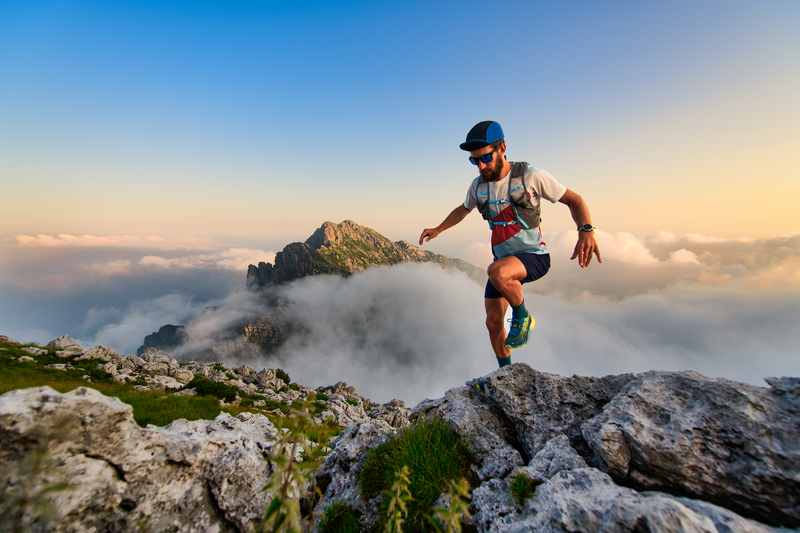
95% of researchers rate our articles as excellent or good
Learn more about the work of our research integrity team to safeguard the quality of each article we publish.
Find out more
REVIEW article
Front. Pharmacol. , 27 May 2024
Sec. Ethnopharmacology
Volume 15 - 2024 | https://doi.org/10.3389/fphar.2024.1398294
Ulcerative colitis (UC) is a chronic inflammatory disease of the intestines that can significantly impact quality of life and lead to various complications. Currently, 5-aminosalicylic acid derivatives, corticosteroids, immunosuppressants, and biologics are the major treatment strategies for UC, but their limitations have raised concerns. Atractylenolides (ATs), sesquiterpene metabolites found in Atractylodes macrocephala Koidz., have shown promising effects in treating UC by exerting immune barrier modulation, alleviating oxidative stress, gut microbiota regulation, improving mitochondrial dysfunction and repairing the intestinal barrier. Furthermore, ATs have been shown to possess remarkable anti-fibrosis, anti-thrombus, anti-angiogenesis and anti-cancer. These findings suggest that ATs hold important potential in treating UC and its complications. Therefore, this review systematically summarizes the efficacy and potential mechanisms of ATs in treating UC and its complications, providing the latest insights for further research and clinical applications.
Ulcerative colitis (UC) is a chronic inflammatory bowel disease characterized by continuous and diffuse inflammation in the colonic and rectal mucosa. As its long course, high recurrence, and high risk of malignant transformation, UC has been classified by the WHO as one of the clinically intractable diseases (Li et al., 2022). In Europe and North America, the highest incidence is 24.3/100,000 and 19.2/100,000, respectively. With changing in lifestyle and dietary habits in Asian countries, the incidence has been increasing annually to 7.6/100,000 to 14.3/1000,000, and the morbidity is 2.3/1,000 to 63.6/1,000. The direct and indirect costs associated with UC reach to €1.25 to €2.91 billion annually in Europe and $0.81 to $1.49 billion in the United States. The expensive treatment costs and the gradually increasing incidence have brought about dual challenges of economic burden and public health. Moreover, UC is caused by environmental factors acting on genetically susceptible populations that lead to gut microbiota imbalance or intestinal barrier damage resulting from mucosal immune dysfunction. Symptoms include persistent or recurrent diarrhea, accompanied by abdominal pain urgency, weight loss, mucus bloody stools and other varying degrees of systemic symptoms, lasting more than 4–6 weeks, leading to repeated intermittent acute episodes that may last for years, causing discomfort to patients affected by this disease (Xue et al., 2023). In response to the clinical symptoms and pathological characteristics of UC, clinical treatment is constantly developing and updating, aiming for better treatment of UC and its complications.
Recently, the first-line drugs for the treatment of UC include 5-aminosalicylic acid, corticosteroids, and immunosuppressants (Feuerstein and Cheifetz, 2017). Various formulas and combination therapies of traditional drugs play an important role in treating mild to moderate UC(Le Berre et al., 2023). In recent years, with in-depth research on the pathogenesis of UC, the development of molecularly targeted biologics has become a revolutionary breakthrough in the treatment of UC. The emergence of biologics has greatly alleviated refractory UC that cannot be relieved by traditional drugs and improved the therapeutic effect of UC(Nakase et al., 2022). With the widespread use of these medications, several adverse reactions have gradually been found. Surveys have shown that 30%–55% of patients have no response to molecularly targeted drugs during induction or develop drug resistance in the later stages of treatment. A study has reported that more than 10% of patients have ineffective drug therapy and require surgical treatment (Xu et al., 2023). In addition, due to the recurrent episodes and numerous complications of UC, patients often suffer from great physical, psychological and economic pressure (Burisch et al., 2023). Therefore, the development of effective treatment strategies is essential for the prevention and treatment of UC and its complications.
Natural products are potential therapeutic strategies for UC in clinical practice (Lu et al., 2023). Atractylodes macrocephala Koidz., one of the commonly used traditional Chinese medicines in clinic (Yang et al., 2021), is widely applied to treat digestive system diseases due to its effects of invigorating the spleen and replenishing qi, drying dampness and promoting diuresis, stopping sweating and calming the fetus (Zhou et al., 2021). Studies have shown that A. macrocephala Koidz. plays an important role in the treatment of UC(Feng et al., 2018; Deng et al., 2019a; Deng et al., 2019b; Chen W. et al., 2021; Yu et al., 2022; Zou and Zhu, 2022). Atractylenolides (ATs) are sesquiterpene compounds extracted from the Atractylodes genus of the Asteraceae family, which is one of the main active ingredients in A. macrocephala Koidz. (Wang Y. M. et al., 2021).
