FTS and NTS, NTRC is a bi-modular protein with NTR and TRX domains reported in 2004 (Serrato et al., 2004). Although NTRC is located in plastids, it is nevertheless reduced by NADPH (Spínola et al., 2008; Pérez-Ruiz and Cejudo, 2009) and behaves as a condensed NTS system important for the response to abiotic and oxidative stress (Serrato et al., 2004; Pérez-Ruiz et al., 2006). Besides the antioxidant role, NTRC functions related to carbon metabolism have been recently proposed (Michalska et al., 2009).
Phylogenetic studies on plastid TRX and sequence comparisons have demonstrated that while TRX m, x, y, and z are of prokaryotic origin (Sahrawy et al., 1996; Arsova et al., 2010), TRX f is closely related to eukaryotic TRX (Sahrawy et al., 1996; Issakidis-Bourguet et al., 2001). It seems reasonable that TRX diversification reflects the complexity of the plastid redox network and the extent of their role played in plant physiology. In recent years, due mainly to the availability of collections of mutant lines, many studies on plastid TRX have focused on the model plant A. thaliana. In this species, two f, four m, two y, and one x and z TRX isoforms have been described. This multiplicity has raised the question of functional redundancy or a specific role for each isoform. In this sense, Issakidis-Bourguet et al. (2001) showed that chloroplast TRX f, m, and x are differentially able to compensate for TRX deficiency in yeast. Since the discovery some decades ago of the preferential activation of chloroplast fructose-1,6-bisphosphatase (FBPase) by TRX f and NADP-malate dehydrogenase (MDH) by TRX m (Schürmann et al., 1981), linking carbon fixation and TRX-mediated activation, many other essential plastid processes such as PETC, oxidative-stress response, starch metabolism, nitrogen metabolism, lipid biosynthesis, protein folding, protein import, translation, or chaperone activity (Balmer et al., 2004; Buchanan and Balmer, 2005; Balsera et al., 2010; Chibani et al., 2010; Sanz-Barrio et al., 2012) have been reported to be under the redox regulation exerted by TRXs. Moreover, initially confined to chloroplasts, growing evidence points to new physiological functions in roots and other heterotrophic organs (Barajas-López et al., 2007; Traverso et al., 2008; Benitez-Alfonso et al., 2009; Fernández-Trijueque et al., 2012).
TRX f and m are Photosynthesis-Related Enzymes, but not Exclusively So
Discerning between functional specificity and redundancy among components of multigenic families proves difficult. Single loss-of-function lines are frequently phenotypically undistinguishable from wild-type plants. To address this question, one possibility would entail obtaining double, triple, or even quadruple loss-of-function mutants. Nevertheless, this approach is time consuming and, in the case of TRX, can be complex because of cross-talks with the GRX family. Some authors have evidenced this cross-talk by inhibiting GSH synthesis (Reichheld et al., 2007). Expression patterns (abundance and tissue location), protein topologies (determining electrostatic and/or hydrophobic interactions), redox potentials, and post-translational modifications are distinctive features that would address the specificity for each pTRX toward a particular target in a specific cell type. Described long before other pTRXs, greater information has been compiled on TRX f and m than on the x, y, or z isoforms. Most recent works have offered further insight into the specific role of TRX f and m in photosynthesis, carbohydrate metabolism, NADPH synthesis, response to abiotic stress and, notably, putative new functions in heterotrophic organs.
Redox Signaling in Photosynthesis Regulation
A large cluster of genes involved in light-harvesting reactions of photosynthesis genes coding for LHCA and LHCB (Light Harvesting Complex) proteins, protoporphyrin IX Mg chelatase, and several proteins of the photosystem I and II reaction centers (PSI and PSII, respectively) are found to be under clock control (Harmer et al., 2000). ATPase activity of Mg chelatase CHLI subunit, from the tetrapyrrole biosynthesis pathway, is activated in vitro by Pissum sativum TRX f (Luo et al., 2012). In vivo experiments with TRX f virus-induced gene-silenced plants produced no phenotype changes in the treated plants, suggesting that low levels of TRX f could be compensated for by the m-type isoform (Balmer et al., 2003; Luo et al., 2012). Nevertheless, in the same work, the silencing of pea TRX f/m induced a pale-green phenotype and ROS accumulation. The authors suggest two possible types of TRX-mediated regulations for the tetrapyrrole biosynthesis pathway: one being transcriptional regulation through plastid-mediated retrograde signaling; and another being an indirect result of the lower Mg chelatase (interacting in vitro with TRX f) activity due to lower TRX f/m activity (Figure 1A).
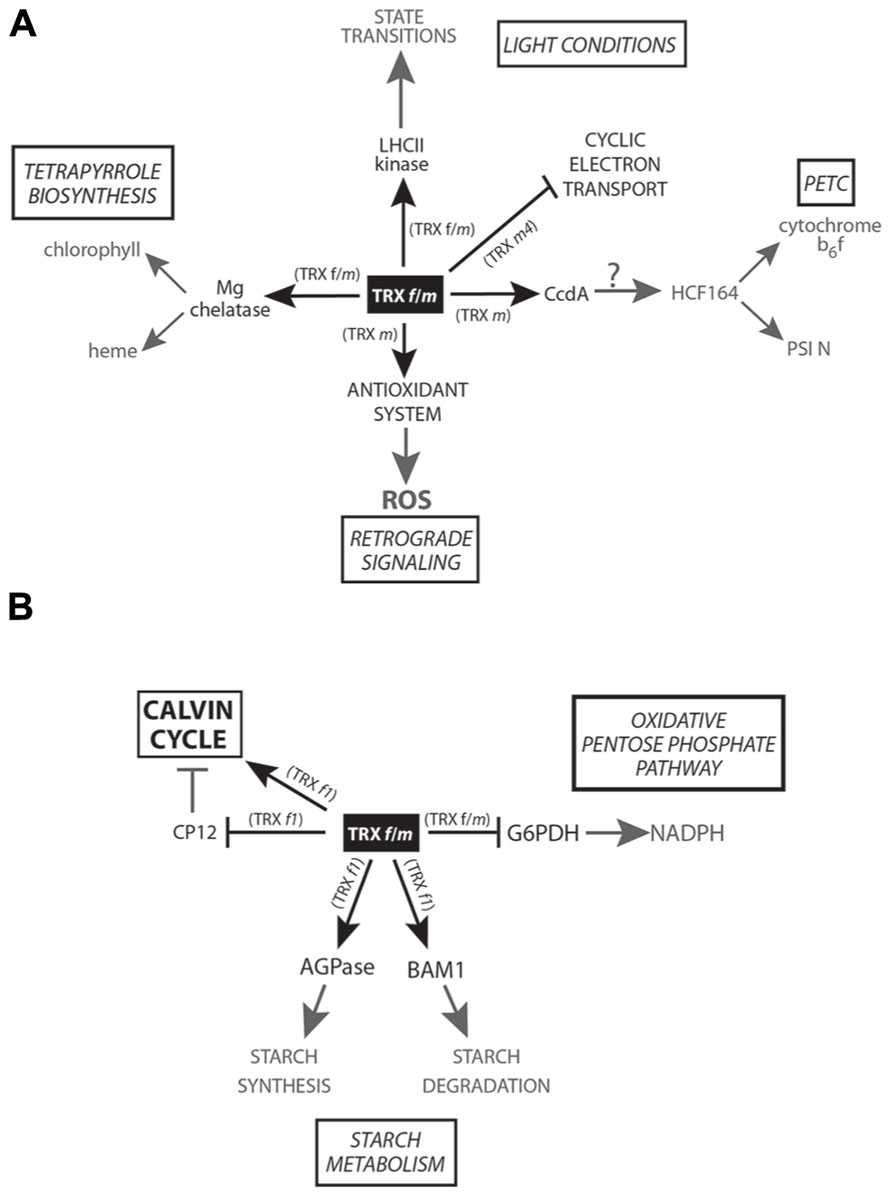
FIGURE 1. Scheme of the TRX f and m-mediated redox signaling in plastids. (A) Main physiological processes related to photosynthesis and retrograde signaling controlled by TRX f and/or m are shown in the figure. (B) Regulation of carbon metabolism carried out by TRX f and/or m.
