- 1Kavli Institute for the Physics and Mathematics of the Universe (WPI), University of Tokyo Institutes for Advanced Study, University of Tokyo, Kashiwa, Japan
- 2Section de Physique, University of Geneva, Geneva, Switzerland
Introduction: The recent discovery of coherent elastic neutrino-nucleus scattering (CEvNS) has created new opportunities to detect and study neutrinos. The interaction cross section in CEvNS scales quadratically with the number of neutrons, making heavy-nuclei targets such as active lead-based detectors ideal. Lead perovskites have emerged in the last decade as revolutionary materials for radiation detection due to their heavy and flexible element composition and their unique optoelectronic properties that result in an excellent energy resolution at an economic cost.
Methodology: In this study, we discuss, for the first time, the physics potential and feasibility of building neutrino detectors using semiconductor lead perovskite crystals as a target.
Results and Discussion: We indicate that existing data with x-rays suggest the suitability of existing lead perovskite sensors to study CEvNS using neutrinos from π decay at rest (π- DAR) sources. Although dedicated research and development will be necessary, we have found significant benefits and no inherent obstacles for the development of lead perovskites as CEvNS detectors.
1 Introduction
Neutrinos are the only known fermions carrying exclusively weak charges and, therefore, are clean probes of the weak interaction and unique messengers of dense matter environments, unaffected by strong and electromagnetic interactions. These appeals, however, result in notably suppressed interaction cross sections, hampering the study of neutrino physics and rendering most applications impractical.
In 1974, the existence of coherent elastic neutrino-nucleus scattering (CEνNS) was pointed out as a consequence of the standard model [1]. In CEνNS, a neutrino transfers momentum to a whole nucleus via the exchange of a virtual Z boson, forcing it to recoil. The interaction cross section for this process is
where GF is the Fermi constant, N (Z) is the number of neutrons (protons), θW is the Weinberg angle, and mN and ER are the nucleon mass and its recoil energy, respectively. The nuclear form factor f(q) characterizes the loss of coherence as a function of the transferred momentum q =
2 Motivations
The discovery of CEνNS and its enhanced cross section shows potential to mitigate the elusiveness of neutrinos and therefore revolutionize their study at energies on the order of a few tens of MeV, which include geoneutrinos [5], reactor neutrinos [6], accelerator neutrinos from meson decays at rest [7-10], solar neutrinos [11], and supernova neutrino bursts [12]. Characterizing the cross section of CEνNS is also essential for dark matter searches as CEνNS constitutes an irreducible background, the so-called neutrino floor [13]. Being mediated by flavor-insensitive neutral currents, the detection of CEνNS provides extended sensitivity to sterile neutrinos [14-16] and other new physics signatures [17-20], and allows the study of the neutrino magnetic moment [21, 22], its effective charge radius [23], and the nuclear neutron form factor [24, 25]. Applications, such as deploying neutrino detectors to increase nuclear security [26, 27], might also be possible. Moreover, CEνNS is relevant to theoretical astrophysics as a key actor during stellar collapse [28-30].
3 CEνNS experiments
Because of the aforementioned findings, an increasing number of CEνNS detector technologies have been proposed [31-42], and several experiments are ongoing or have been proposed: COHERENT [43], using CsI, NaI, high-purity Ge (HPGe), and liquid-Ar targets; CONUS [44], NCC-1701 [45, 46], and νGEN [47] using cryogenic HPGe; MINER [48], using cryogenic HPGe/Si; NUCLEUS [49], using cryogenic CaWO4 and Al2O3; CONNIE [44], using Si charge-coupled devices (CCDs); TEXONO [50], using p-type point-contact Ge; RES-NOVA, using cryogenic PbWO4 [51, 52]; RICOCHET [53], using cryogenic HPGe bolometers; and RED100 [54], using liquid-Xe.
To get the most from CEνNS, an ideal detector should be inexpensive to produce and operate, have excellent energy resolutions to identify nuclear recoils with an energy of a few keV, and be made of heavy nuclear targets to exploit the quadratic scaling of the cross section. In this study, we point out, for the first time, the excellent prospects of lead perovskites to build up future CEνNS detectors and discuss their experimental feasibility in light of existing measurements.
4 Lead perovskites
Lead halide perovskites (LHPs) are novel semiconductors with exceptional optoelectronic properties, a versatile chemical composition, and low-cost synthesis. They typically consist of crystals with structure APbX3, as shown in Figure 1, where A is CH3NH3+ (MA+),
The study of halide perovskites as photosensors was sparked about a decade ago in the context of solar cell development [56] and quickly emerged as an active field of research due to record energy conversion efficiencies [57-64]. Along the process, much has been learned about the basic properties of this material, which combines a low exciton binding energy on the order of few meV [65] with exceptionally long electron–hole diffusion lengths exceeding 1 μm [66], a tunable band gap in the range of 1.2–2.4 eV [67-68], and a high bulk resistivity of 107−10Ω⋅cm at room temperature [69]. The aforementioned combination is unique as it pairs efficient charge carrier production and mobility at a low voltage bias with a high bulk resistivity and orders of magnitude higher than those of Si and Ge, suppressing dark current and noise. Moreover, LHPs naturally allow for the manufacture of crystals with very high atomic numbers, such as CsPbI3, and the design of application-specific perovskite sensors by means of stoichiometry engineering [70, 71]. Furthermore, the synthesis of LHPs is easy and flexible through techniques such as solution processing and melt growth, and single crystals with sizes > 1cm3 can be routinely built [72]. The production cost is also low, with an estimated price of <0.3$/cm3 [55], namely, at a density of 4 g/cm3 and an inexpensive cost of 75 $/kg. Finally, LHPs can be operated inexpensively at room temperature.
