- CNR Istituto per la Scienza e Tecnologia dei Plasmi, Bari, Italy
Nitrogen fixation is crucial for plant growth and global agriculture, especially with the projected population growth requiring a significant increase in food production. Traditional nitrogen fixation relies on the Haber-Bosch (H-B) process, which is energy-intensive and environmentally harmful due to greenhouse gas emissions. Emerging technologies, such as cold plasma, offer promising alternatives with lower energy consumption. Cold plasma facilitates reactive nitrogen species generation under ambient conditions, potentially improving the production efficiency of nitrogen oxides (NOx). However, optimizing cold plasma nitrogen fixation requires a synergy between experimental and theoretical approaches. Accurate input data are essential for refining theoretical models, which can then guide the design of more efficient processes. This integrated approach can leverage renewable energy, operate on smaller scales, and minimize environmental impacts, making cold plasma a sustainable solution for future nitrogen fixation needs.
1 Introduction
Nitrogen (N) is essential for plant development, as it is needed for synthesizing proteins and nucleic acids. Nitrogen fixation is a crucial process for providing these compounds. This is a big issue considering an expected population of nine billion people by 2050 that would require an expansion of global agricultural output by 70%–100% [1].
The established synthesis technology for N fertilizers relies strongly on the Haber-Bosch (H-B) process, to convert N2 gas into biologically available ammonia (NH3). The current industrial production of reactive N (RNS) is 120 teragrams per year (Tg yr−1), twice the amount from all-natural land processes (63 Tg yr−1 [2]). Fertilizer production consumes about 80% of this RNS [3], and is essential for sustaining half of the global human population [4]. However, the efficiency of RNS utilization is poor, with 50%–70% lost to the environment [5, 6]. The accumulation of excess RNS, such as NH3, nitrous oxide (N2O), and nitrate (NO3−), in the atmosphere and ecosystems leads to serious environmental and climate issues [2, 3, 7, 8]. These issues encompass alterations in ecosystem productivity and biodiversity [9, 10], the eutrophication and nitrate pollution of freshwater [10, 11], the deterioration of ozone and air quality [11, 12], and the exacerbation of climate change due to greenhouse gases [3, 7].
At the beginning of the 20th century, the fertilizers produced by H-B substituted the non-renewable mineral ones. Nowadays, the H–B process drains more than 1% of the globally produced energy, releasing more than 3 × 1011 kg year−1 of CO2, and requiring about 2% of the global natural gas production, to synthesize the needed hydrogen for NH3 synthesis [13–15]. Therefore, while H-B processes should be made more sustainable [16], greener strategies in nitrogen fixation should also be pursued. In Figure 1 the energy consumption in terms of MJ consumed per N mole of various processes is represented together with the calculated theoretical limits. Among the explored processes for nitrogen fixation, the most efficient is still the H-B, surpassed only by biological nitrogen fixation. Other industrial-scale processes adopted in the past like the thermal plasma-based Birkeland-Eyde, or the Frank-Caro process leading to calcium cyanamide are far more energy-consuming. Metallo-complexes fixation currently has poor efficiency and a theoretical limit far from the H-B.
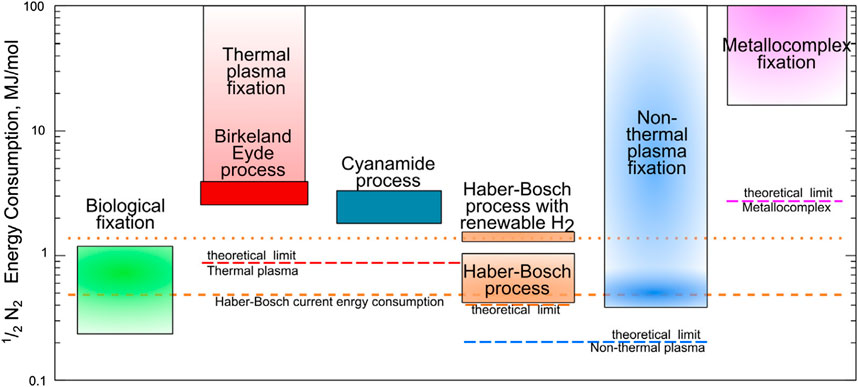
Figure 1. Comparison of the energy consumption for nitrogen fixation with different methods (Reprinted from [15] with permission of Elsevier B.V.).
Since the 1970s, plasma technologies have been pointed to as a possible alternative for large-scale NH3 production, in the presence of a catalyst or not. Different plasma sources have been proposed for ammonia synthesis, such as glow discharge, gliding arc, microwave, radio frequency, pulsed discharges, and dielectric barrier discharges. The current reported energy yield for the plasma and catalytic materials process is ranging from 1.71–58.8 MJ/mol N [17–33]. Best performances are achieved by pulsed discharge systems and dielectric barrier discharge reactors at atmospheric pressure that facilitates the integration of catalytic materials.
Novel approaches based on Cold Plasma are currently being extensively investigated and promise versatile applications. These innovative methods entail subjecting agricultural systems to non-thermal plasma, generating reactive species such as reactive oxygen species (ROS) and reactive nitrogen species (RNS) [34, 35]. One crucial allure of these technologies is the achievement of lower energy consumption (EC) than the H-B process, according to theoretical calculations [36]. It is our opinion, that the main advantage of cold plasma technology is related to their intrinsic non-thermal nature and the energy transfer processes involved in reactive species productions. We will try to give our opinion on the elementary processes leading to nitrogen oxide production and their potential impact on the agrifood industry of this technology reporting briefly on the current status of the ongoing research.
2 Plasma nitrogen fixation in dry air
Direct, efficient production of NOx from air, at variance with current indirect production by NH3 synthesis, using H-B process, would open plenty of possibilities in recycling. NOx allows the direct synthesis of nitric acid (HNO3) [37], which can be used for chemical fertilizers production, but also the fast maturation of natural fertilizers from livestock slurry, an environment-friendly process, which is currently being developed [38].
2.1 Plasma production of NOx from air: the mechanism
Plasma production of NOx from air, such as in lightning, by a thermal plasma was industrially realized by the Birkeland-Eyde process. The NOx production efficiency of a few percent limits its application to a handful of special uses thus making the process not competitive with current industrial processes.