ATs have a variety of pharmacological effects and significant therapeutic effects on inflammatory diseases. In addition, ATs can be rapidly absorbed and metabolized slowly, making them valuable for drug development (Deng et al., 2021). ATs have various configurations, among which AT-I, AT-II and AT-III are extensively studied configurations with strong pharmacological effects. In recent years, many scholars have paid high attention to the pharmacological activities of these three types of ATs (Yang et al., 2021).
Studies have shown that ATs can effectively treat UC through their immune barrier modulation and alleviating oxidative stress, as well as intestinal barrier repair, improvement of mitochondrial dysfunction, and regulation of the intestinal microbiota (Han et al., 2022; Qu et al., 2022). Additionally, it also has the effects of anti-thrombus, reducing multiorgan fibrosis, and decreasing pathological angiogenesis (Yang et al., 2021). These reports suggest that ATs have great advantages in the treatment of UC and its complications. However, the targets of ATs for the treatment of UC and their underlying biological processes remain to be comprehensively reviewed. The aim of this paper is to comprehensively summarize the efficacy and potential mechanisms of ATs for the treatment of UC and its complications and to provide a scientific basis for the in-depth study and clinical application of ATs. Tables 1, 2 summarize the applications of ATs in cellular and animal experiments, respectively.
ATs have a wide range of sources. The dried rhizome of A. macrocephala Koidz., a plant belonging to the Asteraceae family (Wang Y. M. et al., 2021), is the major source of ATs. Plants of the Atractylodes genus are mainly distributed in eastern Asia with seven subspecies, namely, A. macrocephala Koidz., Atractylodes japonica Koidz. ex Kitam., Atractylodes lancea (Thunb.) DC., Atractylodes chinensis (DC.) Koidz., Atractylodes carlinoides (Hand. Mazz.) Kitam., Atractylodes coreana (Nakai) Kitam. and A. lancea (Thunb.) DC. Subsp. Luotianensis (Liu et al., 2016).
The commonly used clinical Atractylodes are the rhizomes of the A. lancea (Thunb.) DC. and A. chinensis (DC.) Koidz. (Wang Y. M. et al., 2021), which are also important sources of ATs. Furthermore, Codonopsis pilosula from the Campanulaceae family is also an important source of ATs. The existence of ATs can be used as an important identification and quality evaluation index of C. pilosula (Wang et al., 2023). Various plants in the Orchidaceae family, such as Cremastra appendiculata also contains ATs (Deng et al., 2021) (Table 3).
ATs belong to the sesquiterpene class of metabolites, which are considered one of the major active metabolites in Atractylodes genus plants. The common sesquiterpenes found in A. macrocephala Koidz. include atractylenolide, atractylon, AT-I, AT-II, AT-III, AT-IV, diacetyl-atractylodinolide, AT-V, AT-VI, AT-VII, 3β-acetoxyatractylenolide, dehydroatractylonolide, isoatractyloside A, atractylodinamide and 8β-methoxy-atractylenolide II (Yao et al., 2019). Furthermore, in recent years, a number of new sesquiterpenoids have been isolated from the rhizomes of A. macrocephala Koidz., such as atractylenolactam A, atractylenolactam B, 8-methoxy-atractylenolide V and 15-acetoxyl atractylenolide III (Hoang et al., 2016; Wang et al., 2022; Hai et al., 2023). Common sesquiterpenoids in A. lancea (Thunb.) DC. include β-eudesmol, acoriol, atractylon, AT-I, AT-II and AT-III (Cho et al., 2016; Zhao et al., 2022). AT-I, AT-II and AT-III are important active metabolites shared by A. macrocephala Koidz., A. lancea (Thunb.) DC., A. chinensis (DC.) Koidz. and A. japonica Koidz. ex Kitam. ATs are being extensively studied due to their wide range of pharmacological activities. Therefore, this paper primarily summarizes the therapeutic effects and potential mechanisms of AT-I, AT-II and AT-III in UC.
As a shared precursor of sesquiterpenoid metabolites, cis-farnesol undergoes an acetyl-CoA reaction to yield farnesyl pyrophosphate, which is further converted into farnesyl caryophyllene. Under the catalytic influence of sesquiterpene cyclase, farnesyl caryophyllene is transformed into sesquiterpenes. These sesquiterpenes experience double bond cleavage and condensation to form intermediates, which subsequently undergo oxidation and dehydration to generate costunolide. Costunolide, being inherently unstable, self-oxidizes to form a diene diol structure. This structure, following varying degrees of oxidation and double bond isomerization, cyclizes to form AT-I, AT-III or couples to create bisabolene lactone. Furthermore, AT-III can be dehydrated to produce AT-II (Yao et al., 2019). The study on cytochrome P450 (CYP450) simulated the oxidation model of mutual transformation for three types of ATs. AT-II can be oxidized to generate AT-III, and it can also be converted to AT-I by dehydration (Kim et al., 2018) (Figure 1).
Studies on the absorption of AT-I in the intestines of rats revealed that it is a highly permeable metabolites that can be rapidly absorbed throughout the intestine. The absorption characteristics of AT-I in different intestinal segments of rats were analyzed by an in vivo intestinal circulation model and high-performance liquid chromatography. There was no significant difference in the absorption rate constant of AT-I in duodenum, jejunum, ileum and colon. The passive diffusion mediated by the P-glycoprotein (Pgp) efflux transport system facilitated its absorption and distribution (Wang et al., 2009a). After oral administration of AT-I in rats at a dose of 50 mg/kg, the peak time (Tmax), maximum concentration (Cmax), area under the curve (AUC), and absorption rate constant (Ka) were 0.37 ± 0.19 h, 0.26 ± 0.05 μg/mL, 1.95 ± 0.30 μg h/mL and 10.08 ± 5.60 h-1, respectively (Wang et al., 2009a; Li et al., 2012).
In addition, rats liver mitochondria were co-cultured with AT-I, and the bio-transformation products were characterized after pretreatment with phenobarbital sodium. The results show that the main metabolites of AT-I were hydroxylated and methylated products, and the C-1, C-2, and C-3 positions of ring A could be specifically oxidized. Therefore, the reaction between AT-I and reactive oxygen species (ROS) may be one of the main mechanisms by which AT-I exerts its anti-inflammatory and anti-cancer effects to prevent or alleviate oxidative damage caused by ROS to bio-molecules (Li and Yang, 2013).
