- 1Lawrence Berkeley National Laboratory (Retired), Berkeley, CA, United States
- 2SLAC National Accelerator Laboratory, Menlo Park, CA, United States
- 3Fermi National Accelerator Laboratory, Batavia, IL, United States
For over half a century, high-energy particle accelerators have been a major enabling technology for particle and nuclear physics research as well as sources of X-rays for photon science research in material science, chemistry and biology. Particle accelerators for energy and intensity Frontier research in particle and nuclear physics continuously push the accelerator community to invent ways to increase the energy and improve the performance of accelerators, reduce their cost, and make them more power efficient. The accelerator community has demonstrated imagination and creativity in developing a plethora of future accelerator ideas and proposals. The technical maturity of the proposed facilities ranges from shovel-ready to those that are still largely conceptual. At this time, over 100 contributed papers have been submitted to the Accelerator Frontier of the US particle physics decadal community planning exercise known as Snowmass’2021. These papers cover a broad spectrum of topics: beam physics and accelerator education, accelerators for neutrinos, colliders for Electroweak/Higgs studies and multi-TeV energies, accelerators for Physics Beyond Colliders and rare processes, advanced accelerator concepts, and accelerator technology for Radio Frequency cavities (RF), magnets, targets and sources. This paper provides an overview of the present state of accelerators for particle physics and gives a brief description of some of the major facilities that have been proposed, their perceived advantages and some of the remaining challenges.
1 Introduction
There are more than 30,000 particle accelerators in operation around the world. Most of them use low energy beams (≪ 1 MeV) and are used in industry [1]. Less numerous are high energy accelerators for research where there are just over a hundred, many of which have been recently constructed for photon science. Particle physics requires pushing the accelerated beams to the highest possible energies [2] and to the highest possible intensities [3]. Below, we briefly consider the most actively developing accelerator projects, such as the high energy lepton and hadron colliders and accelerators for neutrino studies and rare processes searches, and outline the status and progress in accelerator beam physics as well as in the core accelerator technologies - magnets, Radio Frequency cavities (RF), plasma, targets and sources. There are about two dozen energy Frontier colliders that complement or exceed the LHC in their discovery potential. Among them is the three TeV center-of-mass (CoM) CLIC option (100 MV/m accelerating gradient, 50 km long), two 100 km circumference pp colliders: the SPPC in China (75 TeV CoM, based on 12 T Superconducting (SC) magnets) and the FCC-hh at CERN (100 TeV CoM, 16 T SC magnets) [4], and a 10–14 TeV CoM μ+μ− collider (10–14 km circumference, 16 T SC magnets) [5].
2 Energy Frontier Colliders
2.1 Lepton Colliders
Understanding the unification of electromagnetism with the weak interactions is one of the cornerstones of the Standard Model of particle physics and the Higgs is the mechanism that breaks the electroweak symmetry and gives particles their mass. Thus, understanding the electroweak/Higgs sector is a major focus of particle physics. At present, there are as many as eight Higgs/ElectroWeak factories under consideration: e+e− colliders such as the CepC in China and FCC-ee at CERN, both about 100 km circumference, which require O (100 MW) RF systems to sustain high luminosity [6]; or an 11 km long CLIC (CERN) two-beam normal-conducting RF linear accelerator with an average gradient of 72 MV/m [7]; or the 21 km long International Linear Collider (ILC) based on super-conducting RF (SRF) linacs with an average gradient of 31.5 MV/m [8]. Besides technical feasibility and the cost, the most critical requirements for a post-LHC energy Frontier collider include the CoM energy reach, the required AC power consumption (see Figure 1), and the required duration and scale of the R&D effort to reach construction readiness - see the discussion in [2; Table 1].
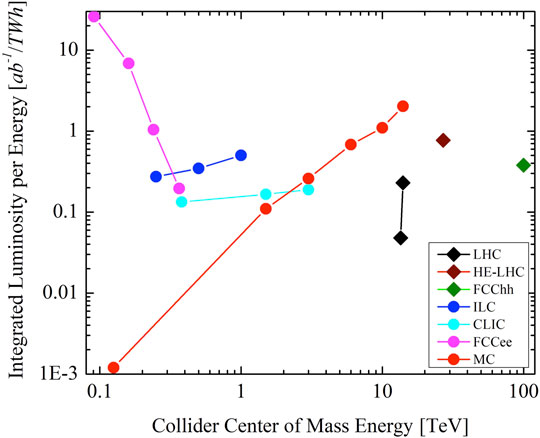
FIGURE 1. Energy efficiency of present and future colliders. Annual integrated luminosity per Terawatt-hour of electric power consumption as a function of the centre-of-mass energy. The LHC—both present and expected after its high-luminosity upgrade (black diamonds) — is contrasted with a variety of proposed particle colliders: the Muon Collider (MC, red circles), the Future Circular electron—positron Collider (FCC-ee, magenta circles) assuming experiments at two collision points, the International Linear Collider (ILC, blue circles), the Compact Linear Collider (CLIC, cyan circles), the High Energy LHC (HE-LHC, magenta diamonds), and the Future Circular proton–proton Collider (FCC-hh, green diamonds). For lepton and hadron colliders operating at the same COM energy, the energy reach of the former is typically larger, by factors that depend, process by process, on the nature of the produced particles and their interactions.
2.1.1 International Linear Collider (ILC)
The ILC is the most technologically mature of the proposed next-generation e+e− colliders - see [8]. The initial phase of the project has a CoM energy of 250 GeV for precision studies of the Higgs boson, a major goal in collider physics. As a linear machine it can operate at higher or lower energies. For example, the initial 21 km length can be extended to reach the threshold for the production of a top antitop quark pair
At 250 GeV, the primary enabling technology is Superconducting RF (SRF) cavities operating at an average gradient of 31.5 MV/m. The European XFEL in Hamburg Germany is a multi-billion dollar project that provides a 10%-scale demonstration of the ILC acceleration systems with over 750 SRF cavities operating at an average of 23 MV/m and producing a 17.5 GeV electron beam for the X-ray Free Electron laser (FEL). A Technical Design Report (TDR) for the ILC has been completed and the project is considered to be “shovel ready.” At this point, the R&D focus is on reducing the cost of the cryomodules. The remaining technical challenges are: improvement of the positron source, achieving the nanometer-scale spot size and stability at the interaction point (IP), and optimizing the damping ring injection and extraction systems.