An energy analysis [15, 36] on the use of cold plasma can however envisage a completely different development, with a theoretical maximum limit of 35% efficiency [36], exceeding the current total efficiency of industrial NOx production [37].
Cold plasma behavior is complex and influenced by numerous parameters and conditions. For instance, electron temperatures might reach 10000 K while gas remains at 400 K and molecular vibrational temperature at 3000 K [39]. To properly study the kinetics of a cold plasma, therefore we need to know vibrational state-to-state processes involving, at least, electron-molecule (e-M) and molecule-molecule collisions. e-M processes transfer electron energy from the discharge to the internal energy, mostly vibrational, of air molecular species. Then, by molecular collisions and vibration-to-vibration (VV) exchange processes, as in N2 (v1)+N2 (v2)→N2 (v1-1)+N2 (v2+1), a significant fraction of molecules increases its vibrational energy [36, 40], provided low-lying levels are continuously replenished by e-M processes. When vibrational energy reaches oxygen dissociation energy (≈5eV), O atoms can initiate the Zel’dovich chain (Equations 1, 2), while N2 remains mostly undissociated.
This is favorable for energy efficiency because it is more efficient to make N2 react rather than obtaining N atoms from dissociation. For the same reasons processes involving excited electronic states of N2 and O2 should be avoided. The ideal Zel’dovich reaction path of Equations 1, 2 is thought to be the most efficient one [36, 40]. However, many other concurrent collision processes take place, such as, for example,: vibration-to-translation (VT) deactivation processes (as in N + O2(v)→N + O2 (v-1)); the reverse of the Zel’dovich (Equation 1) reaction; plasma–walls vibrational deactivation collision; NO dissociation/reaction by collisions with other air species.
2.2 Cold plasma production of NOx from air: coupling modeling with experiment issue
The non-equilibrium nature of cold plasmas requires a guiding model to design experiments to investigate the possible reaction pathways occurring in the discharge systems. Therefore, chemical kinetics modelling is crucial in this context, provided the data used as input for this modelling is accurate and detailed. Up to now, no one has been able to replicate the predictions reported in Ref. [36] (except for [41]) by detailed modelling. Indeed, the main problem for modelling is the extremely approximate data taken as input. For example, the first Zel’dovich reaction Equation 1, i.e., the core of the NO production mechanism from air, has been known for many years only by empirical models. The model of Gordiets [42] considered the possibility of reaction once the reaction threshold has been reached, which occurs, at room temperature, when N2 is at least in v = 12 state. More recent studies [43–46] contemplating the adiabatic path to reaction on the triplet potential energy surfaces (PES), showed a much more complex situation. In [45] a complete compilation of vibrationally detailed data (i.e., considering the whole vibrational ladder of reagents and products) concerning the adiabatic path has been calculated, with an excellent agreement with the experimental thermal rate. The differences in results obtained from the simple models adopted in the past and still used in the literature are huge (orders of magnitude, see [45, 47]), particularly in the energy region between vibrational levels v = 12–30 where most of the reaction occurs. Another aspect of great impact is the determination of the final NO vibration in reaction (Equation 1). In [48] a comparison of a kinetic scheme in air is presented using the same set of rate coefficients calculated in [45, 46, 49, 50], but considering state-to-state or only initially state-selected rate coefficients in (1). The results of N and NO production are qualitatively different, stressing the importance of accurate and detailed molecular dynamics calculations as input of the kinetics.
With a higher probability than reaction (Equation 1), the VT deactivation:
can take place starting from the same collision. This is a big issue for nitrogen fixation because it depletes the N2 vibrational population essential for good efficiency in Equation 1. In fact, in cold plasmas, the strongly endothermic reaction (Equation 1) should be preferentially activated by vibrational pumping of molecular species involved rather than by heating, in order to keep the rate of VT processes like (Equation 3) low. However, the accurate rate value of the process (Equation 3) for v > 1 has remained unknown for decades, with only the v = 1 to v' = 0 rate coefficient known experimentally. The only known result, however, was also dramatically different (even 20 orders of magnitude at 300 K) from theoretical results obtained by quasi-classical (QCT), semiclassical (SC), and quantum methods [45, 51, 52]. Only in very recent years the problem has been solved [52, 53], by recognizing the fundamental role of vibronic transitions of the collisional system and calculating other transitions from v ≠ 1. Indeed, process (Equation 3) shows even other issues when studied theoretically. The methods used for studying molecular collisions of heavy particles can be QCT, SC, or quantum methods on a scale of increasing accuracy and computational cost. In studies on air species aiming to create vibrationally detailed databases, using accurate quantum methods is simply unfeasible. While these methods are essential for establishing benchmarks (as demonstrated in [44]), they are unsuitable for the comprehensive calculations necessary for kinetic modeling. Semiclassical and, first, QCT must be used instead, requiring huge computational resources. However, some processes are difficult to treat even with these simpler methods, due to their specific limitations. In particular, the process in Equation 3 cannot be reproduced theoretically by QCT at low total energy [54]. However, at collision energy values comparable to or higher than the reaction (Equation 1) threshold, QCT becomes suitable for accurate results for the inelastic process (Equation 3). Deactivation processes are surely present in all collision processes of interest in air plasmas. Most of the VT data used in current kinetic models of air plasmas have been calculated by methods based on a forced harmonic oscillator (FHO) semiclassical model [55] or by QCT [56, 57]. However, FHO is designed to treat non-reactive cases at not very high total energy, while QCT tends to be more accurate at higher energy, especially over the threshold for reactions originating from the collisions considered [46]. Merging of different semi- and quasi-classical methods for cold plasma modeling input data calculation is therefore desirable. This merging of methods could be successfully applied potentially to all collisional systems involving air species of interest in this context, such as N2-O2, from which O atoms production depends, and research is active in this sense [39, 46, 54].
Another important collisional system is of course the second Zel’dovich reaction. Even in this case, recent results in [49] are quite complete, accurate, and more suited for nitrogen fixation studies than older studies on this topic [58].