The pharmacokinetic study conducted on rats after intragastric administration of AT-II showed that the elimination half-life (T1/2) and Tmax of the drug were 0.14 h and 0.41 h, respectively, indicating that AT-II was rapidly absorbed in rats. The study also showed that after oral administration of A. macrocephala Koidz. extract equivalent to a dose of 82.82 μg/kg of AT-II in rats, the Cmax, AUC0→12, clearance rate (CLz/F) and T1/2 values of AT-II were 7.99 ± 0.90 ng/mL, 28.46 ± 7.71 ng h/mL, 0.043 ± 0.015 L/(kg•h) and 2.63 ± 1.08 h, respectively (Shi et al., 2012). When co-incubated with rat liver microsomes, AT-II mainly underwent hydroxylation and epoxidation reactions at the double bonds between C-4 and C-15, as well as at positions C-1, C-3, C-8, and C-13, which are easily hydroxylated (Li et al., 2013).
AT-Ⅲ has been reported as a compound with rapid absorption characteristics. After oral administration of AT-III to SD rats at a dose of 100 mg/kg, the results showed that AT-III was rapidly absorbed and distributed evenly in the body (Li et al., 2006). After administering an extract of A. macrocephala Koidz. equivalent to a dose of 185.16 μg/kg of AT-III by gavage in rats, the Cmax, AUC 0→12, CLz/F, and T1/2 values of AT-III were 9.79 ± 1.79 ng/mL, 37.43 ± 7.86 ng h/mL, 0.029 ± 0.0068 L/(kg•h) and 4 ± 1.94 h, respectively (Shi et al., 2012). High-performance liquid chromatography showed that it was mainly excreted through the spleen, followed by liver and kidney. The metabolism pathways of AT-III encompass various processes such as methylation, oxidation, hydroxylation, dihydroxylation, reduction, glycosylation, sulfation, glucuronic acid conjugation, cysteine and N-acetylcysteine binding, among others. After oral administration of AT-III, more than 50 metabolites were identified in rat feces urine and plasma (Jiang et al., 2019).
Current studies have shown that AT-I, AT-II, and AT-III can be quickly absorbed into the blood and detected within 0.0833 h, and the retention time in the plasma is about 12 h. The inverted intestinal sac model indicated that ATs had similar pharmacokinetic characteristics and could be passively transported by all intestinal segments. However, AT-III had the highest absorption rate in all intestinal segments, and the duodenum was the main site for the absorption of AT-II, while AT-I was absorbed rapidly within entire enteric. ATs are considered to be rapidly absorbed without obvious interaction with each other, suggesting good bioavailability based on their pharmacokinetic data (Gao et al., 2018).
Although changes in experimental factors can alter the pharmacokinetic parameters of ATs, they are rapidly absorbed into the blood and slowly eliminated in rats. Furthermore, there have been no reports of toxic side effects from ATs in the clinic. Therefore, ATs show potential for further drug development. Meanwhile, as key metabolites of traditional Chinese medicines A. macrocephala Koidz. and A. lancea (Thunb.) DC., the new drug development of ATs holds significant social value in promoting the utilization and development of these botanical drugs.
Although the etiology of UC remains incompletely elucidated, recent advancements underscore the pivotal role of cytokines and immune cells in the pathogenesis of UC (Neurath, 2014). Within adaptive immunity, the dysregulation of Th1/Th2 and Th17/Treg cells is considered an important reason for the immunological imbalance observed in UC (Geremia et al., 2014; Iboshi et al., 2017). Cohort studies have also highlighted substantial dysregulation in B cell responses in UC, underscoring the potential involvement of humoral immunity in the pathogenesis of this condition (Uzzan et al., 2022). Furthermore, the innate immune system of the mucosa has been extensively investigated. Neutrophils, monocytes, and macrophages collectively form the innate barrier of phagocytic cells, playing a critical role in the pathogenesis of UC (Zhou and Liu, 2017; Uhlig and Powrie, 2018). Consequently, the modulation of the balance of immune cells and cytokines to restore intestinal mucosal homeostasis represents a primary therapeutic target in the clinical management of UC (Tong et al., 2021).
AT-I and AT-III exhibited pronounced immune barrier modulation effects in vivo and vitro. In a murine model of DSS (Dextran Sulfate Sodium Salt)-induced UC, AT-I and AT-III markedly suppressed the production of pro-inflammatory cytokines such as TNF-α, IL-6, cyclooxygenase-2 (COX-2) and iNOS, ameliorating colonic inflammation response (Han et al., 2022; Qu et al., 2022). In a study involving LPS (Lipopolysaccharide)-induced murine macrophages RAW264.7, AT-I, and AT-III shown no inhibitory effect on cell proliferation within the concentration range of 1 μM–100 μM. They effectively inhibited the production of NO, PGE2, TNF-α and IL-6. Their immune barrier modulation effects may be associated with the suppression of the expression of NF-kB, ERK1/2, and p38 (Ji et al., 2014; Ji et al., 2016). Furthermore, research indicates that AT-I exhibits a more pronounced inhibitory effect on the activation of macrophages, leading to the production of TNF-α and NO in response to LPS, compared to AT-III (Li et al., 2007b). Toll-like receptor 4 (TLR4) binding to its ligand, LPS, triggers MyD88 protein adapter-mediated inflammatory responses, playing a pivotal role in the development of UC. Activation of signaling molecules downstream of TLR4, including NF-kB and those mediated by MAPK kinases such as JNK, ERK and p38, results in the transcription of numerous pro-inflammatory genes, including TNF-α, IL-6, IL-1β, and COX-2, subsequently eliciting a UC inflammatory response (Wang G. et al., 2019). Therefore, AT-I and AT-III act as antagonists of the TLR4 receptor and effectively suppress the release of pro-inflammatory cytokines by inhibiting the TLR4/NF-kB/MAPK signaling pathway, leading to a significant improvement in UC.
The systematic pharmacological investigation of the ginsenoside capsule also yielded consistent results. One of the ginsenoside capsule’s bioactive ingredients, AT-I, has been shown to dramatically decrease tissue levels of pro-inflammatory cytokines such IL-6, TNF-α, and INF-γ. Its mechanism is associated with the inhibition of NF-kB activation mediated by the MyD88 protein adapter triggered by the TLR4 receptor, resulting in the transcriptional downregulation of numerous pro-inflammatory genes, including TNF-α, IL-6, IL-1β, and COX-2, thereby mitigating the inflammatory response (Feng et al., 2018). Additionally, a study revealed that AT-I effectively inhibits increased vascular permeability in acetic acid-induced mice and opposes granulation tissue proliferation, indicating therapeutic effects of AT-I on both acute and chronic inflammation (Li et al., 2007a).
