- 1Institute of Infection, Immunology, and Tumor Microenvironment, School of Medicine, Wuhan University of Science and Technology, Wuhan, China
- 2Department of Anatomy, School of Medicine, Wuhan University of Science and Technology, Wuhan, China
Malignant tumors are a category of diseases that possess invasive and metastatic capabilities, with global incidence and mortality rates remaining high. In recent years, the pivotal role of fibrosis in tumor progression, drug resistance, and immune evasion has increasingly been acknowledged. Fibrosis enhances the proliferation, migration, and invasion of tumor cells by modifying the composition and structure of the extracellular matrix, thereby offering protection for immune evasion by tumor cells. The activation of cancer-associated fibroblasts (CAFs) plays a significant role in this process, as they further exacerbate the malignant traits of tumors by secreting a variety of cytokines and growth factors. Anti-fibrotic tumor treatment strategies, including the use of anti-fibrotic drugs and inhibition of fibrosis-related signaling pathways such as Transforming Growth Factor-β (TGF-β), have demonstrated potential in delaying tumor progression and improving the effectiveness of chemotherapy, targeted therapy, and immunotherapy. In the future, by developing novel drugs that target the fibrotic microenvironment, new therapeutic options may be available for patients with various refractory tumors.
1 Introduction
Malignant tumors are a category of abnormal cellular proliferation diseases characterized by invasiveness and metastatic potential. In 2022, it was estimated that there were 20 million new cases and 9.7 million deaths worldwide (Bray et al., 2024). Although cancer treatment methods, such as surgery, radiotherapy, chemotherapy, targeted therapy, and immunotherapy, have continuously advanced, the complexity and heterogeneity of the disease make it challenging to cure, posing a significant global public health problem (Hirsch et al., 2017). Hanahan stated that the progression of tumors involves more than just an increase in tumor cell numbers and must be understood within the framework of the “tumor microenvironment (TME).” (Hanahan and Weinberg, 2011) TME is a complex system composed of tumor cells, stromal cells, immune cells, blood vessels, and the extracellular matrix (ECM). A growing body of research indicates that the TME is vital in the growth, invasion, metastasis, and treatment resistance of multiple tumors. Additionally, tumor cells can secrete cytokines and growth factors, inducing stromal cell reprogramming to regulate the TME, providing new perspectives for clinical treatment (Ding et al., 2024; Mao et al., 2024; Nie et al., 2024).
Fibrosis is defined as the excessive accumulation of ECM components, like collagen, resulting in abnormal alterations in tissue structure and function. It is a chronic and progressive process, typically linked to prolonged inflammation and damage (Rimal et al., 2022). Additionally, fibrosis, as an essential part of the TME, is mainly manifested by the excessive deposition of ECM and the abnormal activation of stromal cells, including tumor-associated fibroblasts (CAFs) and myofibroblasts (Rimal et al., 2022). With deeper research, the intricate interactions between fibrosis and tumors are increasingly being clarified. Fibrosis facilitates tumor cell proliferation, invasion, and immune evasion (Thomas and Radhakrishnan, 2019; Metcalf et al., 2022). Additionally, tumor cells further aggravate fibrosis by secreting pro-fibrotic factors and inducing chronic inflammatory responses (Wu et al., 2020; Giarratana et al., 2024). Anti-fibrotic treatments, including anti-TGF-β therapy and targeting CAFs, have demonstrated important potential in the treatment of malignant tumors (Mohapatra et al., 2022; Li J. et al., 2023). Thus, comprehending the interaction mechanisms between fibrosis and different tumors is crucial for further research, developing novel therapeutic strategies, and enhancing cancer treatment efficacy.
2 Mechanisms that promote fibrosis in malignant tumors
2.1 The function of CAFs
2.1.1 Pro-fibrotic factors induce the activation of CAFs
Pro-fibrotic factors are vital in the activation and transformation of CAFs. Malignant tumor cells secrete pro-fibrotic factors (like TGF-β and PDGF), which can directly induce the transformation of fibroblasts into CAFs. CAFs represent one of the main cell types within the TME. The high expression of α-smooth muscle actin (α-SMA) and the biological characteristics of secreting multiple cytokines by CAFs play a vital role in tumor fibrosis (Geng et al., 2021). In renal clear cell carcinoma (RCC), cancer cells secrete TGF-β, which induces the transformation of normal fibroblasts into CAFs through the TGF-β-Smad2/3 pathway (Wang Y. et al., 2024). circ_0020256 is highly expressed in cholangiocarcinoma (CCA) and enhances CCA cells’ secretion of TGF-β1, which subsequently activates CAFs via Smad2/3 phosphorylation. Mechanistically, circ_0020256 stabilizes KLF4 mRNA by recruiting EIF4A3 protein and increasing its expression. KLF4 then binds to the TGF-β1 promoter, enhancing its transcription in CCA cells (Li Z. et al., 2023). Hepatocellular carcinoma (HCC) cells secrete exosomes containing miRNA-21, directly targeting the PTEN gene and activating the PDK1/AKT signaling pathway, which promotes the transformation of normal hepatic stellate cells (HSCs) into CAFs with pro-cancer characteristics (Zhou et al., 2018). In oral squamous cell carcinoma (OSCC), PDGF secreted by cancer cells binds to PDGFR-β, activating lncRNA LURAP1L-AS1, which subsequently regulates the IKK/NF-κB signaling pathway, facilitating the activation and transformation of fibroblasts (Ren et al., 2021).
2.1.2 ECM remodeling
CAFs contribute to malignant tumor fibrosis by enhancing the synthesis of collagen, fibronectin, and other ECM components, resulting in excessive ECM accumulation in tissues. CAFs produce and secrete substantial quantities of type I and III collagen, the primary components of the ECM. The over-deposition of these collagens results in tissue stiffening and densification (Xu et al., 2024). Gastric cancer cells induce the abnormal expression and secretion of collagen by activating the FAK/AKT pathway in CAFs, driving malignant transformation and fibrosis (Zhang J. et al., 2024). CAFs secrete small extracellular vesicles (sEVs) that associate with the ECM; these sEVs are enriched with active lysyl oxidase (LOX). LOX interacts with collagen I under the action of sEVs, facilitating collagen cross-linking. Moreover, integrin α2β1 in sEVs mediates their binding to collagen, further strengthening the cross-linking process (Liu Y. et al., 2023). In lung cancer models, increased lipid droplet (LD) content in CAFs promotes their pro-tumor phenotype, characterized by high expression of α-SMA and collagen α-2 chain (COL1A2) (Zhang et al., 2022).
Moreover, CAFs secrete matrix metalloproteinases (MMPs) and tissue inhibitors of metalloproteinases (TIMPs), which control the degradation and remodeling of the ECM. In the process of fibrosis, CAFs aggravate fibrosis by adjusting the balance between MMPs and TIMPs, inhibiting normal ECM degradation, and facilitating ECM accumulation and stabilization (Najafi et al., 2019). For instance, when co-cultured with gastric cancer cells, CAFs significantly upregulate IL-17a expression and enhance the expression of MMP2 and MMP9, while downregulating their inhibitors TIMP1 and TIMP2 (Zhang J. et al., 2020).
2.2 Inflammation
Tumor cells promote fibrosis by persistently releasing inflammatory factors like IL-1, TGF-β1, TNF, and IL-6, which activate the NF-κB and JAK/STAT signaling pathways, inducing fibroblast differentiation into a pro-inflammatory phenotype or myofibroblasts. These fibroblasts further drive fibrosis (Anderson-Crannage et al., 2023). In a lung cancer mouse model, tumor cells induce an inflammatory response in the kidneys by secreting nephrotoxic proteins, which increase the expression of IL-6 and monocyte chemoattractant protein-1 (MCP-1), resulting in glomerular capillary collapse and tumor antigen deposition. Concurrently, the TGF-β signaling pathway is activated, triggering renal fibrosis (Hung et al., 2020). In pancreatic ductal adenocarcinoma (PDAC), tumor cells induce an inflammatory response in pancreatic stellate cells (PSCs) by absorbing lipids, which subsequently promotes PSC activation and triggers fibrosis (Hata et al., 2017). In pancreatic neuroendocrine tumors, cancer cells secrete interleukin-1 (IL-1), which induces CAFs to secrete stromal cell-derived factor 1 (SDF1), aggravating the extent of tumor fibrosis (Lai et al., 2024).
2.3 Signaling pathway
2.3.1 TGF-β/Smad signal transduction pathway
The TGF-β/Smad pathway serves as a key regulator in the fibrosis of malignant tumors. This pathway drives fibrosis formation and progression by modulating fibroblast proliferation, differentiation, activation, and the synthesis and deposition of ECM. TGF-β binds to the type II TGF-β receptor (TGF-βRII) on the cell surface, which then activates the kinase activity of TGF-βRI and triggers the phosphorylation of downstream Smad proteins. The phosphorylated Smad2 and Smad3 associate with the co-transcription factor Smad4, forming an active complex that moves into the nucleus to regulate the transcription of fibrosis-related genes (Lee and Massagué, 2022; Li J. et al., 2024).
Activation of the TGF-β-Smad2/3 pathway induces the expression of fibrotic factors, which leads to fibroblast activation, promoting their proliferation and differentiation into myofibroblasts. Myofibroblasts display increased α-SMA expression and an enhanced ability to synthesize ECM, thereby intensifying fibrosis within tumors and promoting tumor growth and progression (Su et al., 2020). The activation of the upstream Notch signaling pathway triggers the TGF-β/Smad pathway, which promotes the migration of mesenchymal stem cells to the stroma and their differentiation into fibroblasts (Peng et al., 2014). In breast cancer, a lack of glutamine can trigger the activation of TGF-β signaling, leading to the activation of associated fibroblasts and subsequent fibrosis. The activity of histone deacetylase 1 and the inhibition of mTORC1 are required for TGF-β signaling activation and the conversion of CAFs into a myofibroblast state (Mezawa et al., 2023). During cachexia, inflammation within tumors drives fibrosis. This process might be driven by TGF-β-induced differentiation of fibroblasts into myofibroblasts, resulting in imbalanced inflammatory cytokine expression, enhanced angiogenesis, and increased ECM components (Lima et al., 2019).
The TGF-β/Smad signaling pathway is essential in fibrosis, tumor progression, and metastasis by enhancing ECM synthesis and deposition. The epigenetic regulators UBR7 and histone methyltransferase EZH2 regulate TGF-β/Smad signaling. With the activation of the TGF-β/Smad pathway, collagen content and lysyl oxidase activity rise, directly impacting ECM stiffness (Adhikari et al., 2024). In unilateral breast cancer-associated lymphedema, TGF-β1 intensifies the fibrosis process by increasing the stiffness of fibroblasts, lymphatic endothelial cells, and lymphatic smooth muscle cells, and by enhancing ECM deposition (Baik et al., 2022).
2.3.2 JAK/STAT signal transduction pathway
The JAK/STAT signaling pathway drives fibrosis formation and reshapes the tumor microenvironment by mediating cell proliferation, differentiation, immune regulation, and inflammatory responses. Cytokines (like IL-6, IFN, and IL-13) bind to receptors, leading to JAK activation, followed by STAT protein phosphorylation. Phosphorylated STAT proteins dimerize and move into the nucleus, where they bind to DNA sequences to regulate the transcription of fibrosis-related genes (Liu X. et al., 2023). Bioinformatics analysis has shown that hub genes are significantly enriched in the JAK/STAT pathway in expression profiles associated with liver fibrosis and liver cancer (Hamdy et al., 2023). With the marked activation of pSTAT5 and pSTAT3, levels of pro-inflammatory and pro-tumor mediators rise, resulting in higher liver tumor burden and significantly increased fibrosis in mice (Cabrera-Galván et al., 2023). In RCC, RCC-derived CXCL5 promotes fibrosis by activating the JAK/STAT3 pathway, facilitating the transformation of normal fibroblasts into CAFs (Liu Y. et al., 2023). Research indicates that reprogrammed mouse liver cells, driven by IL6/Jak/Stat3 signaling pathways, convert into LGR5-positive cells. When transplanted into syngeneic mice, these LGR5-positive cells develop into invasive and metastatic tumors with marked fibrosis, underscoring the significance of the JAK/STAT pathway in malignant tumor fibrosis (Chaker et al., 2024). Research by Grohmann et al. shows that inhibiting STAT-1 signaling prevents T cell recruitment and fibrosis but does not prevent hepatocellular carcinoma; whereas correcting STAT-3 signaling can prevent liver cancer without affecting fibrosis. This research provides a more detailed explanation of the role of the JAK/STAT signaling pathway in malignant tumor fibrosis (Grohmann et al., 2018).
2.3.3 Wnt/β-catenin signal transduction pathway
Wnt proteins bind to cell surface receptors, activating β-catenin, leading to its accumulation and translocation to the nucleus, where it regulates the expression of fibrosis-related genes. These genes generally pertain to ECM synthesis and fibroblast activation (Feng et al., 2018). In lung adenocarcinoma, smoking induces the downregulation of filamin A interacting protein 1-like (FILIP1L), which activates the Wnt/β-catenin signaling pathway, resulting in mucin secretion, inflammation, and fibrosis (Kwon et al., 2022). In oral submucous fibrosis and OSCC tissues, hypermethylation of dickkopf-1 may lead to its downregulation, causing abnormal activation of the Wnt/β-catenin signaling pathway, potentially playing a crucial role in the pathogenesis of oral submucous fibrosis (He et al., 2020). Additionally, proteins associated with the Wnt/β-catenin pathway are highly expressed in pancreatic exocrine tissues, with significant alterations in their cellular and subcellular expression patterns, correlating with increased fibrosis (Bläuer et al., 2019). Stearoyl-CoA desaturase (SCD) in liver tumor-initiating stem-like cells (TIC) is regulated by Wnt/β-catenin signaling. The monounsaturated fatty acids produced by SCD stabilize LRP5/6 mRNA, forming a positive feedback loop that amplifies Wnt signaling, which in turn promotes liver fibrosis and tumor growth (Lai et al., 2017).
2.3.4 Notch signal transduction pathway
The Notch signaling pathway is activated by the interaction between Notch receptors and ligands. After receptor activation, proteolytic cleavage releases the Notch intracellular domain (NICD), which then translocates to the nucleus to regulate the transcription of specific genes. Suppressing Notch signaling can inhibit the activation of the classical TGF-β1 pathway and reduce the peritumoral desmoplastic reaction in cholangiocarcinoma (Mancarella et al., 2022). In liver cancer cells chronically exposed to low concentrations of cadmium, the activation of Notch and AKT/mTOR signaling pathways can induce the expression of the pro-inflammatory cytokine tumor necrosis factor-α (TNF-α) and its downstream target TNF-α-induced protein 8 (TNFAIP8), thus regulating fibrosis and oncogenic signaling in liver cancer cells (Niture et al., 2023). Refer to Figure 1 for the mechanisms by which malignant tumors promote fibrosis.