It is known that carbon metabolism is concomitantly under this circadian rhythm. Therefore, it seems reasonable, given the tight relationship with photosynthesis and carbon fixation, that pTRX genes should have a similar transcriptional regulation in order to optimize these physiological processes. Surprisingly, only two (TRX f2 and m2) out of the six TRX f and m of A. thaliana and the pea TRX f and m1 follow a circadian rhythm (Barajas-López et al., 2011). The rest of TRXs f and m, with the exception of TRX m3, are directly induced by light. This light-independent gene expression could correspond to a more functional specificity of TRX m3 (Benitez-Alfonso et al., 2009), clustered away in phylogenetic trees based on protein-sequence comparisons (Arsova et al., 2010).
Regarding photosynthesis regulation (Figure 1A), TRX is involved in state transitions (Rintamäki et al., 2000). Both TRX f and m are able to in vivo inactivate LHC kinase in response to high light intensities, controlling the relocation of LHC between PSI and PSII under this condition. More recently, in 2006, TRX m and f were found to transfer reducing equivalents (the m-type isoform being more efficient) to HCF164, a thylakoid-membrane-spanning protein with two thioredoxin-like domains participating in the assembly of cytochrome b6f (Lennartz et al., 2001), mediating electron transport between PSII and PSI, and the reduction of the photosynthetic protein PSI-N both in vitro and in isolated thylakoids (Motohashi and Hisabori, 2006). HCF164 reduction might involve CcdA, a thylakoidal protein from bacterial origin targeted by TRX m (Motohashi and Hisabori, 2010). However, this hypothesis is only based on indirect results obtained from in vitro subcellular localization experiments and phenotype similarities between the ccda and hcf164 Arabidopsis mutants. Further in vitro and/or in vivo experimental approaches will be necessary to corroborate this putative interaction and its regulation. Very recently, the analysis of the photosynthetic parameters of an A. thaliana loss-of-function mutant has allowed to uncover a direct photosynthesis control carried out by TRX m4 (Courteille et al., 2013). According to the authors, this TRX m isoform would play an important role in regulating photosynthetic alternative electron pathways in A. thaliana and Nicotiana tabacum chloroplasts, acting as a repressor of the cyclic electron flow (CEF) involved in preserving a proper ATP/NADPH balance. Although this was the first specific function assigned to TRX m4, the physiological significance of this regulation still remains to be clarified. In this sense, the photosynthesis regulation of TRX m has been shown in rice, when the repression of OsTRX m level is responsible for a greater reduction, compared to wild-type plants, in the photosynthetic efficiency under high-irradiance treatments together with other impairments such as thylakoidal ultrastructural changes and a reduced chlorophyll and pigment content (pale-green leaves; Chi et al., 2008).
Until now, pTRX regulation of photosynthesis has been in vivo studied in C3 plants. In C4 photosynthesis, the redox state of the bundle sheat cells and mesophyll cells are expected to have very different redox status due to the spatial separation of the photosynthetic process. This C4-photosynthesis peculiarity could be influencing the relative expression pattern of the different pTRX isoforms in bundle sheat and mesophyll cells and how the photosynthesis is redox controlled in C4 plants. We hope that this intriguing topic could be addressed in a near future.
Control of Carbohydrate Metabolism in Plastids
Sugars are photosynthetic products and, recently, it has been reported that these molecules (together with thiol status in leaves) are regulating the expression of PsTRXf and m1 genes in pea plants (Barajas-López et al., 2012). This regulation is mainly exerted by glucose and sucrose and might involve the transcription factor PsDOF7, able to bind to a pea DOF motif present in PsTRX f and m1 promoters (Barajas-López et al., 2012).
Today, the redox regulation of all Calvin cycle (CC) enzymes exerted by pTRXs is widely acknowledged (Lindahl and Kieselbach, 2009; Meyer et al., 2012). Concerning redox regulation, one of the most intensely studied CC enzymes has been the chloroplast fructose-1,6-bisphosphatase (cFBPase), whose redox activation mechanism is a classical model in enzyme post-translational regulation (Jacquot et al., 1995; Chen and Xu, 1996; Hermoso et al., 1996; Jacquot et al., 1997; López Jaramillo et al., 1997; Chiadmi et al., 1999; Wangensteen et al., 2001; Cazalis et al., 2004). Nevertheless, most of the interaction evidence comes from in vitro studies. In immunocytolocalization experiments with pea chloroplasts, TRX f and m have been found to be non-randomly distributed with respect to some CC-analyzed enzymes, NADP-dependent malate dehydrogenase (NADP-MDH), heat-shock protein 70 (Hsp70), and ATP synthase (Anderson et al., 2008). Based on pTRXs co-localization with non-light activated enzymes, the authors proposed a secondary function for pTRXs as protein linkers facilitating enzyme interactions and/or substrate channeling. Additionally, it has been suggested that mechanisms by means pTRXs would exert a fine-tuned modulation of enzyme activities based on short-lived interactions, not only involving a simple binary on/off mechanism (König et al., 2012).
Apart from the pTRX-mediated activation of CC target enzymes, an additional and indirect activation mode involving CP12, a small chloroplast protein containing four redox-active Cys, was described in the 1990s (Wedel et al., 1997). In its oxidized state, CP12 forms an inhibited complex (Figure 1B) with the CC enzymes NADPH-glyceraldehyde-3-phosphate dehydrogenase (GAPDH) and phosphoribulokinase (PRK), fully restored upon pTRXs reduction (Marri et al., 2009). In plant cells, pTRXs/CP12 system broadens the redox regulatory complexity, providing a faster activation of CC oxidized enzymes and pointing to TRX f1 as the physiological reducer of GAPDH/CP12/PRK complexes in A. thaliana. Further in vivo studies have highlighted the importance of CP12 in carbon partitioning and growth in tobacco plants, leading to proposal of functions other than the single formation of PRK and GAPDH complexes (Howard et al., 2011).
Plastid synchronization of the starch synthesis/degradation processes is crucial for plant growth and development. ADP-glucose pyrophosphorylase (AGPase) is a key redox-activated enzyme for starch biosynthesis in plastids (Fu et al., 1998; Ballicora et al., 2000). Very recently, in Arabidopsis leaves, the role of TRX f1 as AGPase activator (Figure 1B) during light period has been evidenced (Thormählen et al., 2013). In a loss-of-function trx f1 mutant, a lower redox activation of AGPase and a decrease in the starch:sucrose ratio have been detected during the day. AmongTRX f1, m1, x, and y1, AGPase is more efficiently in vitro activated by TRX f1 (followed by TRX m1). These authors hypothesized that AGPase could be activated by TRX f1 during the light period while NTRC would be the activating enzyme in the dark. However, other authors have reported contradictory experimental results and contend that redox modulation is of minor importance for AGPase activity in response to light (Li et al., 2012). Nevertheless, further experiments will be necessary to clarify this important point.
