- 1Department of Neurology, University Hospital of Würzburg, Würzburg, Germany
- 2Department of Anesthesiology, Intensive Care, Emergency Medicine and Pain Therapy, University Hospital of Würzburg, Würzburg, Germany
At any moment in time, cells coordinate and balance their calcium ion (Ca2+) fluxes. The term ‘Ca2+ homeostasis’ suggests that balancing resting Ca2+ levels is a rather static process. However, direct ER Ca2+ imaging shows that resting Ca2+ levels are maintained by surprisingly dynamic Ca2+ fluxes between the ER Ca2+ store, the cytosol, and the extracellular space. The data show that the ER Ca2+ leak, continuously fed by the high-energy consuming SERCA, is a fundamental driver of resting Ca2+ dynamics. Based on simplistic Ca2+ toolkit models, we discuss how the ER Ca2+ leak could contribute to evolutionarily conserved Ca2+ phenomena such as Ca2+ entry, ER Ca2+ release, and Ca2+ oscillations.
Introduction
The tight control of coordinated homeostatic calcium ion (Ca2+) fluxes is of fundamental importance for cellular signaling and health (Berridge et al., 2003). Evolutionary conserved mechanisms maintain Ca2+ levels in every cell type, while different adaptions modulate cell type-specific functions. The major intracellular Ca2+ store is the endoplasmic reticulum (ER) (Verkhratsky, 2005). Despite its central role in the pathophysiology of many severe diseases (Mekahli et al., 2011), our understanding of how ER Ca2+ fluxes shape cellular Ca2+ signaling is still poor. One reason is that Ca2+ signals are typically monitored in the cytosol. With the improvement of ER Ca2+ imaging techniques, it became technically possible to directly monitor Ca2+ dynamics in the ER, with reasonably good spatiotemporal resolution (Rehberg et al., 2008; Samtleben et al., 2013; Rodriguez-Garcia et al., 2014; de Juan-Sanz et al., 2017; Schulte et al., 2022). These experiments unraveled a surprisingly pronounced physiological role of the ER Ca2+ leak in shaping Ca2+ signals (Thastrup et al., 1989; Camello et al., 2002; Flourakis et al., 2006; Samtleben et al., 2015; Lemos et al., 2021).
Principles and limitations of ER Ca2+ imaging
In the cytosol, the resting Ca2+-concentration is about 100 nM. Upon stimulation, cytosolic Ca2+ concentrations rise to 0.5–1 µM and can reach tens of micromolar close to active Ca2+-channels (Bootman and Bultynck, 2020). In the ER lumen, Ca2+ concentrations are in the range of ∼50 µM up to 1 mM. For this reason, ER Ca2+ indicators need a low affinity for Ca2+ [dissociation constant (Kd) ∼100–200 µM] while maintaining high responsiveness to Ca2+.
For ER Ca2+ imaging, three fundamentally different principles were developed. One is based on the direct loading of cells with synthetic acetoxymethyl (AM)-estered Ca2+ indicators, such as Mag-Fura2-AM [Kd ∼ 25–50 µM (Hofer and Machen, 1993; Solovyova and Verkhratsky, 2002)], Mag-Fluo4 [Kd ∼ 22 μM (Laude et al., 2005)] or Fluo5N [∼90 μM (Chen et al., 2015)]. The technique is well-suited for some cell types; however, a certain amount of the indicator becomes reactive in the cytosol, thereby causing ‘mixed’ ER-cytosol signals. To remove undesirable indicator from the cytosol, cells can be permeabilized (e.g., with digitonin or streptolysin) or dialyzed with the help of a patch-clamp pipette (Solovyova and Verkhratsky, 2002). In permeabilized cells, Mag-Fluo4 leaking out of the ER can also be quenched with an antibody (Rossi and Taylor, 2020). Surprisingly, after quenching, Mag-Fluo4-AM showed a much higher KdCa2+-value in the ER (∼1 mM instead of 22 µM), most likely due to incomplete de-esterification in the ER lumen (Rossi and Taylor, 2020).
A strategy to accumulate synthetic Ca2+ indicators in the ER lumen of non-disrupted cells is targeted esterase-induced dye loading (TED) (Rehberg et al., 2008; Samtleben et al., 2013). For TED, a genetically overexpressed carboxylesterase hydrolyses a synthetic low-affinity acetoxymethyl (AM) ester in the ER lumen, thereby forming a hydrophilic dye/Ca2+ complex. The fluorescent Ca2+-dye complex is trapped and enriched in the ER lumen and provides an excellent signal-to-noise ratio (Rehberg et al., 2008). The best available indicator for TED is still Fluo5N-AM (Samtleben et al., 2013). The de-estered, Ca2+-sensitive form of Fluo5N is detectable in the ER for hours. In the cytosol, Fluo5N is reactive but barely visible. Unfortunately, Fluo5N is extremely light sensitive (bleaching and random flashing), making it difficult to image Fluo5N/Ca2+ complexes (Samtleben et al., 2013; Schulte et al., 2022).
The third strategy is based on ER-targeted low-affinity GECIs (genetically encoded Ca2+ indicator) such as the D1ER-derivate D4ER (Kipanyula et al., 2012), CEPIAer (Suzuki et al., 2014), ER-GCaMP6-150/210 (de Juan-Sanz et al., 2017) or ER-GAP-derivates (Rodriguez-Garcia et al., 2014; Alonso et al., 2017). For GECIs, high expression levels using a strong vector promoter are required to achieve an appropriate GECI signal. This increases the risk of protein misfolding or mistargeting by saturating the ER translocation and ER retention and retrieval processes.
We recently compared TED using Fluo5N with the GECI ER-GCaMP6-150. The data showed that TED is well suited to visualize fast Ca2+ signal onsets (Schulte et al., 2022). ER-GCaMP6-150 showed excellent on-off rates, was quite bleach resistant and allowed imaging for up to 1 h on the same cells (Schulte et al., 2022). In all our direct ER imaging experiments, ‘typical’ excitation light conditions could stop ongoing ER Ca2+ oscillations within ∼2 min (Schulte et al., 2022), albeit the indicators themselves were still reactive. We observed the phenomenon, loss of reactivity, in all types of cells we ever investigated (Hek293, HeLa, BHK21, astrocytes, neurons). We do not have an explanation for this observation. Hence, extreme low excitation light conditions might be needed for all ER Ca2+ imaging experiments as the light sensitivity of ER Ca2+ dynamics might be of biological and not methodological origin.