2.3 Current experimental approaches for nitrogen fixation
In most air plasmas, the Zel’dovich reactions (Equations 1, 2) leading to NO productions can further proceed towards NO2, resulting in a gas-phase mixture of nitrogen oxides referred to as NOx. These reactions involve ozone or oxygen radicals oxidizing NO (Equations 4–6):
Following earlier studies on thermal plasma (i.e., electric arc exploiting the Birkeland-Eyde reaction), other plasma types and reactors have been examined for NOx production. This has led to an overabundance of possible sources, making any comparison between different methodologies quite complicated, as no standard reactors or even measurements techniques for different parameters are widely accepted. Energy consumption, for example, is dependent on power and NOx concentration. NOx concentrations can be determined via a plethora of methods: Fourier-transform infrared spectroscopy, mass spectroscopy, chemiluminescence, ion chromatography, as well as a nondispersive infra-red sensor with an ultra-violet sensor through a gas analyzer, in situ laser induced fluorescence, laser Raman spectroscopy, optical absorption. Power can be calculated by the Lissajous method [59, 60], numerically integrating the product of the voltage and current and multiplying by the frequency, or by calorimetric methods by multiplying air density, gas temperature, heat capacity of the air, and volume [61]. Moreover, for a real industrial application, the total power should include all the energy consumption in the production pipeline, such as gas flow system and storage, power sources absorption, etc., that are not always considered.
A great number of plasma sources have been investigated in the context of nitrogen fixation into NOx. Gliding arc (GA) reactors are promising for gas conversion, achieving efficient NOx production with reduced energy. GA plasmas feature a reduced electric field of less than 100 Td and electron energies around 1 eV, ideal for the vibrational excitation of gas molecules. Reactor optimizations, including controlling pressure, specific energy input, and reactor designs, enhance NOx yield and energy efficiency across various setups (EC 0.67–4.8 MJ/mol N) [62–69].
NOx generation in transient spark discharge, which involves non-thermal and thermal plasma phases, has also been achieved. Although a limited volume, which means that only a portion of the N2 gas is exposed to the plasma, innovations like floating electrodes could improve NOx yield and efficiency, reducing energy needs (EC 1.9–40 MJ/mol N) [62, 70–74].
Dielectric Barrier Discharge (DBD) systems could be of great interest for the great versatility they offer in reactor design and for the possibility to operate them at atmospheric pressure. For example, other than simple cylindrical, plug-flow-like reactors, it is possible to incorporate water (for nitrogen fixation into NO3) or other materials in the interelectronic gap. Packed-bed DBD reactors with various catalysts improve NOx yield, resulting in higher energy costs. Promising catalytic materials include γ-Al2O3 and Al2O3-supported metallic nanoparticles (EC 17–33 MJ/mol N) [62, 75–77].
Low-pressure microwave plasmas achieved the best energy consumption and NO yield. However, past claims (EC = 0.28 MJ/mol N [41]) are unconfirmed. Energy use excludes reactor cooling and vacuum needs, that eventually add up and make total consumption higher (EC 0.84–3.76 MJ/mol N) [78, 79].
NOx formation by plasma jets in air or N2 reacting with water results in NO2 and NO3 due to oxygen presence. Key factors affecting NOx production include gas composition, flow rate, and temperature. Studies show reducing the flow rate and increasing oxygen content boosts NOx concentration, while lower gas temperature and electric field enhance production efficiency (EC 0.42–3.6 MJ/mol N) [80–89].
From the existing literature, it can be concluded that efficient NOx production in plasma systems relies on optimizing reduced electric fields to favor vibrational excitation (enhancing Zel’dovich reactions), controlling gas temperature to prevent NO reconversion, and utilizing appropriate catalysts. Key parameters include voltage, electric field strength, gas composition and flow rates, and reactor design.
All the reported results show that the current technology is still far from theoretical limits, but is closing the gap between H-B processes.
3 Future perspective
In countries where agriculture is significant, environmentally sustainable HNO₃ production could serve as an important power-to-chemicals channel, accumulating excess renewable power into valuable chemicals using cold plasma reactors operating at room temperature and atmospheric pressure, thus requiring lower investments than an H-B plant [37]: this would be vital to boosting agriculture in low-income countries. Moreover, a diffused grid energy/fertilizer production usage would be more resilient to natural disasters and armed conflicts than centralized power production and fertilizer import, avoiding direct and indirect related import, transportation, and distribution costs. In our opinion the most viable possible solutions are currently two: production of hybrid fertilizer from slurry and plasma-activated water.
3.1 Hybrid fertilizers
Efficient air plasma production of NOx offers significant advantages for the fertilizer industry, allowing direct synthesis of nitric acid (HNO3) [37], for chemical and hybrid fertilizers. Acidification of livestock slurry process has been used in agriculture [90] since 2003, but the novelty is the easy-to-manage direct fertilizer production by air plasma [38]. The latter process facilitates easy production of fertilizers from slurry and air, with benefits including recycling livestock wastewater, eliminating inorganic feedstock, preserving natural manure components, shorter organic fertilizer production times, avoiding stocking dangerous acids, reducing greenhouse gases (i.e., N2O and CH4), and preventing ammonia emissions. It supports precision agriculture [91], minimizes environmental losses, and can also be applied in principle to human wastewater. Preliminary experiments in the fields show that this “hybrid” (artificial/natural) manure can be extremely effective [92].
3.2 Plasma-activated water
Concurrently, alongside advancements in low-temperature plasma applications, plasma-activated water (PAW) has surfaced as a cutting-edge tool in modern agriculture, with the potential to enhance crop productivity and mitigate industry challenges [93–98]. PAW production involves subjecting water to non-thermal plasma, generating reactive species such as ROS and RNS. The physicochemical alterations induced by plasma treatment on water can be tailored for diverse applications [99]. PAW reactive species elicit beneficial effects on plants, inducing physiological and biochemical changes conducive to growth and stress tolerance [97, 98, 100]. PAW offers a sustainable and eco-friendly alternative to conventional chemical fertilizers and pesticides, applicable in watering crops, seed soaking, and foliage spraying.