Surprisingly, not only starch synthesis is TRX f1 controlled but also degradation through the redox-activated enzyme BAM1 (Figure 1B), an A. thaliana β-amylase controlling stomata opening (Valerio et al., 2011). Although TRX f1 activates both starch synthesis and degradation during the day, these processes take place in different cell types (mesophyll cells and guard cells, respectively). However, light degradation in mesophyll tissue is activated during osmotic-stress situations that trigger BAM1 induction. BAM1 is also expressed in Arabidopsis roots, where NTRC (proved to be less efficient than TRX f in in vitro assays) is the putative activating enzyme (Valerio et al., 2011). Besides the presumed role of NTRC as a redox alternative activator under dark conditions and/or in non-photosynthetic organs, it is feasible that classical pTRXs (the specifically expressed in non-photosynthetic organs) could be activated by the heterotrophic ferredoxin NADP reductases (FNR) isoforms and NADPH (Hanke et al., 2004; Balmer et al., 2006; Barajas-López et al., 2007; Bohrer et al., 2012; Fernández-Trijueque et al., 2012). Despite that some works have focused on the study of pTRX-mediated redox regulation in non-photosynthetic organs, much effort still needs to be done in order to ascertain the true extent of pTRXs in heterotrophic tissues.
Oxidative Activation of Glucose-6-Phosphate Dehydrogenase
Glucose-6-phosphate dehydrogenase (G6PDH) catalyzes the first committed step of the oxidative pentose phosphate pathway (OPPP), a major source of NADPH for plant heterotrophic cells as well as for photosynthetic tissues during the night period. Six genes coding for G6PDH have been identified in Arabidopsis, four predicted to code for plastid isoforms (Wakao and Benning, 2005). The higher number of G6PDH identified in plastids points to the significance of OPPP taking place in this subcellular compartment. In vitro assays have shown the reductive inactivation by DTT, a common feature of at least three out of the four plastidial enzymes (AtG6PDH1, AtG6PDH2, and AtG6PDH3) and not shared with the cytosolic isoforms. AtG6PDH1 is expressed mostly in photosynthetic tissues while AtG6PDH2 and AtG6PDH3 transcripts are accumulated predominantly in roots (Wakao and Benning, 2005). Although a specific TRX m-mediated G6PDH inactivation (Figure 1B) has been previously reported (Wenderoth et al., 1997), Née et al. (2009) have demonstrated that A. thaliana TRX f1 regulate AtG6PDH1 activity in vitro as efficiently as TRX m1 or m4. Nevertheless, these in vitro results must be carefully interpreted and need to be supported by complementary in vivo interaction approaches or by determining the in vivo redox state of G6PDH1 in a TRX f1 loss-of-function mutant. In addition, it would be helpful to know whether pTRXs also regulate root-expressed AtG6PDH2 and AtG6PDH3 in order to elucidate the role of pTRXs in OPPP control and NADPH synthesis. It is tempting to conclude that some of the pTRXs could control their own redox status through the activation/inactivation of OPPP in heterotrophic organs.
ROS Homeostasis in Roots
TRX m3 transcripts are one of the least abundant pTRXs mRNA in leaves, while higher root-transcript levels are comparable to those of TRX m2, m4, and x (Bohrer et al., 2012). Nevertheless, until now, the published results highlight the importance of TRX m3 for root ROS homeostasis (Benitez-Alfonso et al., 2009; Benitez-Alfonso and Jackson, 2009). The results shown in this work localize TRX m3 in root and shoot meristem plastids, this isoform being important for callose deposition and plasmodesmal transport, as well as for arresting the growth of TRX m3 loss-of-function seedlings (gat1 mutant). It is quite surprising, however, that, given the redundancy of ROS detoxifying mechanisms in plant cells and the existing interplay between TRX and GRX signaling pathways, in vital plant meristems, the ROS content was not buffered by other TRX isoforms or GRX members also expressed in roots (Bohrer et al., 2012; Meyer et al., 2012). In fact, other authors hold that the lethality due to TRX m3 inactivation needs to be firmly established (Reichheld et al., 2010).
Although some studies have experimentally proved the presence of Pisum sativum TRX f and m isoforms in roots (Barajas-López et al., 2007) and the response of TRX m to NaCl-induced stress in root pea seedlings (Fernández-Trijueque et al., 2012) there is no information about the precise tissue expression in this organ (excepting TRX m3). According to the online available microarray data from “Arabidopsis eFP Browser” (http://bar.utoronto.ca/efp/cgi-bin/efpWeb.cgi), TRX m2 would be the most abundantly expressed gene in roots, principally in procambium cells. In contrast, TRX m1 would be pericycle and phloem specific while TRX m4 could be mostly expressed in cortex and procambium tissues. The analysis of the data suggests a poor expression in A. thaliana of both TRX f genes in roots compared to the other TRX m genes. Nonetheless, although these data may help to have an approximate idea about the pTRX accumulation in root tissues, we must taking into account that putative further post-transcriptional control could alter the final protein-expression pattern.
TRX x and y: The Subset of pTRXs Specialized in Responding to Oxidative Stress
The first reports describing TRX x and y as members of the plant pTRXs appeared in 2003 and 2004, respectively (Collin et al., 2003; Collin et al., 2004). It bears noting that TRX x, y, and z have higher redox potentials (>-340 mV) than do TRX f and m (<-350 mV; Collin et al., 2003; Collin et al., 2004; Chibani et al., 2011). As mentioned above, one of the key TRX features determining their functional specificity is redox potential. This biochemical characteristic confers these proteins a poor capacity to activate carbon-metabolism enzymes such as FBPase and MDH. On the contrary, x- and y-type TRX can efficiently activate plastid 2-Cys PRX and PRX Q, respectively (Figure 2). The absence of TRX x in the A. thaliana mutant trxx triggers protein carbonylation (stress marker) but does not affect photosynthesis or carbon fixation under long-day conditions (Pulido et al., 2010). Nevertheless, under continuous-light conditions, CO2 fixation is affected in trxx, suggesting that TRX x can undertake a more important role under non-optimal environmental conditions (Pulido et al., 2010).
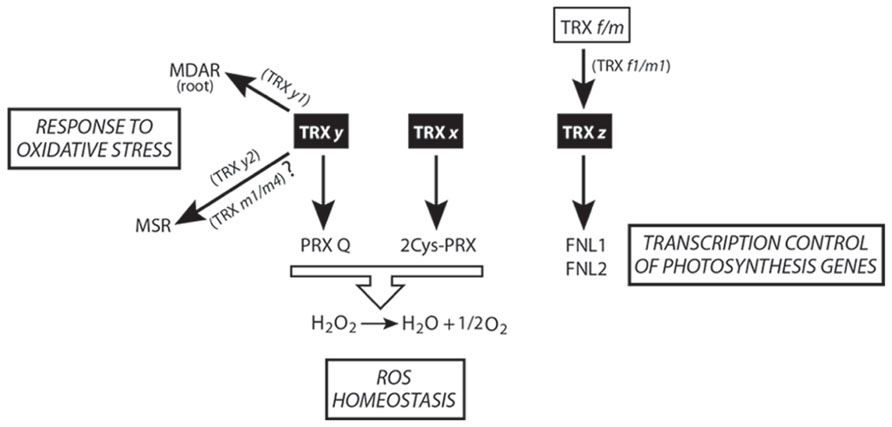
FIGURE 2. Scheme of TRX x, y, and z-mediated redox signaling in plastids. The central physiological processes regulated by these isoforms are shown.