Nowadays, for dual-color Ca2+ imaging (ER/cytosol), a green-fluorescent ER Ca2+ indicator and a red fluorescent cytosolic dye are a good combination (Rodríguez-Prados et al., 2020; Schulte et al., 2022). We recommend using a GECI, such as ER-GCaMP6-150/210, with AM-ester based loading of the cytosolic dye Cal-590 (Birkner and Konnerth, 2019; Schulte et al., 2022). Fluorescence of both dyes can be well separated with standard fluorescence microscopy. The excellent signal-to-noise ratio of Cal-590 allows low-light illumination conditions and does not destroy ER Ca2+ dynamics (Schulte et al., 2022).
Is there a defined ‘resting’ ER Ca2+ concentration?
In physiology, the extracellular and cytosolic ion concentrations are well defined. This is not true for the ER Ca2+ concentration. Resting ER Ca2+ levels were described to be in the range of 50 µM up to 1 mM; meaning a difference factor of ×20. Depending on cell type, indicator, or calibration approach, resting ER Ca2+ concentrations range between 60 and 270 µM in cultured sensory neurons (Solovyova et al., 2002), 700–800 µM in HeLa and Hek293 cells (Tang et al., 2011), ∼150 µM in primary hippocampal neurons (de Juan-Sanz et al., 2017), and ∼400 µM in cultured astrocytes (Rodríguez-Prados et al., 2020).
It is not easy to determine the exact ‘resting’ ER Ca2+ concentration in living cells. The ER Ca2+ range can be estimated in permeabilized [‘leaky cells’ (Streb et al., 1983)] or disrupted [‘whole-cell patch clamp (Solovyova et al., 2002)] conditions, after blockade of the SERCA. Standardized Ca2+ calibration solutions (zero Ca2+ to ∼2–5 mM free Ca2+) are applied extracellularly until an equilibrium state is formed between the extracellular space, the cytosol, and the ER lumen. However, permeabilized cells are no longer in a physiological state, and potential loss of small-molecule Ca2+ dyes may confound Ca2+ calibration. Also, it is difficult to provide a ‘resting’ ER Ca2+ level for living cells with unknown Ca2+ toolkit and physiological state.
We imaged an entire calibration process for ∼20 min with a temporal resolution of 5 Hz (Schulte et al., 2022). The data confirmed that Ca2+ concentration in the ER lumen is about thousand-fold higher than in the cytosol and about 10–20x times lower than in the extracellular space (Schulte et al., 2022). Notably, the ER Ca2+ store can be rapidly refilled, within seconds, when the SERCA is blocked (Schulte et al., 2022). It can well be that this fast passive ER refilling in permeabilized cells is a ‘calcium tunnelling’ phenomenon (Petersen et al., 2017) and occurs through the ER Ca2+ leak channels.
The ER Ca2+ leak, a surprisingly strong intracellular Ca2+ flux
In a simplified view, a living cell generates the resting membrane potential by potassium ions that leak from inside the cell to the outside, via K+ ‘leak’ channels. The driving force of the potassium gradient is maintained by the high energy consuming Na+/K+ ATPase. The fundamental principle is similar for Ca2+ fluxes from the ER Ca2+ store to the cytosol. A very high force drives the Ca2+ from the ER lumen through the ER Ca2+ leak channels into the cytosol, an effect which might be electrogenic (Burdakov et al., 2005; Verkhratsky, 2005). The molecular identity of the ER Ca2+ leak is not entirely clear (Lemos et al., 2021), but there is strong experimental evidence that the Sec61 translocon complex is one of the main mediators of passive ER Ca2+ leak (Flourakis et al., 2006; Schäuble et al., 2012). Sec61 complexes are evolutionarily highly conserved, are ubiquitous, and transcriptome data revealed that they are expressed at very high levels. Sec61 complexes are non-redundant proteins involved in protein synthesis (Lang et al., 2017), meaning that a cell cannot fully avoid ER Ca2+ leak. To maintain ER Ca2+ levels, high activity of the SERCA (sarcoplasmic/endoplasmic reticulum Ca2+ ATPase) is needed.
An easy way to unmask the ER Ca2+ leak is by blocking the SERCA, irreversibly with the drug thapsigargin (Thastrup et al., 1989), or acutely with CPA (cyclopiazonic acid) (Samtleben et al., 2015). The rate at which SERCA blockade empties the ER can vary widely from cell to cell. Direct ER Ca2+ imaging, however, suggests that the ER Ca2+ leak is a strong, temperature-dependent, persistent intracellular Ca2+ flux. In neurons, for instance, SERCA blockade with CPA depletes the ER Ca2+ store within 1–2 min (Samtleben et al., 2015; de Juan-Sanz et al., 2017). In cultured astrocytes, acute application of SERCA blocking agents [thapsigargin (Schulte et al., 2022) or tBHQ (Rodriguez-Prados et al., 2020)] caused a drop in the fluorescence signal (F) from Frest to Fmin in about a minute.
Ca2+-imaging in the cytosol is not well-suited to investigate the spatio-temporal dynamics of the ER Ca2+ leak. In neurons, in presence of extracellular Ca2+, SERCA blockade induces Ca2+ entry over the plasma membrane, thus masking the contribution of the ER Ca2+ leak to the cytosolic Ca2+ transient. In Ca2+-free extracellular solution, the expected cytosolic Ca2+ signal is often barely detectable, because both the ER and cytosolic Ca2+ are rapidly lost to extracellular sites (Samtleben et al., 2015).
The ER Ca2+ leak triggers homeostatic Ca2+ fluxes
Existence of an evolutionarily conserved ER Ca2+ leak raises the question of its influence on homeostatic Ca2+ fluxes (minimal model in Figure 1A). When we blocked neuronal activity of hippocampal neurons and removed extracellular Ca2+ acutely, the ER Ca2+ signal dropped to a Fmin plateau signal within a few minutes (Figure 1B) (Samtleben et al., 2015). Subsequent acute addition of CPA did not further reduce the ER Ca2+ signal. Similarly, metabotropic ER Ca2+ release was abolished in cerebellar Purkinje cells that were kept in Ca2+-free solution for some minutes, as shown with cytosolic Ca2+ imaging (Hartmann et al., 2014). Removal of extracellular Ca2+ appear to empty the ER Ca2+ store virtually completely in some minutes.