Mast cells originate from precursor cells in the bone marrow, serving as the first line of defense in the immune system against various challenges. In a murine model of UC, histamine derived from mast cells mediates neutrophil infiltration into the colonic mucosa through H4R, participating in the inflammatory response. This suggests that mast cells serve as alternative therapeutic targets beyond adaptive immunity (Wechsler et al., 2018). Additionally, compared to non-inflammatory UC regions, research on human colonic tissue show a considerable upregulation of certain mast cell mediators in inflammatory UC regions. Variants that reduce mast cell activity effectively prevent the development of UC (Chen E. et al., 2021). AT-III possesses the capability to reduce mast cell proliferation induced by thymic stromal lymphopoietin (TSLP) and the production of pro-inflammatory cytokines, including IL-6, IL-1β, TNF-α and IL-8 (Yoou et al., 2017). Additionally, AT-III may suppress immune responses by inhibiting the secretion of IL-6 within mast cells (Kang et al., 2011). Therefore, serving as a multifunctional immunoregulator, AT-III plays a crucial role in regulating both innate and adaptive immunity.
In the pathological processes of UC, inflammation and oxidative stress are believed to play pivotal roles, exacerbating the immune response and intestinal damage of UC (Maloy and Powrie, 2011; Zhu and Li, 2012). Chronic inflammation serves as a significant stimulant for the overproduction of ROS (Santhanam et al., 2012), and high concentrations of ROS can damage cellular structures, leading to secondary mucosal injury (Ho et al., 2018). This may contribute to the perpetuation and consolidation of intestinal inflammation in UC. Furthermore, such damage increases the risk of pathogen invasion, which may trigger new immune responses, thereby exacerbating the progression and chronic damage associated with UC. Therefore, anti-inflammatory and anti-oxidant approaches are vital in the clinical treatment of UC.
Treatment with AT-III for UC mice by TNBS-induced for 14 days yielded promising results. AT-III administered at high and medium doses resulted in a considerable improvement in both histopathological damage and symptoms. Also, pro-inflammatory cytokines like TNF-α and IL-1β were downregulated. Additionally, the activity levels of myeloperoxidase were attenuated. AT-III also reduced the expression of pro-oxidative markers, ROS, and malondialdehyde (MDA) in UC mice while enhancing the expression levels of endogenous anti-oxidants, including catalase (CAT), superoxide dismutase (SOD), and glutathione peroxidase (GPx), among others. Further investigations suggested that AT-III may exert its anti-oxidative effects through the modulation of formy1 peptide receptor 1 (FPR1) and nuclear factor erythroid 2-related factor 2 (Nrf2) pathways (Ren et al., 2021a).
Nrf2 is a pivotal transcription factor associated with cellular anti-oxidant responses and serves as the central regulator for maintaining cellular redox homeostasis (Wang R. et al., 2019). Under physiological conditions, Nrf2 resides in the cytoplasm and forms a complex with Kelch-like ECH-associated protein 1 (Keap1), subject to proteasomal degradation, maintaining an inhibitory state. Upon exposure to oxidative stress, Nrf2 dissociates from the Keap1/Nrf2 complex, translocates into the nucleus, forms a heterodimer with small Maf proteins, and binds to anti-oxidant response elements (ARE), thereby activating downstream anti-oxidant proteins such as heme oxygenase-1 (HO-1) to exert anti-oxidative and anti-inflammatory effects (Lin et al., 2020). The extract of A. macrocephala Koidz. was regarded as ideal HO-1 expression promoter, which has better efficacy than sulfasalazine drugs for preventing UC recurrence (Han et al., 2017). There are reports that both AT-II and AT-III have similar anti-oxidant effects that activate the Nrf2 pathway as anti-oxidants (Yang et al., 2017).
While the pathogenesis of UC is still under investigation, the prevailing consensus among researchers suggests that environmental factors, in conjunction with the intestinal microbiota, act on genetically susceptible individuals, triggering immune-inflammatory responses that disrupt the intestinal barrier and lead to the development of UC (Mirkov et al., 2017). The human intestinal tract harbors a diverse microbiome comprising various bacteria, viruses, and phages. Dysbiosis in the intestinal microbiota can promote the development of UC by increasing the pathogenic bacterial load, reducing the levels of beneficial bacteria, and disrupting normal immune tolerance. It is currently established that UC patients exhibit abnormalities in the composition of their intestinal microbiota, potentially involving the reduction in overall diversity and the expansion of pathogenic strains (Ni et al., 2017). Therefore, the management of intestinal dysbiosis is of paramount importance in the comprehensive treatment of UC.
Results from 16S sequencing of fecal samples from UC experimental mice indicate that AT-Ⅰ increases the diversity and abundance of the intestinal microbiota in UC mice. Further investigations reveal that AT-Ⅰ suppresses inflammation through the SPHK1/PI3K/AKT axis and modulates fructose and lactose-related metabolism by targeting two genes (SPHK1 and B4GALT2). This regulation influences the composition of the intestinal microbiota and ameliorates colonic inflammation (Qu et al., 2022). Moreover, a study comparing changes in the gut microbiota of mice following AT-I treatment, utilizing a mouse model of intestinal dysbiosis, demonstrates that AT-I adjusts the gut microbiota by increasing the abundance of beneficial bacteria such as lactobacilli and bifidobacteria, while dose-dependently reducing the abundance of harmful bacteria like Escherichia coli and Candida (Liu et al., 2021). This work provides more evidence that the gut microbiota mediates the inhibitory effect of AT-Ⅰ on tumor growth, with possible mechanisms involving the downregulation of the TLR4/MyD88/NF-kB signaling pathway.
Researchers have also conducted fecal 16S DNA analysis in TNBS-induced mice following AT-III treatment. The data suggests that AT-III effectively ameliorates the reduction of beneficial microbial communities at the genus level in UC mice induced by TNBS, which alters the structure and composition of the gut microbiota. Among these changes, there is a significant increase in lactobacilli, which can modulate the expression level of FPR1 and subsequently regulate the oxidative stress levels in the colorectal tissue (Ren et al., 2021a). Thus, AT-III regulates oxidative stress via the FPR1 and Nrf2 pathways, impacting the gut microbiota’s growth and reducing the TNBS-induced colonic inflammatory response. (Table 4).