4 Conclusion
Concerning future developments in nitrogen fixation, cold plasma seems to be the only promising and industrially scalable method because its use could further lower the EC of the process, based on thermodynamic calculations. The highest energy efficiency is expected when vibrationally excited nitrogen molecules are formed in sufficient quantities, the temperature of the resulting gases is not high enough to decompose the reaction products, and the reaction is channeled into a particular reaction pathway. Atmospheric pressure cold plasma nitrogen fixation has many environmental advantages as it requires only electricity, air, and, depending on the application, wastewater (hybrid fertilizers) or water (PAW) as feedstock. Contrary to large-scale high-pressure ammonia synthesis plants, plasma nitrogen fixation may be performed on a much smaller scale, locally producing the fertilizers and eliminating the problems associated with fertilizers’ transportation and storage. Moreover, such plants can be ideally powered by renewable energy sources, further reducing the environmental impact of fertilizer production.
However, detailed modeling tools are needed to maximize cold plasma nitrogen fixation efficiency. An essential step is establishing an efficient dialogue between practical and theoretical experiments: accurate data sets should be fed to models so that they could shed light on the mechanism pathways that should be preferred to optimize the reaction.
Data availability statement
The original contributions presented in the study are included in the article/supplementary material, further inquiries can be directed to the corresponding author.
Author contributions
DA: Data curation, Writing - review and editing, Writing - original draft, Validation, Conceptualization. PA: Funding acquisition, Data curation, Writing - review and editing, Writing - original draft, Validation, Supervision, Conceptualization. FE: Writing - review and editing, Writing - original draft, Validation, Supervision, Conceptualization.
Funding
The author(s) declare that financial support was received for the research, authorship, and/or publication of this article. European Cooperation in Science and Technology, CA19110; Regione Puglia, Riparti - POC PUGLIA FESRT-FSE 2014/2020.
Conflict of interest
The authors declare that the research was conducted in the absence of any commercial or financial relationships that could be construed as a potential conflict of interest.
The author(s) declared that they were an editorial board member of Frontiers, at the time of submission. This had no impact on the peer review process and the final decision.
Publisher’s note
All claims expressed in this article are solely those of the authors and do not necessarily represent those of their affiliated organizations, or those of the publisher, the editors and the reviewers. Any product that may be evaluated in this article, or claim that may be made by its manufacturer, is not guaranteed or endorsed by the publisher.
Abbreviations
DBD, Dielectric Barrier Discharge; EC, Energy Consumption; e-M, electron-Molecules collisions; FHO, forced harmonic oscillator; GA, Gliding Arc; H-B, Haber Bosch; MJ/mol N, MegaJoules consumed per mole of Nitrogen (fixed) produced; N, nitrogen atoms; NOx, Nitric Oxides; PAW, Plasma Activated Water; QCT, quasi-classical modeling methods; RNS, Reactive Nitrogen Species; ROS, Reactive Oxygen Species; SC, semiclassical modeling methods; Tg yr−1, Tera grams per year; VT, Vibration-to-Translation energy transfer; VV, Vibration-to-Vibration energy transfer.
References
1. Godfray HCJ, Beddington JR, Crute IR, Haddad L, Lawrence D, Muir AF, et al. Food security: the challenge of feeding 9 billion people. Science (1979) (2010) 327:812–818. doi:10.1126/science.1185383
2. Fowler D, Coyle M, Skiba U, Sutton MA, Cape JN, Reis S, et al. The global nitrogen cycle in the twenty-first century. Phil Trans R Soc B: Biol Sci (2013) 368:20130164. doi:10.1098/rstb.2013.0164
3. Galloway JN, Townsend AR, Erisman JW, Bekunda M, Cai Z, Freney JR, et al. Transformation of the nitrogen cycle: recent trends, questions, and potential solutions. Science (1979) 2008:889–92. doi:10.1126/science.1136674
4. Erisman JW, Sutton MA, Galloway J, Klimont Z, Winiwarter W. How a century of ammonia synthesis changed the world. Nat Geosci (2008) 1:636–9. doi:10.1038/ngeo325
5. Cassman KG, Dobermann A, Walters DT. Agroecosystems, nitrogen-use efficiency, and nitrogen management. Ambio (2002) 31:132–40. doi:10.1579/0044-7447-31.2.132
6. Ladha JK, Pathak H, Krupnik TJ, Six J, van Kessel C. Efficiency of fertilizer nitrogen in cereal production: retrospects and prospects. Adv Agron (2005) 87. doi:10.1016/S0065-2113(05)87003-8
7. Erisman JW, Galloway J, Seitzinger S, Bleeker A, Butterbach-Bahl K. Reactive nitrogen in the environment and its effect on climate change. Curr Opin Environ Sustain (2011) 3:281–90. doi:10.1016/j.cosust.2011.08.012
8. Schlesinger WH. On the fate of anthropogenic nitrogen. Proc Natl Acad Sci U S A (2009) 106:203–8. doi:10.1073/pnas.0810193105
9. Tilman D, Isbell F. Biodiversity: recovery as nitrogen declines. Nature (2015) 528. doi:10.1038/nature16320
10. Vitousek PM, Aber JD, Howarth RW, Likens GE, Matson PA, Schindler DW, et al. Human alteration of the global nitrogen cycle: sources and consequences. Ecol Appl (1997) 7:737–50. doi:10.1890/1051-0761(1997)007[0737:HAOTGN]2.0.CO;2
11. Townsend AR, Howarth RW, Bazzaz FA, Booth MS, Cleveland CC, Collinge SK, et al. Human health effects of a changing global nitrogen cycle. Front Ecol Environ (2003) 1:240–6. doi:10.1890/1540-9295(2003)001[0240:hheoac]2.0.co;2
12. Ravishankara AR, Daniel JS, Portmann RW. Nitrous oxide (N2O): the dominant ozone-depleting substance emitted in the 21st century. Science (1979) 2009:123–5. doi:10.1126/science.1176985
13. Van Der Ham CJM, Koper MTM, Hetterscheid DGH. Challenges in reduction of dinitrogen by proton and electron transfer. Chem Soc Rev (2014) 43:5183–91. doi:10.1039/c4cs00085d