2.1.2 Compact Linear Collider (CLIC)
The Compact Linear Collider (CLIC) is a multi-TeV high-luminosity linear e+e− collider proposed by an international collaboration led by CERN - see [7]. The design is based on a staged approach that includes three CoM energies of 380 GeV, 1.5 TeV and three TeV. In contrast to the ILC, CLIC uses a novel two-beam acceleration technique with normal-conducting accelerating structures operating in the range of 70 MV/m to 100 MV/m. A CDR was produced for CLIC in 2012 and, while the design is less mature than the ILC, the CLIC design parameters are well understood and have been reproduced in beam tests indicating that the CLIC performance goals are achievable.
The main risks and uncertainties for CLIC will be in scaling from the small-scale low-power demonstrations to the km-scale high-power two-beam deceleration systems. Efforts to reduce power consumption are ongoing and new estimates show a significant reduction related to improvements in the X-band RF technology and klystron design. Like the ILC, other R&D includes improvement of the positron source, achieving the nanometer-scale spot size and stability at the interaction point (IP), and optimizing the damping ring injection and extraction systems. In general, the spot sizes and stability requirements are tighter for CLIC than for the ILC while the positron system requirements may be easier due to a lower number of e+/sec and a higher macro-pulse repetition rate that eases requirements on a rotating target.
2.1.3 Future Circular e+e− Collider (FCC-ee)
The Future Circular Collider (FCC) is a proposed international collider complex located near Geneva Switzerland. It is based on the same successful staging strategy used for the Large Electron-Positron collider (LEP) and the Large Hadron Collider (LHC). The approximately 100 km tunnel would initially house the FCC-ee e+e− collider that would would offer a broad physics reach operating at four different CoM energies: the Z pole, WW threshold, the ZH production peak and the
The main technologies for the FCC-ee are well-developed. The technology R&D is focused on incremental improvements aimed mainly at further optimizing electrical efficiency, obtaining the required diagnostic precision, and achieving the target performance in terms of beam current and luminosity. Optimization is also desired to improve the performance of the positron source [14]. The greatest challenge facing the facility is the difficulty and cost of tunneling in the Geneva area that the ongoing Feasibility Study is aimed at addressing. The proposed schedule allows for the start of tunnel construction in the early 2030s with first collisions in the early 2040s.
2.1.4 Circular Electron Positron Collider (CepC)
Proposed by chinese scientists in 2012, the CepC is an international scientific project hosted by China to build a 240 GeV circular e+e− collider in an approximately 100 km tunnel - see [6]. Similar to the FCC, the tunnel for the CepC would eventually be used for a Super Proton-Proton Collider (SPPC). A CDR for the CepC was released in November 2018. The TDR is planned for completion at the end of 2022 followed by work on an Engineering Design Report (EDR) that will look at the detailed engineering design of components, site selection and preparations for industrialization.
Like the FCC-ee, the technological basis for the design is well-understood and the R&D focus is now on improving performance of the RF klystrons, SRF cavities, high precision magnets, and vacuum systems. The proposed schedule calls for first collisions in the mid-2030s.
2.1.5 Cool Copper Collider (C3)
The C3 is a linear collider concept based on recent innovations in the technology of cold copper cavities using distributed coupling that allows for increased accelerator performance and better optimization - see [15]. Operation at liquid nitrogen temperature substantially increases the RF efficiency and gradient of normal-conducting copper cavities. For a 250 GeV CoM energy the accelerator is 8 km and, with additional innovation, 550 GeV could be reached using the same footprint. A GeV-scale demonstration facility is proposed to provide input for a TDR. It would include three cryomodules operating at 70, 120 and 170 MeV/m to test the RF design. A gradient of 155 MeV/m would be needed for the 550 GeV upgrade. Other collider subsystems can be based on those developed for CLIC and ILC. Areas of technical focus are development of an ultra-low emittance polarized electron gun which would benefit all linear collider designs, optimization of the RF structures and reducing the cost of the RF sources.
2.1.6 Energy Recovery and Recirculating Linacs (ERLs and RLAs)
Energy Recovery Linacs (ERL) and Circular Colliders are an alternative approach to high energy electron-positron colliders with the aim to significantly reduce beam energy losses and consequently, power consumption. There are two proposed configurations. A circular e+e− Collider with two 100 km storage rings using Energy-Recovery Linacs (CERC) [16,17] or two large linear colliders with damping rings, Linear Energy Recovery Linac Collider (ReLiC) [18]. Starting as a Higgs factory they have the capability of achieving CoM energies up to 600 GeV. The energy as well as the particles are recycled in this scheme and make fully polarized electron and positron beams possible. A large fraction of the energy of the used beams is recovered by decelerating them. The beams are then reinjected into a damping ring where they are cooled and reused. Beam that is lost during the recovery process is replaced via a linear injector into the damping rings. An alternative concept for an SRF ERL-based linear e+e− collider has been recently proposed in [19].
ERL’s are an ongoing technological development. A number of compact ERL facilities and demonstrators have been constructed. The highest circulating Continuous Wave (CW) power was achieved in the IR FEL ERL at Jefferson Laboratory which operated with 8.5 mA at 150 MeV. A global development program for the ERL technology is discussed in [20].
2.1.7 X-Ray Free Electron Laser Compton Collider (XCC)
The XCC concept combines the cold copper distributed coupling technology of C3 with X-ray FELs [21] to create a γ-γ collider. The linac accelerates electron bunches with a gradient of 70 MeV/m until 31 GeV is reached, at which point alternate bunches are diverted to an X-ray FEL to produce circular polarized 1 keV X-rays using a helical undulator. The electron bunches remaining in the linac are accelerated to 62.8 GeV through a final focus system to the e−e− interaction points (IP). The 62.8 GeV electrons then collide with the focused X-ray laser light from the opposite X-ray FEL, producing 62.5 GeV photons with are then collided at 125 GeV CoM in the primary IP. The number of Higgs’ produced in such a machine would be comparable to the ILC, but backgrounds need to be studied in more detail.
Relative to some other e+e− Higgs factory proposals the XCC requires two additional beamlines and collision points and requires significant improvement to X-ray FEL technology. However, if a high brightness polarized RF gun can be developed, damping rings would not be required and the beam energy is half of that required for e+e− Higgs factories, raising the possibility of significant reduction in cost, a common obstacle for large-scale facilities. Gun development is challenging, but will benefit from experience with the LCLS-II-HE SRF gun when it turns on.
2.1.8 Muon Colliders
The muon collider has great potential to extend the energy of lepton colliders by taking advantage of the strong suppression of synchrotron radiation from muons relative to electrons, though the finite lifetime of the muon is a critical issue. This allows for efficient acceleration in rings and a more compact RF system - see Figure 2 - as well as a better defined collision energy. For example, the energy consumption of a 10 TeV CoM energy muon collider is estimated to be lower than CLIC at 3 TeV - see [5]. As a ring, a muon collider may be able to provide luminosity to two detectors.