The histopathological features of UC encompass epithelial ulceration, infiltration of lamina propria immune cells, crypt abscesses, splenomegaly and hepatomegaly, and compromised intestinal barrier function (Xu et al., 2023). The integrity of the intestinal epithelium is a critical determinant affecting the function of the gut barrier. The migration and proliferation of intestinal epithelial cells (IEC) represent fundamental mechanisms for mucosal ulcer healing and wound repair. AT-I promotes the migration and proliferation of IEC-6 cells, which is possibly mediated through the augmentation of cytoplasmic calcium levels, driven by polyamines (Song et al., 2017). Previous research has also shown that both AT-I and AT-III can reverse the reduced expression levels of mucin MUC2 and tight junction proteins (zo-1, occludin) in the colonic tissues of UC mice (Han et al., 2022; Qu et al., 2022). Therefore, AT-I and AT-III can enhance the expression of tight junction proteins, effectively promoting the repair of intestinal epithelium and ameliorating the mucosal barrier function in UC.
The research on the role of A. macrocephala Koidz. extract in intestinal epithelial repair indicates that AT-II and AT-III exert varying degrees of promotion on IEC-6 cell proliferation and migration. AT-III stands out as the primary active ingredient in A. macrocephala Koidz. Extract, showing its ability to stimulate cell proliferation. The combination of ATs exhibits superior effectiveness in promoting intestinal epithelial repair (Ren et al., 2021b). In vivo studies showed that giving Wistar rats 10 mg/kg of AT-III dramatically reduced the amount of ethanol-induced stomach ulcers. AT-III achieves this by upregulating tissue metalloproteinase inhibitors, consequently inhibiting the expression of matrix metalloproteinases, specifically MMP-2 and MMP-9, within the ulcerated gastric tissue (Wang et al., 2010). Hence, a combined utilization of components such as AT-I, AT-II, and AT-III can be considered when promoting intestinal epithelial repair.
Research indicates that patients with UC may possess an inherent susceptibility to mitochondrial dysfunction, which can be masked by the intestinal environment (Ho and Theiss, 2022). Mitochondrial dysfunction leads to the production of high levels of superoxide and hydrogen peroxide within cells. These substances can combine with iron to generate free radicals, causing oxidative damage to macromolecules within the mitochondria, with mitochondrial DNA being particularly vulnerable (Shimada et al., 2012). Mitochondrial damage results in energy deficits that increase the susceptibility to cell death while simultaneously exacerbating the inflammatory response within the UC.
Receptor-γ coactivator 1-α (PGC-1α) is prominently expressed in the uppermost intestinal epithelial cells, furthest from the crypt base. It drives mitochondrial biogenesis and the metabolic shift towards mitochondrial respiration (D'Errico et al., 2011). Research suggests that reduced PGC-1α in intestinal epithelium is associated with mitochondrial dysfunction, epithelial barrier damage, and inflammatory responses in UC. Studies have indicated that enhanced PGC-1α deacetylation can repair damaged mitochondria and preserve intestinal barrier function (Cunningham et al., 2016). AT-III was reported to alleviate mitochondrial dysfunction in DSS-induced colitis mice, consistent with results from LPS-treated IEC-6 cells. Further mechanistic studies reveal that AT-III activates AMP-activated protein kinase (AMPK) and sirtuin 1 (SIRT1), subsequently increasing PGC-1α expression and promoting its deacetylation (Han et al., 2022). Therefore, AMPK/SIRT1/PGC-1α may represent a potential pathway through which AT-III ameliorates mitochondrial dysfunction in the UC intestinal epithelium. Studies suggest that mitochondria play a crucial role in various cellular functions, rapidly responding to extracellular stimuli and cellular demands while dynamically communicating with other organelles (Ni et al., 2015). Maintaining normal mitochondrial function may improve inflammatory responses, oxidative stress, and intestinal epithelial barrier damage in UC (Yeganeh et al., 2018; Wang S. Q. et al., 2019) (Figures 2, 3).
Intestinal fibrosis is considered a common pathological feature of UC and represents a long-term complication characterized by excessive proliferation of myofibroblasts and collagen deposition (Rieder et al., 2017; Laudadio et al., 2022). Under normal circumstances, damaged colonic tissue undergoes a healing process through the intestine. However, if the intestine continues to sustain damage, it can result in chronic intestinal inflammation, marked by persistent injury and repair, ultimately leading to intestinal fibrosis (Wang R. et al., 2021). Intestinal fibrosis can result in frequent luminal narrowing, severely compromising the physiological function of the intestine and significantly impacting the quality of life (Schwab et al., 2019). Current evidence suggests that persistent inflammation is an important factor leading to fibrosis, but anti-inflammatory treatment has not fully reduced the overall incidence of intestinal fibrosis (Abraham et al., 2017; Henderson et al., 2020). Therefore, anti-fibrosis drugs are necessary to be developed.
Research suggests that up-regulating the expression of Nrf2 to inhibit the TGF-β1/Smad pathway can ameliorate inflammation-related intestinal fibrosis, underscoring the potential therapeutic targeting of Nrf2 in alleviating intestinal fibrosis (Wang R. et al., 2021). Previous studies have indicated that AT-III serves as an effective activator of the Nrf2 transcription factor (Zhu and Li, 2012). Therefore, AT-III is anticipated to play a role in anti-intestinal fibrosis through the Nrf2/TGF-β1/Smad pathway. Another study also suggests that AT-III can inhibit epithelial mesenchymal transition (EMT) in IEC-6 cells by activating the AMPK signaling pathway, thereby suppressing cell migration induced by TGF-β. Furthermore, AT-III exerts no inhibitory effect on IEC-6 cell proliferation at dosages ranging from 1 to 20 μmol/L (Huang et al., 2022).