Two y-type isoforms, TRX y1 and y2, are present in Arabidopsis. These oxidoreductases do not activate FBPase and only partially MDH, being efficient reducers of PRX Q (Collin et al., 2004). In Arabidopsis plants, TRX y1 is preferentially expressed in heterotrophic organs (e.g., roots and seeds), while TRX y2 is a more photosynthesis-associated protein. Expression in heterotrophic organs of the y1-type implies the existence of a functional redox system, furnishing reducing power to these TRX. In this sense, Marchand and colleagues reported a list of TRX y targets in Arabidopsis roots, finding numerous proteins involved in detoxification and defense (Figure 2) like monodehydroascorbatereductase (MDAR; Marchand et al., 2010). Notably, although y-type TRX cannot activate FBPase, another of the root-found targets is a putative fructose bisphosphate aldolase, whose reaction product that serves as a substrate for cFBPase and that participates in plant responses to abiotic stress (Lu et al., 2012).
Recently, from the analysis of methionine sulfoxidereductase (MSR) capacity in different TRX loss-of-function mutants, TRX y2 has been proposed as the physiological electron donor of MSR (Figure 2; Laugier et al., 2013). However, according to the results shown in this work, overlapping functions of TRX m1 and m4 as MSR activators cannot be ruled out.
TRX z, A Redox Regulator of the Plastid Transcription
Although a Solanum lycopersicum TRX z ortholog (CITRX) has been reported as an interacting cytosolic partner of the resistance protein Cf-9 (Rivas et al., 2004), in 2006 this protein was identified for the first time as a component of plastid transcriptionally active chromosomes (TACs) from mustard (Sinapis alba) and Arabidopsis (Pfalz et al., 2006) and, 4 years later, named as TRX z (Arsova et al., 2010) or TRX p (Meng et al., 2010) and designated as a new member of pTRXs. The lack of this protein affects transcription (Figure 2) of genes dependent on plastid-encoded RNA polymerase (PEP), essentially photosynthetic-related genes (class I; Arsova et al., 2010). Consequently, A. thaliana trxz has yellow leaves and lacks the ability of autotrophic growth while in Nicotiana benthamiana low TRX z protein levels induce a chlorotic phenotype (Arsova et al., 2010; Meng et al., 2010). Two fructokinase-like proteins (FLN1 and FNL2) reportedly interact in planta with TRX z in a thiol-dependent way. Recombinant FLN1 and FLN2 lack any sugar-phosphorylating activity, suggesting a regulatory rather than a metabolic function (Arsova et al., 2010). Several pieces of evidence, such as in planta interaction between FLNs and TRX z, a similar Arabidopsis leaf phenotype of the trx z and the FLN1 and FNL2 silenced mutants, and a reduced expression of PEP-dependent class I genes in both mutants suggest that TRX z and FLN1 and FLN2 might take part in a signaling pathway, regulating PEP activity in chloroplasts (Arsova et al., 2010). It is noteworthy that, in a parallel work, both TRX z and FNL1, together with other redox proteins, have been reported to take part of PEP complexes in mustard (Schröter et al., 2010). Curiously, the TRX f-target FBPase (Schürmann et al., 1981) is among the proteins found in mustard transcriptional complexes.
Biochemical assays with poplar TRX z have shown that this protein can be reduced by NTRB (Chibani et al., 2010), physiologically important in the case that TRX z is dually targeted to plastids and cytosol (Rivas et al., 2004). The activation of some peroxidases and MSR has led Chibani et al. (2011) to propose TRX z as an alternative electron donor to ROS-detoxifying enzymes. Arabidopsis TRX z is able to form dimers in its oxidized state, being monomerized upon reduction by DTT and, unlike TRX x, y, and f1, is the first pTRX not reduced by FTR (Bohrer et al., 2012). The higher redox potential of TRX z with respect to other pTRXs prompted to Bohrer and colleagues to conduct in vitro reduction assays by using other pTRXs. TRX f1 and m1 behaved as good TRX z reducers, being the first available case of TRX reduced by other TRX (Figure 2). However, additional in vivo experiments (as the determination of the reduction/oxidation TRX z state in loss-of-function pTRX mutants) need to be performed in order to corroborate these intriguing results.
Plastid Cystathionine β-Synthase Domain-Containing Proteins Regulate pTRXs Activity
In the literature, no activating-TRX protein has been reported prior to the work of Yoo et al. (2011), which demonstrated the activating role of plastid cystathionine β-synthase (CBS) domain-containing proteins (CDCPs) over FTS and NTS. CDCPs are members of a large superfamily of ubiquitous proteins able to bind to adenosine-containing ligands such as AMP, ATP, or S-adenosyl methionine (Yoo et al., 2011). In A. thaliana and rice, 34 and 59 CDCPs have been reported, respectively (Kushwaha et al., 2009). CDCPs are located in different subcellular compartments. Two of these proteins, CBSX1 and CBSX2, are located in plastids and are able to activate TRX f, m, x, and y (Yoo et al., 2011). The loss-of-function mutant cbsx1 shows severe growth retardation while CBSX1 overexpressing plants are able to grow faster in free-sucrose medium and display a delayed senescence compared to wild-type plants, resembling transgenic plants overexpressing TRX m (Benitez-Alfonso et al., 2009). Notably, the authors have suggested that CBSX1 would regulate physiological processes in non-green tissues while CBSX2 would be a green-tissue specific protein, reinforcing the above-mentioned idea of the presence of a fully active FTS in heterotrophic organs.
Concluding Remarks
Sometimes, phenotypic differences between TRX mutant lines and wild-type plants are subtle or even missing. However, in order to see whether novel isoforms have conferred adaptive advantages during evolution it would be necessary to perform population-dynamics studies of loss-of-function mutants grown under natural conditions. Although we know that this approach would be time-consuming and difficult to develop, it would give a definite answer to the perennial question of the functional specificity or redundancy of the members of the family of TRX. Additionally, to find a putative relationship between environment adaptation and diversification of plant pTRXs, it would be interesting to analyze whether there would be differences between the number of pTRX isoforms found in plant species living in extreme environments (e.g., deserts) compared with other species living in more stable environments (e.g., rain forests). It is quite probable that the diversification of the pTRXs also responds, at least in part, to the demand of a more complex redox signaling due to the appearance of new specialized organs (and plastid types), as roots and flowers, necessary for the successful land colonization. In roots and some flower tissues, and instead of chloroplasts, specialized non-green plastids are present. It would be logical to think that plants have reprogrammed or adapted the redox-signaling machinery already present in green plastids to redox regulate the light-independent processes occurring in non-green plastids. However, with the exception of a few works already mentioned in this review, there is very little information about the pTRX targets in heterotrophic organs and the light-independent processes they are redox regulating. Further comprehensive studies are still necessary to realize the extent of the redox-signaling role mediated by pTRX in the whole plant.
In our opinion, when we study multigenic families, we almost exclusively focus our attention on the gene coding sequences and on comparative analyses of the primary structures of the peptides they are coding for. However, regulatory sequences (DNA motifs) present in promoters are also a basic part of genes (and sometimes neglected). When revising TRX literature it is quite usual to find in vitro interaction experiments in which two or more pTRX isoforms are reported to have the same or similar affinity for a given target. For instance, Arabidopsis TRX f2 isoform has been omitted from the in vitro interaction experiments of some works reasoning a high sequence similarity with TRX f1. We think that the diversification of the TRX family and other multigenic families could also respond to a plant strategy leading to a more efficient transcriptional regulation. The increase in the number of plant transcription factors and the complexity of the transcriptional machinery could have compromised gene regulation and, consequently, plant survival. One solution could have consisted in organizing regulatory DNA motifs in several promoters. These regulatory sequences could have co-evolved together with the coding regions following a gene duplication event. During evolution, both the promoter changes as the amino acid substitutions of the pTRXs could allow a precise and specific redox signaling in non-green plastid of the heterotrophic organs.