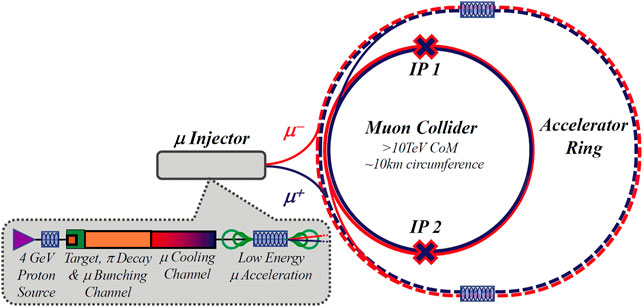
FIGURE 2. A conceptual scheme for the muon collider [22].
There are a number of technological challenges that need to be addressed to be able to take advantage of the large energy and luminosity reach. To this end, an International Muon Collider Collaboration (IMCC) was formed based on a recommendation in the update of the European Strategy for Particle Physics [22]. The initial focus is on 10 TeV with an integrated luminosity of 10 ab−1.
The main critical enabling technologies are 6D cooling of the muon beams, development of high field solenoids and accelerator magnets, very fast ramping magnets, high power targets, a proton driver, and operating with very strong collective effects. There has been recent progress in most of these areas demonstrating feasibility, but in the near term, a robust R&D program will be needed to bring the technology to the same level as linear or circular lepton colliders [20].
The scale of the R&D program can be expected to be a significant fraction of the final facility cost, much as for the linear collider program, where a large fraction of a B$ has been invested in dedicated international test facilities beyond the Stanford Linear Collider. Assuming sufficient funding to support a technically limited program and successful development of key technologies, it may be feasible to start colliding beams in the mid 2040s.
2.1.9 Fermilab Site Fillers
Domestically, the US is fully engaged in the Long Baseline Neutrino Facility (LBNF) and the Deep Underground Neutrino Experiment (DUNE). However, given the long time-frame needed to plan and build large accelerator facilities, preparation for major new projects needs to start soon. The Snowmass decadal strategic planning process for the US Particle Physics community (snowmass21.org) is an opportunity to consider possible options that could potentially be built in the US, and in particular, on the Fermilab site [23]. Given the current situation with the ILC and strong community support for a Higgs factory, it may be time to reconsider the option of hosting it in the US.
Other future collider concepts of intermediate-scale that might feasibly be built on the Fermilab site are also being considered:
• About 7 km long C3 (250 GeV up to 550 GeV);
• Linear colliders utilizing high gradient SRF, standing wave or travelling wave structures (250–500 GeV);
• 16 km circumference circular e+e− collider with (90–240 GeV);
• Same circumference proton-proton collider (24–27 TeV);
• A staged muon collider from a Higgs factory at 125 GeV up to 8–10 TeV.
Each of these options will require varying levels of R&D to produce a CDR by the time of the next Snowmass a decade from now. To accomplish this, it is proposed that the U.S. establish an integrated future colliders R&D program in the DOE Office of High Energy Physics (OHEP) to carry out feasibility studies and collaboratively engage in projects proposed abroad.
2.2 Hadron and Hadron/Lepton Colliders
2.2.1 Future Circular Collider - Hadron/Hadron (FCC-hh)
Proposed as a second phase of the FCC program after FCC-ee, the FCC-hh is a proton-proton collider aimed at increasing the physics reach by an order of magnitude beyond the LHC [24]. Following the same strategy used for LEP and LHC, the accelerators will utilize the same 100 km-scale tunnel. It is one of the relatively mature proposals, and a conceptual design report was published in 2019 [10]. One of the major enabling technologies are the 16 T superconducting magnets required to reach the target energy of 100 TeV. This field level does not currently exist but programs in the US and EU/CERN are actively pursuing the R&D. Experience from the construction of high gradient quadrupoles for the LHC high luminosity (HL-LHC) upgrade will serve as a launching point for further development.
Attaining the desired luminosity of 30⋅1034 cm−2s−1 will be challenging. However, the HL-LHC will be an opportunity to gain considerable experience. Other challenges are related to the size and number of components, and increased number of injector rings, adding considerably to the overall complexity. Crab cavities, necessary for compensating the crossing angle at the interaction points, are still an untested system in hadron colliders, but the HL-LHC will provide an opportunity to develop this technology.
2.2.2 Super Proton-Proton Collider (SPPC)
The SPPC is proposed as the second phase of the CepC-SPPC, sharing the same tunnel in a scheme parallel to the FCC-ee/hh [25]. It is planned to operate at a CoM energy of up to 125 TeV in the final stage using 20T magnets with an intermediate stage at 75 TeV using 12T magnets.
High-field magnets are the key enabling technology for future hadron colliders. A unique feature of the magnet technology is the proposed use of iron-based high temperature superconductor (HTS). This material is still in the R&D stage, but if successful, has the potential for significant cost savings over currently available HTS materials. The project is currently in the pre-CDR phase. In addition to the high field magnets, synchrotron radiation power, luminosity and site power are a consideration in the overall design [26].
3 High Intensity Accelerators for Neutrino Research and Rare Processes
The leading accelerator-based facilities for high energy neutrino research are superbeams based on a conventional beam dump technique: an intense high energy proton beam is directed onto a thick nuclear target producing mostly pions and kaons, that are captured by focusing magnetic horns in order to obtain a well directed beam of same charge secondaries. High-energy neutrino beams are products of the decays of charged pions and kaons in a long decay channel [3]. Superbeams sources operate with proton beam intensities close to the mechanical stability limit of the primary targets which is at present O (1 MW). The most powerful accelerators for neutrino research to date are the rapid cycling synchrotron facility J-PARC in Japan which has reached 515 kW of 30 GeV proton beam power and the Fermilab Main Injector delivering up to 862 kW of 120 GeV protons on target. These facilities support neutrino oscillations research programs at the SuperK experiment (295 km from J-PARC) and MINOS (810 km from Fermilab), correspondingly.
The needs of neutrino physics call for the next generation, higher-power, megawatt and multi-MW-class superbeams facilities. Average proton beam power on the neutrino target scales with the beam energy Eb, number of particle per pulse Nppp and cycle time Tcycle as Pb = (EbNppp)/Tcycle. Corresponding upgrades of the RF system and magnet power supply ramping rate 1/Tcycle have been initiated at J-PARC, while Fermilab has started construction of an 800 MeV SRF H- PIP-II linac (Proton Improvement Plan-II) that will help to boost Nppp and the Main Injector beam power to above 1.2 MW, and considers further facility upgrades to get to 2.4 MW - see Figure 3.