5 Perovskites as radiation detectors
Lead perovskites' striking performance as solar cells and their high atomic numbers1 quickly attracted the interest of the medical imaging community toward this material as x/γ-ray detectors [73-81]. In 2015, MAPbI3 was proven to detect γ-rays from 137Cs [82], and the first x-ray images were obtained [83]. Since then, a steady improvement in x/γ-ray performance metrics and achievements has been reported over time [72, 74, 83, 84], including the best x-ray sensitivities yet achieved in any material [85, 86]. Other radiation types have also been studied with perovskites, specifically neutrons [87] and α [88, 89] and β [90] particles. For a recent review, see [91]. Moreover, perovskite nanoparticles show enormous potential as wavelength shifters, for a review see [92], making them interesting doping materials (≈1 g/L) to build nanocrystal-doped liquid scintillators, with applications in neutrino detection [93]. In this study, nevertheless, we focus on solid lead perovskite crystals as a target, i.e., a detector where 100% of the active volume is made of lead perovskite, enhancing the cross section of CEνNS enhanced due to the presence of lead. As crystals, despite the many achievements that are previously listed, all reported precision measurements involving low-energy O (10–100) keV particles have been based on the detection of recoiling electrons induced by x-ray interactions. Possibly because of this finding, no mentions exist in the literature about the possibility of measuring CEνNS using perovskites. In CEνNS, a nuclear recoil, instead of an electron recoil, needs to be measured. For Ge, it has been measured that nuclear recoils generate about a third of the ionization signal of their electronic counterparts [94]. For lead perovskites, this fraction, the so-called quenching Q, is still unknown. Quenching acts by reducing the signal, therefore, reported sensor metrics in x/γ-ray measurements that are expected to degrade when used to study CEνNS. If Qperovskite ≈ QGe, then the energy resolution Eres for nuclear and electron recoils can be related by
Existing
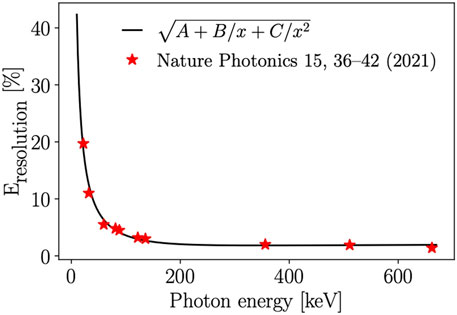
FIGURE 2. Fit to the energy resolution measured for single photons using a CsPbBr3 perovskite at room temperature. Data from [95]. The best parameters are A, B, and C = {4.95, −1098.24, and 189690.18}.
6 Prospects as CEνNS detectors
Producing low-activity lead perovskites should be readily possible, e.g., CsPbI3 consists of Cs and I, both used in the first historical detection of CEνNS [3], and archaeological Pb has recently been demonstrated to be adequate for CEνNS detection [102]. Moreover, CsPbI3 and other lead perovskites are made up of strikingly heavy elements, significantly advantaging the CEνNS interaction cross section of mainstream alternative materials and, in particular, that of Ge. However, the maximum recoil energy decreases linearly with mN, and therefore, the ability of the detector to identify the recoiling nucleus needs to be considered. To account for it, we define the effective cross section, σeff, as a figure of merit, defined as
which can be calculated from Eq. 1 if the detector efficiency, ϵ, is specified. Using it, in Figure 3 CsPbI3 and Ge targets2 are directly compared for some neutrino energies, assuming a detector with perfect (null) efficiency above (below) a certain energy recoil threshold,
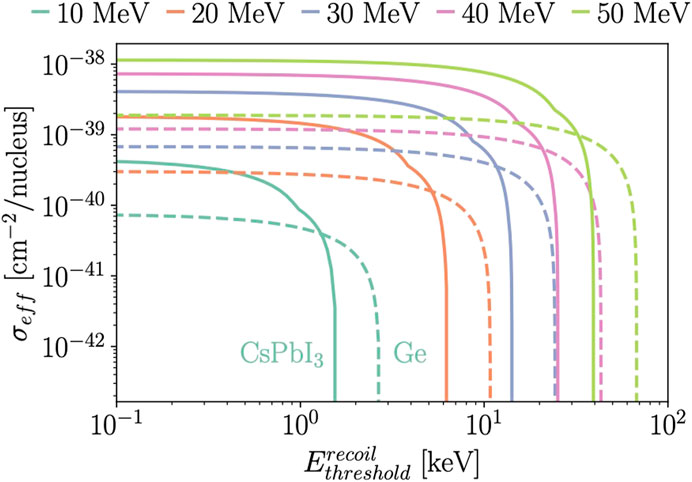
FIGURE 3. CEνNS interaction cross section per nucleus, σ, multiplied by the detector efficiency, ϵ, as a function of the recoil energy threshold,
If, as suggested in the previous section,
The comparison between CsPbI3 and Ge in Figure 3 reflects that for a given fixed neutrino energy, lead perovskites require a smaller
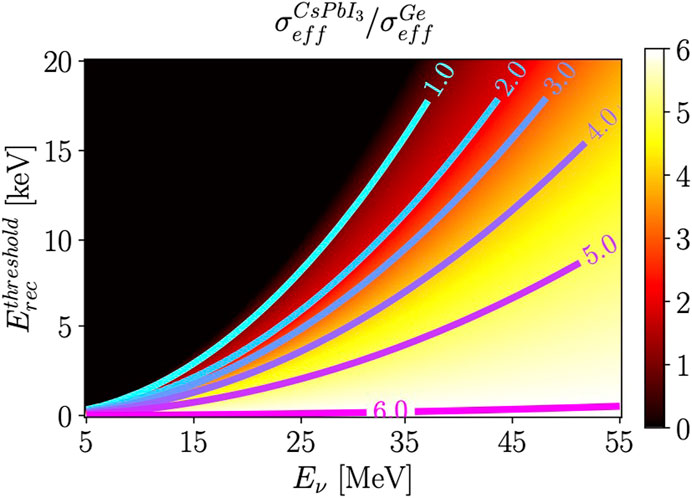
FIGURE 4. The color map depicts the ratio of
7 Discussion and outlook
In just one decade, lead perovskites have been established as novel materials with transformative potential as radiation detectors due to their unique optoelectronic properties.