As we have pointed out, pTRXs redox proteins may regulate a large number of plant physiological processes, and compelling evidence points toward the existence of fully active pTRXs in heterotrophic tissues. According to the works cited in this review, three pTRX functional subsets can be inferred (Figure 3). The first subgroup, related to photosynthesis and carbon metabolism, would be composed of the TRXs f and m isoforms, coupling light and redox-signaling pathways (excluding the Arabidopsis TRX m3 isoform, possibly developing a specific physiological role). In the second subcategory, we could find the x- and y-type TRXs, involved mostly in ROS detoxification and taking part of the complex redox-signaling network regulating plant development. The last subset, related to redox signaling and regulation of photosynthesis-related transcription in chloroplasts, would be composed of only one member, i.e., TRX z. Intriguingly, TRXs f and m are efficient TRX z reducers. Subsequently, it is tempting to conclude that f- and m-type isoforms, in addition to regulate the photosynthesis-related processes mentioned in this review, could also act as redox-signaling molecules linking photosynthesis and plastid transcription. In our opinion, the discovery of CDCPs as pTRX activity regulators should be taken into account in order to improve our knowledge of the external elements modulating the multilevel redox signaling mediated by pTRXs.
Conflict of Interest Statement
The authors declare that the research was conducted in the absence of any commercial or financial relationships that could be construed as a potential conflict of interest.
Acknowledgment
This work was supported by BIO2012-33292 from the “Ministerio de Economía y Competitividad” and “Fondo Europeo de Desarrollo Regional” and grants from Junta de Andalucía (Grupo BIO 154), Spain. Juan Fernández-Trijueque was supported by a predoctoral fellowship from “Ministerio de Educación” (Spain).
References
Anderson, L. E., Fadowole, D., Reyes, B. A., and Carol, A. A. (2008). Distribution of thioredoxin f and m with respect to seven light-activated enzymes and three redox-insensitive proteins in pea leaf chloroplasts. Plant Sci. 174, 432–445. doi: 10.1016/j.plantsci.2008.01.001
Arsova, B., Hoja, U., Wimmelbacher, M., Greiner, E., Ustun, S., Melzer, M., et al. (2010). Plastidial thioredoxin z interacts with two fructokinase-like proteins in a thiol-dependent manner: evidence for an essential role in chloroplast development in Arabidopsis and Nicotiana benthamiana. Plant Cell 22, 1498–1515. doi: 10.1105/tpc.109.071001
Baier, M., and Dietz, K. J. (1999a). Alkyl hydroperoxide reductases: the way out of the oxidative breakdown of lipids in chloroplasts. Trends Plant Sci. 4, 166–168. doi: 10.1016/S1360-1385(99)01398-9
Baier, M., and Dietz, K. J. (1999b). Protective function of chloroplast 2-cysteine peroxiredoxin in photosynthesis. Evidence from transgenic Arabidopsis. Plant Physiol. 119, 1407–1414. doi: 10.1104/pp.119.4.1407
Baier, M., and Dietz, K. J. (2005). Chloroplasts as source and target of cellular redox regulation: a discussion on chloroplast redox signals in the context of plant physiology. J. Exp. Bot. 56, 1449–1462. doi: 10.1093/jxb/eri161
Balmer, Y., Koller, A., Del Val, G., Manieri, W., Schurmann, P., and Buchanan, B. B. (2003). Proteomics gives insight into the regulatory function of chloroplast thioredoxins. Proc. Natl. Acad. Sci. U.S.A. 100, 370–375. doi: 10.1073/pnas.232703799
Balmer, Y., Koller, A., Del Val, G. D., Schurmann, P., and Buchanan, B. B. (2004). Proteomics uncovers proteins interacting electrostatically with thioredoxin in chloroplasts. Photosynth. Res. 79, 275–280. doi: 10.1023/B:PRES.0000017207.88257.d4
Balmer, Y., Vensel, W. H., Cai, N., Manieri, W., Schürmann, P., Hurkman, W. J., et al. (2006). A complete ferredoxin/thioredoxin system regulates fundamental processes in amyloplasts. Proc. Natl. Acad. Sci. U.S.A. 103, 2988–2993. doi: 10.1073/pnas.0511040103
Balsera, M., Soll, J., and Buchanan, B. B. (2010). Redox extends its regulatory reach to chloroplast protein import. Trends Plant Sci. 15, 515–521. doi: 10.1016/j.tplants.2010.06.002
Ballicora, M. A., Frueauf, J. B., Fu, Y., Schürmann, P., and Preiss, J. (2000). Activation of the potato tuber ADP-glucose pyrophosphorylase by thioredoxin. J. Biol. Chem. 275, 1315–1320. doi: 10.1074/jbc.275.2.1315
Barajas-López, J., Serrato, A. J., Cazalis, R., Meyer, Y., Chueca, A., Reichheld, J. P., et al. (2011). Circadian regulation of chloroplastic f and m thioredoxins through control of the CCA1 transcription factor. J. Exp. Bot. 62, 2039–2051. doi: 10.1093/jxb/erq394
Barajas-López, J., Serrato, A. J., Olmedilla, A., Chueca, A., and Sahrawy, M. (2007). Localization in roots and flowers of pea chloroplastic thioredoxin f and thioredoxin m proteins reveals new roles in non-photosynthetic organs. Plant Physiol. 145, 946–960. doi: 10.1104/pp.107.105593
Barajas-López, J., Tezycka, J., Travaglia, C. N., Serrato, A. J., Chueca, A., Thormahlen, I., et al. (2012). Expression of the chloroplast thioredoxins f and m is linked to short-term changes in the sugar and thiol status in leaves of Pisum sativum. J. Exp. Bot. 63, 4887–4900. doi: 10.1093/jxb/ers163
Barranco-Medina, S., Lázaro, J. J., and Dietz, K. J. (2009). The oligomeric conformation of peroxiredoxins links redox state to function. FEBS Lett. 583, 1809–1816. doi: 10.1016/j.febslet.2009.05.029
Bellin, D., Asai, S., Delledonne, M., and Yoshioka, H. (2013). Nitric oxide as a mediator for defense responses. Mol. Plant Microbe Interact. 26, 271–277. doi: 10.1094/MPMI-09-12-0214-CR
Benitez-Alfonso, Y., Cilia, M., San Roman, A., Thomas, C., Maule, A., Hearn, S., et al. (2009). Control of Arabidopsis meristem development by thioredoxin-dependent regulation of intercellular transport. Proc. Natl. Acad. Sci. U.S.A. 106, 3615–3620. doi: 10.1073/pnas.0808717106
Benitez-Alfonso, Y., and Jackson, D. (2009). Redox homeostasis regulates plasmodesmal communication in Arabidopsis meristems. Plant Signal. Behav. 4, 655–659. doi: 10.4161/psb.4.7.8992
Bohrer, A. S., Massot, V., Innocenti, G., Reichheld, J. P., Issakidis-Bourguet, E., and Vanacker, H. (2012). New insights into the reduction systems of plastidial thioredoxins point out the unique properties of thioredoxin z from Arabidopsis. J. Exp. Bot. 63, 6315–6323. doi: 10.1093/jxb/ers283