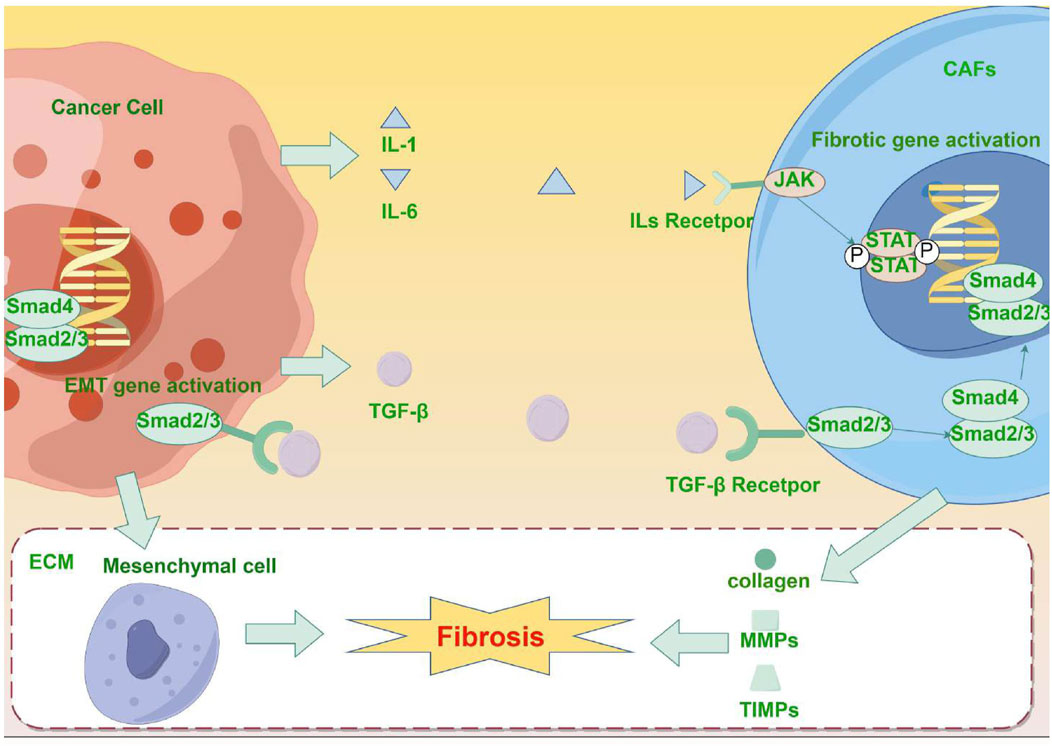
Figure 1. (By Figdraw, ID: RASTR41933) Mechanism of fibrosis promotion by malignant tumors: Tumor cells secrete pro-fibrotic factor TGF-β and activate CAFs through the TGF-β/Smad signaling pathway. Furthermore, tumor cells and CAFs secrete inflammatory factors (such as IL-1 and IL-6), which can further induce the production of additional pro-fibrotic factors, thus further activating CAFs. The activated CAFs promote the synthesis of collagen, fibronectin, and other ECM components, and affect ECM degradation and remodeling by regulating the secretion of MMPs and TIMPs. Additionally, the activation of the TGF-β/Smad signaling pathway is linked to the occurrence of EMT in malignant tumors, and the interaction between EMT and ECM remodeling further accelerates the fibrosis process.
2.3.5 The cross-talk effects of signaling pathways
It is worth noting that during the fibrosis process in malignant tumors, multiple signaling pathways do not function independently but often co-regulate fibrosis and tumor progression through complex interaction mechanisms. The interactions between different signaling pathways form a highly integrated network, which has a profound impact on the tumor microenvironment, cell proliferation, invasion, and treatment resistance. For instance, TGF-β1-induced activation of activating transcription factor 4 (ATF4) is dependent on the activation of the classical TGF-β1/Smad3 signaling and mTORC1-4E-BP1. ATF4 then promotes the de novo synthesis of enzymes from the serine-glycine biosynthesis pathway and transcription of the GLUT1 gene. This process meets the biosynthetic demands required for enhanced ECM synthesis (Selvarajah et al., 2019).
3 The effect of fibrosis on tumor progression
3.1 Enhances tumor proliferation and survival
Fibrosis results in the abnormal accumulation of extracellular matrix (ECM), especially the increase in collagen, fibronectin, and hyaluronic acid. These ECM components not only offer structural support for tumor cells but also interact with cell surface receptors, activating signaling pathways that promote proliferation and survival. In liver cancer, Sema3C supports tumor fibrosis by promoting the proliferation of hepatic stellate cells (HSCs). Moreover, Sema3C interacts with NRP1 and ITGB1 receptors, activating the AKT/Gli1/c-Myc signaling pathway, promoting the self-renewal and proliferation of HCC cells (Peng et al., 2024). The increased tissue stiffness due to fibrosis further promotes tumor cell proliferation and survival via mechanotransduction pathways, such as the YAP pathway (Schrader et al., 2011; Deng et al., 2022).
Fibroblasts and CAFs within the fibrotic microenvironment secrete numerous growth factors, such as TGF-β and EGF. These factors facilitate tumor cell proliferation and survival by activating downstream signaling pathways. In prostate cancer, TGF-β1 is recognized as a highly secreted growth factor in CAFs, significantly enhancing tumor cell growth and proliferation in both in vivo and in vitro settings (Dy et al., 2019). In PDAC, CAF-derived thrombospondin 1 (TSP1) activates TGF-β signaling, leading to the loss of Smad4 expression in cancer cells and accelerating their proliferation and migration (Matsumura et al., 2022). In cholangiocarcinoma, CAF-secreted TSP-4 binds to integrin α2 on cancer cells, activating HSF1 and Akt signaling pathways. Activated HSF1 further enhances TGF-β1 expression and secretion, inducing the transformation of fibroblasts into CAFs and creating a positive feedback loop that promotes cell proliferation and advances cholangiocarcinoma progression (Shi et al., 2021).
3.2 Enhances angiogenesis
3.2.1 Secretion of angiogenesis-promoting factors
In malignant tumors, fibrosis promotes angiogenesis through various mechanisms, supplying the necessary nutrients and oxygen for tumor growth and expansion. During the fibrosis process in malignant tumors, fibroblasts and CAFs are activated, leading to the secretion of significant amounts of pro-angiogenic factors, including vascular endothelial growth factor (VEGF), platelet-derived growth factor (PDGF), and basic fibroblast growth factor (bFGF). These factors interact with receptors on vascular endothelial cells, activating signaling pathways that promote the formation of new blood vessels (Sobierajska et al., 2020).
In several malignant tumors, such as head and neck squamous cell carcinoma, RCC, and cholangiocarcinoma, CAFs can directly secrete VEGF to promote angiogenesis (Sun et al., 2022; Zhou et al., 2022; Liu J. et al., 2023). In colorectal cancer patients, exosomes released by CAFs enhance endothelial cell proliferation, migration, and angiogenesis by increasing the expression and secretion of VEGF. Specifically, circ_0084043 is highly expressed in CAF-derived exosomes and regulates HIF-1α and VEGFA by sponging miR-140-3p, suggesting that the circ_0084043/miR-140-3p/VEGF signaling pathway plays a critical role in CAF exosome-induced angiogenesis (Payervand et al., 2024). miR-210 secreted by lung cancer cells enhances angiogenesis by increasing VEGF via the activation of the JAK2/STAT3 signaling pathway in CAFs (Fan et al., 2020). Similarly, research by Dai et al. (2022) showed that CAF-derived extracellular vesicles promote angiogenesis in colorectal adenocarcinoma cells via the miR-135b-5p/FOXO1 axis, indicating the crucial role of non-coding RNAs in enhancing the secretion of angiogenic factors by CAFs.
Moreover, several signaling pathways are also crucial in regulating VEGF secretion. When CAFs are co-cultured with glioma C6 cells, the expression levels of VEGF-A and EGF proteins are significantly elevated, thereby enhancing glioma cell invasiveness, proliferation, and angiogenesis (Zhang S. et al., 2023). In triple-negative breast cancer (TNBC), particularly in patients with BRCA1 mutations, iCAFs have been found to be enriched and promote angiogenesis by interacting with tumor endothelial cells (TECs) via VEGF signaling. iCAFs activate angiogenesis-related genes (such as FLT1 and KDR) in TECs through the VEGF signaling pathway, promoting endothelial cell migration and sprouting angiogenesis (Lee et al., 2024). In breast cancer, the upregulation of VEGF-A and IL-8, along with their upstream effectors mTOR and HIF-1α, can enhance the pro-angiogenic potential of CAFs (Al-Kharashi et al., 2022). In melanoma, CD38-positive CAFs promote tumor cell migration and invasion, as well as endothelial cell tube formation, by secreting factors like VEGF-A, FGF-2, and CXCL-12 through paracrine signaling in vitro (Ben Baruch et al., 2020).
PDGF and other angiogenesis-promoting factors likewise play a crucial role in driving angiogenesis facilitated by CAFs. Chu et al. (2022) discovered that VEGF, angiopoietin, bFGF, and other factors secreted by CAFs are crucial in the angiogenesis of precancerous and malignant lesions in laryngeal cancer. In OSCC, reprogramming of glucose metabolism results in increased secretion of angiogenesis-promoting factors (VEGF-A, PDGF-C, and MMP9) by CAFs, which enhances the angiogenic phenotype (Li X. et al., 2022). In cholangiocarcinoma, CAFs secrete stem cell factor (SCF), which recruits mast cells and stimulates them to release hyaluronic acid (HA) via the MRGPRX2-Gαq signaling pathway. These bile-induced MCs subsequently release PDGF-B, which further enhances angiogenesis in cholangiocarcinoma (Shi et al., 2024).
3.2.2 ECM remodeling
Fibrosis caused by malignant tumors results in excessive extracellular matrix (ECM) deposition, offering a physical scaffold for new blood vessel formation and supporting vascular expansion within the dense matrix. In cholangiocarcinoma, the overexpression of PI3Kδ is closely related to stromal remodeling, manifesting as a thick ECM at the basement membrane and significant angiogenesis and lymphangiogenesis. The mechanism involves PI3Kδ promoting ECM remodeling via the TGFβ/Src/Notch signaling pathway, which in turn enhances angiogenesis (Bou Malham et al., 2023). In bladder cancer, the Sigma 1 receptor (Sig1R) can regulate crosstalk between the ECM and tumor cells, facilitating ECM-mediated cell proliferation and angiogenesis (Feng et al., 2023).
3.2.3 Hypoxia and the activation of HIF-1α
Fibrosis increases the density of tumor tissue, limiting oxygen diffusion and creating a hypoxic microenvironment. Hypoxia-inducible factors (HIFs) become stabilized and activated in hypoxic conditions, enhancing the expression of pro-angiogenic genes like VEGF, which drives the formation of new blood vessels (Yehia et al., 2015; De Marco et al., 2022). Pancreatic cancer features excessive desmoplastic reaction and a hypoxic microenvironment within the solid tumor mass. Hypoxia induces the production of HIF-1, which not only enhances the migration of pancreatic stellate cells (PSCs) and the expression of type I collagen but also increases VEGF secretion, promoting angiogenesis (Masamune et al., 2008; N et al., 2016).
3.3 Enhances immune evasion
The dense ECM structure created by fibrosis obstructs immune cell infiltration, diminishing their tumor-killing capacity and aiding tumor cells in evading immune surveillance. In the fibrotic tumor microenvironment, tumor-associated macrophages (TAMs) initiate collagen synthesis via the TGF-β signaling pathway, causing tumor tissue stiffening and establishing a metabolic environment that impairs CD8+ T cell function. Macrophages engaged in collagen synthesis deplete arginine in the environment and produce proline and secrete ornithine, which further suppresses the antitumor response of CD8+ T cells. Therefore, fibrosis not only physically repels CD8+ T cells but also weakens the immune response against cancer by altering the metabolic environment (Tharp et al., 2024). In non-small cell lung cancer (NSCLC), significant fibrosis corresponds with reduced T cell infiltration, resulting in impaired immune surveillance. Fibrosis not only accelerates tumor progression but also reduces the number and function of dendritic cells and alters macrophage phenotypes, further intensifying immune suppression (Herzog et al., 2023). Liver fibrosis enhances tumor immune evasion in hepatocellular carcinoma, resulting in decreased CD8+ T cell infiltration and increased expression of the immune checkpoint molecule programmed death-ligand 1 (PD-L1). Specifically, Golgi membrane protein 1 (GOLM1) in fibrosis induces PD-L1 expression via the activation of the EGFR pathway, thereby suppressing antitumor immune responses (Ke et al., 2021). In the lung adenocarcinoma microenvironment, CAFs increase the expression of PD-L1 in tumor cells by secreting cytokines like CXCL2. High PD-L1 expression allows tumor cells to suppress CD8+ T cell activity in the immune system, facilitating immune evasion (Inoue et al., 2019). Regulatory T cells (Tregs) and CAFs interact to collaboratively enhance fibrosis and immune suppression. Specifically, IL-33 enhances Treg cell activity through the IL1RL1 signaling pathway, and these Tregs interact with CAFs via the AREG/EGFR axis, inducing CAFs into a pro-fibrotic and immunosuppressive state (Sun et al., 2023). However, it is important to note that fibrosis in malignant tumors can facilitate tumor immune evasion while also constraining tumor size expansion (Li et al., 2021).
4 Fibrosis enhances treatment resistance
4.1 Chemotherapy resistance
Chemotherapy is a treatment approach that employs chemical agents to kill cancer cells or inhibit their growth and division, commonly used in the treatment of various malignant tumors. However, chemoresistance is a significant challenge in the treatment of malignant tumors, and it is often accompanied by fibrosis in affected patients. In a pancreatic ductal adenocarcinoma model, ectopic tumors showed more pronounced fibrosis, which led to increased resistance to FOLFIRINOX chemotherapy. Despite similar drug absorption in tumor tissues, fibrosis and microenvironmental differences significantly impacted the treatment response (Erstad et al., 2018). In breast cancer, fibrosis-related signaling pathways are significantly upregulated in patients who do not achieve a complete response to neoadjuvant chemotherapy; patients with high fibrosis have lower complete response rates and shorter survival durations (Wang X. et al., 2024).