In this study, we highlight their potential as neutrino detector targets and discussed, for the first time, their suitability for the study of CEνNS. In particular, we note that existing x-ray data indicate that current lead perovskites sensors might already be suitable to study π-DAR neutrinos and discuss their implications. In general, with the available data, no impediments are apparent that prevent further development of the concept of lead perovskites for neutrino detection. Nonetheless, we highlight the necessity of determining the quenching fraction for recoiling the nucleus in lead perovskites to evaluate its exact effect. In any case, to bring perovskites to their ultimate detection potential and enable their full range of applications, active R&D is required. In particular, efforts to optimize lead perovskite sensors for the detection of single low-energy particles would be significantly beneficial for the development of this technology within the field of experimental neutrino physics.
Lastly, we note that CEνNS and some dark-matter models share the same signal mechanism, i.e., the detection of nuclear recoils. Therefore, any progress in this direction might benefit both the neutrino and dark-matter research communities.
Data availability statement
The original contributions presented in the study are included in the article/Supplementary Material; further inquiries can be directed to the corresponding authors.
Author contributions
CJ-V and FS contributed to the conception and design of the study. CJ-V performed the cross section calculations. CJ-V wrote the first draft of the manuscript. CJ-V and FS wrote sections of the manuscript. All authors contributed to the article and approved the submitted version.
Funding
This project was partially inspired by the ZPro project funded by the Barcelona Institute of Technology (BIST). Open access funding was provided by the University of Geneva.
Acknowledgments
The authors acknowledge fruitful discussions with E. Palomares and valuable feedback from J. I. Collar and L. Pattavina.
Conflict of interest
The authors declare that the research was conducted in the absence of any commercial or financial relationships that could be construed as a potential conflict of interest.
Publisher’s note
All claims expressed in this article are solely those of the authors and do not necessarily represent those of their affiliated organizations, or those of the publisher, the editors, and the reviewers. Any product that may be evaluated in this article, or claim that may be made by its manufacturer, is not guaranteed or endorsed by the publisher.
Footnotes
1Photon attenuation increases ∝ Z4, where Z is the atomic number.
2For CsPbI3, the weighted average (Cs + Pb+3I)/5 is used in the result of Eq. 3.
3Perovskites might be able to operate without the need for cryogenic systems, as supported by existing x-ray data.
References
1. Freedman DZ. Coherent effects of a weak neutral current. Phys Rev D (1974) 9:1389–92. doi:10.1103/physrevd.9.1389
2. Scholberg K. Coherent elastic neutrino-nucleus scattering. J Phys Conf Ser (2020) 1468:012126. doi:10.1088/1742-6596/1468/1/012126
3. Akimov D, Albert JB, An P, Awe C, Barbeau PS, Becker B, et al. Observation of coherent elastic neutrino-nucleus scattering. Science (2017) 357:1123. (COHERENT) arXiv:1708.01294 [nucl-ex].
4. Akimov D, Albert JB, An P, Awe C, Barbeau PS, Becke B, et al. First measurement of coherent elastic neutrino-nucleus scattering on argon. Phys Rev Lett (2021) 126:012002. (COHERENT) arXiv:2003.10630 [nucl-ex]. doi:10.1103/PhysRevLett.126.012002
5. Sramek O, McDonough WF, Kite ES, Lekic V, Dye S, Zhong S. Geophysical and geochemical constraints on geoneutrino fluxes from Earth's mantle. Earth Planet Sci Lett (2013) 361:356–66. arXiv:1207.0853 [physics.geo-ph]. doi:10.1016/j.epsl.2012.11.001
6. Qian X, Peng JC. Physics with reactor neutrinos. Rept Prog Phys (2019) 82:036201. arXiv:1801.05386 [hep-ex]. doi:10.1088/1361-6633/aae881
7. Ajimura S, Cheoun MK, Choi JH, Furuta H, Harada M, Hasegawa S, et al. Technical design report (TDR): searching for a sterile neutrino at J-PARC MLF (E56, JSNS2) (2017). arXiv:1705.08629 [physics.ins-det].