Buchanan, B. B., and Balmer, Y. (2005). Redox regulation: a broadening horizon. Annu. Rev. Plant Biol. 56, 187–220. doi: 10.1146/annurev.arplant.56.032604.144246
Cazalis, R., Chueca, A., Sahrawy, M., and López-Gorgé, J. (2004). Construction of chimeric cytosolic fructose-1,6-bisphosphatases by insertion of a chloroplastic redox regulatory cluster. J. Physiol. Biochem. 60, 7–21. doi: 10.1007/BF03168216
Collin, V., Issakidis-Bourguet, E., Marchand, C., Hirasawa, M., Lancelin, J. M., Knaff, D. B., et al. (2003). The Arabidopsis plastidial thioredoxins: new functions and new insights into specificity. J. Biol. Chem. 278, 23747–23752. doi: 10.1074/jbc.M302077200
Collin, V., Lamkemeyer, P., Miginiac-Maslow, M., Hirasawa, M., Knaff, D. B., Dietz, K. J., et al. (2004). Characterization of plastidial thioredoxins from Arabidopsis belonging to the new y-type. Plant Physiol. 136, 4088–4095. doi: 10.1104/pp.104.052233
Courteille, A., Vesa, S., Sanz-Barrio, R., Cazale, A. C., Becuwe-Linka, N., Farran, I., et al. (2013). Thioredoxin m4 controls photosynthetic alternative electron pathways in Arabidopsis. Plant Physiol. 161, 508–520. doi: 10.1104/pp.112.207019
Couturier, J., Chibani, K., Jacquot, J. P., and Rouhier, N. (2013). Cysteine-based redox regulation and signaling in plants. Front. Plant Sci. 4:105. doi: 10.3389/fpls.2013.00105
Chen, Y., and Xu, G. J. (1996). Redox modifications of spinach chloroplast fructose-1,6-bisphosphatase. Biochem. Mol. Biol. Int. 39, 941–948. doi: 10.1080/15216549600201092
Chi, Y. H., Moon, J. C., Park, J. H., Kim, H. S., Zulfugarov, I. S., Fanata, W. I., et al. (2008). Abnormal chloroplast development and growth inhibition in rice thioredoxin m knock-down plants. Plant Physiol. 148, 808–817. doi: 10.1104/pp.108.123547
Chiadmi, M., Navaza, A., Miginiac-Maslow, M., Jacquot, J. P., and Cherfils, J. (1999). Redox signalling in the chloroplast: structure of oxidized pea fructose-1,6-bisphosphate phosphatase. EMBO J. 18, 6809–6815. doi: 10.1093/emboj/18.23.6809
Chibani, K., Couturier, J., Selles, B., Jacquot, J. P., and Rouhier, N. (2010). The chloroplastic thiol reducing systems: dual functions in the regulation of carbohydrate metabolism and regeneration of antioxidant enzymes, emphasis on the poplar redoxin equipment. Photosynth. Res. 104, 75–99. doi: 10.1007/s11120-009-9501-8
Chibani, K., Tarrago, L., Schurmann, P., Jacquot, J. P., and Rouhier, N. (2011). Biochemical properties of poplar thioredoxin z. FEBS Lett. 585, 1077–1081. doi: 10.1016/j.febslet.2011.03.006
Dietz, K. J., Jacob, S., Oelze, M. L., Laxa, M., Tognetti, V., De Miranda, S. M., et al. (2006). The function of peroxiredoxins in plant organelle redox metabolism. J. Exp. Bot. 57, 1697–1709. doi: 10.1093/jxb/erj160
Fernández-Trijueque, J., Barajas-López J. D., Chueca, A., Cazalis, R., Sahrawy, M., and Serrato, A. J. (2012). Plastid thioredoxins f and m are related to the developing and salinity response of post-germinating seeds of Pisum sativum. Plant Sci. 188-189, 82–88. doi: 10.1016/j.plantsci.2012.01.006
Fu, Y., Ballicora, M. A., Leykam, J. F., and Preiss, J. (1998). Mechanism of reductive activation of potato tuber ADP-glucose pyrophosphorylase. J. Biol. Chem. 273, 25045–25052. doi: 10.1074/jbc.273.39.25045
Grun, S., Lindermayr, C., Sell, S., and Durner, J. (2006). Nitric oxide and gene regulation in plants. J. Exp. Bot. 57, 507–516. doi: 10.1093/jxb/erj053
Hanke, G. T., Kurisu, G., Kusunoki, M., and Hase, T. (2004). Fd: FNR electron transfer complexes: evolutionary refinement of structural interactions. Photosynth. Res. 81, 317–327. doi: 10.1023/B:PRES.0000036885.01534.b8
Harmer, S. L., Hogenesch, J. B., Straume, M., Chang, H. S., Han, B., Zhu, T., et al. (2000). Orchestrated transcription of key pathways in Arabidopsis by the circadian clock. Science 290, 2110–2113. doi: 10.1126/science.290.5499.2110
Hermoso, R., Castillo, M., Chueca, A., Lázaro, J. J., Sahrawy, M., and López-Gorgé, J. L. (1996). Binding site on pea chloroplast fructose-1,6-bisphosphatase involved in the interaction with thioredoxin. Plant Mol. Biol. 30, 455–465. doi: 10.1007/BF00049324
Howard, T. P., Fryer, M. J., Singh, P., Metodiev, M., Lytovchenko, A., Obata, T., et al. (2011). Antisense suppression of the small chloroplast protein CP12 in tobacco alters carbon partitioning and severely restricts growth. Plant Physiol. 157, 620–631. doi: 10.1104/pp.111.183806
Issakidis-Bourguet, E., Mouaheb, N., Meyer, Y., and Miginiac-Maslow, M. (2001). Heterologous complementation of yeast reveals a new putative function for chloroplast m-type thioredoxin. Plant J. 25, 127–135. doi: 10.1046/j.0960-7412.2000.00943.x
Jacquot, J. P., López-Jaramillo, J., Chueca, A., Cherfils, J., Lemaire, S., Chedozeau, B., et al. (1995). High-level expression of recombinant pea chloroplast fructose-1,6-bisphosphatase and mutagenesis of its regulatory site. Eur. J. Biochem. 229, 675–681. doi: 10.1111/j.1432-1033.1995.tb20513.x
Jacquot, J. P., López-Jaramillo, J., Miginiac-Maslow, M., Lemaire, S., Cherfils, J., Chueca, A., et al. (1997). Cysteine-153 is required for redox regulation of pea chloroplast fructose-1,6-bisphosphatase. FEBS Lett. 401, 143–147. doi: 10.1016/S0014-5793(96)01459-7
König, J., Muthuramalingam, M., and Dietz, K. J. (2012). Mechanisms and dynamics in the thiol/disulfide redox regulatory network: transmitters, sensors and targets. Curr. Opin. Plant Biol 15, 261–268. doi: 10.1016/j.pbi.2011.12.002
Kushwaha, H. R., Singh, A. K., Sopory, S. K., Singla-Pareek, S. L., and Pareek, A. (2009). Genome wide expression analysis of CBS domain containing proteins in Arabidopsis thaliana (L.) Heynh and Oryza sativa L. reveals their developmental and stress regulation. BMC Genomics 10:200. doi: 10.1186/1471-2164-10-200
Laloi, C., Apel, K., and Danon, A. (2004). Reactive oxygen signalling: the latest news. Curr. Opin. Plant Biol. 7, 323–328. doi: 10.1016/j.pbi.2004.03.005
Laloi, C., Stachowiak, M., Pers-Kamczyc, E., Warzych, E., Murgia, I., and Apel, K. (2007). Cross-talk between singlet oxygen- and hydrogen peroxide-dependent signaling of stress responses in Arabidopsis thaliana. Proc. Natl. Acad. Sci. U.S.A. 104, 672–677. doi: 10.1073/pnas.0609063103