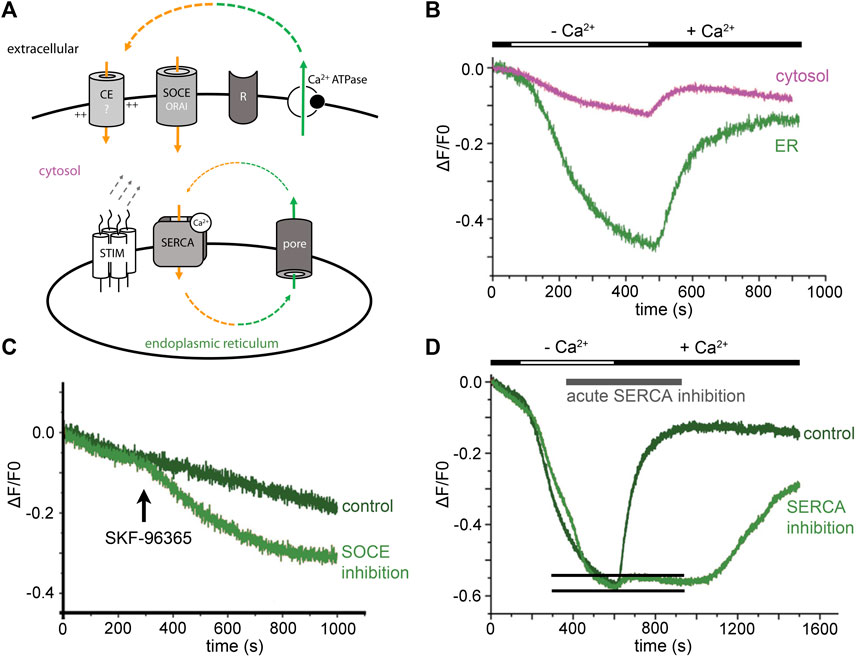
FIGURE 1. ER Ca2+ leak in neurons triggers resting Ca2+ entry. (A) Minimal model: ER Ca2+ leak and cellular Ca2+ loss (green) need to be counterbalanced from extracellular sides (orange). Activity of the SERCA closes the Ca2+ loop. Leaking Ca2+ is partly rescued from the cytosol by the SERCA, but this cannot prevent ER Ca2+ depletion in Ca2+-free solution. The best candidates for homeostatic Ca2+ influx are ORAI channels and yet unknown Ca2+ entry (CE?) mediators. (B) During extracellular Ca2+ withdrawal, ER Ca2+ levels start to drop within seconds. Extracellular Ca2+ is needed to counterbalance homeostatic ER Ca2+ loss. (C) Inhibition of Ca2+ entry by short application of the SOCE-inhibitor SKF-96365 (25 µM) reduces ER Ca2+ levels. (D) ER Ca2+ refilling with and without acute SERCA blockade. A short, transient ER Ca2+ signal is observed in presence of the SERCA inhibitor (indicated by two black lines). The original experiments were performed in primary hippocampal neurons, during neuronal activity blockade with 100 nM tetrodotoxin. Cells were not stimulated via any receptor (R). Cytosolic Ca2+ signals were measured with Oregon Green 488 BAPTA-1 and are shown in magenta. TED with Fluo5N-AM were used for direct ER Ca2+ imaging (green lines in A–C). [Modified according to (Samtleben et al., 2015)].
Evidently, passive ER Ca2+ loss cannot be restored from cytosolic calcium by SERCA activity alone. Hence, a constitutively active resting Ca2+ entry is needed to maintain ER Ca2+ levels. The phenomenon in which depletion of the intracellular Ca2+-store activates Ca2+ influx is called store-operated Ca2+ entry (capacitive Ca2+ entry) (Putney et al., 2017). We tested SOCE-blockers to find out whether a constitutively active calcium entry mechanism compensates passive ER Ca2+ loss. The data confirmed that acute application of SOCE-blockers (SKF-96365/BTP-2) induces an immediate drop in ER Ca2+ levels (Figure 1C) (Samtleben et al., 2015). Thus, resting Ca2+ influx over the plasma membrane exists and is, in the end, triggered by the ER Ca2+ leak and maintained by SERCA activity (summarized in Figure 1A).
The resting Ca2+ influx is functionally relevant as it is a distinct mechanism for regulating gene expression (Lalonde et al., 2014) and seem to also trigger local Ca2+ influx events, so-called ‘signal-close-to-noise Ca2+ activity’ (Prada et al., 2018). The Ca2+ toolkit underlying homeostatic Ca2+ influx mechanisms is not well known (Figure 1A) but in hippocampal neurons, it is resistant to an inhibitor cocktail containing TTX (for voltage-gated sodium channels), APV and CNQX (to block ionotropic glutamate receptors), and Ni2+-ions (to reduce activity of low-threshold activated VGCCs) (Prada et al., 2018). We think that constitutive active ORAI channels contribute to ER-leak-triggered, homeostatic Ca2+ influx (Figure 1A).
Why are neurons or astrocytes investing so much energy in maintaining homeostatic Ca2+ fluxes via the extracellular space? Perhaps, resting Ca2+ fluxes are needed to signal neuronal health. More SOCE-like Ca2+ entry or less active removal of cytosolic Ca2+ would lead to cellular Ca2+ overload. This has clinical implications. For instance, when SOCE blockers are used to prevent acute or neurodegenerative Ca2+ overload, resting homeostatic Ca2+ influx would be reduced. This would also reduce ER Ca2+ levels and thereby induce ER stress signaling (Mekahli et al., 2011) and mitochondrial dysfunction (Garbincius and Elrod, 2022).
SERCA-independent ER refilling
Theoretically, fast passive Ca2+ influx from the extracellular side might be enough to locally refill the ER lumen. In a direct ER Ca2+ imaging experiment, we emptied the ER Ca2+ store of neurons in Ca2+-free solution (Figure 1D). When we re-added extracellular Ca2+ and blocked the SERCA acutely, a short, transient increase in ER Ca2+ levels was observed (Samtleben et al., 2015). We cannot exclude incomplete block of the SERCA in this experiment. However, the transient-like character of the signal suggests that Ca2+ enters the ER passively and is then lost through the ER Ca2+ leak. Future developments in life-cell imaging combined with super-resolution techniques might solve the question whether there are regulated ‘tunnel-like’ microdomains between the ER lumen and the extracellular space. General models for Ca2+ tunnelling mechanisms are discussed since many years (Petersen et al., 2017). It can well be that ER Ca2+ sparks (Cheng and Lederer, 2008) depend on such a ‘tunnel-like’ microdomain. Proximity of ‘leaky’ ER microdomains, ORAI, and Stim complexes might be a minimal requirement for electrogenic, local Ca2+ signals. The fluxes should be sufficient to trigger voltage-dependent Ca2+ influx as well as local induction of Ca2+-induced Ca2+ release (Ca2+-iCR) (see later).