8. Garoby R, Vergara A, Danared H, Alonso I, Bargallo E, Cheymol B, et al. The European spallation source design. Phys Scripta (2018) 93:014001. doi:10.1088/1402-4896/aa9bff
9. Wang F, Liang T, Yin W, Yu Q, He L, Tao J, et al. Physical design of target station and neutron instruments for China Spallation Neutron Source. Sci China Phys Mech Astron (2013) 56:2410–24. doi:10.1007/s11433-013-5345-5
10. Alonso J, Avignone FT, Barletta WA, Barlow R, Baumgartner HT. Expression of interest for a novel search for CP violation in the neutrino sector: DAEdALUS (2010). arXiv:1006.0260 [physics.ins-det].
11. Bahcall JN, Pinsonneault MH, Basu S. Solar models: Current epoch and time dependences, neutrinos, and helioseismological properties. Astrophys J (2001) 555:990–1012. arXiv:astro-ph/0010346. doi:10.1086/321493
12. Burrows A, Vartanyan D. Core-collapse supernova explosion theory. Nature (2021) 589:29–39. arXiv:2009.14157 [astro-ph.SR]. doi:10.1038/s41586-020-03059-w
13. Billard J, Strigari L, Figueroa-Feliciano E. Implication of neutrino backgrounds on the reach of next generation dark matter direct detection experiments. Phys Rev D (2014) 89:023524. arXiv:1307.5458 [hep-ph]. doi:10.1103/physrevd.89.023524
14. Anderson AJ, Conrad JM, Figueroa-Feliciano E, Ignarra C, Karagiorgi G, Scholberg K, et al. Measuring active-to-sterile neutrino oscillations with neutral current coherent neutrino-nucleus scattering. Phys Rev D (2012) 86:013004. arXiv:1201.3805 [hep-ph]. doi:10.1103/physrevd.86.013004
15. Dutta B, Gao Y, Mahapatra R, Mirabolfathi N, Strigari LE, Walker JW, et al. Sensitivity to oscillation with a sterile fourth generation neutrino from ultralow threshold neutrino-nucleus coherent scattering. Phys Rev D (2016) 94:093002. arXiv:1511.02834 [hep-ph]. doi:10.1103/physrevd.94.093002
16. Kosmas TS, Papoulias DK, Tortola M, Valle JWF. Probing light sterile neutrino signatures at reactor and Spallation Neutron Source neutrino experiments. Phys Rev D (2017) 96:063013. arXiv:1703.00054 [hep-ph]. doi:10.1103/physrevd.96.063013
17. Krauss LM. Low-energy neutrino detection and precision tests of the standard model. Phys Lett B (1991) 269:407–11. doi:10.1016/0370-2693(91)90192-s
18. Barranco J, Miranda OG, Rashba TI. Sensitivity of low energy neutrino experiments to physics beyond the standard model. Phys Rev D (2007) 76:073008. arXiv:hep-ph/0702175. doi:10.1103/physrevd.76.073008
19. deNiverville P, Pospelov M, Ritz A. Light new physics in coherent neutrino-nucleus scattering experiments. Phys Rev D (2015) 92:095005. arXiv:1505.07805 [hep-ph]. doi:10.1103/physrevd.92.095005
20. Dutta B, Mahapatra R, Strigari LE, Walker JW. Sensitivity toZ-prime and nonstandard neutrino interactions from ultralow threshold neutrino-nucleus coherent scattering. Phys Rev D (2016) 93:013015. arXiv:1508.07981 [hep-ph]. doi:10.1103/physrevd.93.013015
21. Dodd AC, Papageorgiu E, Ranfone S. The effect of a neutrino magnetic moment on nuclear excitation processes. Phys Lett B (1991) 266:434–8. doi:10.1016/0370-2693(91)91064-3
22. Kosmas TS, Miranda OG, Papoulias DK, Tortola M, Valle JWF. Probing neutrino magnetic moments at the Spallation Neutron Source facility. Phys Rev D (2015) 92:013011. arXiv:1505.03202 [hep-ph]. doi:10.1103/physrevd.92.013011
23. Papavassiliou J, Bernabeu J, Passera M. Neutrino-nuclear coherent scattering and the effective neutrino charge radius. PoS (2006) 192. HEP2005 arXiv:hep-ph/0512029. doi:10.48550/arXiv.hep-ph/0512029
24. Patton K, Engel J, McLaughlin GC, Schunck N. Neutrino-nucleus coherent scattering as a probe of neutron density distributions. Phys Rev C (2012) 86:024612. arXiv:1207.0693 [nucl-th]. doi:10.1103/physrevc.86.024612
25. Amanik PS, McLaughlin GC. Nuclear neutron form factor from neutrino–nucleus coherent elastic scattering. J Phys G (2009) 36:015105. doi:10.1088/0954-3899/36/1/015105