14. Tanabe Y, Nishibayashi Y. Developing more sustainable processes for ammonia synthesis. Coord Chem Rev (2013) 257:2551–64. doi:10.1016/j.ccr.2013.02.010
15. Cherkasov N, Ibhadon AO, Fitzpatrick P. A review of the existing and alternative methods for greener nitrogen fixation. Chem Eng Process Process Intensification (2015) 90:24–33. doi:10.1016/j.cep.2015.02.004
16. Smith C, Hill AK, Torrente-Murciano L. Current and future role of Haber-Bosch ammonia in a carbon-free energy landscape. Energy Environ Sci (2020) 13:331–44. doi:10.1039/c9ee02873k
17. Shah J, Wu T, Lucero J, Carreon MA, Carreon ML. Nonthermal plasma synthesis of ammonia over Ni-MOF-74. ACS Sustain Chem Eng (2019) 7:377–83. doi:10.1021/acssuschemeng.8b03705
18. Shah JR, Harrison JM, Carreon ML. Ammonia plasma-catalytic synthesis using low melting point alloys. Catalysts (2018) 8:437. doi:10.3390/catal8100437
19. Hu J, Wildfire C, Stiegman AE, Dagle RA, Shekhawat D, Abdelsayed V, et al. Microwave-driven heterogeneous catalysis for activation of dinitrogen to ammonia under atmospheric pressure. Chem Eng J (2020) 397:125388. doi:10.1016/j.cej.2020.125388
20. Brandenburg R, Bogaerts A, Bongers W, Fridman A, Fridman G, Locke BR, et al. White paper on the future of plasma science in environment, for gas conversion and agriculture. Plasma Process Polym (2019) 16. doi:10.1002/ppap.201700238
21. Kim M, Biswas S, Nava G, Wong BM, Mangolini L. Reduced energy cost of ammonia synthesis via RF plasma pulsing. ACS Sustain Chem Eng (2022) 10:15135–47. doi:10.1021/acssuschemeng.2c04593
22. Peng P, Chen P, Addy M, Cheng Y, Anderson E, Zhou N, et al. Atmospheric plasma-assisted ammonia synthesis enhanced via synergistic catalytic absorption. ACS Sustain Chem Eng (2019) 7:100–4. doi:10.1021/acssuschemeng.8b03887
23. Hong J, Prawer S, Murphy AB. Plasma catalysis as an alternative route for ammonia production: status, mechanisms, and prospects for progress. ACS Sustain Chem Eng (2018) 6:15–31. doi:10.1021/acssuschemeng.7b02381
24. Iwamoto M, Akiyama M, Aihara K, Deguchi T. Ammonia synthesis on wool-like Au, Pt, Pd, Ag, or Cu electrode catalysts in nonthermal atmospheric-pressure plasma of N 2 and H 2. ACS Catal (2017) 7:6924–9. doi:10.1021/acscatal.7b01624
25. Peng P, Li Y, Cheng Y, Deng S, Chen P, Ruan R. Atmospheric pressure ammonia synthesis using non-thermal plasma assisted catalysis. Plasma Chem Plasma Process (2016) 36:1201–10. doi:10.1007/s11090-016-9713-6
26. Peng P, Cheng Y, Hatzenbeller R, Addy M, Zhou N, Schiappacasse C, et al. Ru-based multifunctional mesoporous catalyst for low-pressure and non-thermal plasma synthesis of ammonia. Int J Hydrogen Energ (2017) 42:19056–66. doi:10.1016/j.ijhydene.2017.06.118
27. Li S, van Raak T, Gallucci F. Investigating the operation parameters for ammonia synthesis in dielectric barrier discharge reactors. J Phys D Appl Phys (2020) 53:014008. doi:10.1088/1361-6463/ab4b37
28. Zhu X, Hu X, Wu X, Cai Y, Zhang H, Tu X. Ammonia synthesis over γ -Al 2 O 3 pellets in a packed-bed dielectric barrier discharge reactor. J Phys D Appl Phys (2020) 53:164002. doi:10.1088/1361-6463/ab6cd1
29. Xie Q, Zhuge S, Song X, Lu M, Yu F, Ruan R, et al. Non-thermal atmospheric plasma synthesis of ammonia in a DBD reactor packed with various catalysts. J Phys D Appl Phys (2020) 53:064002. doi:10.1088/1361-6463/ab57e5
30. Liu C, Brown NMD, Meenan BJ. Uniformity analysis of dielectric barrier discharge (DBD) processed polyethylene terephthalate (PET) surface. Appl Surf Sci (2006) 252:2297–310. doi:10.1016/j.apsusc.2005.04.016
31. Zeng X, Zhang S, Liu Y, Hu X, Ostrikov KK, Shao T. Energy-efficient pathways for pulsed-plasma-activated sustainable ammonia synthesis. ACS Sustain Chem Eng (2023) 11:1110–20. doi:10.1021/acssuschemeng.2c06259
32. Bai M, Zhang Z, Bai M, Bai X, Gao H. Synthesis of ammonia using CH4/N2 plasmas based on micro-gap discharge under environmentally friendly condition. Plasma Chem Plasma Process (2008) 28:405–14. doi:10.1007/s11090-008-9132-4
33. Ma Y, Tian Y, Zeng Y, Tu X. Plasma synthesis of ammonia in a tangled wire dielectric barrier discharge reactor: effect of electrode materials. J Energ Inst (2021) 99:137–44. doi:10.1016/j.joei.2021.09.002
34. Ambrico PF, Šimek M, Morano M, De Miccolis Angelini RM, Minafra A, Trotti P, et al. Reduction of microbial contamination and improvement of germination of sweet basil (Ocimum basilicum L.) seeds via surface dielectric barrier discharge. J Phys D Appl Phys (2017) 50:305401. doi:10.1088/1361-6463/aa77c8
35. Ambrico PF, Šimek M, Ambrico M, Morano M, Prukner V, Minafra A, et al. On the air atmospheric pressure plasma treatment effect on the physiology, germination and seedlings of basil seeds. J Phys D Appl Phys (2020) 53:104001. doi:10.1088/1361-6463/ab5b1b
36. Rusanov VD, Fridman AA, Sholin GV. The physics of a chemically active plasma with nonequilibrium vibrational excitation of molecules. Soviet Phys Uspekhi (1981) 24:447–74. doi:10.1070/PU1981v024n06ABEH004884
37. Rouwenhorst KHR, Jardali F, Bogaerts A, Lefferts L. From the Birkeland–Eyde process towards energy-efficient plasma-based NO X synthesis: a techno-economic analysis. Energ Environ Sci (2021) 14:2520–34. doi:10.1039/D0EE03763J
38. Ingels R, Graves DB. Improving the efficiency of organic fertilizer and nitrogen use via air plasma and distributed renewable energy. Plasma Med (2015) 5:257–70. doi:10.1615/PlasmaMed.2016015763
39. Capitelli M, Celiberto R, Colonna G, Esposito F, Gorse C, Hassouni K, et al. Fundamental aspects of plasma chemical physics. New York, NY: Springer New York (2016). doi:10.1007/978-1-4419-8185-1
40. Fridman A. Plasma chemistry. Cambridge: Cambridge University Press (2008). doi:10.1017/CBO9780511546075
41. Azizov R, Zhivotov V, Krotov M, Rusanov V, Tarasov Y, Fridman A. Synthesis of nitrogen oxides in a nonequilibrium microwave discharge under electron-cyclotron resonance conditions. Khimiya Vysok Energii (1980) 14:366–8.