Fibrosis is frequently accompanied by epithelial-mesenchymal transition (EMT), which converts tumor cells from an epithelial phenotype to a mesenchymal phenotype. EMT provides tumor cells with enhanced migratory ability and resistance to apoptosis, thereby increasing their resistance to chemotherapy. In 5-Fu-resistant (5-FU) breast cancer cell lines, tumor cells induce normal dermal fibroblasts to convert into a CAF phenotype via TGF-β1 paracrine signaling, promoting fibrosis, reducing E-cadherin expression, and facilitating EMT (Chandra Jena et al., 2021). CAFs can transfer exosomes to colorectal cancer cells, promoting stemness and EMT in CRC cells, which in turn enhances resistance to 5-FU/oxaliplatin (L-OHP) chemotherapy. Mechanistically, exosomes induce miR-92a-3p production, activating the Wnt/β-catenin pathway, inhibiting FBXW7 and MOAP1 expression, and suppressing mitochondrial apoptosis, thereby enhancing stemness and chemoresistance (Hu et al., 2019). In ovarian cancer, CAFs may activate the Wnt/β-catenin pathway via the CXCL12/CXCR4 axis, promoting cisplatin resistance by inducing EMT (Zhang F. et al., 2020). IL-6 derived from CAFs plays a crucial role in maintaining the paracrine loop between CAFs and NSCLC cells by enhancing EMT in NSCLC cells. This paracrine loop enhances intercellular communication, which subsequently leads to the development of chemoresistance (Shintani et al., 2016).
In addition to EMT, fibrosis can promote chemoresistance by activating tumor stem cell properties and anti-apoptotic signaling pathways. In PDAC, proliferating resident macrophages (proliferating rMφs) significantly increase tumor resistance to chemotherapy by promoting fibrosis and immune suppression. Multi-omics analysis found that these macrophages promote cancer cell survival during chemotherapy by producing more deoxycytidine (dC) and less dC kinase (dCK), reducing the absorption of gemcitabine (Zhang J. et al., 2023). Additionally, CAFs promote tumor fibrosis via the IL1β-IRAK4 signaling pathway, which enhances tumor cell survival and proliferation, resulting in gemcitabine resistance (Zhang et al., 2018). In lung adenocarcinoma, cancer stem cells (CSCs) secrete the acute-phase protein serum amyloid A (SAA), remodeling the tumor microenvironment, promoting fibrosis, and enhancing cisplatin (DDP) chemoresistance (Wang et al., 2023). In ovarian cancer, high expression of CHI3L1 (a secretory glycoprotein) is closely linked to fibrosis. CHI3L1 activates the Akt and Erk signaling pathways, enhancing the expression of β-catenin and SOX2, promoting stem-like characteristics in ovarian cancer cells, such as resistance to apoptosis, thereby increasing paclitaxel chemoresistance (Lin et al., 2019). In CAF-derived exosomes, the significantly upregulated circBIRC6 promotes the SUMOylation of XRCC4, enhancing its interaction with SUMO1 at lysine 115, facilitating XRCC4 chromatin localization, and increasing pancreatic cancer cell resistance to oxaliplatin (Zheng et al., 2023). In conclusion, fibrosis can enhance chemotherapy resistance through multiple mechanisms, including EMT, CSC, and anti-apoptotic pathways.
4.2 Resistance to immunotherapy
Presently, immunotherapy is an emerging cancer treatment method that fights cancer by enhancing or regulating the immune system. Immune checkpoint inhibitors are widely used immunotherapy strategies, among which PD-1/PD-L1 inhibitors (like pembrolizumab and nivolumab) and CTLA-4 inhibitors (such as ipilimumab) have shown significant therapeutic potential in tumor immunotherapy (Li W. et al., 2022). In tumors that respond to immunotherapy, the TME shows enrichment of immune cells and CAFs, along with pro-inflammatory signaling and ECM remodeling, which aligns with proliferative fibrosis and immune-mediated tumor regression. However, tumor heterogeneity may result in immune-deficient regions, promoting immune evasion and early recurrence via HCC-CAF interactions and the expression of cancer stem cell markers. This indicates that fibrosis may contribute to immunotherapy resistance in certain cases, heightening treatment challenges (Zhang M. et al., 2023). In breast cancer, fibrosis facilitates immunotherapy resistance by increasing TAMs, EMT, fibroblast proliferation, ECM enhancement, and Wnt pathway activation. These alterations together create an immune-tolerant microenvironment, diminishing the effectiveness of PD-1 inhibitors (Yuan et al., 2022). Further research by Song et al. (2024) revealed a link between anti-PD-L1 therapy and fibrosis: During liver fibrosis, pathogenic Th17 cells (pTh17) significantly increase, and anti-PD-L1 therapy promotes pTh17 cell infiltration and activation in the liver. These pTh17 cells secrete IL-17A, which increases PD-L1 expression on the surface of hepatocellular carcinoma cells, further worsening liver cirrhosis and leading to resistance to anti-PD-L1 therapy (Song et al., 2024).
4.3 CAFs enhance resistance to targeted therapy
Targeted therapy is a form of cancer treatment that specifically targets certain molecules or signaling pathways in cancer cells. Unlike traditional chemotherapy, targeted therapy precisely identifies and inhibits abnormal proteins or genetic mutations in cancer cells, preventing tumor growth and spread while minimizing harm to normal cells.
4.3.1 Resistance to tyrosine kinase inhibitors
In the fibrotic microenvironment, CAFs play a key role in promoting resistance to tyrosine kinase inhibitors (TKIs). For example, in RCC, CAFs facilitate resistance to VEGFR-TKIs (Ambrosetti et al., 2022). In HCC, bioinformatics analysis identified SPP1 secreted by CAFs as a candidate molecule for resistance to sorafenib and lenvatinib. CAF-secreted SPP1 activates the RAF/MAPK and PI3K/AKT/mTOR pathways via the integrin-PKCα signaling pathway and promotes EMT, resulting in TKI resistance (Eun et al., 2023). CAFs enhance the secretion of HGF and IGF-1, activating the c-met and IGF-1R receptors, leading to increased ANXA2 expression and phosphorylation, inducing EMT and resulting in resistance to EGFR-TKIs (e.g., gefitinib) in NSCLC (Yi et al., 2018). Similarly, in NSCLC, CAFs derived from osimertinib-resistant cells secrete higher levels of IL-6, IL-8, and hepatocyte growth factor (HGF), express stronger CAF markers such as α-SMA, FAP, and PDGFR, and increase stemness and osimertinib resistance in NSCLC cells (Huang W. et al., 2021). In EGFR-TKI-resistant tumors, part of the CAF-derived tumor stroma is composed of EMT-derived tumor cells that express resistance markers, such as epithelial membrane protein-1. CAFs secrete paracrine factors that reduce the inhibitory effects of TKIs on pEGFR and pMAPK, thereby promoting tumor cell survival and drug resistance (Sr et al., 2010). In gastric cancer, cancer cells secrete lactate, inducing CAFs to produce BDNF, activating the TrkB-Nrf2 signaling pathway, inhibiting anlotinib-induced apoptosis and reactive oxygen species (ROS) generation, thus reducing drug efficacy (Jin et al., 2021). In RCC, CAFs increase sunitinib resistance by secreting CXCL3, which activates the CXCR2-ERK1/2 signaling pathway in tumor cells, promoting EMT and stemness (Wang Y. et al., 2024).
4.3.2 Resistance to monoclonal antibodies
Monoclonal antibodies (mAbs) are a crucial class of drugs in targeted therapy, specifically targeting certain antigens or receptors on cancer cell surfaces. They kill specific cancer cells by directly blocking signal transduction, activating ADCC, or inducing complement-dependent cytotoxicity (CDC). Common monoclonal antibodies include trastuzumab, bevacizumab, and cetuximab.
Trastuzumab can target the HER2 receptor and is used for treating HER2-positive breast cancer and gastric cancer. CAFs are enriched in trastuzumab-resistant HER2-positive breast cancer cases. These CAFs secrete immunosuppressive factors IDO1 and TDO2, inhibiting NK cell-mediated antibody-dependent cellular cytotoxicity (ADCC), thereby causing resistance to trastuzumab (Du et al., 2023). CAF-derived Neuregulin 1 (NRG1) also mediates trastuzumab resistance in breast cancer by activating the HER3/AKT signaling pathway. However, pertuzumab may reverse resistance by targeting this pathway (Guardia et al., 2021). Mao et al. (2015)’s research shows that CAFs can induce trastuzumab resistance by expanding cancer stem cells and activating multiple pathways including NF-κB, JAK/STAT3, and PI3K/AKT.
Bevacizumab targets VEGF to inhibit angiogenesis and is used in the treatment of colorectal cancer, non-small cell lung cancer, renal cell carcinoma, and more. In OSCC, CAFs play a key role in angiogenesis by secreting sEVs. CAF-derived sEVs bind to VEGF and activate the VEGFR2 signaling pathway in human umbilical vein endothelial cells (HUVECs). Even after Bevacizumab treatment, VEGF bound to sEVs can continue to activate VEGFR2. This indicates that sEVs secreted by CAFs can bind VEGF via heparan sulfate proteoglycans on their surface, making them resistant to Bevacizumab (Li et al., 2020).
Cetuximab targets EGFR and is used for targeted therapy in colorectal cancer and head and neck squamous cell carcinoma (HNSCC). In CRC, CAFs significantly increase CRC cell resistance to Cetuximab by regulating the expression of the EMT key factor SNAI1 and remodeling the ECM (Galindo-Pumariño et al., 2022). In HNSCC, TGF-β-activated CAFs limit Cetuximab efficacy by upregulating the TGF-β signaling pathway, thereby enhancing drug resistance in the tumor microenvironment (Yegodayev et al., 2020). Further studies indicate that CAF-derived MMP-1 expression increases in both tumor cells and CAFs, promoting resistance to Cetuximab (Johansson et al., 2012). Refer to Figure 2 for fibrosis-promoted malignant tumor progression and treatment resistance. Refer to Table 1 for details on how fibrosis promotes treatment resistance.
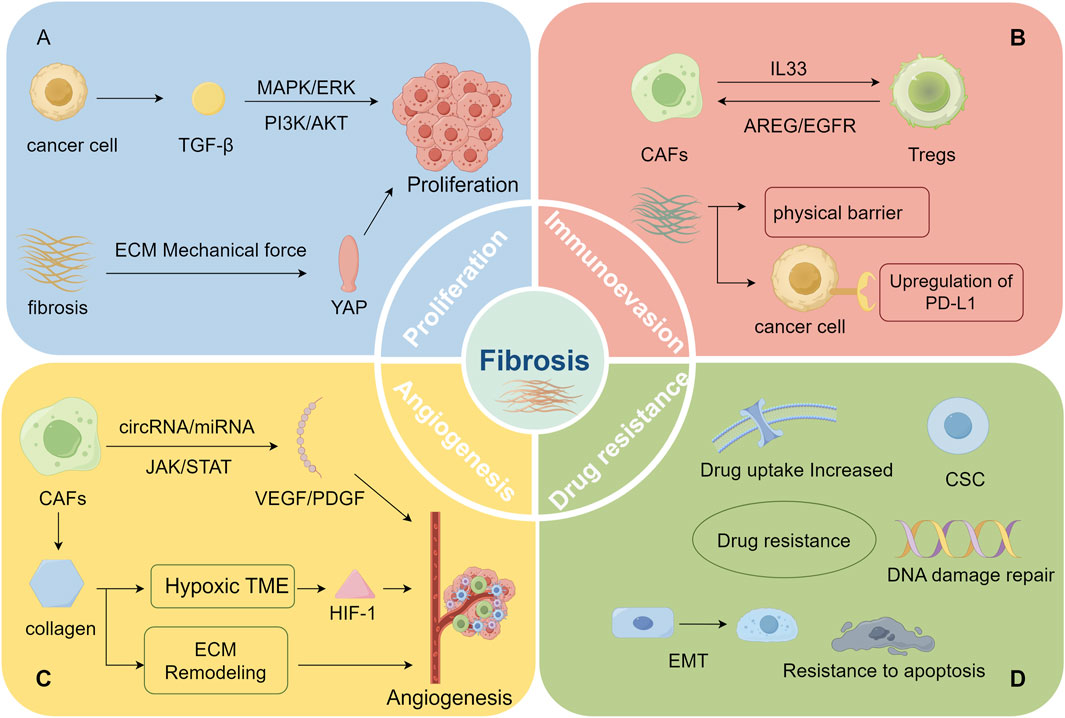
Figure 2. (By Figdraw, ID: RYWAR2b2b6) Fibrosis facilitates malignant tumor progression: (A) enhances tumor proliferation, (B) promotes immune evasion, (C) drives angiogenesis, (D) increases treatment resistance (A) Malignant tumors enhance tumor cell proliferation by secreting TGF-β, which activates pathways such as PI3K-AKT and MAPK/ERK. Furthermore, the increased tissue stiffness from fibrosis promotes tumor cell proliferation via mechanotransduction pathways (like the YAP pathway). (B) The interaction between Treg cells and CAFs not only promotes fibrosis but also intensifies immunosuppression. Moreover, the dense ECM structure resulting from fibrosis obstructs immune cell infiltration and aids tumor cells in evading immune surveillance by upregulating PD-L1 expression. (C) CAFs enhance the secretion of pro-angiogenic factors like VEGF and PDGF via the regulation of non-coding RNAs and signaling pathways such as JAK, thereby promoting tumor angiogenesis. The excessive deposition of ECM provides a physical scaffold for new blood vessel formation, supporting vascular expansion within the dense matrix. Additionally, excessive ECM accumulation restricts oxygen, activating HIF-1 and further promoting angiogenesis. (D) Fibrosis facilitates treatment resistance in malignant tumors through multiple mechanisms, including CSC activation, EMT induction, apoptosis inhibition, enhanced DNA damage repair, and reduced drug uptake, thereby weakening the efficacy of chemotherapy, targeted therapy, and immunotherapy.