26. Stewart C, Abou-Jaoude A, Erickson A. Employing antineutrino detectors to safeguard future nuclear reactors from diversions. Nat Commun (2019) 10:3527. doi:10.1038/s41467-019-11434-z
27. Bernstein A, Bowden N, Goldblum BL, Huber P, Jovanovic I, Mattingly J. Colloquium: Neutrino detectors as tools for nuclear security. Rev Mod Phys (2020) 92:011003. arXiv:1908.07113 [physics.soc-ph]. doi:10.1103/revmodphys.92.011003
28. Wilson JR. Coherent neutrino scattering and stellar collapse. Phys Rev Lett (1974) 32:849–52. doi:10.1103/physrevlett.32.849
29. Schramm DN, Arnett WD. Neutral currents and supernovas. Phys Rev Lett (1975) 34:113–6. doi:10.1103/physrevlett.34.113
30. Freedman DZ, Schramm DN, Tubbs DL. The weak neutral current and its effects in stellar collapse. Ann Rev Nucl Part Sci (1977) 27:167–207. doi:10.1146/annurev.ns.27.120177.001123
31. Drukier A, Stodolsky L. Principles and applications of a neutral-current detector for neutrino physics and astronomy. Phys Rev D (1984) 30:2295–309. doi:10.1103/physrevd.30.2295
32. Cabrera B, Krauss LM, Wilczek F. Bolometric detection of neutrinos. Phys Rev Lett (1985) 55:25–8. doi:10.1103/physrevlett.55.25
33. Formaggio JA, Figueroa-Feliciano E, Anderson AJ. Sterile neutrinos, coherent scattering, and oscillometry measurements with low-temperature bolometers. Phys Rev D (2012) 85:013009. arXiv:1107.3512 [hep-ph]. doi:10.1103/physrevd.85.013009
34. Braggio C, Bressi G, Carugno G, Feltrin E, Galeazzi G. Massive silicon or germanium detectors at cryogenic temperature. Nucl Instrum Meth A (2006) 568:412–5. doi:10.1016/j.nima.2006.06.008
35. Barbeau PS, Collar JI, Tench O. Large-mass ultralow noise germanium detectors: performance and applications in neutrino and astroparticle physics. JCAP (2007) 09:009. arXiv:nucl-ex/0701012. doi:10.1088/1475-7516/2007/09/009
36. Fernandez Moroni G, Estrada J, Paolini EE, Cancelo G, Tiffenberg J, Molina J. Charge coupled devices for detection of coherent neutrino-nucleus scattering. Phys Rev D (2015) 91:072001. arXiv:1405.5761 [physics.ins-det]. doi:10.1103/physrevd.91.072001
37. Horowitz CJ, Coakley KJ, McKinsey DN. Supernova observation via neutrino-nucleus elastic scattering in the CLEAN detector. Phys Rev D (2003) 68:023005. arXiv:astro-ph/0302071. doi:10.1103/physrevd.68.023005
38. Bondar A, Buzulutskov A, Grebenuk A, Pavlyuchenko D, Snopkov R, Tikhonov Y, et al. A two-phase argon avalanche detector operated in a single electron counting mode. Nucl Instrum Meth A (2007) 574:493–9. arXiv:physics/0611068. doi:10.1016/j.nima.2007.01.090
39. Joshi TH, Sangiorgio S, Bernstein A, Foxe M, Hagmann C, Jovanovic I, et al. First measurement of the ionization yield of nuclear recoils in liquid argon, Phys Rev Lett (2014) 112:171303. arXiv:1402.2037 [physics.ins-det]. 10.1103/PhysRevLett.112.171303.
40. Akimov DY, Alexandrov IS, Aleshin VI, Belov VA, Bolozdynya AI, Burenkov AA, et al. Prospects for observation of neutrino-nuclear neutral current coherent scattering with two-phase Xenon emission detector. JINST (2013) 8:P10023. arXiv:1212.1938 [physics.ins-det]. doi:10.1088/1748-0221/8/10/P10023
41. Brice SJ, Cooper RL, DeJongh F, Empl A, Garrison LM, Hime A, et al. A new method for measuring coherent elastic neutrino nucleus scattering at an off-Axis high-energy neutrino beam target. Phys Rev D (2014) 89:072004. arXiv:1311.5958 [physics.ins-det]. doi:10.1103/PhysRevD.89.072004
42. Collar JI, Fields NE, Hai M, Hossbach TW, Orrell JL, Overman CT, et al. Coherent neutrino-nucleus scattering detection with a CsI[Na] scintillator at the SNS spallation source. Nucl Instrum Meth A (2015) 773:56–65. arXiv:1407.7524 [physics.ins-det]. doi:10.1016/j.nima.2014.11.037
43. Akimov D, An P, Awe C, Barbeau PS, Barton P, Becker B, et al. “The COHERENT experiment at the spallation neutron source (2015). (COHERENT) arXiv:1509.08702 [physics.ins-det].