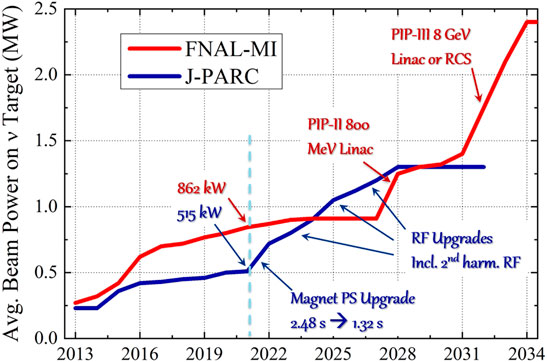
FIGURE 3. Beam power progress and plans for J-PARC and Fermilab Main Injector - two leading superbeam facilities for neutrino research (from [3]).
Many existing and planned high intensity accelerators can be effectively used for fixed target experiments complementary to high-energy-Frontier colliders [27]. For example, a recent CERN study of the Physics Beyond Colliders (PBC) [28] resulted in numerous accelerator-based proposals, ranging from a gamma-factories [29] to explorations of the dark sector, to precision measurements of either strongly interacting processes or light, feebly interacting particles at beam dump facilities, such as, e.g., the SHiP experiment [30] at the SPS North Area [31]. A similar study is underway in the US as part of the Snowmass process. It considers a variety of potentially available beams ranging from 800 MeV to 120 GeV protons [32–34] to multi-GeV electrons [35].
4 Accelerator Technologies and Advanced Accelerators
The cost of large accelerators is set by the scale (energy, length, power) and technology. Typically, accelerator components (Normal Conducting (NC) or/and Superconducting (SC) magnets and RF systems) account for 50 ± 10% of the total cost, while the civil construction takes 35 ± 15%, and power production, delivery and distribution technology adds the remaining 15 ± 10% [36]. While the last two parts are mostly determined by industry, the magnet and RF technologies are linchpins in the progress of accelerators.
4.1 Superconducting Magnet R&D
Superconducting magnets are required for many of the proposed facilities, in some cases with operational parameters well beyond current state-of-the-art, such as muon colliders or next generation high energy hadron colliders. This need was recognized by the last P5 process and the US Department of Energy-Office of High Energy Physics initiated the US Magnet Development Program (MDP) [37,38], a general R&D program that pulls together US HEP magnet research groups at DOE laboratories and Universities under a common collaboration, with focused mission and goals. Another similar collaborative effort, the High Field Magnet (HFM) program has recently been organized in the EU [39]. Superconducting Nb-Ti magnets needed for colliders operate with fields up to 8.3 T (in the LHC). Dipole fields in excess of 16 T (up to 24 T) are needed, and solenoids, primarily for the muon collider, up to 50 T [40]. A recently tested US MDP prototype Nb3Sn magnet has reached 14.5 T [41] - see Figure 4. Fields above 16 T require the use of high temperature superconductors (HTS); Bi-2212, REBCO and Fe-based either in lieu of or combined in a hybrid scheme with Nb3Sn. The fastest rapid cycling magnets (required for a muon collider) show up to 300 T/s ramping rates in the HTS-based superconducting magnet test at Fermilab [42].
4.2 RF Technology R&D
The highest gradient large-scale NC RF system is the 28 MV/m linac of the SwissFEL at the PSI (Switzerland). Up to 100 MV/m accelerating beam gradients were achieved in the CLIC 12 GHz structures at the CERN test facility, while up to 150 MV/m gradients were reported in the first test of short 11.4 GHz NC structures cooled to 77 K at SLAC [15] - Figure 5. As for the SC RF, the largest scale accelerator to date is the 17.5 GeV 1.3 GHz pulsed linac of the European XFEL at DESY that has achieved an average beam gradient of roughly 23 MV/m with a nominal Superconducting RF (SRF) cavity quality factor of Q0 ≃ 1.4 ⋅ 1010 at 2 K. The full ILC specification on the beam acceleration gradient of 31.5 MV/m has been demonstrated at the FNAL FAST facility [43]. Recent advances in SRF cavity processing such as nitrogen doping allow further improvement of the quality factor to (3–6) ⋅ 1010 (hence, reducing the required cryogenic cooling power) and an initial cryomodule for the LCLS-II-HE, operated at 25 MV/m CM with a Q0 = 3 ⋅ 1010. Further R&D aims for 50 MV/m gradients in 1.3 GHz structures [44]. An active ongoing accelerator R&D program for future multi-MW proton beams includes development of more efficient power supplies with capacitive energy storage and recovery, more economical RF power sources such as 80% efficient klystrons, magnetrons, and solid-state RF sources (compared to current ∼ 55%) see for example [45,46].
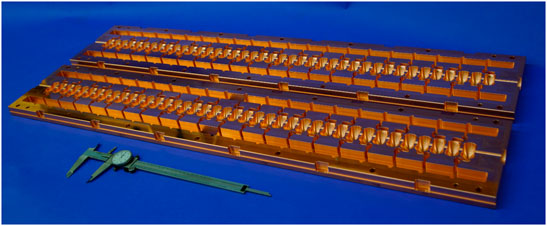
FIGURE 5. Both halves of the C3 prototype structure prior to braze. The 1 m structure consists of 40 cavities. A rf manifold that runs parallel to the structure feeds 20 cavities on each side. The structure operates at 5.712 GHz - from [45].
4.3 Targets and Sources R&D
The next generation of high power targets for future accelerators will use more complex geometries, novel materials, and new concepts (like flowing granular materials, Ammigan et al. [47]). Under active development are advanced numerical approaches that satisfy the physical design requirements of reliable beam targets [48]. In parallel, there is work to be done on radiation hardened beam instrumentation [49], development of irradiation methods for high power targets and irradiation facilities [50].
There are challenges in high-intensity high-brightness beam sources for future accelerators. They are particularly formidable for sources of beams with special characteristics, such as polarized electrons and ions or ultra-small emittances, and for tertiary and secondary particles, such as muons and positrons. High intensity positron sources, which are critical for future e+e− linear colliders, may be simplified using advanced accelerator concepts and photon-driven schemes that can potentially outperform conventional e+ production techniques [51].
4.4 Advanced Acceleration Methods
4.4.1 Acceleration in Plasma
Electric fields due to charge separation in plasma can sustain
Plasma waves can be effectively excited by short and powerful external drivers. Three effective ways to excite plasma have been demonstrated in the past 2 decades: 1) by intense electron bunches (9 GeV acceleration over 1.3 m at 1017cm−3) at the SLAC FACET facility [53]; 2) short laser pulses resulted in an electron beam energy gain of up to 7.8 GeV over 20 cm in a few 1017cm−3 plasma at the LBNL BELLA facility [54]; and 3) by self-modulated high energy proton bunches (2 GeV over 10 m at 1015cm−3) at the CERN AWAKE experiment [55]. In principle, plasma-based linear accelerators can be employed in high energy e+e− colliders, but a significant long-term R&D effort is required to address many critical issues, such as acceleration of positrons, beamstrahlung, staging of multiple plasma acceleration cells, power efficiency, emittance control, jitter and scattering in plasma, etc [2,20].