Moreover, several studies have indicated that ATs can exert effective therapeutic effects in various fibrotic diseases. Experiments conducted using an in vitro unilateral ureteral obstruction (UUO) murine model have shown that AT-Ⅰ inhibits myofibroblast phenotype and fibrotic development in the murine kidney by targeting fibroblast-to-myofibroblast differentiation (FMD) as well as EMT (Guo et al., 2021). Research on a bleomycin-induced pulmonary fibrosis model in Sprague-Dawley rats has demonstrated that AT-III mitigates oxidative stress and lung fibrotic damage in the rat pulmonary fibrosis model by activating the Nrf2/NQO1/HO-1 pathway (Huai and Ding, 2020). Current experiments suggest that the upregulation of the Nrf2/NQO1/HO-1 pathway can effectively ameliorate UC, rendering it a potential therapeutic strategy for UC treatment (Amirshahrokhi and Imani, 2023; Ekhtiar et al., 2023). Hence, pending additional validation through research, one mechanism by which AT-III exerts its anti-intestinal fibrosis properties may be the stimulation of the Nrf2/NQO1/HO-1 pathway. These findings collectively indicate that AT-I and AT-III hold promise as potential drugs for the future treatment of UC associated intestinal fibrosis.
In the colonic tissues of both UC patients and experimental UC animal models, platelet activation has been observed, leading to increased thrombus formation by binding to the vascular endothelium. Extraintestinal thrombosis is more common in the DSS-induced UC animal, which also exhibits an increase in serum coagulation markers. Thrombus formation results in ischemic inflammation in the intestinal microvascular system, further exacerbating tissue damage. Furthermore, research has indicated a local increase in procoagulant and prothrombotic events within the microvascular system of UC intestinal tissues, which is associated with subclinical systemic thrombosis in patients. Thrombus formation is a significant complication in inflammatory bowel disease (IBD) patients, accounting for an estimated 25% of IBD-related mortality causes. The risk of thrombotic events is particularly elevated during disease flares and periods of chronic inflammation. Therefore, thrombus formation plays a crucial role in the progression of UC and underscores the importance of early consideration of anti-thrombotic therapy for UC patients (Nelson et al., 2023).
Research has shown that both AT-II and AT-III exhibit significant anti-thrombotic effects. AT-II at a concentration of 10 μmol/L effectively inhibits in vitro aggregation of mouse and human platelets stimulated by collagen. This effect is possibly achieved by suppressing the PI3K-Akt pathway, thereby inhibiting platelet activation (Chen et al., 2016a). Furthermore, thromboxane analog (U46619)-induced human platelet aggregation in vitro as well as adenosine triphosphate (ATP) production from platelet-dense granules are markedly inhibited by AT-III. The inhibitory effect is concentration-dependent, with the most pronounced inhibition observed at an AT-III concentration of 5 μmol/L. Further investigations suggest that AT-III may inhibit platelet activation by influencing the MAPK and PI3K-Akt pathways, thus mitigating thrombus formation (Chen et al., 2016b).
AT-II and AT-III also significantly reduce the spreading of human platelets on immobilized fibrinogen, delay clot retraction in plasma, increase the time to initial occlusion in the FeCl3-induced carotid artery thrombosis model in mice, and prolong bleeding time. Research has additionally revealed that the inhibitory effects of AT-II and AT-III on platelet activation are akin to the aspirin (Chen et al., 2017). Consequently, AT-II and AT-III may be very promising as therapeutic agents for the management of UC that is complicated with thrombus formation, although more thorough investigation is needed to confirm this. Moreover, AT-II and AT-III’s anti-platelet aggregation activities have significant therapeutic implications for vascular thrombotic disorders and offer a possible path for the creation of new anti-platelet medications.
Angiogenesis is a hallmark of chronic inflammatory diseases (Britzen-Laurent et al., 2023). Pathological analysis of human and murine colitis tissues reveals significant alterations in the colonic microvascular system, including vascular dilation, congestion, edema, angiogenesis, microvascular occlusion, and the presence of tortuous vessels of varying diameters (Haep et al., 2015). These abnormal vascular changes precede the development of mucosal ulcers and significantly amplify intestinal inflammatory responses (Britzen-Laurent et al., 2023). In the colitis tissues of both mice and humans, vessels formed under the backdrop of chronic inflammation often exhibit an immature phenotype and are frequently associated with excessive thrombosis or vascular constriction (Langer et al., 2019). Therefore, the pathological generation of blood vessels both enhances inflammation and impairs mucosal healing, making it a critical factor in the chronic progression of UC (Sandor et al., 2006).
The application of anti-angiogenic agents is advantageous in the treatment of inflammatory diseases to some extent (Herrera-Gómez et al., 2022). Studies indicate that most quiescent and moderately active IBD patients exhibit good tolerance to anti-VEGF therapy. In vivo and vitro experiments based on inflammation models show that AT-I effectively inhibits angiogenesis in chronic inflammation by reducing the expression of NO, TNF-α, IL-1β, IL-6, VEGF and PIGF (Wang et al., 2009b). Research also suggests that AT-III exerts its anti-angiogenic effects through the direct inhibition of endothelial cells in vitro and vivo. Thus, inhibition of angiogenesis may be one of the mechanisms by which AT-I and AT-III exert their anti-UC effects. However, further validation is required to elucidate their efficacy and mechanisms.
Colitis-associated colorectal cancer (CAC) is a malignant condition of the colon that arises due to recurrent episodes of chronic intestinal inflammation. It represents one of the most severe complications of UC (Beaugerie and Itzkowitz, 2015). Chronic inflammation is recognized as one of the primary factors contributing to the development of cancer in humans, and long-term, irreversible damage to the gastrointestinal structure and function in UC patients elevates the risk of developing colorectal cancer (Wang S. Q. et al., 2019). Previous research has indicated a positive correlation between the incidence of colorectal cancer in Asian UC patients and the duration of their UC. As the duration of the disease increases, the incidence of colorectal cancer gradually rises. CAC differs from sporadic colorectal cancer in terms of its more severe pathological characteristics, worse prognosis, and a greater number of lesions (Terzić et al., 2010). Consequently, the reduction of UC duration, inhibition of inflammation-driven carcinogenesis, and prevention of CAC development play pivotal roles in the prevention and management of CAC.