44. Aguilar-Arevalo A, Bertou X, Bonifazi C, Butner M, Cancelo G, Castaneda Vazquez A, et al. The CONNIE experiment. J Phys Conf Ser (2016) 761:012057. arXiv:1608.01565 [physics.ins-det]. doi:10.1088/1742-6596/761/1/012057
45. Colaresi J, Collar JI, Hossbach TW, Kavner ARL, Lewis CM, Robinson AE, et al. First results from a search for coherent elastic neutrino-nucleus scattering at a reactor site. Phys Rev D (2021) 104:072003. arXiv:2108.02880 [hep-ex]. doi:10.1103/physrevd.104.072003
46. Colaresi J, Collar JI, Hossbach TW, Lewis CM, Yocum KM. Measurement of coherent elastic neutrino-nucleus scattering from reactor antineutrinos. Phys Rev Lett (2022) 129:211802. arXiv:2202.09672 [hep-ex]. doi:10.1103/physrevlett.129.211802
47. Belov V, Brudanin V, Egorov V, Filosofov D, Fomina M, Gurov Y, et al. The νGeN experiment at the kalinin nuclear power plant. JINST (2015) 10:P12011. doi:10.1088/1748-0221/10/12/p12011
48. Agnolet G, Baker W, Barker D, Beck R, Carroll TJ, Cesar J, et al. Background studies for the MINER coherent neutrino scattering reactor experiment. Nucl Instrum Meth A (2017) 853:53. (MINER) arXiv:1609.02066 [physics.ins-det]. doi:10.48550/arXiv.1609.02066
49. Strauss R, Rothe J, Angloher G, Bento A, Gütlein A, Hauff D, et al. The ν-cleus experiment: a gram-scale fiducial-volume cryogenic detector for the first detection of coherent neutrino-nucleus scattering. Eur Phys J C (2017) 77:506. arXiv:1704.04320 [physics.ins-det]. doi:10.1140/epjc/s10052-017-5068-2
50. Singh L, Wong HT. Low energy neutrino physics with sub-keV Ge-detectors at kuo-sheng neutrino laboratory. J Phys Conf Ser (2017) 888:012124. TEXONO. doi:10.1088/1742-6596/888/1/012124
51. Pattavina L, Ferreiro Iachellini N, Tamborra I. Neutrino observatory based on archaeological lead. Phys Rev D (2020) 102:063001. arXiv:2004.06936 [astro-ph.HE]. doi:10.1103/physrevd.102.063001
52. Ferreiro Iachellini N, Pattavina L, Abdelhameed AH, Bento A, Canonica L, Danevich F, et al. Operation of an archaeological lead PbWO$$_4$$ crystal to search for neutrinos from astrophysical sources with a transition edge sensor. J Low Temp Phys (2022) 209:872–8. arXiv:2111.07638 [physics.ins-det]. doi:10.1007/s10909-022-02823-8
53. Augier C, Beaulieu G, Belov V, Berge L, Billard J, Bres G, et al. Ricochet progress and status. J. Low Temp. Phys. (2023) 212:127–137. doi:10.1007/s10909-023-02971-5
54. Akimov DY, Belov V, Bolozdynya A, Dolgolenko A, Efremenko Y, Etenko A, et al. First ground-level laboratory test of the two-phase xenon emission detector RED-100. JINST (2020) 15:P02020. arXiv:1910.06190 [physics.ins-det]. doi:10.1088/1748-0221/15/02/p02020
55. Wei H, Huang J. Halide lead perovskites for ionizing radiation detection. Nat Commun (2019) 10:1.
56. Kojima A, Teshima K, Shirai Y, Miyasaka T. Organometal halide perovskites as visible-light sensitizers for photovoltaic cells. J Am Chem Soc (2009) 131:6050–1. doi:10.1021/ja809598r
57. Green MA, Ho-Baillie A, Snaith HJ. The emergence of perovskite solar cells. Nat Photon (2014) 8:506–14. doi:10.1038/nphoton.2014.134
58. Jung HS, Park NG. Perovskite solar cells: from materials to devices. small (2015) 11:10–25. doi:10.1002/smll.201402767
59. Park NG. Perovskite solar cells: An emerging photovoltaic technology. Mater Today (2015) 18:65–72. doi:10.1016/j.mattod.2014.07.007
60. Correa-Baena J-P, Saliba M, Buonassisi T, Grätzel M, Abate A, Tress W, et al. Promises and challenges of perovskite solar cells. Science (2017) 358:739–44. doi:10.1126/science.aam6323
61. Huang J, Yuan Y, Shao Y, Yan Y. Understanding the physical properties of hybrid perovskites for photovoltaic applications. Nat Rev Mater (2017) 2:1.
62. Yang D, Yang R, Wang K, Wu C, Zhu X, Feng J, et al. High efficiency planar-type perovskite solar cells with negligible hysteresis using EDTA-complexed SnO2. Nat Commun (2018) 9:1. doi:10.1038/s41467-018-05760-x
63. Kim JY, Lee J-W, Jung HS, Shin H, Park NG. High-efficiency perovskite solar cells. Chem Rev (2020) 120:7867–918. doi:10.1021/acs.chemrev.0c00107
64. Yoo JJ, Seo G, Chua MR, Park TG, Lu Y, Rotermund F, et al. Efficient perovskite solar cells via improved carrier management. Nature (2021) 590:587–93. doi:10.1038/s41586-021-03285-w
65. Miyata A, Mitioglu A, Plochocka P, Portugall O, Wang JT-W, Stranks SD, et al. Direct measurement of the exciton binding energy and effective masses for charge carriers in organic–inorganic tri-halide perovskites. Nat Phys (2015) 11:582–7. doi:10.1038/nphys3357