Shaping of Ca2+ fluxes by the ER Ca2+ leak: Clues from cultured astrocytes
In our recent work, we used cultured cortical astrocytes (mouse) and dual color Ca2+ imaging (ER/cytosol) to find out how ER Ca2+ dynamics shape homeostatic Ca2+ fluxes. Astrocytes are well suited as a prototypical Ca2+ model (Verkhratsky and Nedergaard, 2018; Lim et al., 2021). The cells are very responsive and can be cultured with high purity, which makes it easy to determine their Ca2+ toolkit, e.g., with RNA-seq (Hasel et al., 2017; Schulte et al., 2022). The cell model expresses two IP3 receptors (Itpr1 and Itpr2), but no ryanodine receptors. Notably, there is just one SERCA (SERCA2, Atp2a2) and a high amount of plasma membrane Ca2+-ATPases (Atp2b1, Atp2b4). Sec61a and other ER Ca2+ leak channel candidates (e.g., Tmco1, presenilin) are highly expressed (Schulte et al., 2022). Based on transcriptome data and analysis of calcium signal profiles observed in individual cells, minimal models can be designed to discuss how the ER Ca2+ leak could shape Ca2+ signals.
The ER Ca2+ leak is interlinked with Ca2+-induced Ca2+ release
Cultured cortical astrocytes show depolarization-dependent ER Ca2+ release (Rodríguez-Prados et al., 2020), but do not express ryanodine receptors (Hasel et al., 2017; Schulte et al., 2022). Surprisingly, ER Ca2+ refilling by Ca2+ entry can induce Ca2+-iCR, which empties the ER Ca2+ store again, and keeps cytosolic Ca2+ levels high (Figure 2A) (Schulte et al., 2022). In astrocytes, the effect was observed: 1) after adenosine-induced Ca2+ release in Ca2+-free solution and delayed re-adding of extracellular Ca2+ (Figure 2A, middle panel); 2) during ER replenishment by homeostatic Ca2+ entry after depleting the ER Ca2+ store in Ca2+-free solution (Figure 2A, lower panel; for neurons see Figure 1B). The effect might be mediated by IP3-receptors, or ER Ca2+ leak channels by a yet unknown mechanism. Returning to resting ER Ca2+ levels would then require cytosolic Ca2+ export to extracellular sides, e.g. by active transport via Ca2+-ATPases or secondary active transport mechanisms (Na+/Ca2+ exchanger).
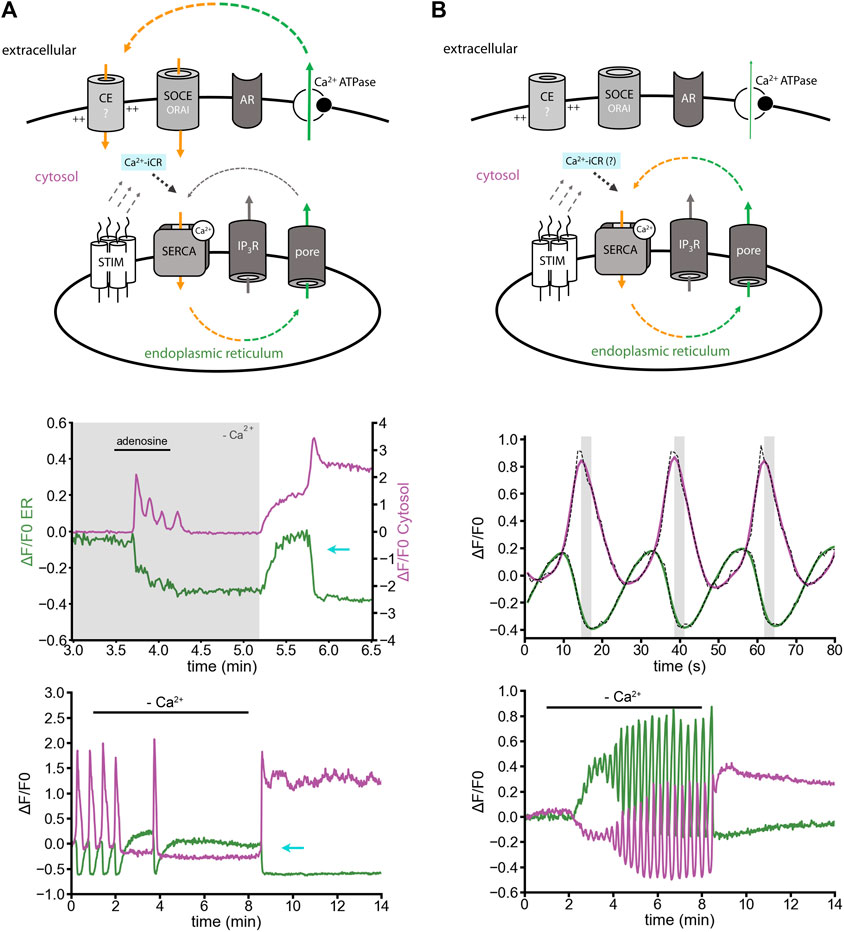
FIGURE 2. Induced ER Ca2+ release versus ER Ca2+ oscillation in cultured astrocytes. Simultaneous imaging of ER and cytosolic Ca2+ with the indicators: ER-GCaMP6-150 (green) and Cal-590-AM (magenta) in non-disrupted cells. (A) Minimal model: Ca2+-induced Ca2+ release (Ca2+-iCR, cyan) is induced by SOCE-like Ca2+ entry after ER replenishment. Middle panel: In Ca2+-free solution, adenosine-induced Ca2+ oscillations persist for about half a minute. Delayed Ca2+ replenishment induces a Ca2+-iCR phenomenon, in absence of ryanodine receptors (cyan arrow). Lower panel: Removal of extracellular Ca2+ and subsequent re-adding of extracellular Ca2+ can be sufficient to stimulate Ca2+-iCR. (B) (Minimal model) Spontaneous Ca2+ oscillation in Ca2+-free solution. (Middle panel) Spontaneous Ca2+ oscillations are shaped by a circular relationship between ER and cytosolic signals. Simultaneous imaging revealed a time lag of some seconds between peak signals in the cytosol and the ER Ca2+ release signal (grey). Raw traces (black dashed line) and the low-pass filtered traces (in color) are plotted. Lower panel: Spontaneous Ca2+ oscillation in Ca2+-free solution. The sarco-/endoplasmic reticulum Ca2+ ATPase (SERCA) recycles intracellular Ca2+. Candidates for ER Ca2+ leak are IP3 receptors and ER Ca2+ leak channels. Ca2+ released from the ER stays in the cell, is not exported to extracellular sides (compare with Figure 3), and the SERCA adapts its activity to the cytosolic Ca2+ concentration. [Modified according to (Schulte et al., 2022)].