There are three remarkable recent developments on the way to practical plasma-based beam accelerators: 1) EuPRAXIA—a 569 MEuro European plasma accelerator project proposal, supported by 50 institutions from 15 countries, and aiming for 5 GeV electron beam acceleration and development of plasma-based FELs—has been included in the ESRFI 10–20 year roadmap [56]; 2) the laser wakefield accelerator at SIOM/CAS in Shanghai (China) has achieved an outstanding quality of the accelerated 0.5 GeV electron beam (produced by a 200 TW laser exciting plasma in a 6 mm He gas jet) sufficient for initiation of the FEL generation of 27 nm light in the downstream undulators—making it the first demonstration of the plasma-based FEL [57]; and 3) a similar free electron lasing demonstration at the LNF-INFN with a beam-driven plasma accelerator [58].
4.4.2 Acceleration in Structures
Relativistic electrons or positrons passing near a material boundary produce wakefields when the particle velocity exceeds the Cherenkov radiation condition. The longitudinal wakes can be used to accelerate an appropriately phased trailing beam [59] or can be extracted by a high efficiency RF coupler as a high power RF source [60]. There has been significant progress on another approach that utilizes laser-driven microstructure accelerators [61].
Structure wakefield acceleration (SWFA) has an advantage for application to linear colliders because the structures naturally accelerate electrons and positrons and are expected to have smaller challenges preserving the beam emittance than plasma accelerators. Recent progress in development has increased the gradient to 300 MV/m and wakefield power generation to 500 MW [62]. The next proposed steps are to design, construct and test a laboratory-scale SWFA module and incorporate it into a SWFA-based facility design with the intent of implementing a dedicated test facility as a scalable demonstration of the technology aimed at an energy Frontier machine.
5 Beam Physics Advances
Accelerator and beam physics (ABP), the science of charged particle beams, is an essential aspect of designing and building the next Frontier accelerators. A robust and scientifically challenging program in accelerator and beam physics is critical for the field of particle physics to be productive and successful. Major ABP challenges aim at improving the reliability, performance, safety, and cost reduction of future accelerators and push the envelope of beam intensity, beam quality, beam measurement and control, and development of methods to model and predict beam behavior.
There are many notable recent developments in the physics of beams - see. e.g. [2]. Here we will present those in the fields of beam cooling and artificial intelligence and machine learning (AI/ML) and then summarize other developments across the field.
5.1 Beam Cooling
Beam cooling refers to the process of increasing the beam phase space density (ideally, 6D and loss-less) or, equivalently, beam emittance reduction. Widely used methods include synchrotron radiation damping, electron, stochastic and laser cooling. Over the past few years, we have seen several novel cooling schemes experimentally demonstrated at operational accelerators. A true breakthrough was the demonstration of the ionization cooling of 140 MeV/c muons at the MICE experiment at RAL (United Kingdom)—some 10% beam emittance reduction was observed in a single pass through the cooling section [63]. In 2020, “bunched” electron beam cooling of ions in RHIC (γ ∼ 5)—remarkable by the pioneering use of high quality bunched electron beams from an electron beam RF photoinjector gun (before, only DC electron accelerators were used with limited capability to get to very high energies)—was demonstrated at BNL [64]. Earlier this year another outstanding result was reported by the Fermilab team which has successfully carried out a proof-of-principle experiment on the optical stochastic cooling of 100 MeV electrons in the IOTA ring in which the use of undulator magnets - instead of electrostatic pickups in traditional stochastic cooling setups - allowed to expand the feedback system bandwidth by several orders of magnitude to a THz range [65]. Finally, the proof-of-principle tests of a novel coherent electron cooling of the record-high energy 26 GeV/u ions have started this year at BNL [66].
5.2 Artificial Intelligence and Machine Learning for Accelerators
Efforts have been ramping-up in recent years to use Artificial Intelligence and Machine Learning (AI/ML) to enhance the performance of accelerators and beamlines [67,68]. There is significant promise of applications of AI/ML in beam diagnostics, controls, and modeling [69]. Opportunity exists in broadening AI/ML methods for early detection of a broad range of accelerator component or subsystem failures [70] and for optimization of advanced numerical simulations through identification of the most promising combinations of parameters thereby reducing the total number of required simulations ([71]).
5.3 Other Beam Physics
A recent community exercise summed up the needs and directions of future developments in ABP [72]. Among many, those include issues related to beam loss control in high-intensity high-power accelerators (space-charge effects, instabilities, collimation, electron lens compensation, integrable optics, etc) which require innovative approaches, theoretical and experimental studies (at, e.g., the IOTA ring [73], and operational accelerators in the US and abroad) and validated computer models/codes. A key challenge would be to reduce particle losses (dN/N) at a faster rate than increases in achieved beam intensity (power) (N) [3].
Future circular and linear e+e− colliders (FCC-ee, CepC, gamma-gamma Higgs factory) require collision optimization studies including 3D beam size flip-flop from the beam-beam effect, polarization and Interaction Region (IR) design; pico-meter vertical emittance preservation techniques in high-charge circular colliders with strong focusing IR, detector solenoids, and beam-beam effects; end-to-end emittance preservation simulations for linear colliders should be augmented with experimental tests of the beam-based alignment techniques in the presence of realistic external noise sources; plasma-lens-based final focus and beam transport system design [74,75].
Required ABP explorations for future hadron colliders include efficient collimation techniques [76], electron lenses for Landau damping and collimation [77], dynamic aperture optimization methods to make possible new integrable optics solutions [78], and studies to obtain lower emittances from new particle sources for injecting beams in high-bunch-charge colliders.
6 Summary
Accelerator science and technology has advanced significantly in the decade since the last Snowmass/Particle Physics Project Prioritization Panel (P5) strategic planning process. Advances in super-conducting and normal-conducting RF cavities, high field magnets, particle sources, targets, and advanced acceleration technologies as well as advances in the modeling and understanding of beam physics have allowed the development of many new or improved proposals for future accelerators to advance particle physics. Current activities such as the High Luminosity LHC upgrade, SuperKEKB, PIP-II, the ESS, the EIC and FAIR also serve as platforms for these future accelerators.