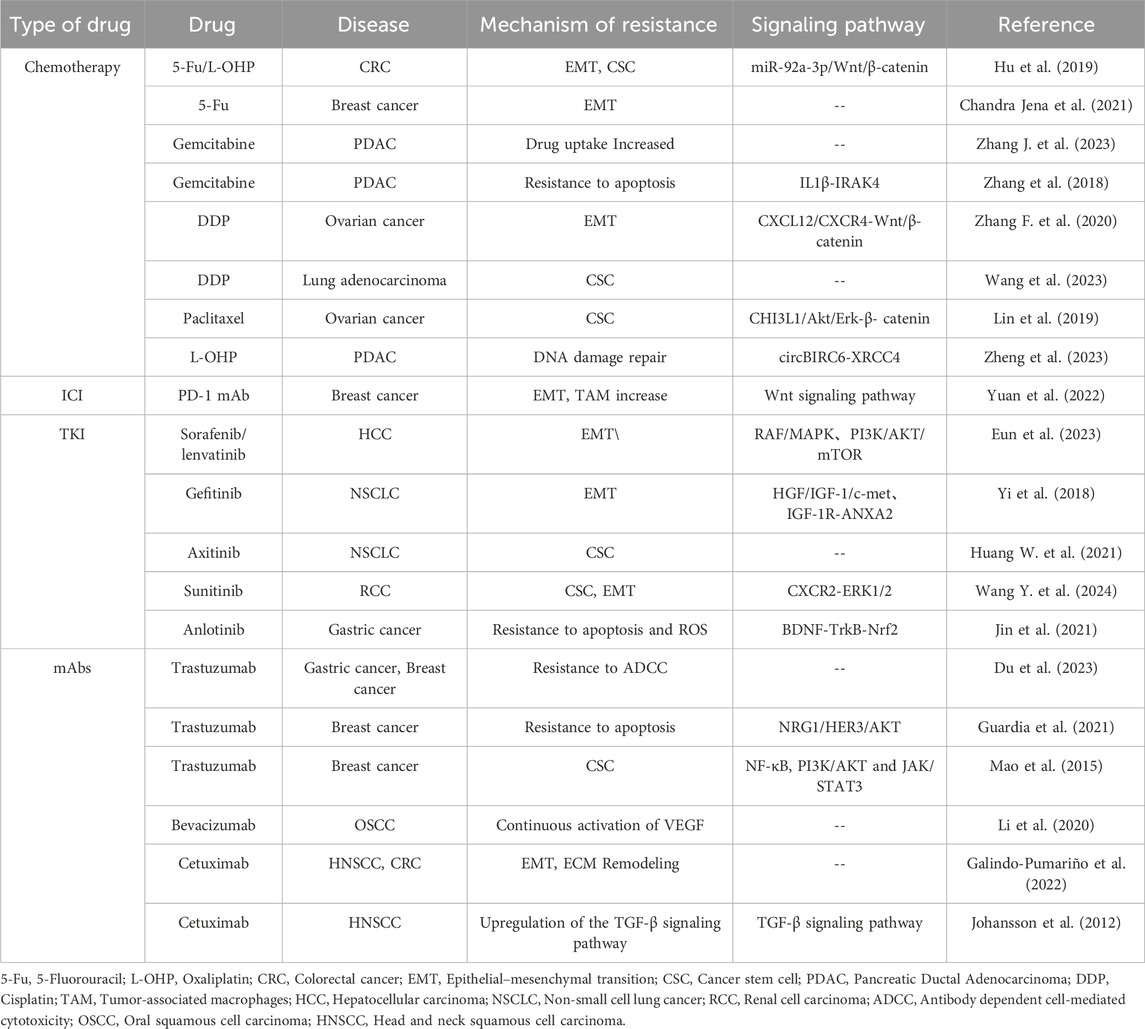
Table 1. Summarize the mechanisms and associated signaling pathways through which fibrosis promotes resistance to chemotherapy, immunotherapy, and targeted therapy.
5 Tumor therapeutic strategies targeting fibrosis
5.1 Nintedanib
5.1.1 Clinical trials
Nintedanib is a small-molecule TKI with antifibrotic and anti-inflammatory properties, mainly used in the treatment of idiopathic pulmonary fibrosis. The clinical use of antifibrotic drugs such as Nintedanib can significantly improve the survival time of patients with certain malignant tumors. In refractory metastatic CRC, Nintedanib combined with capecitabine is well tolerated and clinically more effective than regorafenib or trifluridine/tipiracil monotherapy. In a study of 36 patients, the median progression-free survival (PFS) was 3.4 months, and the median overall survival (OS) was 8.9 months after 18 weeks (Boland et al., 2024). Nintedanib combined with chemotherapy significantly improved PFS in NSCLC patients, though it had no significant impact on OS. A meta-analysis of three randomized controlled trials involving 2,270 patients showed that PFS in the Nintedanib group was significantly better than in the placebo group (HR = 0.79; 95% CI 0.71–0.88, p < 0.0001) (Alhadeethi et al., 2024). Additionally, a multicenter retrospective study indicated that Nintedanib combined with docetaxel had some efficacy in advanced NSCLC patients following the failure of immune checkpoint inhibitors (ICI) and/or chemotherapy. In 96 patients, the objective response rate (ORR) was 18.8%, the disease control rate (DCR) was 57.3%, the median PFS was 3.0 months, and the median OS was 8.0 months. Particularly in patients treated with Nintedanib and docetaxel after first-line ChT-ICI therapy, the ORR was 29.2%, the DCR was 66.7%, and the median PFS was 4.0 months (Ljubicic et al., 2023). These studies indicate that Nintedanib can effectively improve survival time in patients with certain malignant tumors.
However, there are ongoing debates regarding the response rate and safety of Nintedanib. In a double-blind, randomized, phase 2 trial adding Nintedanib to neoadjuvant chemotherapy for muscle-invasive bladder cancer, the pathological complete response rate (pCR) was similar between the Nintedanib and placebo groups (37% vs. 32%). However, the Nintedanib group showed a higher incidence of grade 3 or higher toxic events (93% vs. 79%), with the most common serious adverse events being thromboembolic events (30% vs. 21%) and neutropenia (39% vs. 11%) (Hussain et al., 2022). In ovarian cancer, the Nintedanib treatment group showed worse PFS and OS compared to the placebo group, along with higher toxicity (92% vs. 69% for grade 3/4 adverse events), primarily consisting of hematologic and gastrointestinal side effects (Ferron et al., 2023). Refer to Table 2 for the clinical trial results of Nintedanib.
5.1.2 Sensitization to chemotherapy and immunotherapy
Combining Nintedanib with immunotherapy or chemotherapy drugs can significantly improve treatment outcomes and promote tumor cell death. Nintedanib significantly inhibits tumor growth in mouse models. When combined with anti-PD-1 antibodies, Nintedanib enhances antitumor efficacy primarily by reducing the number of TAMs and polarizing them into the antitumor M1 phenotype. The combination therapy also restores macrophage phagocytic function, enhancing treatment effectiveness (Tada et al., 2023). In malignant tumors, combining Nintedanib with PD-L1 enhances immune cell infiltration and activation within the tumor, boosts interferon-γ response, and activates MHC class I-mediated antigen presentation. It also promotes PD-L1 expression and STAT3 phosphorylation, thereby improving the effectiveness of immunotherapy (Tu et al., 2022). In PDACs, Nintedanib inhibits CAF secretion of IL-6 by blocking the PDGFRβ signaling pathway. Moreover, MSLN-targeted chimeric antigen receptor-NK cells combined with Nintedanib significantly enhanced tumor-killing ability in xenograft models, triggering robust NK cell infiltration (Lee et al., 2023).
In a xenograft model derived from gastric adenocarcinoma cells, Nintedanib inhibited tumor cell proliferation, reduced tumor angiogenesis, and increased tumor cell death. Notably, when combined with docetaxel and irinotecan, it significantly extended the animals’ survival (Awasthi et al., 2023).
5.2 Pirfenidone (PFD)
5.2.1 PFD suppresses tumor invasion capability
Clinically, PFD is an approved drug used to treat idiopathic pulmonary fibrosis. It alleviates fibrotic responses by inhibiting TGF-β and other profibrotic factors and can significantly reduce tumor invasiveness by inhibiting EMT, regulating immune responses in the tumor microenvironment, and remodeling the ECM. For instance, PFD can inhibit the growth of breast tumors in mice and alcohol-promoted metastasis (Li H. et al., 2024). In TNBC, PFD reduces the expression of EMT-related transcription factors and mesenchymal genes by inhibiting the TGF-β/Smad signaling pathway, thereby inhibiting the proliferation, migration, and invasion of breast cancer cells while promoting apoptosis (Luo et al., 2023). PFD promotes the downregulation of ZEB1 via miR-200 in NSCLC exosomes, slowing down migration, invasion, and EMT processes (Liu et al., 2022). In RCC, PFD significantly inhibits the progression of renal cancer by targeting the TGF-β signaling pathway. PFD decreases TGF-β expression and secretion, blocking TGF-β-induced EMT and thus reducing the proliferation, migration, and invasion of renal cancer cells. Additionally, PFD enhances the immunosuppressive tumor microenvironment by limiting the recruitment of tumor-infiltrating myeloid-derived suppressor cells (MDSCs) (Wang et al., 2022). PFD targets CAFs, inhibiting EMT and stemness features in breast cancer cells. In breast cancer samples with a high stromal index, CAFs promote cancer cell spheroid formation and induce the expression of YAP1, VIM, and CD44. PFD treatment significantly reduces cancer cell migration and the protein expression levels of these genes (Es et al., 2021). PFD inhibits the expression of CAFs, hyaluronic acid, and collagen I, reducing tumor stromal pressure, eliminating the immunosuppressive microenvironment, and increasing cytotoxic T lymphocyte infiltration, thereby remodeling the desmoplastic tumor microenvironment. Moreover, PFD, in combination with therapies targeting the mitochondrial ROS-PYK2 pathway, significantly inhibits the growth and metastasis of malignant breast cancer (Zuo et al., 2021). PFD effectively eliminates the ethanol-mediated promotion of the TGF-β/RUNX3/Snail axis in CRC metastasis by specifically blocking the TGF-β signaling pathway (Zheng et al., 2019).
5.2.2 Sensitization to chemotherapy and immunotherapy
In chemotherapy, PFD can significantly enhance tumor cell death. PFD can reprogram several biological pathways, inhibiting tumor cell secretion of PDGF by downregulating the TGM2/NF-kB/PDGFB pathway, thus exerting antifibrotic effects. This leads to a reduction in collagen X and fibronectin secretion by CAFs, and in a mouse pancreatic tumor orthotopic model, PFD showed the potential to enhance gemcitabine sensitivity (Lei et al., 2024). PFD’s use in NSCLC primarily focuses on its antitumor and chemosensitizing effects. PFD exerts anticancer effects by inhibiting the TGF-β1 signaling pathway, reducing lactate and ATP production, and thus inhibiting glycolysis. When combined with cisplatin, PFD enhances the targeted inhibition of TGF-β1, improving chemotherapy sensitivity in A549 and H1299 cells (Zhang S. et al., 2024). In TNBC, PFD inhibits the TGF-β/Smad signaling pathway, reducing the expression of EMT-related transcription factors and mesenchymal genes, inhibiting breast cancer cell proliferation, migration, and invasion, and promoting apoptosis. Additionally, although PFD has a relatively mild standalone antitumor effect in vivo, its combination with nab-paclitaxel (nab-PTX) significantly enhances the anticancer effect in TNBC (Luo et al., 2023).
PFD demonstrates significant potential when combined with immunotherapy. When combined with PD-L1 inhibitors, PFD significantly delays tumor growth, improves survival rates, enhances both innate and adaptive immune responses, increases immune cell infiltration, and optimizes T cell localization. This combination therapy also effectively alleviates lung fibrosis and reduces tumor growth (Qin et al., 2020). In bladder cancer, the combination of PD-L1 inhibitors and PFD can significantly inhibit bladder cancer progression, potentially by modulating the tumor immune microenvironment and inhibiting tumor cell epithelial-mesenchymal transition (Chen et al., 2024).
5.2.3 Targeted drug delivery increases therapeutic efficacy
In pancreatic cancer, PFD combined with miR-138-5p, delivered through targeted engineered exosomes, successfully reprogrammed CAFs, inhibiting their pro-tumor effects. The combination inhibited the TGF-β signaling pathway and collagen synthesis, significantly improving the TME, reducing tumor pressure, enhancing the penetration of the chemotherapeutic drug gemcitabine, and increasing the sensitivity of cancer cells to chemotherapy (Zhou et al., 2024). In Jia et al. (2024)’s study, cell membrane-fused liposomes were used for targeted delivery of PFD and doxorubicin to inhibit CAF activity and remodel the TME, thereby significantly enhancing chemotherapy efficacy in TNBC. Furthermore, the optimized delivery strategy amplified the effects of anti-PD-L1 immunotherapy (Jia et al., 2024). Targeted drug delivery provides new insights for precision medicine in clinical practice.
5.3 Galunisertib
5.3.1 Clinical trials
Galunisertib is a selective inhibitor of TGF-β receptor type I (ALK5), capable of blocking TGF-β signaling, inhibiting tumor growth and metastasis, and demonstrating potential in the treatment of malignant tumors. In a trial evaluating Galunisertib combined with nivolumab for NSCLC treatment, patients received Galunisertib (150 mg, 14 days on/14 days off) along with nivolumab (3 mg/kg IV every 2 weeks). 24% of patients showed confirmed partial responses, and 16% of patients exhibited stable disease. The median progression-free survival was 5.26 months, and the median overall survival was 11.99 months. The response rate for locally advanced NSCLC is generally low, about 10%–20%, suggesting that this drug may partially increase patient survival rates (Nadal et al., 2023). In another study, patients with locally advanced rectal cancer received Galunisertib-containing neoadjuvant chemoradiotherapy, resulting in an increase in the complete response rate to 32% with good tolerability, markedly increases the complete response rate compared to the previous treatment regimen (Yamazaki et al., 2022). Galunisertib has demonstrated potential in increasing complete response rates clinically, and its efficacy deserves further evaluation in randomized trials.
5.3.2 Sensitization to chemotherapy and immunotherapy
Fibrosis forms a physical barrier and also creates an immunosuppressive microenvironment by secreting multiple cytokines. Anti-TGF-β drugs reduce fibrosis and can partially relieve this immunosuppression, promoting the infiltration of T cells and other immune effector cells into the tumor area. In OSCC, Galunisertib downregulates TGF-β signaling, enhances CD8+ T cell activity, and improves the efficacy of anti-PD-1 immunotherapy (Tao et al., 2024). In aggressive B-cell non-Hodgkin lymphoma (B-NHL), Galunisertib promotes immune system activation, reduces detrimental Treg cells, and prevents CD8+ T cell exhaustion (Rej et al., 2023). In PDAC, Galunisertib combined with dual immune checkpoint inhibitors (anti-PD-L1 and CTLA-4) significantly inhibits tumor growth and induces the infiltration of antitumor M1 macrophages. Additionally, it can enhance the immune system’s tumor-attacking ability by reducing the number of tumor-associated immunosuppressive cells (Rana et al., 2022). Galunisertib combined with IL-15-activated dendritic cells significantly enhances immunotherapy efficacy in highly invasive and metastatic mouse lymphoma. This combination therapy improves prognosis by inhibiting Treg cells in tumor-draining lymph nodes and spleen and through the inactivation of p-SMAD2 and Neuropilin-1 (Hira et al., 2020).