Is the ER Ca2+ leak the basic trigger for spontaneous Ca2+ oscillations?
Ca2+ oscillations in astrocytes can also appear spontaneously, without an obvious external stimulator. Ca2+ oscillations are often linked to changing speed of ER Ca2+ efflux, depending on IP3 receptor activity or IP3 metabolism (Dupont et al., 2011). In our view, increased IP3 levels are certainly a trigger of Ca2+ oscillations, though it is likely not maintaining the Ca2+ oscillation (Figure 2B). ER/cytosol Ca2+ signals during spontaneous Ca2+ oscillations are in a non-linear (circular) slope relationship with a spatiotemporal time-lag in the range of seconds (Schulte et al., 2022). Furthermore, oscillatory ER Ca2+ fluxes can go on for minutes, in presence and absence of extracellular Ca2+ (Figure 2B, lower panel) (Schulte et al., 2022).
We would like to suggest the following model: a passive ER Ca2+ leak pore, like Sec61a, maybe in concert with other passive ER Ca2+ leak mediators (Lemos et al., 2021), mediates a constant ER Ca2+ flux to the cytosol. The ER Ca2+ leak is powerful and fast enough to shape the spatiotemporal profile of the ER Ca2+ oscillations. The Ca2+ stays in the cell and the SERCA increases its activity depending on the cytosolic Ca2+ concentration. Thus, Ca2+-dependency of the SERCA2 (Satoh et al., 2011) might be sufficient to explain the oscillatory behavior of ER-Ca2+ influx and efflux (Figure 2B). The weakness of this model is that Sec61a, the Ca2+ leak channel that would best fit to the concept, is a highly regulated protein (Daverkausen-Fischer and Pröls, 2022). Still, the sum of all ER leak mechanisms could fulfill the fundamental property of a passive ER Ca2+ leak pore through which Ca2+ flow is rather fast, for instance as seen after SERCA inhibition. How the ER Ca2+ leak mechanisms are regulated, how it’s activity is reduced, enhanced, activated or blocked, remains to be understood.
Mitochondria are also handling Ca2+, show mitochondrial Ca2+ (mCa2+) oscillations and are involved in the oscillation cycle, and might account for the temporal shift between ER and cytosolic calcium signals (Ishii et al., 2006; Lim et al., 2021). Much focus was put on the function of the mitochondrial Ca2+ uniporter complex (MCU complex) (Stefani et al., 2011). However, there is also MCU-independent Ca2+ uptake to mitochondria (Garbincius and Elrod, 2022). For instance, in MCU knockout cells, agonist-induced increase in mCa2+ is strongly reduced, or even abolished (Young et al., 2022; Álvarez-Illera et al., 2020). However, mCa2+ oscillations can be MCU-independent, at least in C. elegans (Álvarez-Illera et al., 2020). How mitochondria handle Ca2+ during Ca2+ oscillations, in response to stimuli or in cases of mCa2+-overload or underload, might be analyzed with triple color imaging experiments. For such experiments, simultaneous Ca2+ imaging, e.g. ER Ca2+ in green, cytosolic Ca2+ in red and mitochondrial Ca2+ in far-red, need to be validated.
The ER Ca2+-leak shapes agonist-induced Ca2+ fluxes
One widely studied Ca2+ signal is IP3-induced Ca2+ release (IP3-iCR). In astrocytes, IP3-iCR can be activated by adenosine via the highly expressed metabotropic receptor Adora1a (Figure 3A, minimal model). Fast adenosine stimuli activate fast ER Ca2+ release and subsequent Ca2+ oscillations (Figure 3A, lower panel) (Schulte et al., 2022). ATP, in contrast to adenosine, does not only evoke IP3-iCR through metabotropic P2Y receptors, but also opens ionotropic P2X receptors (Figure 3B, minimal model). When we stimulated astrocytes with ATP, a long-lasting cytosolic Ca2+ signal was induced (Figure 3B, lower panel). This Ca2+ entry shoulder did not contribute to ER refilling, but was likely preventing it (Schulte et al., 2022).