42. Gordiets BF, Ferreira CM, Guerra VL, Loureiro JMAH, Nahorny J, Pagnon D, et al. Kinetic model of a low-pressure N2–O2 flowing glow discharge. IEEE Trans Plasma Sci (1995) 23:750–68. doi:10.1109/27.467998
43. Gamallo P, González M, Sayós R. Ab initio derived analytical fits of the two lowest triplet potential energy surfaces and theoretical rate constants for the N(4S)+NO(X 2Π) system. J Chem Phys (2003) 119:2545–56. doi:10.1063/1.1586251
44. Akpinar S, Armenise I, Defazio P, Esposito F, Gamallo P, Petrongolo C, et al. Quantum mechanical and quasiclassical Born–Oppenheimer dynamics of the reaction N2 on the N2O and surfaces. Chem Phys (2012) 398:81–9. doi:10.1016/j.chemphys.2011.05.005
45. Esposito F, Armenise I. Reactive, inelastic, and dissociation processes in collisions of atomic oxygen with molecular nitrogen. J Phys Chem A (2017) 121:6211–9. doi:10.1021/acs.jpca.7b04442
46. Esposito F. On the relevance of accurate input data for vibrational kinetics in air cold plasmas: the case of nitrogen fixation. Plasma Sourc Sci Technol (2022) 31:094010. doi:10.1088/1361-6595/ac9082
47. Armenise I, Esposito F. N2, O2, NO state-to-state vibrational kinetics in hypersonic boundary layers: the problem of rescaling rate coefficients to uniform vibrational ladders. Chem Phys (2015) 446:30–46. doi:10.1016/j.chemphys.2014.11.004
48. Armenise I. Low temperature state-to-state vibrational kinetics of O + N2(v) and N + O2(v) collisions. Chem Phys (2023) 571:111937. doi:10.1016/j.chemphys.2023.111937
49. Esposito F, Armenise I. Reactive, inelastic, and dissociation processes in collisions of atomic nitrogen with molecular oxygen. J Phys Chem A (2021) 125:3953–64. doi:10.1021/acs.jpca.0c09999
50. Armenise I, Esposito F. N + O2(v) collisions: reactive, inelastic and dissociation rates for state-to-state vibrational kinetic models. Chem Phys (2021) 551:111325. doi:10.1016/j.chemphys.2021.111325
51. Ivanov MV, Schinke R, Mcbane GC. Theoretical investigation of vibrational relaxation of NO(2 Π), O, and N in collisions with O(3 P). Mol Phys (2007) 105:1183–91. doi:10.1080/00268970701288087
52. Hong Q, Bartolomei M, Esposito F, Coletti C, Sun Q, Pirani F. Reconciling experimental and theoretical vibrational deactivation in low-energy O + N 2 collisions. Phys Chem Chem Phys (2021) 23:15475–9. doi:10.1039/D1CP01976G
53. Hong Q, Bartolomei M, Pirani F, Esposito F, Sun Q, Coletti C. Vibrational deactivation in O(3 P) + N 2 collisions: from an old problem towards its solution. Plasma Sourc Sci Technol (2022) 31:084008. doi:10.1088/1361-6595/ac86f3
54. Esposito F. Reactivity, relaxation and dissociation of vibrationally excited molecules in low-temperature plasma modeling. Rend Lincei Sci Fis Nat (2019) 30:57–66. doi:10.1007/s12210-019-00778-9
55. Lino da Silva M, Loureiro J, Guerra V. A multiquantum dataset for vibrational excitation and dissociation in high-temperature O2–O2 collisions. Chem Phys Lett (2012) 531:28–33. doi:10.1016/j.cplett.2012.01.074
56. Esposito F, Armenise I, Capitelli M. N–N2 state to state vibrational-relaxation and dissociation rates based on quasiclassical calculations. Chem Phys (2006) 331:1–8. doi:10.1016/j.chemphys.2006.09.035
57. Esposito F, Armenise I, Capitta G, Capitelli M. O–O2 state-to-state vibrational relaxation and dissociation rates based on quasiclassical calculations. Chem Phys (2008) 351:91–8. doi:10.1016/j.chemphys.2008.04.004
58. Bose D, Candler GV. Thermal rate constants of the O2+N→NO+O reaction based on the A2′and A4′ potential-energy surfaces. J Chem Phys (1997) 107:6136–45. doi:10.1063/1.475132
59. Pipa AV, Koskulics J, Brandenburg R, Hoder T. The simplest equivalent circuit of a pulsed dielectric barrier discharge and the determination of the gas gap charge transfer. Rev Scientific Instr (2012) 83:115112. doi:10.1063/1.4767637
60. Pipa AV, Koskulics J, Brandenburg R, Hoder T. Erratum: “The simplest equivalent circuit of a pulsed dielectric barrier discharge and the determination of the gas gap charge transfer”. Rev Sci Instrum (2012) 83:115112. doi:10.1063/1.4767637
61. Archambault-Caron M, Gagnon H, Nisol B, Piyakis K, Wertheimer MR. Precise energy and temperature measurements in dielectric barrier discharges at atmospheric pressure. Plasma Sourc Sci Technol (2015) 24:045004. doi:10.1088/0963-0252/24/4/045004
62. Pei X, Gidon D, Yang YJ, Xiong Z, Graves DB. Reducing energy cost of NOx production in air plasmas. Chem Eng J (2019) 362:217–28. doi:10.1016/j.cej.2019.01.011
63. Vervloessem E, Aghaei M, Jardali F, Hafezkhiabani N, Bogaerts A. Plasma-based N 2 fixation into NO x: insights from modeling toward optimum yields and energy costs in a Gliding Arc plasmatron. ACS Sustain Chem Eng (2020) 8:9711–20. doi:10.1021/acssuschemeng.0c01815