66. Stranks SD, Eperon GE, Grancini G, Menelaou C, Alcocer MJ, Leijtens T, et al. Electron-hole diffusion lengths exceeding 1 micrometer in an organometal trihalide perovskite absorber. Science (2013) 342:341–4. doi:10.1126/science.1243982
67. Ju D, Dang Y, Zhu Z, Liu H, Chueh C-C, Li X, et al. Tunable band gap and long carrier recombination lifetime of stable mixed CH3NH3PbxSn1–xBr3 single crystals. Chem Mater (2018) 30:1556–65. doi:10.1021/acs.chemmater.7b04565
68. Unger E, Kegelmann L, Suchan K, Sörell D, Korte L, Albrecht S. Roadmap and roadblocks for the band gap tunability of metal halide perovskites. J Mater Chem A (2017) 5:11401–9. doi:10.1039/c7ta00404d
69. Pisoni A, Jacimovic J, Barisic OS, Spina M, Gaál R, Forró L, et al. Ultra-low thermal conductivity in organic–inorganic hybrid perovskite CH3NH3PbI3. J Phys Chem Lett (2014) 5:2488–92. doi:10.1021/jz5012109
70. Emara J, Schnier T, Pourdavoud N, Riedl T, Meerholz K, Olthof S. Impact of film stoichiometry on the ionization energy and electronic structure of CH3NH3PbI3Perovskites. Adv Mater (2016) 28:553–9. doi:10.1002/adma.201503406
71. Xiao Y, Jia S, Bu N, Li N, Liu Y, Liu M, et al. Grain and stoichiometry engineering for ultra-sensitive perovskite X-ray detectors. J Mater Chem A (2021) 9:25603–10. doi:10.1039/d1ta07585c
72. He Y, Matei L, Jung HJ, McCall KM, Chen M, Stoumpos CC, et al. High spectral resolution of gamma-rays at room temperature by perovskite CsPbBr3 single crystals. Nat Commun (2018) 9:1. doi:10.1038/s41467-018-04073-3
73. Shrestha S, Fischer R, Matt GJ, Feldner P, Michel T, Osvet A, et al. High-performance direct conversion X-ray detectors based on sintered hybrid lead triiodide perovskite wafers. Nat Photon (2017) 11:436–40. doi:10.1038/nphoton.2017.94
74. Wei W, Zhang Y, Xu Q, Wei H, Fang Y, Wang Q, et al. Monolithic integration of hybrid perovskite single crystals with heterogenous substrate for highly sensitive X-ray imaging. Nat Photon (2017) 11:315–21. doi:10.1038/nphoton.2017.43
75. Kim YC, Kim KH, Son D-Y, Jeong D-N, Seo J-Y, Choi YS, et al. Printable organometallic perovskite enables large-area, low-dose X-ray imaging. Nature (2017) 550:87–91. doi:10.1038/nature24032
76. García de Arquer FP, Armin A, Meredith P, Sargent EH. Solution-processed semiconductors for next-generation photodetectors. Nat Rev Mater (2017) 2:1. doi:10.1038/natrevmats.2016.100
77. Gill HS, Elshahat B, Kokil A, Li L, Mosurkal R, Zygmanski P, et al. Flexible perovskite based X-ray detectors for dose monitoring in medical imaging applications. Phys Med (2018) 5:20–3. doi:10.1016/j.phmed.2018.04.001
78. Zhuang R, Wang X, Ma W, Wu Y, Chen X, Tang L, et al. Highly sensitive X-ray detector made of layered perovskite-like (NH4)3Bi2I9 single crystal with anisotropic response. Nat Photon (2019) 13:602–8. doi:10.1038/s41566-019-0466-7
79. Zhou F, Li Z, Lan W, Wang Q, Ding L, Jin Z. Halide perovskite, a potential scintillator for X-ray detection. Small Methods (2020) 4:2000506. doi:10.1002/smtd.202000506
80. Li X, Meng C, Huang B, Yang D, Xu X, Zeng H. All-perovskite integrated X-ray detector with ultrahigh sensitivity. Adv Opt Mater (2020) 8:2000273. doi:10.1002/adom.202000273
81. Su Y, Ma W, Yang YM. Perovskite semiconductors for direct X-ray detection and imaging. J Semiconductors (2020) 41:051204. doi:10.1088/1674-4926/41/5/051204
82. Dong Q, Fang Y, Shao Y, Mulligan P, Qiu J, Cao L, et al. Electron-hole diffusion lengths > 175 μm in solution-grown CH 3 NH 3 PbI 3 single crystals. Science (2015) 347:967–70. doi:10.1126/science.aaa5760
83. Yakunin S, Sytnyk M, Kriegner D, Shrestha S, Richter M, Matt GJ, et al. Detection of X-ray photons by solution-processed lead halide perovskites. Nat Photon (2015) 9:444–9. doi:10.1038/nphoton.2015.82
84. Wei H, Fang Y, Mulligan P, Chuirazzi W, Fang H-H, Wang C, et al. Sensitive X-ray detectors made of methylammonium lead tribromide perovskite single crystals. Nat Photon (2016) 10:333–9. doi:10.1038/nphoton.2016.41
85. Jiang J, Xiong M, Fan K, Bao C, Xin D, Pan Z, et al. Synergistic strain engineering of perovskite single crystals for highly stable and sensitive X-ray detectors with low-bias imaging and monitoring. Nat Photon (2022) 16:575–81. doi:10.1038/s41566-022-01024-9
86. He Y, Hadar I, De Siena MC, Klepov VV, Pan L, Chung DY, et al. Sensitivity and detection limit of spectroscopic-grade perovskite CsPbBr3 crystal for hard X-ray detection. Adv Funct Mater (2022) 32:2112925. doi:10.1002/adfm.202112925
87. Andričević P, Náfrádi G, Kollár M, Náfrádi B, Lilley S, Kinane C, et al. Hybrid halide perovskite neutron detectors. Scientific Rep (2021) 11:1.