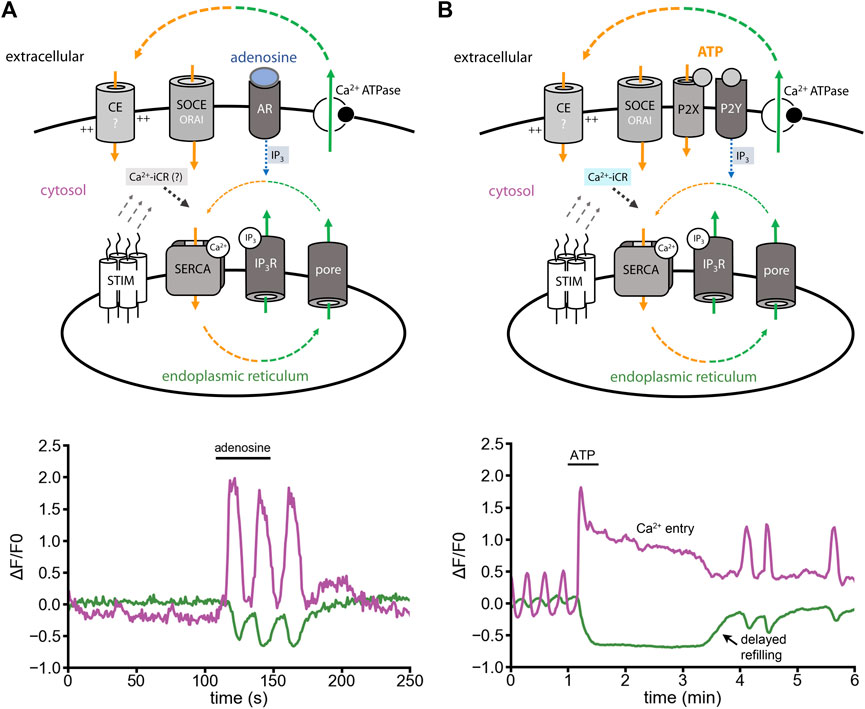
FIGURE 3. Adenosine- versus ATP-induced Ca2+ release in cultured astrocytes. Experiments were performed in presence of extracellular Ca2+. (A) Minimal model: Adenosine evokes IP3-iCR (IP3, blue). Ca2+ oscillations are induced by IP3-iCR. Ca2+ release and Ca2+ entry are in balance. Theoretically, Ca2+-iCR should contribute to the Ca2+ fluxes (indicated in grey). Lower panel: Oscillatory Ca2+ cycle induced by adenosine (10 µM) with cytosolic Ca2+ signals (magenta) and ER Ca2+ signals (green). (B) Minimal model: ATP binds to P2X and P2Y receptors. Metabotropic P2Y receptors evoke IP3-iCR (blue). P2X receptors might promote depolarizing Ca2+ influx, activation of voltage-gated Ca2+ channels and ER Ca2+ release through Ca2+-iCR (cyan). Lower panel: In response to ATP, the cytosolic Ca2+ increases while the ER Ca2+ decreases. Ca2+ entry is induced and creates a signal shoulder in the cytosol. The ER is not refilled during the Ca2+ entry shoulder. [Modified according to (Schulte et al., 2022)].
Why is the ATP-induced signal so different from exclusively metabotropic signals? The best explanation is that ATP activates a mixture of IP3-iCR and Ca2+-iCR (Figure 3B). Here, Ca2+-iCR would delay the refilling of the ER even though Ca2+ entry is ongoing (Figure 3B). In context of above-mentioned data (Figure 2), we think that a pronounced ER Ca2+ leak, and not only IP3-receptors, counteract ER refilling.
The ER Ca2+ leak, a fundamental driver of homeostatic Ca2+ fluxes
Over the last years, our view on the ER Ca2+ leak has drastically changed. The process can no longer be seen as an ‘unavoidable’ side effect of protein translation or as a slow, passive intracellular Ca2+ flux. Data from neurons and astrocytes clearly show that resting ER Ca2+ leak is upstream of resting Ca2+ entry, and thereby indirectly responsible for resting Ca2+ levels in the cytosol and ER. The ER Ca2+ leak fundamentally shapes cellular Ca2+ signals and ER Ca2+ oscillations.
One of the most exciting questions for future research is how ER leak channels contribute to microdomain signaling [Ca2+-tunnelling (Petersen et al., 2017)], local Ca2+ sparks (Cheng and Lederer, 2008), and electrogenic effects for local cellular excitability (Burdakov et al., 2005). Genetically engineered voltage dyes, targeted to the inner-side of the ER-membrane, might help to address electrogenic effects of ER leak channels.
For clinical research, it will be important to know how the ‘Ca2+ overload’ phenomenon arises and contributes to mitochondrial dysfunction and cell damage (Mekahli et al., 2011; Garbincius and Elrod, 2022). It can well be that Ca2+ overload in the cytosol and in mitochondria is triggered by an increased ER Ca2+ leak (ER Ca2+ ‘underload’) that continuously promotes resting homeostatic Ca2+ influx.
Author contributions
AS and RB conceptualized, wrote, and revised the manuscript. Both authors contributed equally to the article and approved the submitted version.
Funding
The study was funded by the Deutsche Forschungsgemeinschaft (DFG) BL567/3–2, ID Project: 194101929 (RB) and the Evangelisches Studienwerk Villigst (AS).
Acknowledgments
We thank Rohini Gupta for her input.
Conflict of interest
The authors declare that the research was conducted in the absence of any commercial or financial relationships that could be construed as a potential conflict of interest.
Publisher’s note
All claims expressed in this article are solely those of the authors and do not necessarily represent those of their affiliated organizations, or those of the publisher, the editors and the reviewers. Any product that may be evaluated in this article, or claim that may be made by its manufacturer, is not guaranteed or endorsed by the publisher.
References
Alonso M. T., Rojo-Ruiz J., Navas-Navarro P., Rodriguez-Prados M., Garcia-Sancho J. (2017). Measuring Ca(2+) inside intracellular organelles with luminescent and fluorescent aequorin-based sensors. Biochim. Biophys. Acta. Mol. Cell Res. 1864 (6), 894–899. doi:10.1016/j.bbamcr.2016.12.003
Álvarez-Illera P., García-Casas P., Fonteriz R. I., Montero M., Alvarez J. (2020). Mitochondrial Ca(2+) dynamics in MCU knockout C. elegans worms. Int. J. Mol. Sci. 21 (22), E8622. doi:10.3390/ijms21228622
Berridge M. J., Bootman M. D., Roderick H. L. (2003). Calcium signalling: Dynamics, homeostasis and remodelling. Nat. Rev. Mol. Cell Biol. 4 (7), 517–529. doi:10.1038/nrm1155
Birkner A., Konnerth A. (2019). Deep two-photon imaging in vivo with a red-shifted calcium indicator. Methods Mol. Biol. 1929, 15–26. doi:10.1007/978-1-4939-9030-6_2
Bootman M. D., Bultynck G. (2020). Fundamentals of cellular calcium signaling: A primer. Cold Spring Harb. Perspect. Biol. 12 (1), a038802. doi:10.1101/cshperspect.a038802
Burdakov D., Petersen O. H., Verkhratsky A. (2005). Intraluminal calcium as a primary regulator of endoplasmic reticulum function. Cell calcium 38 (3-4), 303–310. doi:10.1016/j.ceca.2005.06.010
Camello C., Lomax R., Petersen O. H., Tepikin A. V. (2002). Calcium leak from intracellular stores--the enigma of calcium signalling. Cell calcium 32 (5-6), 355–361. doi:10.1016/s0143416002001926