64. Patil BS, Peeters FJJ, van Rooij GJ, Medrano JA, Gallucci F, Lang J, et al. Plasma assisted nitrogen oxide production from air: using pulsed powered gliding arc reactor for a containerized plant. AIChE J (2018) 64:526–37. doi:10.1002/aic.15922
65. Wang W, Patil B, Heijkers S, Hessel V, Bogaerts A. Nitrogen fixation by Gliding Arc plasma: better insight by chemical kinetics modelling. ChemSusChem (2017) 10:2145–57. doi:10.1002/cssc.201700095
66. Tsonev I, O’Modhrain C, Bogaerts A, Gorbanev Y. Nitrogen fixation by an arc plasma at elevated pressure to increase the energy efficiency and production rate of NO x. ACS Sustain Chem Eng (2023) 11:1888–97. doi:10.1021/acssuschemeng.2c06357
67. Muzammil I, Lee DH, Dinh DK, Kang H, Roh SA, Kim Y-N, et al. A novel energy efficient path for nitrogen fixation using a non-thermal arc. RSC Adv (2021) 11:12729–38. doi:10.1039/D1RA01357B
68. Chen H, Wu A, Mathieu S, Gao P, Li X, Xu BZ, et al. Highly efficient nitrogen fixation enabled by an atmospheric pressure rotating gliding arc. Plasma Process Polym (2021) 18:18. doi:10.1002/ppap.202000200
69. Van Alphen S, Ahmadi Eshtehardi H, O’Modhrain C, Bogaerts J, Van Poyer H, Creel J, et al. Effusion nozzle for energy-efficient NOx production in a rotating gliding arc plasma reactor. Chem Eng J (2022) 443:136529. doi:10.1016/j.cej.2022.136529
70. Rehbein N, Cooray V. NO production in spark and corona discharges. J Electrostat (2001) 51–52:333–9. doi:10.1016/S0304-3886(01)00115-2
71. Janda M, Martišovitš V, Hensel K, Machala Z. Generation of antimicrobial NOx by atmospheric air transient spark discharge. Plasma Chem Plasma Process (2016) 36:767–81. doi:10.1007/s11090-016-9694-5
72. Pavlovich MJ, Ono T, Galleher C, Curtis B, Clark DS, Machala Z, et al. Air spark-like plasma source for antimicrobial NOxgeneration. J Phys D Appl Phys (2014) 47:505202. doi:10.1088/0022-3727/47/50/505202
73. Abdelaziz AA, Teramoto Y, Nozaki T, Kim H-H. Toward reducing the energy cost of NO x formation in a spark discharge reactor through pinpointing its mechanism. ACS Sustain Chem Eng (2023) 11:4106–18. doi:10.1021/acssuschemeng.2c06535
74. Zhang S, Zong L, Zeng X, Zhou R, Liu Y, Zhang C, et al. Sustainable nitrogen fixation with nanosecond pulsed spark discharges: insights into free-radical-chain reactions. Green Chem (2022) 24:1534–44. doi:10.1039/D1GC03859A
75. Patil BS, Cherkasov N, Lang J, Ibhadon AO, Hessel V, Wang Q. Low temperature plasma-catalytic NO x synthesis in a packed DBD reactor: effect of support materials and supported active metal oxides. Appl Catal B (2016) 194:123–33. doi:10.1016/j.apcatb.2016.04.055
76. Roy NC, Maira N, Pattyn C, Remy A, Delplancke M-P, Reniers F. Mechanisms of reducing energy costs for nitrogen fixation using air-based atmospheric DBD plasmas over water in contact with the electrode. Chem Eng J (2023) 461:141844. doi:10.1016/j.cej.2023.141844
77. Li Y, Qin L, Wang H-L, Li S-S, Yuan H, Yang D-Z. High efficiency NOx synthesis and regulation using dielectric barrier discharge in the needle array packed bed reactor. Chem Eng J (2023) 461:141922. doi:10.1016/j.cej.2023.141922
78. Mutel B, Dessaux O, Goudmand P. Energy cost improvement of the nitrogen oxides synthesis in a low pressure plasma. Revue de Physique Appliquée (1984) 19:461–4. doi:10.1051/rphysap:01984001906046100
79. Kim T, Song S, Kim J, Iwasaki R. Formation of NO x from air and N 2/O 2 mixtures using a nonthermal microwave plasma system. Jpn J Appl Phys (2010) 49:126201. doi:10.1143/JJAP.49.126201
80. Peng P, Chen P, Addy M, Cheng Y, Zhang Y, Anderson E, et al. In situ plasma-assisted atmospheric nitrogen fixation using water and spray-type jet plasma. Chem Commun (2018) 54:2886–9. doi:10.1039/c8cc00697k
81. Gorbanev Y, Vervloessem E, Nikiforov A, Bogaerts A. Nitrogen fixation with water vapor by nonequilibrium plasma: toward sustainable ammonia production. ACS Sustain Chem Eng (2020) 8:2996–3004. doi:10.1021/acssuschemeng.9b07849
82. Toth JR, Abuyazid NH, Lacks DJ, Renner JN, Sankaran RM. A plasma-water droplet reactor for process-intensified, continuous nitrogen fixation at atmospheric pressure. ACS Sustain Chem Eng (2020) 8:14845–54. doi:10.1021/acssuschemeng.0c04432
83. Peng P, Schiappacasse C, Zhou N, Addy M, Cheng Y, Zhang Y, et al. Plasma in situ gas–liquid nitrogen fixation using concentrated high-intensity electric field. J Phys D Appl Phys (2019) 52:494001. doi:10.1088/1361-6463/ab3ea6