Galunisertib, when combined with chemotherapy drugs, enhances therapeutic efficacy. In B-NHL, Galunisertib enhances the antiproliferative and pro-apoptotic effects of doxorubicin and further inhibits tumor growth by upregulating p-P38 MAPK and inhibiting the TGF-β/Smad2/3 and PI3K/AKT signaling pathways (Rej et al., 2023).
5.4 Tranilast
Tranilast is an anti-allergic medication originally used to treat allergic conditions such as bronchial asthma, allergic rhinitis, and eczema. However, as research has advanced, Tranilast has demonstrated potential in the treatment of fibrosis-related diseases and certain cancers by inhibiting fibroblast activation, reducing malignant tumor resistance, and decreasing tumor proliferation. For instance, in CRC, Tranilast inhibits tumor growth by reducing tumor size, fibrosis, and angiogenesis. When combined with 5-FU, Tranilast further enhances the antitumor effect, leading to increased ROS production, decreased collagen deposition, and enhanced tumor necrosis (Hashemzehi et al., 2021).
5.4.1 Tranilast impacts CAF function
Tranilast inhibits the migration of M2 macrophages by suppressing CXCL12 secretion by CAFs, while also inhibiting tumor growth, fibrosis, and the infiltration of M2 macrophages and mast cells. Additionally, it significantly promotes CD8+ lymphocyte infiltration into the tumor, thereby inducing cancer cell apoptosis via immune response (Nakamura et al., 2022). In NSCLC, Tranilast inhibits IL-6 secretion by CAFs, blocks CAF-induced upregulation of the STAT3 signaling pathway, reduces EMT, and reverses CAF-mediated resistance of NSCLC to osimertinib/selumetinib (Ochi et al., 2022). Furthermore, Tranilast, by inhibiting CAF activity, prevents them from promoting the survival and radioresistance of nasopharyngeal carcinoma cells via the IL-8/NF-κB pathway following radiotherapy (Huang W.-C. et al., 2021).
5.4.2 Tranilast suppresses the TGF-β signaling pathway
In lung cancer, Tranilast inhibits TGF-β1-induced EMT and cell invasion by suppressing Smad4 expression, leading to reduced pleural dissemination of cancer cells (Takahashi et al., 2020). In breast cancer, Tranilast modulates the TGF-β signaling pathway by increasing AKT1 phosphorylation and reducing ERK1/2 phosphorylation, causing cell cycle arrest after the G1/S phase. Additionally, Tranilast upregulates p53, induces PARP cleavage, promotes tumor cell apoptosis, and modulates cell migration and invasion by inhibiting TGF-β (Subramaniam et al., 2010).
5.4.3 Tranilast enhances the TME
Tranilast combined with Doxil treatment normalizes the TNBC TME by significantly reducing ECM components, increasing tumor blood vessel diameter and pericyte coverage, and improving tumor perfusion and oxygenation. These changes enhanced the antitumor immune response and improved therapeutic efficacy. Additionally, Tranilast restored T cell infiltration and reduced the migration of T cells away from immunosuppressive CAFs. The combination of Tranilast and Doxil also significantly increased the levels of immunostimulatory M1 macrophages in tumor tissue, enhancing the efficacy of immune checkpoint inhibitors (such as anti-PD-1/anti-CTLA-4) (Panagi et al., 2020).
6 Conclusion and outlook
In conclusion, as research deepens, the interaction between fibrosis and malignant tumors has received increasing attention. Fibrosis is not merely a consequence of tumor development but plays a crucial role in tumor progression, resistance to therapy, and immune evasion. Fibrosis facilitates tumor cell proliferation, migration, and invasion by altering the composition and structure of the extracellular matrix, while also offering a protective niche for tumor cells to evade immune surveillance. Additionally, fibrosis is closely linked to the activation of tumor-associated fibroblasts, which secrete various cytokines and growth factors, further exacerbating the malignancy of tumors.
Strategies targeting fibrosis in tumor treatment exhibit broad prospects, as fibrosis plays a key role in tumor progression and drug resistance. Inhibiting fibrosis-related signaling pathways, such as TGF-β, can not only suppress tumor cell proliferation and metastasis but also enhance the effects of chemotherapy, targeted therapy, and immunotherapy. For instance, TGF-β inhibitors can decrease fibrosis, improve drug permeability, and increase treatment effectiveness. Moreover, drugs targeting CAFs in the tumor microenvironment have demonstrated potential in preclinical research (Conte, 2022).
In the future, anti-fibrosis therapies are likely to become a crucial part of cancer treatment, especially when combined with current therapies, providing new options for patients with difficult-to-treat tumors. Through ongoing research, anti-fibrosis strategies will offer crucial support in enhancing treatment outcomes and improving patients’ quality of life.
Author contributions
HC: Conceptualization, Writing–original draft. XX: Conceptualization, Writing–review and editing. JL: Writing–original draft. YX: Writing–review and editing. XnL: Writing–review and editing. KZ: Writing–review and editing. HJ: Writing–review and editing. XaL: Writing–review and editing. ML: Writing–review and editing, Funding acquisition.
Funding
The author(s) declare that financial support was received for the research, authorship, and/or publication of this article. This work was supported by grants from the National College Student Innovation and Entrepreneurship. Training Program Project (202210488001), Provincial College Student Innovation and Entrepreneurship Training Program Project (S202410488175).
Acknowledgments
Thanks to the researchers who published relevant papers. We would like to thank the academic editor and reviewers for their important contributions that improved the quality of this article. Thanks to the language polishing service provided by ChatGPT 4.
Conflict of interest
The authors declare that the research was conducted in the absence of any commercial or financial relationships that could be construed as a potential conflict of interest.
Publisher’s note
All claims expressed in this article are solely those of the authors and do not necessarily represent those of their affiliated organizations, or those of the publisher, the editors and the reviewers. Any product that may be evaluated in this article, or claim that may be made by its manufacturer, is not guaranteed or endorsed by the publisher.
References
Adhikari, S., Singh, V., Nandi, S., Ghosal, M., Raj, N. S., Khanna, J., et al. (2024). UBR7 in concert with EZH2 inhibits the TGF-β signaling leading to extracellular matrix remodeling. Cell Rep. 43, 114394. doi:10.1016/j.celrep.2024.114394
Alhadeethi, A., Adel Awwad, S., Abed, M., Amin, A. M., Aboelkhier, M. M., Yassin, M. N. A., et al. (2024). Nintedanib in combination with chemotherapy in the treatment of non-small cell lung cancer: a systematic review and meta-analysis. Cureus 16, e53812. doi:10.7759/cureus.53812
Al-Kharashi, L. A., Tulbah, A., Arafah, M., Eldali, A. M., Al-Tweigeri, T., and Aboussekhra, A. (2022). High DNMT1 expression in stromal fibroblasts promotes angiogenesis and Unfavorable outcome in locally advanced breast cancer patients. Front. Oncol. 12, 877219. doi:10.3389/fonc.2022.877219
Ambrosetti, D., Coutts, M., Paoli, C., Durand, M., Borchiellini, D., Montemagno, C., et al. (2022). Cancer-associated fibroblasts in renal cell carcinoma: implication in prognosis and resistance to anti-angiogenic therapy. BJU Int. 129, 80–92. doi:10.1111/bju.15506
Anderson-Crannage, M., Ascensión, A. M., Ibanez-Solé, O., Zhu, H., Schaefer, E., Ottomanelli, D., et al. (2023). Inflammation-mediated fibroblast activation and immune dysregulation in collagen VII-deficient skin. Front. Immunol. 14, 1211505. doi:10.3389/fimmu.2023.1211505
Auberle, C., Gao, F., Sloan, M., Morgensztern, D., Winkler, L., Ward, J. P., et al. (2024). A pilot study of nintedanib in molecularly selected patients with advanced non-small cell lung cancer. J. Thorac. Dis. 16, 3782–3793. doi:10.21037/jtd-23-1717
Auliac, J.-B., Monnet, I., Bizieux, A., Greillier, L., Geier, M., Falchero, L., et al. (2021). Multicenter phase II trial of nintedanib plus docetaxel in second-line treatment in advanced non-squamous non-small cell lung cancer patients refractory to first-line platin-based chemotherapy (REFRACT GFPC 02-15 study). Lung Cancer 161, 122–127. doi:10.1016/j.lungcan.2021.09.007
Awasthi, N., Schwarz, M. A., Kaurich, Q., Zhang, C., Hilberg, F., and Schwarz, R. E. (2023). Enhancing gastric cancer conventional chemotherapy effects by triple angiokinase inhibitor nintedanib in preclinical models. Front. Oncol. 13, 1145999. doi:10.3389/fonc.2023.1145999
Baik, J. E., Park, H. J., Kataru, R. P., Savetsky, I. L., Ly, C. L., Shin, J., et al. (2022). TGF-β1 mediates pathologic changes of secondary lymphedema by promoting fibrosis and inflammation. Clin. Transl. Med. 12, e758. doi:10.1002/ctm2.758
Ben Baruch, B., Mantsur, E., Franco-Barraza, J., Blacher, E., Cukierman, E., and Stein, R. (2020). CD38 in cancer-associated fibroblasts promotes pro-tumoral activity. Lab. Invest 100, 1517–1531. doi:10.1038/s41374-020-0458-8
Bläuer, M., Laaninen, M., Sand, J., and Laukkarinen, J. (2019). Wnt/β-catenin signalling plays diverse functions during the process of fibrotic remodelling in the exocrine pancreas. Pancreatology 19, 252–257. doi:10.1016/j.pan.2019.02.003
Boland, P. M., Ebos, J. M. L., Attwood, K., Mastri, M., Fountzilas, C., Iyer, R. V., et al. (2024). A phase I/II study of nintedanib and capecitabine for refractory metastatic colorectal cancer. JNCI Cancer Spectr. 8, pkae017. doi:10.1093/jncics/pkae017
Bou Malham, V., Benzoubir, N., Vaquero, J., Desterke, C., Agnetti, J., Song, P. X., et al. (2023). Intrinsic cancer cell phosphoinositide 3-kinase δ regulates fibrosis and vascular development in cholangiocarcinoma. Liver Int. 43, 2776–2793. doi:10.1111/liv.15751
Bray, F., Laversanne, M., Sung, H., Ferlay, J., Siegel, R. L., Soerjomataram, I., et al. (2024). Global cancer statistics 2022: GLOBOCAN estimates of incidence and mortality worldwide for 36 cancers in 185 countries. CA A Cancer J. Clin. 74, 229–263. doi:10.3322/caac.21834
Cabrera-Galván, J. J., Araujo, E., de Mirecki-Garrido, M., Pérez-Rodríguez, D., Guerra, B., Aranda-Tavío, H., et al. (2023). SOCS2 protects against chemical-induced hepatocellular carcinoma progression by modulating inflammation and cell proliferation in the liver. Biomed. Pharmacother. 157, 114060. doi:10.1016/j.biopha.2022.114060
Chaker, D., Desterke, C., Moniaux, N., Bani, M.-A., Oudrhiri, N., Faivre, J., et al. (2024). Direct reprogramming of hepatocytes into JAK/Stat-Dependent LGR5+ liver cells able to initiate intrahepatic cholangiocarcinoma. Stem Cells 42, 301–316. doi:10.1093/stmcls/sxae006
Chandra Jena, B., Kanta Das, C., Banerjee, I., Das, S., Bharadwaj, D., Majumder, R., et al. (2021). Paracrine TGF-β1 from breast cancer contributes to chemoresistance in cancer associated fibroblasts via upregulation of the p44/42 MAPK signaling pathway. Biochem. Pharmacol. 186, 114474. doi:10.1016/j.bcp.2021.114474
Chen, S., Zhang, S., Fan, W., Sun, W., Liu, B., Liu, J., et al. (2024). Efficacy of combined treatment with pirfenidone and PD-L1 inhibitor in mice bearing ectopic bladder cancer xenograft. Nan Fang. Yi Ke Da Xue Xue Bao 44, 210–216. doi:10.12122/j.issn.1673-4254.2024.02.02
Chu, Y., Fang, Y., Wu, H., Chen, J., and Cheng, L. (2022). Vocal fold fibroblasts promote angiogenesis in vocal fold leukoplakia by secreting pro-angiogenic factors. Auris Nasus Larynx 49, 1009–1018. doi:10.1016/j.anl.2022.04.009
Conte, E. (2022). Targeting monocytes/macrophages in fibrosis and cancer diseases: therapeutic approaches. Pharmacol. Ther. 234, 108031. doi:10.1016/j.pharmthera.2021.108031
Dai, X., Xie, Y., and Dong, M. (2022). Cancer-associated fibroblasts derived extracellular vesicles promote angiogenesis of colorectal adenocarcinoma cells through miR-135b-5p/FOXO1 axis. Cancer Biol. Ther. 23, 76–88. doi:10.1080/15384047.2021.2017222