Chen M., Van Hook M. J., Thoreson W. B. (2015). Ca2+ diffusion through endoplasmic reticulum supports elevated intraterminal Ca2+ levels needed to sustain synaptic release from rods in darkness. J. Neurosci. 35 (32), 11364–11373. doi:10.1523/JNEUROSCI.0754-15.2015
Cheng H., Lederer W. J. (2008). Calcium sparks. Physiol. Rev. 88 (4), 1491–1545. doi:10.1152/physrev.00030.2007
Daverkausen-Fischer L., Pröls F. (2022). Regulation of calcium homeostasis and flux between the endoplasmic reticulum and the cytosol. J. Biol. Chem. 298, 102061. doi:10.1016/j.jbc.2022.102061
de Juan-Sanz J., Holt G. T., Schreiter E. R., de Juan F., Kim D. S., Ryan T. A. (2017). Axonal endoplasmic reticulum Ca2+ content controls release probability in CNS nerve terminals. Neuron 93 (4), 867–881. doi:10.1016/j.neuron.2017.01.010
Dupont G., Combettes L., Bird G. S., Putney J. W. (2011). Calcium oscillations. Cold Spring Harb. Perspect. Biol. 3 (3), a004226. doi:10.1101/cshperspect.a004226
Flourakis M., Van Coppenolle F., Lehen'kyi V., Beck B., Skryma R., Prevarskaya N. (2006). Passive calcium leak via translocon is a first step for iPLA2-pathway regulated store operated channels activation. FASEB J. official Publ. Fed. Am. Soc. Exp. Biol. 20 (8), 1215–1217. doi:10.1096/fj.05-5254fje
Garbincius J. F., Elrod J. W. (2022). Mitochondrial calcium exchange in physiology and disease. Physiol. Rev. 102 (2), 893–992. doi:10.1152/physrev.00041.2020
Hartmann J., Karl R. M., Alexander R. P., Adelsberger H., Brill M. S., Ruhlmann C., et al. (2014). STIM1 controls neuronal Ca²⁺ signaling, mGluR1-dependent synaptic transmission, and cerebellar motor behavior. Neuron 82 (3), 635–644. doi:10.1016/j.neuron.2014.03.027
Hasel P., Dando O., Jiwaji Z., Baxter P., Todd A. C., Heron S., et al. (2017). Neurons and neuronal activity control gene expression in astrocytes to regulate their development and metabolism. Nat. Commun. 8, 15132. doi:10.1038/ncomms15132
Hofer A. M., Machen T. E. (1993). Technique for in situ measurement of calcium in intracellular inositol 1, 4, 5-trisphosphate-sensitive stores using the fluorescent indicator mag-fura-2. Proc. Natl. Acad. Sci. U. S. A. 90 (7), 2598–2602. doi:10.1073/pnas.90.7.2598
Kipanyula M. J., Contreras L., Zampese E., Lazzari C., Wong A. K., Pizzo P., et al. (2012). Ca2+ dysregulation in neurons from transgenic mice expressing mutant presenilin 2. Aging Cell 11 (5), 885–893. doi:10.1111/j.1474-9726.2012.00858.x
Ishii Kiyoaki, Hirose Kenzo, Iino Masamitsu (2006). Ca2+ shuttling between endoplasmic reticulum and mitochondria underlying Ca2+ oscillations. EMBO Rep. 7 (4), 390–396. doi:10.1038/sj.embor.7400620
Lalonde J., Saia G., Gill G. (2014). Store-operated calcium entry promotes the degradation of the transcription factor Sp4 in resting neurons. Sci. Signal. 7 (328), ra51. doi:10.1126/scisignal.2005242
Lang S., Pfeffer S., Lee P. H., Cavalie A., Helms V., Forster F., et al. (2017). An update on Sec61 channel functions, mechanisms, and related diseases. Front. Physiol. 8, 887. doi:10.3389/fphys.2017.00887
Laude A. J., Tovey S. C., Dedos S. G., Potter B. V., Lummis S. C., Taylor C. W. (2005). Rapid functional assays of recombinant IP3 receptors. Cell calcium 38 (1), 45–51. doi:10.1016/j.ceca.2005.04.001
Lemos F. O., Bultynck G., Parys J. B. (2021). A comprehensive overview of the complex world of the endo- and sarcoplasmic reticulum Ca(2+)-leak channels. Biochim. Biophys. Acta. Mol. Cell Res. 1868 (7), 119020. doi:10.1016/j.bbamcr.2021.119020
Lim D., Semyanov A., Genazzani A., Verkhratsky A. (2021). Calcium signaling in neuroglia. Int. Rev. Cell Mol. Biol. 362, 1–53. doi:10.1016/bs.ircmb.2021.01.003
Mekahli D., Bultynck G., Parys J. B., De Smedt H., Missiaen L. (2011). Endoplasmic-reticulum calcium depletion and disease. Cold Spring Harb. Perspect. Biol. 3 (6), a004317. doi:10.1101/cshperspect.a004317
Petersen O. H., Courjaret R., Machaca K. (2017). Ca(2+) tunnelling through the ER lumen as a mechanism for delivering Ca(2+) entering via store-operated Ca(2+) channels to specific target sites. J. Physiol. 595 (10), 2999–3014. doi:10.1113/JP272772
Prada J., Sasi M., Martin C., Jablonka S., Dandekar T., Blum R. (2018). An open source tool for automatic spatiotemporal assessment of calcium transients and local 'signal-close-to-noise' activity in calcium imaging data. PLoS Comput. Biol. 14 (3), e1006054. doi:10.1371/journal.pcbi.1006054
Putney J. W., Steinckwich-Besancon N., Numaga-Tomita T., Davis F. M., Desai P. N., D'Agostin D. M., et al. (2017). The functions of store-operated calcium channels. Biochim. Biophys. Acta. Mol. Cell Res. 1864 (6), 900–906. doi:10.1016/j.bbamcr.2016.11.028
Rehberg M., Lepier A., Solchenberger B., Osten P., Blum R. (2008). A new non-disruptive strategy to target calcium indicator dyes to the endoplasmic reticulum. Cell calcium 44 (4), 386–399. doi:10.1016/j.ceca.2008.02.002
Rodriguez-Garcia A., Rojo-Ruiz J., Navas-Navarro P., Aulestia F. J., Gallego-Sandin S., Garcia-Sancho J., et al. (2014). GAP, an aequorin-based fluorescent indicator for imaging Ca2+ in organelles. Proc. Natl. Acad. Sci. U. S. A. 111 (7), 2584–2589. doi:10.1073/pnas.1316539111