Besides, AT-I has a pronounced inhibition of colon tumor formation in the AOM/DSS mouse model. In vivo and vitro experiments, AT-I treatment effectively suppresses colon tumor volume growth in AOM/DSS-induced mice, significantly reduces the cell viability of human HCT116 and SW480 cells, and induces apoptosis. The mechanism underlying these effects is associated with AT-I inhibiting the expression of NLRP3, Caspase-1, and ASC, as well as the subsequent release of IL-1β (Qin et al., 2021). Previous research has identified SIRT6 as a target effector of AT-I through molecular docking techniques. Further investigation reveals that AT-I enhances the deacetylase activity of SIRT6 in hepatocytes, promoting PPARα transcription and translation, thereby increasing the expression of its target genes to expedite fatty acid oxidation. Simultaneously, AT-I weakens NF-kB-mediated NLRP3 inflammasome formation, macrophage infiltration, and the expression levels of inflammatory cytokines such as TNF-α, IL-6 and IL-1β, thus suppressing hepatic inflammation and steatosis. Additionally, knocking out SIRT6 genes from mouse livers reduced the inhibitory effect of AT-I on NLRP3 inflammasomes and inflammatory responses induced by high-fat diets (Kong et al., 2022). Inflammasomes are believed to mediate host defense against microbial pathogens while maintaining intestinal homeostasis, excessive activation can lead to inflammatory diseases such as CRC (Beaugerie and Itzkowitz, 2015). Therefore, targeting the SIRT6/PPARα/NF-kB/NLRP3 pathway may be a potential way for AT-I to exert its anti-inflammatory and anti-cancer effects, which requires further validation. Studies also suggest that the inhibitory effect of AT-I on CAC is related to blocking Drp1-mediated mitochondrial fission (Qin et al., 2021). Thus, AT-I could serve as a potential effective anti-cancer drug, playing an important role in the treatment of UC and CAC.
Furthermore, research has reported that AT-Ⅰ exerts its anti-colorectal cancer effects through multiple mechanisms, including the inhibition of tumor cell proliferation, induction of cell death, regulation of cancer stemness, and enhancement of cancer cell immunogenicity (Li et al., 2020; Wang et al., 2020; Xu et al., 2021). It holds promise as a potential drug for combating colorectal cancer. AT-Ⅰ has inhibitory effects on various cancer cell types, and studies indicate that it inhibits tumor cells in breast cancer, lung cancer, ovarian cancer, and other malignancies through multiple pathways (Yao et al., 2019). Preliminary clinical trials using AT-Ⅰ purified extract in gastric cancer patients with cachexia suggest that AT-I contributes to alleviating cachexia symptoms (Liu et al., 2008). However, current clinical evidence is insufficient, and further research at the clinical level is required to confirm AT-I efficacy and safety.
AT-II can enhance the sensitivity of cancer cells to drugs. When the concentration of AT-II reaches 150 mg/L, it significantly inhibits the proliferation of colorectal cancer Lovo cells in a dose-dependent manner. In regard to AT-III, studies indicate that AT-III can promote the expression of pro-apoptotic genes such as Bax, caspase-2, and caspase-9 while inhibiting the expression of anti-apoptotic gene Bcl-2. It regulates the Bax/Bcl-2 apoptotic signaling pathway, thus promoting cell apoptosis and significantly inhibiting the growth of HCT-116 tumor xenografts in nude mice (Zhang et al., 2022). Therefore, AT-II and AT-III also hold potential as drugs for combating colorectal cancer, and their role in CAC warrants further research and evidence-based support. (Figures 4, 5).
Figure 4. Potential therapeutic mechanisms of ATs for UC and its complications. Note: These effects require validation in vivo.
The latest findings show that ATs have both similarities and distinctions in their pharmacological effects for treating UC. AT-I and AT-III act as antagonists of the TLR4 receptor on white blood cells, effectively reducing the release of pro-inflammatory factors by blocking the TLR-4/NF-kB/MAPK pathway. This indicates strong anti-inflammatory effects in experiments conducted in vitro and in vivo (Ji et al., 2014; Ji et al., 2016). Nrf2, a transcription factor, leading to notable antioxidant effects, can be activated by AT-III. Both AT-II and AT-III can trigger the Nrf2/HO-1 pathway to combat oxidative stress and improve colonic inflammation (Ren et al., 2021a). While both AT-I and AT-III can enhance beneficial bacteria abundance and diversity to modulate intestinal microbiota structure, their mechanisms differ slightly (Zhu and Li, 2012; Qu et al., 2022). AT-I, AT-II, and AT-III all promote epithelial cell repair to enhance intestinal barrier function (Song et al., 2017), although any differences in their repair-promoting effects remain unexplored at present. Overall, ATs exhibit promising potential as therapeutic agents for UC by significantly inhibiting its development through various pathways.
Ulcerative colitis (UC) is a chronic, recurrent disease with various complications that increase its clinical severity. Preventing and treating these complications are crucial in managing UC. AT-II and AT-III have significant anti-thrombotic effects (Chen et al., 2016a; Chen et al., 2016b), possibly through inhibiting the activation of the PI3K-Akt pathway. Studies show that patients with IBD have about three times higher risk of venous thromboembolism (VTE) compared to normal individuals, which increases during disease flares. Hospitalized IBD patients without active bleeding during flare-ups are recommended anticoagulant prophylaxis (Nguyen et al., 2014). AT-II and AT-III not only alleviate inflammation in UC but also inhibit platelet activation similarly to aspirin. They hold promise as potential drugs for treating UC and its thrombotic complications. In terms of anti-fibrosis effects, both AT-I and AT-III can inhibit EMT in IEC-6 cells and renal fibrosis through various pathways. Activation of Nrf2 may be one of the targets that AT-III exerts its anti-intestinal fibrosis effects (Huai and Ding, 2020; Guo et al., 2021; Huang et al., 2022). Research on AT-II’s anti-fibrotic properties is lacking currently. Both AT-I and AT-III possess anti-angiogenic effects (Wang et al., 2009b), although their efficacy in treating UC and its complications through this mechanism requires further validation. Notably, studies have shown that ATs inhibit cancer progression by targeting angiogenesis (Yang et al., 2021). Furthermore, CAC is one of the most severe complications in the development of UC, making effective prevention and treatment crucial (Keller et al., 2019). Research shows that post-treatment with AT-I effectively inhibits colon tumor growth in AOM/DSS-induced mice (Beaugerie and Itzkowitz, 2015). Clinical trials using purified AT-I solution suggest it helps alleviate cachexia symptoms in gastric cancer patients (Liu et al., 2008). While the therapeutic effects of AT-II and AT-III on CAC are not yet reported, their significant efficacy against colorectal cancer has been observed in vitro and vivo experiment (Zhang et al., 2022). Therefore, ATs not only have significant therapeutic effects on UC but also show promising potential in treating its complications, which could reduce the recurrence and improve the cure rate of UC.