Thus, the ongoing Snowmass and P5 strategic planning process will have the opportunity and challenge of a variety of proposals to consider in developing a strategy for the future of particle physics. These proposals vary widely in terms of physics potential, cost, size, maturity and executable time-frame. However, with the support of a strong international accelerator and particle physics community, we are confident that there are multiple paths that will deliver a multi-decadal program in accelerator-based particle physics.
Author Contributions
All authors listed have made a substantial, direct, and intellectual contribution to the work and approved it for publication.
Funding
This manuscript has been authored with support from the Department of Energy under Contract No. DE-AC02-07CH11359 with the Fermi Research Alliance, LLC and Contract No. DE-AC0276SF00515 with the SLAC National Accelerator Laboratory.
Conflict of Interest
The authors declare that the research was conducted in the absence of any commercial or financial relationships that could be construed as a potential conflict of interest.
Publisher’s Note
All claims expressed in this article are solely those of the authors and do not necessarily represent those of their affiliated organizations, or those of the publisher, the editors and the reviewers. Any product that may be evaluated in this article, or claim that may be made by its manufacturer, is not guaranteed or endorsed by the publisher.
Acknowledgments
We greatly appreciate discussions with Pushpa Bhat (FNAL), Dmitri Denisov (BNL), and Meenakshi Narain (Brown University) within the framework of the APS Snowmass’21 HEP planning process.
References
1. Shiltsev V. Particle Beams behind Physics Discoveries. Phys Today (2020) 73. doi:10.1063/pt.3.4452
2. Shiltsev V, Zimmermann F. Modern and Future Colliders. Rev Mod Phys (2021) 93:015006. doi:10.1103/revmodphys.93.015006
3. Shiltsev V. Superbeams and Neutrino Factories - Two Paths to Intense Accelerator-Based Neutrino Beams. Mod Phys Lett A (2020) 35:2030005. doi:10.1142/s0217732320300050
4. Benedikt M, Zimmermann F. The Physics and Technology of the Future Circular Collider. Nat Rev Phys (2019) 1:238–40. doi:10.1038/s42254-019-0048-0
5. Long KR, Lucchesi D, Palmer MA, Pastrone N, Schulte D, Shiltsev V. Muon Colliders to Expand Frontiers of Particle Physics. Nat Phys (2021) 17:289–92. doi:10.1038/s41567-020-01130-x
6. Lou X. The Circular Electron Positron Collider. Nat Rev Phys (2019) 1:232–4. doi:10.1038/s42254-019-0047-1
7. Stapnes S. The Compact Linear Collider. Nat Rev Phys (2019) 1:235–7. doi:10.1038/s42254-019-0051-5
8. Michizono S. The International Linear Collider. Nat Rev Phys (2019) 1:244–5. doi:10.1038/s42254-019-0044-4
9.The FCC Collaboration. FCC Physics Opportunities: Future Circular Collider Conceptual Design Report Volume 1. Eur Phys J C (2019) 79.
12.CERN Council. Organisational Structure of the FCC Feasibility Study. In: Restricted CERN Council - Two-Hundred-And-Third Session (2021). CERN/SPC/1155/Rev.2.
13.CERN Council. Main Deliverables and Timeline of the FCC Feasibility Study. In: Restricted CERN Council - Two-Hundred-And-Third Session (2021). CERN/SPC/1161.
14. Agapov I, Benedikt M, Blondel A, Boscolo M, Brunner O, Chamizo Llatas M, et al. Future Circular Lepton Collider FCC-Ee: Overview and Status. arXiv:2203.08310 [physics.acc-ph] (2022).
15. Bai M, Barklow T, Bartoldus R, Breidenbach M, Grenier P, Huang Z, et al. C3: A “Cool” Route to the Higgs Boson and beyond. arXiv preprint arXiv:2110.15800 (2021).
16. Litvinenko VN, Roser T, Chamizo-Llatas M. High-energy high-luminosity e+e− collider using energy-recovery linacs. Phys Lett B (2020) 804:135394. doi:10.1016/j.physletb.2020.135394
17. Litvinenko VN, Bachhawat N, Chamizo-Llatas M, Meot F, Roser T. CERC - circular e+e− collider using Energy-Recovery Linac. arXiv preprint arXiv:2203.07358 (2022).
18. Litvinenko VN, Bachhawat N, Chamizo-Llatas M, Jing Y, Meot F, Petrushina I, et al. The ReLiC: Recycling linear e+e− collider. arXiv preprint arXiv:2203.06476 (2022).
19. Telnov VI. A high-luminosity superconducting twin e+e- linear collider with energy recovery. J Inst (2021) 16:P12025. doi:10.1088/1748-0221/16/12/p12025
20. Adolphsen C, Angal-Kalinin D, Arndt T, Arnold M, Assmann R, Auchmann B, et al. European Strategy for Particle Physics Accelerator R&D Roadmap. Tech Rep (2022). CERN-2022-001.
21. Barklow T, Dong S, Emma C, Duris J, Huang Z, Naji A, et al. XCC: An X-ray FEL-Based γγ Collider Higgs Factory. arXiv preprint arXiv:2203.08484 (2022).
22.European Strategy Group. 2020 Update of the European Strategy for Particle Physics (Brochure) (2020). CERN-ESU-015.
23. Bhat P, Jindariani S, Ambrosio G, Apollinari G, Belomestnykh S, Bross A, et al. Future Collider Options for the US. arXiv preprint arXiv:2203.08088 (2022).
24. Benedikt M, Chance A, Dalena B, Denisov D, Giovannozzi M, Gutleber J, et al. Future Circular Hadron Collider FCC-Hh: Overview and Status. arXiv preprint arXiv:2203.07804 (2022).
25. Tang J, Zhang Y, Xu Q, Gao J, Lou X, Wang Y. Snowmass 2021 white Paper AF4-SPPC. arXiv preprint arXiv:2203.07987 (2022).
26. Tang J. Design Concept for a Future Super Proton-Proton Collider. Front Phys (2022) 2022:138. doi:10.3389/fphy.2022.828878
27. Jaeckel J, Lamont M, Vallée C. The Quest for New Physics with the Physics beyond Colliders Programme. Nat Phys (2020) 16:393–401. doi:10.1038/s41567-020-0838-4
28. Beacham J, Burrage C, Curtin D, De Roeck A, Evans J, Feng JL, et al. Physics beyond Colliders at CERN: beyond the Standard Model Working Group Report. J Phys G: Nucl Part Phys (2019) 47:010501. doi:10.1088/1361-6471/ab4cd2
29. Budker D, Berengut JC, Flambaum VV, Gorchtein M, Jin J, Karbstein F, et al. Expanding Nuclear Physics Horizons with the Gamma Factory. Annalen der Physik (2022) 534:2100284. doi:10.1002/andp.202100284
30. Ahdida C, Albanese R, Alexandrov A, Anokhina A, Aoki S, Arduini G, et al. Sensitivity of the SHiP experiment to Heavy Neutral Leptons. J High Energ Phys (2019) 2019:1–20.