84. Kumari S, Pishgar S, Schwarting ME, Paxton WF, Spurgeon JM. Synergistic plasma-assisted electrochemical reduction of nitrogen to ammonia. Chem Commun (2018) 54:13347–50. doi:10.1039/C8CC07869F
85. Hawtof R, Ghosh S, Guarr E, Xu C, Mohan Sankaran R, Renner JN. Catalyst-free, highly selective synthesis of ammonia from nitrogen and water by a plasma electrolytic system. Sci Adv (2019) 5:eaat5778. doi:10.1126/sciadv.aat5778
86. Sakakura T, Uemura S, Hino M, Kiyomatsu S, Takatsuji Y, Yamasaki R, et al. Excitation of H 2 O at the plasma/water interface by UV irradiation for the elevation of ammonia production. Green Chem (2018) 20:627–33. doi:10.1039/C7GC03007J
87. Sakakura T, Murakami N, Takatsuji Y, Haruyama T. Nitrogen fixation in a plasma/liquid interfacial reaction and its switching between reduction and oxidation. The J Phys Chem C (2020) 124:9401–8. doi:10.1021/acs.jpcc.0c02392
88. Sakakura T, Murakami N, Takatsuji Y, Morimoto M, Haruyama T. Contribution of discharge excited atomic N, N 2 *, and N 2 + to a plasma/liquid interfacial reaction as suggested by quantitative analysis. ChemPhysChem (2019) 20:1467–74. doi:10.1002/cphc.201900212
89. Haruyama T, Namise T, Shimoshimizu N, Uemura S, Takatsuji Y, Hino M, et al. Non-catalyzed one-step synthesis of ammonia from atmospheric air and water. Green Chem (2016) 18:4536–41. doi:10.1039/C6GC01560C
90. Fangueiro D, Hjorth M, Gioelli F. Acidification of animal slurry– a review. J Environ Manage (2015) 149:46–56. doi:10.1016/j.jenvman.2014.10.001
91. Sutton MA, Bleeker A, Howard CM, Erisman JW, Abrol YP, Bekunda M, et al. Our nutrient world: the challenge to produce more food and energy with less pollution. Centre Ecol and Hydrol behalf Glob Partnership Nutrient Manag (Gpnm) Int Nitrogen Initiat (Ini) (2013).
92. Graves DB, Bakken LB, Jensen MB, Ingels R. Plasma activated organic fertilizer. Plasma Chem Plasma Process (2019) 39:1–19. doi:10.1007/s11090-018-9944-9
93. Zhang S, Rousseau A, Dufour T. Promoting lentil germination and stem growth by plasma activated tap water, demineralized water and liquid fertilizer. RSC Adv (2017) 7:31244–51. doi:10.1039/c7ra04663d
94. Cortese E, Settimi AG, Pettenuzzo S, Cappellin L, Galenda A, Famengo A, et al. Plasma-activated water triggers rapid and sustained cytosolic Ca2+ elevations in arabidopsis thaliana. Plants (2021) 10:2516. doi:10.3390/plants10112516
95. Adhikari B, Adhikari M, Ghimire B, Park G, Choi EH. Cold atmospheric plasma-activated water irrigation induces defense hormone and gene expression in tomato seedlings. Sci Rep (2019) 9:16080. doi:10.1038/s41598-019-52646-z
96. Vichiansan N, Chatmaniwat K, Sungkorn M, Leksakul K, Chaopaisarn P, Boonyawan D. Effect of plasma-activated water generated using plasma jet on tomato (Solanum lycopersicum L. Var. cerasiforme) seedling growth. J Plant Growth Regul (2023) 42:935–45. doi:10.1007/s00344-022-10603-7
97. Aceto D, Rotondo PR, Porfido C, Bottiglione B, Paciolla C, Terzano R, et al. Assessing plasma activated water irrigation effects on tomato seedlings. Front Phys (2024) 12. doi:10.3389/fphy.2024.1399910
98. Zambon Y, Contaldo N, Laurita R, Várallyay E, Canel A, Gherardi M, et al. Plasma activated water triggers plant defence responses. Sci Rep (2020) 10:19211. doi:10.1038/s41598-020-76247-3
99. Thirumdas R, Kothakota A, Annapure U, Siliveru K, Blundell R, Gatt R, et al. Plasma activated water (PAW): chemistry, physico-chemical properties, applications in food and agriculture. Trends Food Sci Technol (2018) 77:21–31. doi:10.1016/j.tifs.2018.05.007
Keywords: nitrogen fixation, air plasma, atmospheric pressure cold plasmas, Zel’dovich reaction, modelling
Citation: Aceto D, Ambrico PF and Esposito F (2024) Air cold plasmas as a new tool for nitrogen fixation in agriculture: underlying mechanisms and current experimental insights. Front. Phys. 12:1455481. doi: 10.3389/fphy.2024.1455481
Received: 26 June 2024; Accepted: 13 September 2024;
Published: 09 October 2024.
Edited by:
Mario J. Pinheiro, University of Lisbon, PortugalReviewed by:
Biswajit Sahu, West Bengal State University, IndiaCopyright © 2024 Aceto, Ambrico and Esposito. This is an open-access article distributed under the terms of the Creative Commons Attribution License (CC BY). The use, distribution or reproduction in other forums is permitted, provided the original author(s) and the copyright owner(s) are credited and that the original publication in this journal is cited, in accordance with accepted academic practice. No use, distribution or reproduction is permitted which does not comply with these terms.
*Correspondence: Paolo F. Ambrico, cGFvbG9mcmFuY2VzY28uYW1icmljb0BjbnIuaXQ=