Lamotte, O., Courtois, C., Barnavon, L., Pugin, A., and Wendehenne, D. (2005). Nitric oxide in plants: the biosynthesis and cell signalling properties of a fascinating molecule. Planta 221, 1–4. doi: 10.1007/s00425-005-1494-8
Laugier, E., Tarrago, L., Courteille, A., Innocenti, G., Eymery, F., Rumeau, D., et al. (2013). Involvement of thioredoxin y2 in the preservation of leaf methionine sulfoxide reductase capacity and growth under high light. Plant Cell Environ. 36, 670–682. doi: 10.1111/pce.12005
Lennartz, K., Plucken, H., Seidler, A., Westhoff, P., Bechtold, N., and Meierhoff, K. (2001). HCF164 encodes a thioredoxin-like protein involved in the biogenesis of the cytochrome b(6)f complex in Arabidopsis. Plant Cell 13, 2539–2551. doi: 10.1105/tpc.010245
Li, J., Almagro, G., Munoz, F. J., Baroja-Fernández, E., Bahaji, A., Montero, M., et al. (2012). Post-translational redox modification of ADP-glucose pyrophosphorylase in response to light is not a major determinant of fine regulation of transitory starch accumulation in Arabidopsis leaves. Plant Cell Physiol. 53, 433–444. doi: 10.1093/pcp/pcr193
Lindahl, M., and Kieselbach, T. (2009). Disulphide proteomes and interactions with thioredoxin on the track towards understanding redox regulation in chloroplasts and cyanobacteria. J Proteomics 72, 416–438. doi: 10.1016/j.jprot.2009.01.003
López Jaramillo, J., Chueca, A., Jacquot, J. P., Hermoso, R., Lazaro, J. J., Sahrawy, M., et al. (1997). High-yield expression of pea thioredoxin m and assessment of its efficiency in chloroplast fructose-1,6-bisphosphatase activation. Plant Physiol. 114, 1169–1175. doi: 10.1104/pp.114.4.1169
Lu, W., Tang, X., Huo, Y., Xu, R., Qi, S., Huang, J., et al. (2012). Identification and characterization of fructose 1,6-bisphosphate aldolase genes in Arabidopsis reveal a gene family with diverse responses to abiotic stresses. Gene 503, 65–74. doi: 10.1016/j.gene.2012.04.042
Luo, T., Fan, T., Liu, Y., Rothbart, M., Yu, J., Zhou, S., et al. (2012). Thioredoxin redox regulates ATPase activity of magnesium chelatase CHLI subunit and modulates redox-mediated signaling in tetrapyrrole biosynthesis and homeostasis of reactive oxygen species in pea plants. Plant Physiol. 159, 118–130. doi: 10.1104/pp.112.195446
Marchand, C. H., Vanacker, H., Collin, V., Issakidis-Bourguet, E., Marechal, P. L., and Decottignies, P. (2010). Thioredoxin targets in Arabidopsis roots. Proteomics 10, 2418–2428. doi: 10.1002/pmic.200900835
Marri, L., Zaffagnini, M., Collin, V., Issakidis-Bourguet, E., Lemaire, S. D., Pupillo, P., et al. (2009). Prompt and easy activation by specific thioredoxins of calvin cycle enzymes of Arabidopsis thaliana associated in the GAPDH/CP12/PRK supramolecular complex. Mol. Plant 2, 259–269. doi: 10.1093/mp/ssn061
Meng, L., Wong, J. H., Feldman, L. J., Lemaux, P. G., and Buchanan, B. B. (2010). A membrane-associated thioredoxin required for plant growth moves from cell to cell, suggestive of a role in intercellular communication. Proc. Natl. Acad. Sci. U.S.A. 107, 3900–3905. doi: 10.1073/pnas.0913759107
Meyer, Y., Belin, C., Delorme-Hinoux, V., Reichheld, J. P., and Riondet, C. (2012). Thioredoxin and glutaredoxin systems in plants: molecular mechanisms, crosstalks, and functional significance. Antioxid. Redox. Signal. 17, 1124–1160. doi: 10.1089/ars.2011.4327
Meyer, Y., Buchanan, B. B., Vignols, F., and Reichheld, J. P. (2009). Thioredoxins and glutaredoxins: unifying elements in redox biology. Annu. Rev. Genet. 43, 335–367. doi: 10.1146/annurev-genet-102108-134201
Michalska, J., Zauber, H., Buchanan, B. B., Cejudo, F. J., and Geigenberger, P. (2009). NTRC links built-in thioredoxin to light and sucrose in regulating starch synthesis in chloroplasts and amyloplasts. Proc. Natl. Acad. Sci. U.S.A. 106, 9908–9913. doi: 10.1073/pnas.0903559106
Motohashi, K., and Hisabori, T. (2006). HCF164 receives reducing equivalents from stromal thioredoxin across the thylakoid membrane and mediates reduction of target proteins in the thylakoid lumen. J. Biol. Chem. 281, 35039–35047. doi: 10.1074/jbc.M605938200
Motohashi, K., and Hisabori, T. (2010). CcdA is a thylakoid membrane protein required for the transfer of reducing equivalents from stroma to thylakoid lumen in the higher plant chloroplast. Antioxid. Redox. Signal. 13, 1169–1176. doi: 10.1089/ars.2010.3138
Née, G., Zaffagnini, M., Trost, P., and Issakidis-Bourguet, E. (2009). Redox regulation of chloroplastic glucose-6-phosphate dehydrogenase: a new role for f-type thioredoxin. FEBS Lett. 583, 2827–2832. doi: 10.1016/j.febslet.2009.07.035
Neill, S., Barros, R., Bright, J., Desikan, R., Hancock, J., Harrison, J., et al. (2008a). Nitric oxide, stomatal closure, and abiotic stress. J. Exp. Bot. 59, 165–176. doi: 10.1093/jxb/erm293
Neill, S., Bright, J., Desikan, R., Hancock, J., Harrison, J., and Wilson, I. (2008b). Nitric oxide evolution and perception. J. Exp. Bot. 59, 25–35. doi: 10.1093/jxb/erm218
Noctor, G., and Foyer, C. H. (1998). ASCORBATE AND GLUTATHIONE: keeping active oxygen under control. Annu. Rev. Plant Physiol. Plant Mol. Biol. 49, 249–279. doi: 10.1146/annurev.arplant.49.1.249
Pérez-Ruiz, J. M., and Cejudo, F. J. (2009). A proposed reaction mechanism for rice NADPH thioredoxin reductase C, an enzyme with protein disulfide reductase activity. FEBS Lett. 583, 1399–1402. doi: 10.1016/j.febslet.2009.03.067
Pérez-Ruiz, J. M., Spínola, M. C., Kirchsteiger, K., Moreno, J., Sahrawy, M., and Cejudo, F. J. (2006). Rice NTRC is a high-efficiency redox system for chloroplast protection against oxidative damage. Plant Cell 18, 2356–2368. doi: 10.1105/tpc.106.041541
Pfalz, J., Liere, K., Kandlbinder, A., Dietz, K. J., and Oelmuller, R. (2006). pTAC2, -6, and -12 are components of the transcriptionally active plastid chromosome that are required for plastid gene expression. Plant Cell 18, 176–197. doi: 10.1105/tpc.105.036392
Pulido, P., Spínola, M. C., Kirchsteiger, K., Guinea, M., Pascual, M. B., Sahrawy, M., et al. (2010). Functional analysis of the pathways for 2-Cys peroxiredoxin reduction in Arabidopsis thaliana chloroplasts. J. Exp. Bot. 61, 4043–4054. doi: 10.1093/jxb/erq218