31. Ahdida C, Alia R, Arduini G, Arnalich A, Avigni P, Bardou F, et al. SPS Beam Dump Facility–Comprehensive Design Study. arXiv preprint arXiv:1912.06356 (2019).
32. Pellico W, Bhat C, Eldred J, Johnstone C, Johnstone J, Seiya K, et al. FNAL PIP-II Accumulator Ring. arXiv preprint arXiv:2203.07339 (2022).
33. Toups M, Van de Water R, Batell B, Brice S, deNiverville P, Eldred J, et al. SBN-BD: O(10 GeV) Proton Beam Dump at Fermilab’s PIP-II Linac. arXiv preprint arXiv:2203.08102 (2022).
34. Apyan A, Batell B, Berlin A, Blinov N, Chaharom C, Cuadra S, et al. DarkQuest: A Dark Sector Upgrade to SpinQuest at the 120 GeV Fermilab Main Injector. arXiv preprint arXiv:2203.08322 (2022).
35. Akesson T, Blinov N, Brand-Baugher L, Bravo C, Bryngemark LK, Butti P, et al. Current Status and Future Prospects for the Light Dark Matter eXperiment. arXiv preprint arXiv:2203.08192 (2022).
36. Shiltsev V. A Phenomenological Cost Model for High Energy Particle Accelerators. J Inst (2014) 9:T07002. doi:10.1088/1748-0221/9/07/t07002
37. Gourlay SA, Prestemon SO, Zlobin AV, Cooley L, Larbalestier D. The US Magnet Development Program Plan. In: Tech. Rep. Berkeley, CA, USA: Lawrence Berkeley National Lab.(LBNL (2016).
38. Ambrosio G, Amm K, Anerella M, Apollinari G, Arbelaez D, Auchmann B, et al. A Strategic Approach to advance Magnet Technology for Next Generation Colliders. arXiv preprint arXiv:2203.13985 (2022).
39. Védrine P, Garcia-Tabarès L, Auchmann B, Ballarino A, Baudouy B, Bottura L, et al. High Field Magnet Development for HEP in Europe: a Proposal from LDG HFM Expert Panel. arXiv preprint arXiv:2203.08054 (2022).
40. Alexahin YI, Barzi E, Gianfelice-Wendt E, Kapin V, Kashikhin V, Mokhov N, et al. Critical Problems of Energy Frontier Muon Colliders: Optics, Magnets and Radiation. arXiv preprint arXiv:2203.10431 (2022).
41. Zlobin A, Turrioni D, Krave S, Stoynev S, Caspi S, Carmichael J, et al. Reassembly and Test of High-Field Nb3 Sn Dipole Demonstrator MDPCT1. IEEE Trans Appl Supercond (2021) 31:4000506.
42. Piekarz H, Claypool B, Hays S, Kufer M, Shiltsev V, Zlobin A, et al. Fast Cycling HTS Based Superconducting Accelerator Magnets: Feasibility Study and Readiness Demonstration Program Driven by Neutrino Physics and Muon Collider Needs. arXiv preprint arXiv:2203.06253 (2022).
43. Broemmelsiek D, Chase B, Edstrom D, Harms E, Leibfritz J, Nagaitsev S, et al. Record High-Gradient SRF Beam Acceleration at Fermilab. New J Phys (2018) 20:113018. doi:10.1088/1367-2630/aaec57
44. Grassellino A, Romanenko A, Trenikhina Y, Checchin M, Martinello M, Melnychuk OS, et al. Unprecedented Quality Factors at Accelerating Gradients up to 45 MVm−1in Niobium Superconducting Resonators via Low Temperature Nitrogen Infusion. Supercond Sci Technol (2017) 30:094004. doi:10.1088/1361-6668/aa7afe
45. Nanni EA, Breidenbach M, Vernieri C, Belomestnykh S, Bhat P, Nagaitsev S, et al. C3 Demonstration Research and Development Plan. arXiv preprint arXiv:2203.09076 (2022).
46. Ives RL, Read M, Bui T, Marsden D, Collins G, Chase B, et al. High Efficiency, Low Cost, RF Sources for Accelerators and Colliders. arXiv preprint arXiv:2203.12043 (2022).
47. Ammigan K, Bidhar S, Pellemoine F, Pronskikh V, Pushka D, Yonehara K, et al. Novel Materials and Concepts for Next-Generation High Power Target Applications. arXiv preprint arXiv:2203.08357 (2022).
48. Barbier C, Bidhar S, Calviani M, Dooling J, Gao J, Jacques A, et al. Modeling Needs for High Power Targets. arXiv preprint arXiv:2203.04714 (2022).
49. Yonehara K. Radiation Hardened Beam Instrumentations for Multi-Mega-Watt Beam Facilities. arXiv preprint arXiv:2203.06024 (2022).
50. Pellemoine F, Barbier C, Sun Y, Ammigan K, Bidhar S, Zwaska B, et al. Irradiation Facilities and Irradiation Methods for High Power Targets. arXiv preprint arXiv:2203.08239 (2022).
51. Chaikovska I, Chehab R, Kubytskyi V, Ogur S, Ushakov A, Variola A, et al. Positron Sources: from Conventional to Advanced Accelerator Concepts-Based Colliders. arXiv preprint arXiv:2202.04939 (2022). doi:10.1088/1748-0221/17/05/p05015
53. Litos M, Adli E, Allen JM, An W, Clarke CI, Corde S, et al. 9 GeV Energy Gain in a Beam-Driven Plasma wakefield Accelerator. Plasma Phys Control Fusion (2016) 58:034017. doi:10.1088/0741-3335/58/3/034017
54. Gonsalves AJ, Nakamura K, Daniels J, Benedetti C, Pieronek C, De Raadt TCH, et al. Petawatt Laser Guiding and Electron Beam Acceleration to 8 GeV in a Laser-Heated Capillary Discharge Waveguide. Phys Rev Lett (2019) 122:084801. doi:10.1103/PhysRevLett.122.084801
55. Adli E, Ahuja A, Apsimon O, Apsimon R, Bachmann A-M, Barrientos D, et al. Acceleration of Electrons in the Plasma wakefield of a Proton bunch. Nature (2018) 561:363–7. doi:10.1038/s41586-018-0485-4
56. Assmann R, Weikum M, Akhter T, Alesini D, Alexandrova A, Anania M, et al. Eupraxia Conceptual Design Report. Eur Phys J Spec Top (2020) 229:3675–4284.