Rodríguez-Prados M., Rojo-Ruiz J., García-Sancho J., Alonso M. T. (2020). Direct monitoring of ER Ca2+ dynamics reveals that Ca2+ entry induces ER-Ca2+ release in astrocytes. Pflugers Arch. 472 (4), 439–448. doi:10.1007/s00424-020-02364-7
Rodriguez-Prados M., Rojo-Ruiz J., Garcia-Sancho J., Alonso M. T. (2020). Direct monitoring of ER Ca(2+) dynamics reveals that Ca(2+) entry induces ER-Ca(2+) release in astrocytes. Pflugers Arch. 472 (4), 439–448. doi:10.1007/s00424-020-02364-7
Rossi A. M., Taylor C. W. (2020). Reliable measurement of free Ca(2+) concentrations in the ER lumen using Mag-Fluo-4. Cell calcium 87, 102188. doi:10.1016/j.ceca.2020.102188
Stefani Diego De, Raffaello Anna, Teardo Enrico, Szabò Ildikò, Rizzuto Rosario (2011). A forty-kilodalton protein of the inner membrane is the mitochondrial calcium uniporter. Nature 476 (7360), 336–340. doi:10.1038/nature10230
Samtleben S., Jaepel J., Fecher C., Andreska T., Rehberg M., Blum R. (2013). Direct imaging of ER calcium with targeted-esterase induced dye loading (TED). J. Vis. Exp. 75 (75), e50317. doi:10.3791/50317
Samtleben S., Wachter B., Blum R. (2015). Store-operated calcium entry compensates fast ER calcium loss in resting hippocampal neurons. Cell calcium 58 (2), 147–159. doi:10.1016/j.ceca.2015.04.002
Satoh K., Matsu-ura T., Enomoto M., Nakamura H., Michikawa T., Mikoshiba K. (2011). Highly cooperative dependence of sarco/endoplasmic reticulum calcium ATPase (SERCA) 2a pump activity on cytosolic calcium in living cells. J. Biol. Chem. 286 (23), 20591–20599. doi:10.1074/jbc.M110.204685
Schäuble N., Lang S., Jung M., Cappel S., Schorr S., Ulucan O., et al. (2012). BiP-mediated closing of the Sec61 channel limits Ca2+ leakage from the ER. EMBO J. 31 (15), 3282–3296. doi:10.1038/emboj.2012.189
Schulte A., Bieniussa L., Gupta R., Samtleben S., Bischler T., Doering K., et al. (2022). Homeostatic calcium fluxes, ER calcium release, SOCE, and calcium oscillations in cultured astrocytes are interlinked by a small calcium toolkit. Cell calcium 101, 102515. doi:10.1016/j.ceca.2021.102515
Solovyova N., Verkhratsky A. (2002). Monitoring of free calcium in the neuronal endoplasmic reticulum: An overview of modern approaches. J. Neurosci. Methods 122 (1), 1–12. doi:10.1016/s0165-0270(02)00300-x
Solovyova N., Veselovsky N., Toescu E. C., Verkhratsky A. (2002). Ca(2+) dynamics in the lumen of the endoplasmic reticulum in sensory neurons: Direct visualization of Ca(2+)-induced Ca(2+) release triggered by physiological Ca(2+) entry. EMBO J. 21 (4), 622–630. doi:10.1093/emboj/21.4.622
Streb H., Irvine R. F., Berridge M. J., Schulz I. (1983). Release of Ca2+ from a nonmitochondrial intracellular store in pancreatic acinar cells by inositol-1, 4, 5-trisphosphate. Nature 306 (5938), 67–69. doi:10.1038/306067a0
Suzuki J., Kanemaru K., Ishii K., Ohkura M., Okubo Y., Iino M. (2014). Imaging intraorganellar Ca2+ at subcellular resolution using CEPIA. Nat. Commun. 5, 4153. doi:10.1038/ncomms5153
Tang S., Wong H-C., Wang Z-M., Huang Y., Zou J., Zhuo Y., et al. (2011). Design and application of a class of sensors to monitor Ca2+ dynamics in high Ca2+ concentration cellular compartments. Proc. Natl. Acad. Sci. U. S. A. 108 (39), 16265–16270. doi:10.1073/pnas.1103015108
Thastrup O., Dawson A. P., Scharff O., Foder B., Cullen P. J., Drobak B. K., et al. (1989). Thapsigargin, a novel molecular probe for studying intracellular calcium release and storage. Agents Actions 27 (1-2), 17–23. doi:10.1007/BF02222186
Verkhratsky A., Nedergaard M. (2018). Physiology of astroglia. Physiol. Rev. 98 (1), 239–389. doi:10.1152/physrev.00042.2016
Verkhratsky A. (2005). Physiology and pathophysiology of the calcium store in the endoplasmic reticulum of neurons. Physiol. Rev. 85 (1), 201–279. doi:10.1152/physrev.00004.2004
Young Michael, Schug Zachary T., Booth David, Yule David, Mikoshiba Katsuhiko, Hajnoczky Gyorgy, et al. (2022). Metabolic adaptation to the chronic loss of Ca(2+) signaling induced by KO of IP3 receptors or the mitochondrial Ca(2+) uniporter. J. Biol. Chem. 298 (1), 101436. doi:10.1016/j.jbc.2021.101436
Keywords: Ca2+ homeostasis, Ca2+ ion analysis, ER Ca2+ store, ER Ca2+ imaging, store-operated Ca2+ entry, Ca2+ leak, SERCA, Ca2+ oscillation
Citation: Schulte A and Blum R (2022) Shaped by leaky ER: Homeostatic Ca2+ fluxes. Front. Physiol. 13:972104. doi: 10.3389/fphys.2022.972104
Received: 17 June 2022; Accepted: 16 August 2022;
Published: 07 September 2022.
Edited by:
Richard Zimmermann, Saarland University, GermanyReviewed by:
Agustín Guerrero-Hernández, Centro de Investigaciones y Estudios Avanzados (CINVESTAV-IPN), MexicoJan B. Parys, KU Leuven, Belgium
Copyright © 2022 Schulte and Blum. This is an open-access article distributed under the terms of the Creative Commons Attribution License (CC BY). The use, distribution or reproduction in other forums is permitted, provided the original author(s) and the copyright owner(s) are credited and that the original publication in this journal is cited, in accordance with accepted academic practice. No use, distribution or reproduction is permitted which does not comply with these terms.
*Correspondence: Robert Blum, Ymx1bV9yQHVrdy5kZQ==