Reichheld, J. P., Khafif, M., Riondet, C., Droux, M., Bonnard, G., and Meyer, Y. (2007). Inactivation of thioredoxin reductases reveals a complex interplay between thioredoxin and glutathione pathways in Arabidopsis development. Plant Cell 19, 1851–1865. doi: 10.1105/tpc.107.050849
Reichheld, J. P., Riondet, C., Delorme, V., Vignols, F., and Meyer, Y. (2010). Thioredoxins and glutaredoxins in development. Plant Sci. 178, 420–423. doi: 10.1016/j.plantsci.2010.03.001
Rintamäki, E., Martinsuo, P., Pursiheimo, S., and Aro, E. M. (2000). Cooperative regulation of light-harvesting complex II phosphorylation via the plastoquinol and ferredoxin-thioredoxin system in chloroplasts. Proc. Natl. Acad. Sci. U.S.A. 97, 11644–11649. doi: 10.1073/pnas.180054297
Rivas, S., Rougon-Cardoso, A., Smoker, M., Schauser, L., Yoshioka, H., and Jones, J. D. (2004). CITRX thioredoxin interacts with the tomato Cf-9 resistance protein and negatively regulates defence. EMBO J. 23, 2156–2165. doi: 10.1038/sj.emboj.7600224
Rouhier, N., Couturier, J., and Jacquot, J. P. (2006). Genome-wide analysis of plant glutaredoxin systems. J. Exp. Bot. 57, 1685–1696. doi: 10.1093/jxb/erl001
Sahrawy, M., Hecht, V., Lopez-Jaramillo, J., Chueca, A., Chartier, Y., and Meyer, Y. (1996). Intron position as an evolutionary marker of thioredoxins and thioredoxin domains. J. Mol. Evol. 42, 422–431. doi: 10.1007/BF02498636
Sanz-Barrio, R., Fernández-San Millan, A., Carballeda, J., Corral-Martínez, P., Segui-Simarro, J. M., and Farran, I. (2012). Chaperone-like properties of tobacco plastid thioredoxins f and m. J. Exp. Bot. 63, 365–379. doi: 10.1093/jxb/err282
Schröter, Y., Steiner, S., Matthai, K., and Pfannschmidt, T. (2010). Analysis of oligomeric protein complexes in the chloroplast sub-proteome of nucleic acid-binding proteins from mustard reveals potential redox regulators of plastid gene expression. Proteomics 10, 2191–2204. doi: 10.1002/pmic.200900678
Schürmann, P., Maeda, K., and Tsugita, A. (1981). Isomers in thioredoxins of spinach chloroplasts. Eur. J. Biochem. 116, 37–45. doi: 10.1111/j.1432-1033.1981.tb05297.x
Serrato, A. J., Pérez-Ruiz, J. M., Spínola, M. C., and Cejudo, F. J. (2004). A novel NADPH thioredoxin reductase, localized in the chloroplast, which deficiency causes hypersensitivity to abiotic stress in Arabidopsis thaliana. J. Biol. Chem. 279, 43821–43827. doi: 10.1074/jbc.M404696200
Spínola, M. C., Pérez-Ruiz, J. M., Pulido, P., Kirchsteiger, K., Guinea, M., González, M., et al. (2008). NTRC new ways of using NADPH in the chloroplast. Physiol. Plant. 133, 516–524. doi: 10.1111/j.1399-3054.2008.01088.x
Thormählen, I., Ruber, J., Von Roepenack-Lahaye, E., Ehrlich, S. M., Massot, V., Hummer, C., et al. (2013). Inactivation of thioredoxin f1 leads to decreased light activation of ADP-glucose pyrophosphorylase and altered diurnal starch turnover in leaves of Arabidopsis plants. Plant Cell Environ. 36, 16–29. doi: 10.1111/j.1365-3040.2012.02549.x
Traverso, J. A., Vignols, F., Cazalis, R., Serrato, A. J., Pulido, P., Sahrawy, M., et al. (2008). Immunocytochemical localization of Pisum sativum TRXs f and m in non-photosynthetic tissues. J. Exp. Bot. 59, 1267–1277. doi: 10.1093/jxb/ern037
Valerio, C., Costa, A., Marri, L., Issakidis-Bourguet, E., Pupillo, P., Trost, P., et al. (2011). Thioredoxin-regulated beta-amylase (BAM1) triggers diurnal starch degradation in guard cells, and in mesophyll cells under osmotic stress. J. Exp. Bot. 62, 545–555. doi: 10.1093/jxb/erq288
Wakao, S., and Benning, C. (2005). Genome-wide analysis of glucose-6-phosphate dehydrogenases in Arabidopsis. Plant J. 41, 243–256. doi: 10.1111/j.1365-313X.2004.02293.x
Wangensteen, O. S., Chueca, A., Hirasawa, M., Sahrawy, M., Knaff, D. B., and López-Gorgé, J. (2001). Binding features of chloroplast fructose-1,6-bisphosphatase-thioredoxin interaction. Biochim. Biophys. Acta 1547, 156–166. doi: 10.1016/S0167-4838(01)00178-9
Wedel, N., Soll, J., and Paap, B. K. (1997). CP12 provides a new mode of light regulation of Calvin cycle activity in higher plants. Proc. Natl. Acad. Sci. U.S.A. 94, 10479–10484. doi: 10.1073/pnas.94.19.10479
Wenderoth, I., Scheibe, R., and Von Schaewen, A. (1997). Identification of the cysteine residues involved in redox modification of plant plastidic glucose-6-phosphate dehydrogenase. J. Biol. Chem. 272, 26985–26990. doi: 10.1074/jbc.272.43.26985
Wilson, I. D., Neill, S. J., and Hancock, J. T. (2008). Nitric oxide synthesis and signalling in plants. Plant Cell Environ. 31, 622–631. doi: 10.1111/j.1365-3040.2007.01761.x
Xing, S., Lauri, A., and Zachgo, S. (2006). Redox regulation and flower development: a novel function for glutaredoxins. Plant Biol. (Stuttg.) 8, 547–555. doi: 10.1055/s-2006-924278
Yoo, K. S., Ok, S. H., Jeong, B. C., Jung, K. W., Cui, M. H., Hyoung, S., et al. (2011). Single cystathionine beta-synthase domain-containing proteins modulate development by regulating the thioredoxin system in Arabidopsis. Plant Cell 23, 3577–3594. doi: 10.1105/tpc.111.089847
Keywords: thioredoxin, redox signaling, photosynthesis, oxidative stress, carbon metabolism
Citation: Serrato AJ, Fernández-Trijueque J, Barajas-López J-D, Chueca A and Sahrawy M (2013) Plastid thioredoxins: a “one-for-all” redox-signaling system in plants. Front. Plant Sci. 4:463. doi: 10.3389/fpls.2013.00463
Received: 15 July 2013; Accepted: 28 October 2013;
Published online: 21 November 2013.
Edited by:
Jean-Philippe Reichheld, Centre National de la Recherche Scientifique, FranceReviewed by:
Xinguang Zhu, Chinese Academy of Sciences, ChinaEzequiel Petrillo, University of Buenos Aires, Argentina
Copyright © 2013 Serrato, Fernández-Trijueque, Barajas-López, Chueca and Sahrawy. This is an open-access article distributed under the terms of the Creative Commons Attribution License (CC BY). The use, distribution or reproduction in other forums is permitted, provided the original author(s) or licensor are credited and that the original publication in this journal is cited, in accordance with accepted academic practice. No use, distribution or reproduction is permitted which does not comply with these terms.
*Correspondence: Mariam Sahrawy, Department of Biochemistry, Cell and Molecular Biology of Plants, Estación Experimental del Zaidín, Consejo Superior de Investigaciones Científicas, Profesor Albareda 1, 18008 Granada, Spain e-mail: mariam.sahrawy@eez.csic.es