57. Wang W, Feng K, Ke L, Yu C, Xu Y, Qi R, et al. Free-electron Lasing at 27 Nanometres Based on a Laser wakefield Accelerator. Nature (2021) 595:516–20. doi:10.1038/s41586-021-03678-x
58. Shpakov V, Anania M, Behtouei M, Bellaveglia M, Biagioni A, Cesarini M, et al. First Emittance Measurement of the Beam-Driven Plasma wakefield Accelerated Electron Beam. Phys Rev Acc Beams (2021) 24:051301. doi:10.1103/physrevaccelbeams.24.051301
59. Gai W, Schoessow P, Cole B, Konecny R, Norem J, Rosenzweig J, et al. Experimental Demonstration of Wake-Field Effects in Dielectric Structures. Phys Rev Lett (1988) 61:2756–8. doi:10.1103/physrevlett.61.2756
60. Gao F, Conde M, Gai W, Jing C, Konecny R, Liu W, et al. Design and Testing of a 7.8 GHz Power Extractor Using a Cylindrical Dielectric-Loaded Waveguide. Phys Rev Spec Topics-Accelerators Beams (2008) 11:041301. doi:10.1103/physrevstab.11.041301
61. England R, Filippetto D, Torrisi G, Bacci A, Della Valle G, Mascali D, et al. Laser-driven Structure-Based Accelerators. arXiv preprint arXiv:2203.08981 (2022).
62. Jing C, Power J, Shao J, Ha G, Piot P, Lu X, et al. Continuous and Coordinated Efforts of Structure wakefield Acceleration (SWFA) Development for an Energy Frontier Machine. arXiv preprint arXiv:2203.08275 (2022).
63.MICE collaboration. Demonstration of Cooling by the Muon Ionization Cooling experiment. Nature (2020) 578:53–9. doi:10.1038/s41586-020-1958-9
64. Fedotov AV, Altinbas Z, Belomestnykh S, Ben-Zvi I, Blaskiewicz M, Brennan M, et al. Experimental Demonstration of Hadron Beam Cooling Using Radio-Frequency Accelerated Electron Bunches. Phys Rev Lett (2020) 124:084801. doi:10.1103/PhysRevLett.124.084801
65. Jarvis J, Lebedev V, Romanov A, Broemmelsiek D, Carlson K, Chattopadhyay S, et al. First Experimental Demonstration of Optical Stochastic Cooling. arXiv preprint arXiv:2203.08899 (2022).
66. Wang G, Litvinenko V, Pinayev I, Jing Y, Kayran D, Ma J, et al. Summary of the CeC experiment in RHIC Run 21 [(No. CA/AP/656; BNL-222354-2021-TECH]. In: Tech. Rep. Upton, NY, USA: Brookhaven National Lab.(BNL (2021).
67. Duris J, Kennedy D, Hanuka A, Shtalenkova J, Edelen A, Baxevanis P, et al. Bayesian Optimization of a Free-Electron Laser. Phys Rev Lett (2020) 124:124801. doi:10.1103/physrevlett.124.124801
68. Arpaia P, Azzopardi G, Blanc F, Bregliozzi G, Buffat X, Coyle L, et al. Machine Learning for Beam Dynamics Studies at the CERN Large Hadron Collider. Nucl Instr Methods Phys Res Section A: Acc Spectrometers, Detectors Associated Equipment (2021) 985:164652. doi:10.1016/j.nima.2020.164652
69. Edelen AL, Biedron SG, Chase BE, Edstrom D, Milton SV, Stabile P. Neural Networks for Modeling and Control of Particle Accelerators. IEEE Trans Nucl Sci (2016) 63:878–97. doi:10.1109/TNS.2016.2543203
70. Edelen A, Biedron S, Milton S, Edelen J. First Steps toward Incorporating Image Based Diagnostics into Particle Accelerator Control Systems Using Convolutional Neural Networks. arXiv preprint arXiv:1612.05662 (2016).
71. Koser D, Waites L, Winklehner D, Frey M, Adelmann A, Conrad J. Input Beam Matching and Beam Dynamics Design Optimization of the IsoDAR RFQ Using Statistical and Machine Learning Techniques. arXiv preprint arXiv:2112.02579 (2021).
72. Nagaitsev S, Huang Z, Power J, Vay J-L, Piot P, Spentzouris L, et al. Accelerator and Beam Physics Research Goals and Opportunities. arXiv preprint arXiv:2101.04107 (2021). doi:10.2172/1764152
73. Antipov S, Broemmelsiek D, Bruhwiler D, Edstrom D, Harms E, Lebedev V, et al. IOTA (Integrable Optics Test Accelerator): Facility and Experimental Beam Physics Program. J Inst (2017) 12:T03002. doi:10.1088/1748-0221/12/03/t03002
74. Ariniello R, Doss C, Hunt-Stone K, Cary J, Litos M. Transverse Beam Dynamics in a Plasma Density Ramp. Phys Rev Acc Beams (2019) 22:041304. doi:10.1103/physrevaccelbeams.22.041304
76. Scandale W, Taratin AM. Channeling and Volume Reflection of High-Energy Charged Particles in Short Bent Crystals. Crystal Assisted Collimation of the Accelerator Beam Halo. Phys Rep (2019) 815:1–107. doi:10.1016/j.physrep.2019.04.003
77. Shiltsev V. Electron Lenses: Historical Overview and Outlook. J Inst (2021) 16:P03039. doi:10.1088/1748-0221/16/03/p03039
Keywords: accelerators, colliders, beam physics, magnets, particle sources, RF acceleration, plasma acceleration
Citation: Gourlay S, Raubenheimer T and Shiltsev V (2022) Challenges of Future Accelerators for Particle Physics Research. Front. Phys. 10:920520. doi: 10.3389/fphy.2022.920520
Received: 14 April 2022; Accepted: 27 May 2022;
Published: 27 June 2022.
Edited by:
Patrick Le Du, Commissariat à l'Energie Atomique et aux Energies Alternatives (CEA), FranceReviewed by:
Michelangelo Mangano, European Organization for Nuclear Research (CERN), SwitzerlandMarco Incagli, Istituto Nazionale di Fisica Nucleare—sezione di Pisa, Italy
Copyright © 2022 Gourlay, Raubenheimer and Shiltsev. This is an open-access article distributed under the terms of the Creative Commons Attribution License (CC BY). The use, distribution or reproduction in other forums is permitted, provided the original author(s) and the copyright owner(s) are credited and that the original publication in this journal is cited, in accordance with accepted academic practice. No use, distribution or reproduction is permitted which does not comply with these terms.
*Correspondence: Stephen Gourlay, c2Fnb3VybGF5QGxibC5nb3Y=