De Marco, M., Del Papa, N., Reppucci, F., Iorio, V., Basile, A., Falco, A., et al. (2022). BAG3 induces α-SMA expression in human fibroblasts and its over-expression correlates with poorer survival in fibrotic cancer patients. J. Cell Biochem. 123, 91–101. doi:10.1002/jcb.30171
Deng, B., Zhao, Z., Kong, W., Han, C., Shen, X., and Zhou, C. (2022). Biological role of matrix stiffness in tumor growth and treatment. J. Transl. Med. 20, 540. doi:10.1186/s12967-022-03768-y
Ding, L., Wu, L., Cao, Y., Wang, H., Li, D., Chen, W., et al. (2024). Modulating tumor-associated macrophage polarization by anti-maRCO mAb exerts anti-osteosarcoma effects through regulating osteosarcoma cell proliferation, migration and apoptosis. J. Orthop. Surg. Res. 19, 453. doi:10.1186/s13018-024-04950-2
Du, R., Zhang, X., Lu, X., Ma, X., Guo, X., Shi, C., et al. (2023). PDPN positive CAFs contribute to HER2 positive breast cancer resistance to trastuzumab by inhibiting antibody-dependent NK cell-mediated cytotoxicity. Drug Resist Updat 68, 100947. doi:10.1016/j.drup.2023.100947
Dy, S., Jq, W., Zh, H., Mf, H., and Hb, S. (2019). Cancer-associated fibroblast regulate proliferation and migration of prostate cancer cells through TGF-β signaling pathway. Life Sci. 235, 116791. doi:10.1016/j.lfs.2019.116791
Erstad, D. J., Sojoodi, M., Taylor, M. S., Ghoshal, S., Razavi, A. A., Graham-O’Regan, K. A., et al. (2018). Orthotopic and heterotopic murine models of pancreatic cancer and their different responses to FOLFIRINOX chemotherapy. Dis. Model Mech. 11, dmm034793. doi:10.1242/dmm.034793
Es, H. A., Cox, T. R., Sarafraz-Yazdi, E., Thiery, J. P., and Warkiani, M. E. (2021). Pirfenidone reduces epithelial-mesenchymal transition and spheroid formation in breast carcinoma through targeting cancer-associated fibroblasts (CAFs). Cancers (Basel) 13, 5118. doi:10.3390/cancers13205118
Ettrich, T. J., Perkhofer, L., Decker, T., Hofheinz, R.-D., Heinemann, V., Hoffmann, T., et al. (2021). Nintedanib plus mFOLFOX6 as second-line treatment of metastatic, chemorefractory colorectal cancer: the randomised, placebo-controlled, phase II TRICC-C study (AIO-KRK-0111). Int. J. Cancer 148, 1428–1437. doi:10.1002/ijc.33296
Eun, J. W., Yoon, J. H., Ahn, H. R., Kim, S., Kim, Y. B., Lim, S. B., et al. (2023). Cancer-associated fibroblast-derived secreted phosphoprotein 1 contributes to resistance of hepatocellular carcinoma to sorafenib and lenvatinib. Cancer Commun. (Lond) 43, 455–479. doi:10.1002/cac2.12414
Fan, J., Xu, G., Chang, Z., Zhu, L., and Yao, J. (2020). miR-210 transferred by lung cancer cell-derived exosomes may act as proangiogenic factor in cancer-associated fibroblasts by modulating JAK2/STAT3 pathway. Clin. Sci. (Lond) 134, 807–825. doi:10.1042/CS20200039
Feng, Y., Ren, J., Gui, Y., Wei, W., Shu, B., Lu, Q., et al. (2018). Wnt/β-Catenin-Promoted macrophage alternative activation contributes to kidney fibrosis. J. Am. Soc. Nephrol. 29, 182–193. doi:10.1681/ASN.2017040391
Feng, Z., Tianli, Y., Liuhua, Z., R, L., J, L., J, Z., et al. (2023). Sig1R activates extracellular matrix-induced bladder cancer cell proliferation and angiogenesis by combing β-integrin. Aging 15, 4182–4201. doi:10.18632/aging.204721
Ferron, G., De Rauglaudre, G., Becourt, S., Delanoy, N., Joly, F., Lortholary, A., et al. (2023). Neoadjuvant chemotherapy with or without nintedanib for advanced epithelial ovarian cancer: lessons from the GINECO double-blind randomized phase II CHIVA trial. Gynecol. Oncol. 170, 186–194. doi:10.1016/j.ygyno.2023.01.008
Galindo-Pumariño, C., Collado, M., Castillo, M. E., Barquín, J., Romio, E., Larriba, M. J., et al. (2022). SNAI1-expressing fibroblasts and derived-extracellular matrix as mediators of drug resistance in colorectal cancer patients. Toxicol. Appl. Pharmacol. 450, 116171. doi:10.1016/j.taap.2022.116171
Geng, X., Chen, H., Zhao, L., Hu, J., Yang, W., Li, G., et al. (2021). Cancer-associated fibroblast (CAF) heterogeneity and targeting therapy of CAFs in pancreatic cancer. Front. Cell Dev. Biol. 9, 655152. doi:10.3389/fcell.2021.655152
Giarratana, A. O., Prendergast, C. M., Salvatore, M. M., and Capaccione, K. M. (2024). TGF-β signaling: critical nexus of fibrogenesis and cancer. J. Transl. Med. 22, 594. doi:10.1186/s12967-024-05411-4
Grohmann, M., Wiede, F., Dodd, G. T., Gurzov, E. N., Ooi, G. J., Butt, T., et al. (2018). Obesity drives STAT-1-dependent NASH and STAT-3-dependent HCC. Cell 175, 1289–1306. doi:10.1016/j.cell.2018.09.053
Guardia, C., Bianchini, G., Arpí-Llucià, O., Menendez, S., Casadevall, D., Galbardi, B., et al. (2021). Preclinical and clinical characterization of fibroblast-derived neuregulin-1 on trastuzumab and pertuzumab activity in HER2-positive breast cancer. Clin. Cancer Res. 27, 5096–5108. doi:10.1158/1078-0432.CCR-20-2915
Hamdy, H., Yang, Y., Cheng, C., and Liu, Q. (2023). Identification of potential hub genes related to aflatoxin B1, liver fibrosis and hepatocellular carcinoma via integrated bioinformatics analysis. Biol. (Basel) 12, 205. doi:10.3390/biology12020205
Hanahan, D., and Weinberg, R. A. (2011). Hallmarks of cancer: the next generation. Cell 144, 646–674. doi:10.1016/j.cell.2011.02.013
Hashemzehi, M., Yavari, N., Rahmani, F., Asgharzadeh, F., Soleimani, A., Shakour, N., et al. (2021). Inhibition of transforming growth factor-beta by Tranilast reduces tumor growth and ameliorates fibrosis in colorectal cancer. EXCLI J. 20, 601–613. doi:10.17179/excli2020-2932
Hata, T., Kawamoto, K., Eguchi, H., Kamada, Y., Takamatsu, S., Maekawa, T., et al. (2017). Fatty acid-mediated stromal reprogramming of pancreatic stellate cells induces inflammation and fibrosis that fuels pancreatic cancer. Pancreas 46, 1259–1266. doi:10.1097/MPA.0000000000000943
He, X., Xu, C., Wu, X., Wang, M., Guo, Y., Zhang, W., et al. (2020). Expression and methylation of Dickkopf-1 in the pathogenesis and malignant transformation of oral submucous fibrosis. J. Oral Pathology and Med. 49, 809–815. doi:10.1111/jop.13096
Herzog, B. H., Baer, J. M., Borcherding, N., Kingston, N. L., Belle, J. I., Knolhoff, B. L., et al. (2023). Tumor-associated fibrosis impairs immune surveillance and response to immune checkpoint blockade in non-small cell lung cancer. Sci. Transl. Med. 15, eadh8005. doi:10.1126/scitranslmed.adh8005
Hira, S. K., Rej, A., Paladhi, A., Singh, R., Saha, J., Mondal, I., et al. (2020). Galunisertib drives Treg fragility and promotes dendritic cell-mediated immunity against experimental lymphoma. iScience 23, 101623. doi:10.1016/j.isci.2020.101623
Hirsch, F. R., Scagliotti, G. V., Mulshine, J. L., Kwon, R., Curran, W. J., Wu, Y.-L., et al. (2017). Lung cancer: current therapies and new targeted treatments. Lancet 389, 299–311. doi:10.1016/S0140-6736(16)30958-8
Hu, J. L., Wang, W., Lan, X. L., Zeng, Z. C., Liang, Y. S., Yan, Y. R., et al. (2019). CAFs secreted exosomes promote metastasis and chemotherapy resistance by enhancing cell stemness and epithelial-mesenchymal transition in colorectal cancer. Mol. Cancer 18, 91. doi:10.1186/s12943-019-1019-x
Huang, W., Zhang, L., Yang, M., Wu, X., Wang, X., Huang, W., et al. (2021). Cancer-associated fibroblasts promote the survival of irradiated nasopharyngeal carcinoma cells via the NF-κB pathway. J. Exp. Clin. Cancer Res. 40, 87. doi:10.1186/s13046-021-01878-x
Huang, W.-C., Yadav, V. K., Cheng, W.-H., Wang, C.-H., Hsieh, M.-S., Huang, T.-Y., et al. (2021). The MEK/ERK/miR-21 signaling is critical in osimertinib resistance in EGFR-mutant non-small cell lung cancer cells. Cancers (Basel) 13, 6005. doi:10.3390/cancers13236005
Hung, C.-C., Zhen, Y.-Y., Niu, S.-W., Hsu, J.-F., Lee, T.-H., Chuang, H.-H., et al. (2020). Lung cancer cell-derived secretome mediates paraneoplastic inflammation and fibrosis in kidney in mice. Cancers (Basel) 12, 3561. doi:10.3390/cancers12123561
Hussain, S. A., Lester, J. F., Jackson, R., Gornall, M., Qureshi, M., Elliott, A., et al. (2022). Addition of nintedanib or placebo to neoadjuvant gemcitabine and cisplatin in locally advanced muscle-invasive bladder cancer (NEOBLADE): a double-blind, randomised, phase 2 trial. Lancet Oncol. 23, 650–658. doi:10.1016/S1470-2045(22)00158-9
Ikeda, S., Ogura, T., Kato, T., Kenmotsu, H., Agemi, Y., Tokito, T., et al. (2024). Nintedanib plus chemotherapy for small cell lung cancer with comorbid idiopathic pulmonary fibrosis. Ann. Am. Thorac. Soc. 21, 635–643. doi:10.1513/AnnalsATS.202311-941OC
Inoue, C., Miki, Y., Saito, R., Hata, S., Abe, J., Sato, I., et al. (2019). PD-L1 induction by cancer-associated fibroblast-derived factors in lung adenocarcinoma cells. Cancers (Basel) 11, 1257. doi:10.3390/cancers11091257
Jia, N., Wang, Q., Li, W., Chen, D., and Hu, H. (2024). Membrane fusion liposomes deliver antifibrotic and chemotherapeutic drugs sequentially to enhance tumor treatment efficacy by reshaping tumor microenvironment. Adv. Healthc. Mater 13, e2400219. doi:10.1002/adhm.202400219
Jin, Z., Lu, Y., Wu, X., Pan, T., Yu, Z., Hou, J., et al. (2021). The cross-talk between tumor cells and activated fibroblasts mediated by lactate/BDNF/TrkB signaling promotes acquired resistance to anlotinib in human gastric cancer. Redox Biol. 46, 102076. doi:10.1016/j.redox.2021.102076
Johansson, A.-C., Ansell, A., Jerhammar, F., Lindh, M. B., Grénman, R., Munck-Wikland, E., et al. (2012). Cancer-associated fibroblasts induce matrix metalloproteinase-mediated cetuximab resistance in head and neck squamous cell carcinoma cells. Mol. Cancer Res. 10, 1158–1168. doi:10.1158/1541-7786.MCR-12-0030
Ke, M.-Y., Xu, T., Fang, Y., Ye, Y.-P., Li, Z.-J., Ren, F.-G., et al. (2021). Liver fibrosis promotes immune escape in hepatocellular carcinoma via GOLM1-mediated PD-L1 upregulation. Cancer Lett. 513, 14–25. doi:10.1016/j.canlet.2021.05.007
Kwon, M., Rubio, G., Wang, H., Riedlinger, G., Adem, A., Zhong, H., et al. (2022). Smoking-associated downregulation of FILIP1L enhances lung adenocarcinoma progression through mucin production, inflammation, and fibrosis. Cancer Res. Commun. 2, 1197–1213. doi:10.1158/2767-9764.CRC-22-0233
Lai, K. K. Y., Kweon, S.-M., Chi, F., Hwang, E., Kabe, Y., Higashiyama, R., et al. (2017). Stearoyl-CoA desaturase promotes liver fibrosis and tumor development in mice via a Wnt positive-signaling loop by stabilization of low-density lipoprotein-receptor-related proteins 5 and 6. Gastroenterology 152, 1477–1491. doi:10.1053/j.gastro.2017.01.021
Lai, T.-Y., Chiang, T.-C., Lee, C.-Y., Kuo, T.-C., Wu, C.-H., Chen, Y.-I., et al. (2024). Unraveling the impact of cancer-associated fibroblasts on hypovascular pancreatic neuroendocrine tumors. Br. J. Cancer 130, 1096–1108. doi:10.1038/s41416-023-02565-8
Leboulleux, S., Kapiteijn, E., Litière, S., Schöffski, P., Godbert, Y., Rodien, P., et al. (2024). Safety and efficacy of nintedanib as second-line therapy for patients with differentiated or medullary thyroid cancer progressing after first-line therapy. A randomized phase II study of the EORTC Endocrine Task Force (protocol 1209-EnTF). Front. Endocrinol. (Lausanne) 15, 1403687. doi:10.3389/fendo.2024.1403687
Lee, C. M., Hwang, Y., Jeong, J. W., Kim, M., Lee, J., Bae, S. J., et al. (2024). BRCA1 mutation promotes sprouting angiogenesis in inflammatory cancer-associated fibroblast of triple-negative breast cancer. Cell Death Discov. 10, 5. doi:10.1038/s41420-023-01768-5
Lee, J. H., and Massagué, J. (2022). TGF-β in developmental and fibrogenic EMTs. Semin. Cancer Biol. 86, 136–145. doi:10.1016/j.semcancer.2022.09.004
Lee, Y. E., Go, G.-Y., Koh, E.-Y., Yoon, H.-N., Seo, M., Hong, S.-M., et al. (2023). Synergistic therapeutic combination with a CAF inhibitor enhances CAR-NK-mediated cytotoxicity via reduction of CAF-released IL-6. J. Immunother. Cancer 11, e006130. doi:10.1136/jitc-2022-006130
Lei, Y., Xu, J., Xiao, M., Wu, D., Xu, H., Yang, J., et al. (2024). Pirfenidone alleviates fibrosis by acting on tumour-stroma interplay in pancreatic cancer. Br. J. Cancer 130, 1505–1516. doi:10.1038/s41416-024-02631-9