88. He Y, Liu Z, McCall KM, Lin W, Chung DY, Wessels BW, et al. Perovskite CsPbBr3 single crystal detector for alpha-particle spectroscopy. Nucl Instr Methods Phys Res Section A: Acc Spectrometers, Detectors Associated Equipment (2019) 922:217–21. doi:10.1016/j.nima.2019.01.008
89. Xie A, Hettiarachchi C, Maddalena F, Witkowski ME, Makowski M, Drozdowski W, et al. Lithium-doped two-dimensional perovskite scintillator for wide-range radiation detection. Commun Mater (2020) 1:37. doi:10.1038/s43246-020-0038-x
90. Yu D, Wang P, Cao F, Gu Y, Liu J, Han Z, et al. Two-dimensional halide perovskite as β-ray scintillator for nuclear radiation monitoring. Nat Commun (2020) 11:3395. doi:10.1038/s41467-020-17114-7
91. Liu F, Wu R, Zeng Y, Wei J, Li H, Manna L, et al. Halide perovskites and perovskite related materials for particle radiation detection. Nanoscale (2022) 14:6743–60. doi:10.1039/d2nr01292h
92. Dey A, Ye J, De A, Debroye E, Ha SK, Bladt E, et al. State of the art and prospects for halide perovskite nanocrystals. ACS nano (2021) 15:10775–981. doi:10.1021/acsnano.0c08903
93. Graham E, Gooding D, Gruszko J, Grant C, Naranjo B, Winslow L. Light yield of Perovskite nanocrystal-doped liquid scintillator. JINST (2019) 14:P11024. doi:10.1088/1748-0221/14/11/p11024
94. Martineau O, Benoit A, Bergé L, Broniatowski A, Chabert L, Chambon B, et al. Calibration of the EDELWEISS cryogenic heat-and-ionization germanium detectors for dark matter search. Nucl Instrum Meth A (2004) 530:426. arXiv:astro-ph/0310657.
95. He Y, Petryk M, Liu Z, Chica DG, Hadar I, Leak C, et al. CsPbBr3 perovskite detectors with 1.4% energy resolution for high-energy γ-rays. Nat Photon (2021) 15:36–42. doi:10.1038/s41566-020-00727-1
96. Pan Z, Wu L, Jiang J, Shen L, Yao K. Searching for high-quality halide perovskite single crystals toward X-ray detection. J Phys Chem Lett (2022) 13:2851–61. doi:10.1021/acs.jpclett.2c00450
97. Aranda C, Bisquert J, Guerrero A. Impedance spectroscopy of perovskite/contact interface: Beneficial chemical reactivity effect. J Chem Phys (2019) 151:124201. doi:10.1063/1.5111925
98. Lin C-H, Hu L, Guan X, Kim J, Huang C-Y, Huang J-K, et al. Electrode engineering in halide perovskite electronics: Plenty of room at the interfaces. Adv Mater (2022) 34:2108616. doi:10.1002/adma.202108616
99. Song Y, Li L, Bi W, Hao M, Kang Y, Wang A, et al. Atomistic surface passivation of CH 3 NH 3 PbI 3 perovskite single crystals for highly sensitive coplanar-structure X-ray detectors. Research (2020) 2020:5958243. doi:10.34133/2020/5958243
100. Wei H, DeSantis D, Wei W, Deng Y, Guo D, Savenije TJ, et al. Dopant compensation in alloyed CH3NH3PbBr3−xClx perovskite single crystals for gamma-ray spectroscopy. Nat Mater (2017) 16:826–33. doi:10.1038/nmat4927
101. Wu J, Wang L, Feng A, Yang S, Li N, Jiang X, et al. Self-powered FA 0.55 MA 0.45 PbI 3 single-crystal perovskite X-ray detectors with high sensitivity. Adv Funct Mater (2022) 32:2109149. doi:10.1002/adfm.202109149
102. Beeman JW, Benato G, Bucci C, Canonica L, Carniti P, Celi E, et al. Characterization of a kg-scale archaeological lead-based cryogenic detectors for the RES-NOVA experiment. Appl Radiat Isot (2023) 194:110704. arXiv:2206.05116 [physics.ins-det]. doi:10.48550/arXiv.2206.05116
103. Akimov D, An P, Awe C, Barbeau PS, Becke B, Belov V, et al. Simulating the neutrino flux from the spallation neutron source for the COHERENT experiment. Phys Rev D (2022) 106:032003. COHERENT arXiv:2109.11049 [hep-ex].
Keywords: neutrino, nuclear coherent scattering, perovskites, novel detectors, low-energy interactions
Citation: Jesús-Valls C and Sánchez F (2023) Lead perovskites as CEνNS detectors. Front. Phys. 11:1191954. doi: 10.3389/fphy.2023.1191954
Received: 22 March 2023; Accepted: 18 July 2023;
Published: 17 August 2023.
Edited by:
Digesh Raut, Washington College, United StatesReviewed by:
Ge Yang, North Carolina State University, United StatesAtanu Pathak, Purdue University Northwest, United States
Peter R. Hobson, Queen Mary University of London, United Kingdom
Copyright © 2023 Jesús-Valls and Sánchez. This is an open-access article distributed under the terms of the Creative Commons Attribution License (CC BY). The use, distribution or reproduction in other forums is permitted, provided the original author(s) and the copyright owner(s) are credited and that the original publication in this journal is cited, in accordance with accepted academic practice. No use, distribution or reproduction is permitted which does not comply with these terms.
*Correspondence: César Jesús-Valls, Y2VzYXIuamVzdXMtdmFsbHNAaXBtdS5qcA==; Federico Sánchez, ZmVkZXJpY28uc2FuY2hlem5pZXRvQHVuaWdlLmNo