Li, H., Xu, M., Chen, D., Wen, W., and Luo, J. (2024). Pirfenidone ameliorates alcohol-induced promotion of breast cancer in mice. Front. Oncol. 14, 1351839. doi:10.3389/fonc.2024.1351839
Li, J., Liu, X., Zang, S., Zhou, J., Zhang, F., Sun, B., et al. (2020). Small extracellular vesicle-bound vascular endothelial growth factor secreted by carcinoma-associated fibroblasts promotes angiogenesis in a bevacizumab-resistant manner. Cancer Lett. 492, 71–83. doi:10.1016/j.canlet.2020.08.030
Li, J., Wu, X., Ni, X., Li, Y., Xu, L., Hao, X., et al. (2023). Angiotensin receptor blockers retard the progression and fibrosis via inhibiting the viability of AGTR1+CAFs in intrahepatic cholangiocarcinoma. Clin. Transl. Med. 13, e1213. doi:10.1002/ctm2.1213
Li, J., Zou, Y., Kantapan, J., Su, H., Wang, L., and Dechsupa, N. (2024). TGF-β/Smad signaling in chronic kidney disease: exploring post-translational regulatory perspectives (Review). Mol. Med. Rep. 30, 143. doi:10.3892/mmr.2024.13267
Li, T., Liu, J., Wang, Y., Zhou, C., Shi, Q., Huang, S., et al. (2021). Liver fibrosis promotes immunity escape but limits the size of liver tumor in a rat orthotopic transplantation model. Sci. Rep. 11, 22846. doi:10.1038/s41598-021-02155-9
Li, W., Wu, F., Zhao, S., Shi, P., Wang, S., and Cui, D. (2022). Correlation between PD-1/PD-L1 expression and polarization in tumor-associated macrophages: a key player in tumor immunotherapy. Cytokine Growth Factor Rev. 67, 49–57. doi:10.1016/j.cytogfr.2022.07.004
Li, X., Jiang, E., Zhao, H., Chen, Y., Xu, Y., Feng, C., et al. (2022). Glycometabolic reprogramming-mediated proangiogenic phenotype enhancement of cancer-associated fibroblasts in oral squamous cell carcinoma: role of PGC-1α/PFKFB3 axis. Br. J. Cancer 127, 449–461. doi:10.1038/s41416-022-01818-2
Lima, J. D. C. C., Simoes, E., de Castro, G., Morais, M. R. P. T., de Matos-Neto, E. M., Alves, M. J., et al. (2019). Tumour-derived transforming growth factor-β signalling contributes to fibrosis in patients with cancer cachexia. J. Cachexia Sarcopenia Muscle 10, 1045–1059. doi:10.1002/jcsm.12441
Lin, H.-W., Chiang, Y.-C., Sun, N.-Y., Chen, Y.-L., Chang, C.-F., Tai, Y.-J., et al. (2019). CHI3L1 results in poor outcome of ovarian cancer by promoting properties of stem-like cells. Endocr. Relat. Cancer 26, 73–88. doi:10.1530/ERC-18-0300
Liu, J., Cao, L., Li, Y., Deng, P., Pan, P., Hu, C., et al. (2022). Pirfenidone promotes the levels of exosomal miR-200 to down-regulate ZEB1 and represses the epithelial-mesenchymal transition of non-small cell lung cancer cells. Hum. Cell 35, 1813–1823. doi:10.1007/s13577-022-00766-6
Liu, J., Wang, F., and Luo, F. (2023). The role of JAK/STAT pathway in fibrotic diseases: molecular and cellular mechanisms. Biomolecules 13, 119. doi:10.3390/biom13010119
Liu, X., Li, J., Yang, X., Li, X., Kong, J., Qi, D., et al. (2023). Carcinoma-associated fibroblast-derived lysyl oxidase-rich extracellular vesicles mediate collagen crosslinking and promote epithelial-mesenchymal transition via p-FAK/p-paxillin/YAP signaling. Int. J. Oral Sci. 15, 32. doi:10.1038/s41368-023-00236-1
Liu, Y., Qu, H.-C., and Huang, Y. (2023). Renal clear cell carcinoma-derived CXCL5 drives tumor-associated fibroblast formation and facilitates cancer progression. Pathol. Res. Pract. 244, 154319. doi:10.1016/j.prp.2023.154319
Li Z., Z., Chen, Z., Li, S., Qian, X., Zhang, L., Long, G., et al. (2023). Circ_0020256 induces fibroblast activation to drive cholangiocarcinoma development via recruitment of EIF4A3 protein to stabilize KLF4 mRNA. Cell Death Discov. 9, 161. doi:10.1038/s41420-023-01439-5
Ljubicic, L., Janzic, U., Unk, M., Terglav, A. S., Mohorcic, K., Seiwerth, F., et al. (2023). Efficacy and safety of nintedanib and docetaxel in patients with previously treated lung non-squamous non-small cell lung cancer: a multicenter retrospective real-world analysis. Radiol. Oncol. 57, 397–404. doi:10.2478/raon-2023-0040
Luo, D., Zeng, X., Zhang, S., Li, D., Cheng, Z., Wang, Y., et al. (2023). Pirfenidone suppressed triple-negative breast cancer metastasis by inhibiting the activity of the TGF-β/SMAD pathway. J. Cell Mol. Med. 27, 456–469. doi:10.1111/jcmm.17673
Makiguchi, T., Tanaka, H., Okudera, K., Taima, K., and Tasaka, S. (2023). Safety and feasibility of carboplatin and paclitaxel in combination with nintedanib for non-small cell lung cancer patients with idiopathic pulmonary fibrosis: a prospective pilot study. Transl. Lung Cancer Res. 12, 719–726. doi:10.21037/tlcr-22-699
Mancarella, S., Gigante, I., Serino, G., Pizzuto, E., Dituri, F., Valentini, M. F., et al. (2022). Crenigacestat blocking notch pathway reduces liver fibrosis in the surrounding ecosystem of intrahepatic CCA viaTGF-β inhibition. J. Exp. and Clin. Cancer Res. 41, 331. doi:10.1186/s13046-022-02536-6
Mao, T., Zhang, M., Peng, Z., Tang, M., Li, T., and Liang, C. (2024). Integrative analysis of ferroptosis-related genes reveals that ABHD12 is a novel prognostic biomarker and facilitates hepatocellular carcinoma tumorigenesis. Discov. Oncol. 15, 330. doi:10.1007/s12672-024-01211-w
Mao, Y., Zhang, Y., Qu, Q., Zhao, M., Lou, Y., Liu, J., et al. (2015). Cancer-associated fibroblasts induce trastuzumab resistance in HER2 positive breast cancer cells. Mol. Biosyst. 11, 1029–1040. doi:10.1039/c4mb00710g
Masamune, A., Kikuta, K., Watanabe, T., Satoh, K., Hirota, M., and Shimosegawa, T. (2008). Hypoxia stimulates pancreatic stellate cells to induce fibrosis and angiogenesis in pancreatic cancer. Am. J. Physiology-Gastrointestinal Liver Physiology 295, G709–G717. doi:10.1152/ajpgi.90356.2008
Matsumura, K., Hayashi, H., Uemura, N., Ogata, Y., Zhao, L., Sato, H., et al. (2022). Thrombospondin-1 overexpression stimulates loss of Smad4 and accelerates malignant behavior via TGF-β signal activation in pancreatic ductal adenocarcinoma. Transl. Oncol. 26, 101533. doi:10.1016/j.tranon.2022.101533
Metcalf, K. J., Hayward, M.-K., Berens, E., Ironside, A. J., Stashko, C., Hwang, E. S., et al. (2022). Immunosuppressive glycoproteins associate with breast tumor fibrosis and aggression. Matrix Biol. Plus 14, 100105. doi:10.1016/j.mbplus.2022.100105
Mezawa, Y., Wang, T., Daigo, Y., Takano, A., Miyagi, Y., Yokose, T., et al. (2023). Glutamine deficiency drives transforming growth factor-β signaling activation that gives rise to myofibroblastic carcinoma-associated fibroblasts. Cancer Sci. 114, 4376–4387. doi:10.1111/cas.15955
Mohapatra, D., Das, B., Suresh, V., Parida, D., Minz, A. P., Nayak, U., et al. (2022). Fluvastatin sensitizes pancreatic cancer cells toward radiation therapy and suppresses radiation- and/or TGF-β-induced tumor-associated fibrosis. Lab. Investig. 102, 298–311. doi:10.1038/s41374-021-00690-7
Nadal, E., Saleh, M., Aix, S. P., Ochoa-de-Olza, M., Patel, S. P., Antonia, S., et al. (2023). A phase Ib/II study of galunisertib in combination with nivolumab in solid tumors and non-small cell lung cancer. BMC Cancer 23, 708. doi:10.1186/s12885-023-11153-1
Najafi, M., Farhood, B., and Mortezaee, K. (2019). Extracellular matrix (ECM) stiffness and degradation as cancer drivers. J. Cell Biochem. 120, 2782–2790. doi:10.1002/jcb.27681
Nakamura, Y., Kinoshita, J., Yamaguchi, T., Aoki, T., Saito, H., Hamabe-Horiike, T., et al. (2022). Crosstalk between cancer-associated fibroblasts and immune cells in peritoneal metastasis: inhibition in the migration of M2 macrophages and mast cells by Tranilast. Gastric Cancer 25, 515–526. doi:10.1007/s10120-021-01275-5
Nie, J., Wang, C., Zheng, L., Liu, Y., Wang, C., Chang, Y., et al. (2024). Epigenetic agents plus anti-PD-1 reshapes tumor microenvironment and restores antitumor efficacy in Hodgkin lymphoma. Blood, blood, 2024024487. doi:10.1182/blood.2024024487
Niture, S., Gadi, S., Lin, M., Qi, Q., Niture, S. S., Moore, J. T., et al. (2023). Cadmium modulates steatosis, fibrosis, and oncogenic signaling in liver cancer cells by activating notch and AKT/mTOR pathways. Environ. Toxicol. 38, 783–797. doi:10.1002/tox.23731
N, L., Y, L., Z, L., C, H., Y, Y., M, L., et al. (2016). Hypoxia inducible factor 1 (HIF-1) recruits macrophage to activate pancreatic stellate cells in pancreatic ductal adenocarcinoma. Int. J. Mol. Sci. 17, 799. doi:10.3390/ijms17060799
Ochi, K., Suzawa, K., Thu, Y. M., Takatsu, F., Tsudaka, S., Zhu, Y., et al. (2022). Drug repositioning of tranilast to sensitize a cancer therapy by targeting cancer-associated fibroblast. Cancer Sci. 113, 3428–3436. doi:10.1111/cas.15502
Otsubo, K., Kishimoto, J., Ando, M., Kenmotsu, H., Minegishi, Y., Horinouchi, H., et al. (2022). Nintedanib plus chemotherapy for nonsmall cell lung cancer with idiopathic pulmonary fibrosis: a randomised phase 3 trial. Eur. Respir. J. 60, 2200380. doi:10.1183/13993003.00380-2022
Panagi, M., Voutouri, C., Mpekris, F., Papageorgis, P., Martin, M. R., Martin, J. D., et al. (2020). TGF-β inhibition combined with cytotoxic nanomedicine normalizes triple negative breast cancer microenvironment towards anti-tumor immunity. Theranostics 10, 1910–1922. doi:10.7150/thno.36936
Payervand, N., Pakravan, K., Razmara, E., Vinu, K. K., Ghodsi, S., Heshmati, M., et al. (2024). Exosomal circ_0084043 derived from colorectal cancer-associated fibroblasts promotes in vitro endothelial cell angiogenesis by regulating the miR-140–3p/HIF-1α/VEGF signaling axis. Heliyon 10, e31584. doi:10.1016/j.heliyon.2024.e31584
Peng, H., Yang, M., Feng, K., Lv, Q., and Zhang, Y. (2024). Semaphorin 3C (Sema3C) reshapes stromal microenvironment to promote hepatocellular carcinoma progression. Signal Transduct. Target Ther. 9, 169. doi:10.1038/s41392-024-01887-0
Peng, Y., Li, Z., Yang, P., Newton, I. P., Ren, H., Zhang, L., et al. (2014). Direct contacts with colon cancer cells regulate the differentiation of bone marrow mesenchymal stem cells into tumor associated fibroblasts. Biochem. Biophys. Res. Commun. 451, 68–73. doi:10.1016/j.bbrc.2014.07.074
Qin, W., Zou, J., Huang, Y., Liu, C., Kang, Y., Han, H., et al. (2020). Pirfenidone facilitates immune infiltration and enhances the antitumor efficacy of PD-L1 blockade in mice. Oncoimmunology 9, 1824631. doi:10.1080/2162402X.2020.1824631
Rana, M., Kansal, R., Chaib, M., Teng, B., Morrrison, M., Hayes, D. N., et al. (2022). The pancreatic cancer immune tumor microenvironment is negatively remodeled by gemcitabine while TGF-β receptor plus dual checkpoint inhibition maintains antitumor immune cells. Mol. Carcinog. 61, 549–557. doi:10.1002/mc.23401
Rej, A., Paladhi, A., Daripa, S., Sarkar, D., Bhattacharyya, S., Mondal, I., et al. (2023). Galunisertib synergistically potentiates the doxorubicin-mediated antitumor effect and kickstarts the immune system against aggressive lymphoma. Int. Immunopharmacol. 114, 109521. doi:10.1016/j.intimp.2022.109521
Ren, X., Li, L., Wu, J., Lin, K., He, Y., and Bian, L. (2021). PDGF-BB regulates the transformation of fibroblasts into cancer-associated fibroblasts via the lncRNA LURAP1L-AS1/LURAP1L/IKK/IκB/NF-κB signaling pathway. Oncol. Lett. 22, 537. doi:10.3892/ol.2021.12798
Rimal, R., Desai, P., Daware, R., Hosseinnejad, A., Prakash, J., Lammers, T., et al. (2022). Cancer-associated fibroblasts: origin, function, imaging, and therapeutic targeting. Adv. Drug Deliv. Rev. 189, 114504. doi:10.1016/j.addr.2022.114504
Schrader, J., Gordon-Walker, T. T., Aucott, R. L., van Deemter, M., Quaas, A., Walsh, S., et al. (2011). Matrix stiffness modulates proliferation, chemotherapeutic response, and dormancy in hepatocellular carcinoma cells. Hepatology 53, 1192–1205. doi:10.1002/hep.24108
Selvarajah, B., Azuelos, I., Platé, M., Guillotin, D., Forty, E. J., Contento, G., et al. (2019). mTORC1 amplifies the ATF4-dependent de novo serine-glycine pathway to supply glycine during TGF-β1-induced collagen biosynthesis. Sci. Signal 12, eaav3048. doi:10.1126/scisignal.aav3048
Shi, A., Liu, Z., Fan, Z., Li, K., Liu, X., Tang, Y., et al. (2024). Function of mast cell and bile-cholangiocarcinoma interplay in cholangiocarcinoma microenvironment. Gut 73, 1350–1363. doi:10.1136/gutjnl-2023-331715
Shi, Y., Sun, L., Zhang, R., Hu, Y., Wu, Y., Dong, X., et al. (2021). Thrombospondin 4/integrin α2/HSF1 axis promotes proliferation and cancer stem-like traits of gallbladder cancer by enhancing reciprocal crosstalk between cancer-associated fibroblasts and tumor cells. J. Exp. Clin. Cancer Res. 40, 14. doi:10.1186/s13046-020-01812-7
Shintani, Y., Fujiwara, A., Kimura, T., Kawamura, T., Funaki, S., Minami, M., et al. (2016). IL-6 secreted from cancer-associated fibroblasts mediates chemoresistance in NSCLC by increasing epithelial-mesenchymal transition signaling. J. Thorac. Oncol. 11, 1482–1492. doi:10.1016/j.jtho.2016.05.025
Sobierajska, K., Ciszewski, W. M., Sacewicz-Hofman, I., and Niewiarowska, J. (2020). Endothelial cells in the tumor microenvironment. Adv. Exp. Med. Biol. 1234, 71–86. doi:10.1007/978-3-030-37184-5_6
Song, M., Wang, L., Jiang, S., Liang, J., Li, W., Rao, W., et al. (2024). Pathogenic Th17 cell-mediated liver fibrosis contributes to resistance to PD-L1 antibody immunotherapy in hepatocellular carcinoma. Int. Immunopharmacol. 129, 111601. doi:10.1016/j.intimp.2024.111601
Sr, M., S, V., W, Z., A, H., Db, A., and A, J. (2010). Cancer-associated fibroblasts derived from EGFR-TKI-resistant tumors reverse EGFR pathway inhibition by EGFR-TKIs. Mol. cancer Res. MCR 8, 809–820. doi:10.1158/1541-7786.MCR-09-0460
Su, J., Morgani, S. M., David, C. J., Wang, Q., Er, E. E., Huang, Y.-H., et al. (2020). TGF-β orchestrates fibrogenic and developmental EMTs via the RAS effector RREB1. Nature 577, 566–571. doi:10.1038/s41586-019-1897-5
Subramaniam, V., Chakrabarti, R., Prud’homme, G. J., and Jothy, S. (2010). Tranilast inhibits cell proliferation and migration and promotes apoptosis in murine breast cancer. Anticancer Drugs 21, 351–361. doi:10.1097/CAD.0b013e328334992c
Sun, B.-Y., Zhou, C., Guan, R.-Y., Liu, G., Yang, Z.-F., Wang, Z.-T., et al. (2022). Dissecting intra-tumoral changes following immune checkpoint blockades in intrahepatic cholangiocarcinoma via single-cell analysis. Front. Immunol. 13, 871769. doi:10.3389/fimmu.2022.871769
Sun, R., Zhao, H., Gao, D. S., Ni, A., Li, H., Chen, L., et al. (2023). Amphiregulin couples IL1RL1+ regulatory T cells and cancer-associated fibroblasts to impede antitumor immunity. Sci. Adv. 9, eadd7399. doi:10.1126/sciadv.add7399
Tada, A., Minami, T., Kitai, H., Higashiguchi, Y., Tokuda, M., Higashiyama, T., et al. (2023). Combination therapy with anti-programmed cell death 1 antibody plus angiokinase inhibitor exerts synergistic antitumor effect against malignant mesothelioma via tumor microenvironment modulation. Lung Cancer 180, 107219. doi:10.1016/j.lungcan.2023.107219
Takahashi, K., Menju, T., Nishikawa, S., Miyata, R., Tanaka, S., Yutaka, Y., et al. (2020). Tranilast inhibits TGF-β1-induced epithelial-mesenchymal transition and invasion/metastasis via the suppression of Smad4 in human lung cancer cell lines. Anticancer Res. 40, 3287–3296. doi:10.21873/anticanres.14311
Tao, Z.-Y., Wang, L., Zhu, W.-Y., Zhang, G., and Su, Y.-X. (2024). Lingual denervation improves the efficacy of anti-PD-1 immunotherapy in oral squamous cell carcinomas by downregulating TGFβ signaling. Cancer Res. Commun. 4, 418–430. doi:10.1158/2767-9764.CRC-23-0192
Tharp, K. M., Kersten, K., Maller, O., Timblin, G. A., Stashko, C., Canale, F. P., et al. (2024). Tumor-associated macrophages restrict CD8+ T cell function through collagen deposition and metabolic reprogramming of the breast cancer microenvironment. Nat. Cancer 5, 1045–1062. doi:10.1038/s43018-024-00775-4
Thomas, D., and Radhakrishnan, P. (2019). Tumor-stromal crosstalk in pancreatic cancer and tissue fibrosis. Mol. Cancer 18, 14. doi:10.1186/s12943-018-0927-5
Tu, J., Xu, H., Ma, L., Li, C., Qin, W., Chen, X., et al. (2022). Nintedanib enhances the efficacy of PD-L1 blockade by upregulating MHC-I and PD-L1 expression in tumor cells. Theranostics 12, 747–766. doi:10.7150/thno.65828
Wang, G., Zhou, X., Guo, Z., Huang, N., Li, J., Lv, Y., et al. (2022). The Anti-fibrosis drug Pirfenidone modifies the immunosuppressive tumor microenvironment and prevents the progression of renal cell carcinoma by inhibiting tumor autocrine TGF-β. Cancer Biol. Ther. 23, 150–162. doi:10.1080/15384047.2022.2035629
Wang, X., Chen, B., Zhang, H., Peng, L., Liu, X., Zhang, Q., et al. (2024). Integrative analysis identifies molecular features of fibroblast and the significance of fibrosis on neoadjuvant chemotherapy response in breast cancer. Int. J. Surg. 110, 4083–4095. doi:10.1097/JS9.0000000000001360
Wang, X., Wen, S., Du, X., Zhang, Y., Yang, X., Zou, R., et al. (2023). SAA suppresses α-PD-1 induced anti-tumor immunity by driving TH2 polarization in lung adenocarcinoma. Cell Death Dis. 14, 718. doi:10.1038/s41419-023-06198-w
Wang, Y., Ding, W., Hao, W., Gong, L., Peng, Y., Zhang, J., et al. (2024). CXCL3/TGF-β-mediated crosstalk between CAFs and tumor cells augments RCC progression and sunitinib resistance. iScience 27, 110224. doi:10.1016/j.isci.2024.110224
Wu, Y., Min, J., Ge, C., Shu, J., Tian, D., Yuan, Y., et al. (2020). Interleukin 22 in liver injury, inflammation and cancer. Int. J. Biol. Sci. 16, 2405–2413. doi:10.7150/ijbs.38925
Xu, S., Zheng, S., Ma, N., Zhang, H., Shi, J., Huang, J., et al. (2024). Rhein potentiates doxorubicin in treating triple negative breast cancer by inhibiting cancer-associated fibroblasts. Biochem. Pharmacol. 223, 116139. doi:10.1016/j.bcp.2024.116139
Yamazaki, T., Gunderson, A. J., Gilchrist, M., Whiteford, M., Kiely, M. X., Hayman, A., et al. (2022). Galunisertib plus neoadjuvant chemoradiotherapy in patients with locally advanced rectal cancer: a single-arm, phase 2 trial. Lancet Oncol. 23, 1189–1200. doi:10.1016/S1470-2045(22)00446-6
Yegodayev, K. M., Novoplansky, O., Golden, A., Prasad, M., Levin, L., Jagadeeshan, S., et al. (2020). TGF-Beta-Activated cancer-associated fibroblasts limit cetuximab efficacy in preclinical models of head and neck cancer. Cancers (Basel) 12, 339. doi:10.3390/cancers12020339
Yehia, L., Boulos, F., Jabbour, M., Mahfoud, Z., Fakhruddin, N., and El-Sabban, M. (2015). Expression of HIF-1α and markers of angiogenesis are not significantly different in triple negative breast cancer compared to other breast cancer molecular subtypes: implications for future therapy. PLoS One 10, e0129356. doi:10.1371/journal.pone.0129356
Yi, Y., Zeng, S., Wang, Z., Wu, M., Ma, Y., Ye, X., et al. (2018). Cancer-associated fibroblasts promote epithelial-mesenchymal transition and EGFR-TKI resistance of non-small cell lung cancers via HGF/IGF-1/ANXA2 signaling. Biochim. Biophys. Acta Mol. Basis Dis. 1864, 793–803. doi:10.1016/j.bbadis.2017.12.021
Yuan, H., Jin, L., Xiang, H., Bhattacharya, A., Brandish, P. E., Baltus, G., et al. (2022). Resistance of MMTV-NeuT/ATTAC mice to anti-PD-1 immune checkpoint therapy is associated with macrophage infiltration and Wnt pathway expression. Oncotarget 13, 1350–1358. doi:10.18632/oncotarget.28330
Zhang, D., Li, L., Jiang, H., Li, Q., Wang-Gillam, A., Yu, J., et al. (2018). Tumor-stroma IL1β-IRAK4 feedforward circuitry drives tumor fibrosis, chemoresistance, and poor prognosis in pancreatic cancer. Cancer Res. 78, 1700–1712. doi:10.1158/0008-5472.CAN-17-1366
Zhang, F., Cui, J.-Y., Gao, H.-F., Yu, H., Gao, F.-F., Chen, J.-L., et al. (2020). Cancer-associated fibroblasts induce epithelial-mesenchymal transition and cisplatin resistance in ovarian cancer via CXCL12/CXCR4 axis. Future Oncol. 16, 2619–2633. doi:10.2217/fon-2020-0095
Zhang, J., Li, S., Zhao, Y., Ma, P., Cao, Y., Liu, C., et al. (2020). Cancer-associated fibroblasts promote the migration and invasion of gastric cancer cells via activating IL-17a/JAK2/STAT3 signaling. Ann. Transl. Med. 8, 877. doi:10.21037/atm-20-4843
Zhang, J., Song, J., Tang, S., Zhao, Y., Wang, L., Luo, Y., et al. (2023). Multi-omics analysis reveals the chemoresistance mechanism of proliferating tissue-resident macrophages in PDAC via metabolic adaptation. Cell Rep. 42, 112620. doi:10.1016/j.celrep.2023.112620
Zhang, M., Bi, B., Liu, G., and Fan, X. (2024). PYCR1 expresses in cancer-associated fibroblasts and accelerates the progression of C6 glioblastoma. Histol. Histopathol. 18762. doi:10.14670/HH-18-762
Zhang, S., Yuan, L., Danilova, L., Mo, G., Zhu, Q., Deshpande, A., et al. (2023). Spatial transcriptomics analysis of neoadjuvant cabozantinib and nivolumab in advanced hepatocellular carcinoma identifies independent mechanisms of resistance and recurrence. Genome Med. 15, 72. doi:10.1186/s13073-023-01218-y
Zhang, Y., Gu, Z., Wan, J., Lou, X., Liu, S., Wang, Y., et al. (2022). Stearoyl-CoA Desaturase-1 dependent lipid droplets accumulation in cancer-associated fibroblasts facilitates the progression of lung cancer. Int. J. Biol. Sci. 18, 6114–6128. doi:10.7150/ijbs.74924
Zhang J., J., Fu, L., Wang, H., Yonemura, A., Semba, T., Yasuda-Yoshihara, N., et al. (2024). RAC1-mediated integrin alpha-6 expression in E-cadherin-deficient gastric cancer cells promotes interactions with the stroma and peritoneal dissemination. Cancer Lett. 591, 216901. doi:10.1016/j.canlet.2024.216901
Zhang S., S., Wang, Y., Luo, D., Cheng, Z., Zeng, Q., Wang, G., et al. (2024). Pirfenidone inhibits TGF-β1-induced metabolic reprogramming during epithelial-mesenchymal transition in non-small cell lung cancer. J. Cell Mol. Med. 28, e18059. doi:10.1111/jcmm.18059
Zheng, K., Yu, J., Chen, Z., Zhou, R., Lin, C., Zhang, Y., et al. (2019). Ethanol promotes alcohol-related colorectal cancer metastasis via the TGF-β/RUNX3/Snail axis by inducing TGF-β1 upregulation and RUNX3 cytoplasmic mislocalization. EBioMedicine 50, 224–237. doi:10.1016/j.ebiom.2019.11.011
Zheng, S., Tian, Q., Yuan, Y., Sun, S., Li, T., Xia, R., et al. (2023). Extracellular vesicle-packaged circBIRC6 from cancer-associated fibroblasts induce platinum resistance via SUMOylation modulation in pancreatic cancer. J. Exp. Clin. Cancer Res. 42, 324. doi:10.1186/s13046-023-02854-3
Zhou, J., Schwenk-Zieger, S., Kranz, G., Walz, C., Klauschen, F., Dhawan, S., et al. (2022). Isolation and characterization of head and neck cancer-derived peritumoral and cancer-associated fibroblasts. Front. Oncol. 12, 984138. doi:10.3389/fonc.2022.984138
Zhou, P., Du, X., Jia, W., Feng, K., and Zhang, Y. (2024). Engineered extracellular vesicles for targeted reprogramming of cancer-associated fibroblasts to potentiate therapy of pancreatic cancer. Signal Transduct. Target Ther. 9, 151. doi:10.1038/s41392-024-01872-7
Zhou, Y., Ren, H., Dai, B., Li, J., Shang, L., Huang, J., et al. (2018). Hepatocellular carcinoma-derived exosomal miRNA-21 contributes to tumor progression by converting hepatocyte stellate cells to cancer-associated fibroblasts. J. Exp. Clin. Cancer Res. 37, 324. doi:10.1186/s13046-018-0965-2
Keywords: fibrosis, tumor, CAFs, TGF-β, EMT
Citation: Chen H, Xu X, Li J, Xue Y, Li X, Zhang K, Jiang H, Liu X and Li M (2024) Decoding tumor-fibrosis interplay: mechanisms, impact on progression, and innovative therapeutic strategies. Front. Pharmacol. 15:1491400. doi: 10.3389/fphar.2024.1491400
Received: 04 September 2024; Accepted: 09 October 2024;
Published: 23 October 2024.
Edited by:
Yaying Sun, Fudan University, ChinaCopyright © 2024 Chen, Xu, Li, Xue, Li, Zhang, Jiang, Liu and Li. This is an open-access article distributed under the terms of the Creative Commons Attribution License (CC BY). The use, distribution or reproduction in other forums is permitted, provided the original author(s) and the copyright owner(s) are credited and that the original publication in this journal is cited, in accordance with accepted academic practice. No use, distribution or reproduction is permitted which does not comply with these terms.
*Correspondence: Xiaoliu Liu, NzM0Mjg2NTg4QHFxLmNvbQ==; Mingzhe Li, NzI3NDE3NjI5QHFxLmNvbQ==
†These authors have contributed equally to this work