Although ATs have advantages in the treatment of UC, their clinical application still has a long way to go. ATs are currently understudied and more studies are still needed to validate the efficacy and mechanisms of ATs in UC and its complications. Additionally, in vitro experiments have shown that ATs can be cytotoxic at certain doses (Ji et al., 2014; Huang et al., 2022). Cytotoxicity does not necessarily indicate adverse clinical reactions to the botanical drugs. However, experimental dosages of ATs (ranging from 5 to 50 mg/kg) vary among studies, making it difficult to determine the appropriate dosage range for treating UC. Therefore, further investigation on dosages and duration through controlled variables is necessary to provide guidance for safe clinical application. Lastly, current animal experiment results may not fully represent clinical effectiveness, and high-quality clinical trials are needed in the future to validate the safety and efficacy of ATs.
Furthermore, based on the current research findings of ATs, potential future research directions could be explored. Firstly, there are variations in the therapeutic effects among different ATs, thus, further validation is needed to determine if combining ATs could enhance treatment effects. Secondly, studies have shown that using ATs alone or in combination with cytotoxic drugs can help treat cancer or reduce side effects of radiotherapy and chemotherapy (Bailly, 2021). As the therapeutic advantages of ATs in UC and its complications, they may potentially serve as ideal adjunct medications for other UC treatments in the future, synergistically enhancing efficacy or reducing toxicity. In conclusion, ATs deserve further research in the future.
HQ: Conceptualization, Writing–original draft. ZY: Conceptualization, Writing–original draft. YH: Writing–review and editing. MW: Writing–review and editing. LC: Writing–review and editing. LL: Writing–review and editing. ZH: Visualization, Writing–review and editing. QZ: Visualization, Writing–review and editing. CZ: Visualization, Writing–review and editing. MY: Visualization, Writing–review and editing. XW: Visualization, Writing–review and editing. QY: Supervision, Writing–review and editing. KQ: Supervision, Writing–review and editing.
The author(s) declare that financial support was received for the research, authorship, and/or publication of this article. This study was supported by the National Natural Science Foundation of China (81973742), Xing Lin Scholars Program of Chengdu University of TCM (QJJJ2022002), Sichuan Provincial Natural Science Foundation (2023NSFSC0686), Chengdu University of TCM Joint Innovation Fund (LH202402022).
Figures 2–5 were created with BioRender.com (https://www.biorender.com/).
The authors declare that the research was conducted in the absence of any commercial or financial relationships that could be construed as a potential conflict of interest.
All claims expressed in this article are solely those of the authors and do not necessarily represent those of their affiliated organizations, or those of the publisher, the editors and the reviewers. Any product that may be evaluated in this article, or claim that may be made by its manufacturer, is not guaranteed or endorsed by the publisher.
The Supplementary Material for this article can be found online at: https://www.frontiersin.org/articles/10.3389/fphar.2024.1398294/full#supplementary-material
Abraham, B. P., Ahmed, T., and Ali, T. (2017). Inflammatory bowel disease: pathophysiology and current therapeutic approaches. Handb. Exp. Pharmacol. 239, 115–146. doi:10.1007/164_2016_122
Amirshahrokhi, K., and Imani, M. (2023). Levetiracetam attenuates experimental ulcerative colitis through promoting Nrf2/HO-1 antioxidant and inhibiting NF-κB, proinflammatory cytokines and iNOS/NO pathways. Int. Immunopharmacol. 119, 110165. doi:10.1016/j.intimp.2023.110165
Bailly, C. (2021). Atractylenolides, essential components of Atractylodes-based traditional herbal medicines: antioxidant, anti-inflammatory and anticancer properties. Eur. J. Pharmacol. 891, 173735. doi:10.1016/j.ejphar.2020.173735
Beaugerie, L., and Itzkowitz, S. H. (2015). Cancers complicating inflammatory bowel disease. N. Engl. J. Med. 372 (15), 1441–1452. doi:10.1056/NEJMra1403718
Britzen-Laurent, N., Weidinger, C., and Stürzl, M. (2023). Contribution of blood vessel activation, remodeling and barrier function to inflammatory bowel diseases. Int. J. Mol. Sci. 24 (6), 5517. doi:10.3390/ijms24065517
Burisch, J., Zhao, M., Odes, S., De Cruz, P., Vermeire, S., Bernstein, C. N., et al. (2023). The cost of inflammatory bowel disease in high-income settings: a Lancet Gastroenterology and Hepatology Commission. Lancet Gastroenterol. Hepatol. 8 (5), 458–492. doi:10.1016/s2468-1253(23)00003-1
Chen, E., Chuang, L. S., Giri, M., Villaverde, N., Hsu, N. Y., Sabic, K., et al. (2021a). Inflamed ulcerative colitis regions associated with MRGPRX2-mediated mast cell degranulation and cell activation modules, defining a new therapeutic target. Gastroenterology 160 (5), 1709–1724. doi:10.1053/j.gastro.2020.12.076
Chen, W., He, L., Zhong, L., Sun, J., Zhang, L., Wei, D., et al. (2021b). Identification of active compounds and mechanism of huangtu decoction for the treatment of ulcerative colitis by network pharmacology combined with experimental verification. Drug Des. Dev. Ther. 15, 4125–4140. doi:10.2147/DDDT.S328333
Chen, Y., Yang, W., Guo, L., Wu, X., Zhang, T., Liu, J., et al. (2017). Atractylodes lactone compounds inhibit platelet activation. Platelets 28 (2), 194–202. doi:10.1080/09537104.2016.1209477
Chen, Y. Z., Yang, W. L., and Guo, L. Y. (2016a). Antiplatelet effect of Atractylenolide II and its influence on the phosphorylation level of protein kinase B in platelets. Chin. J. Med. Guide 13 (11), 18–21+26.
Chen, Y. Z., Yang, W. L., and Guo, L. Y. (2016b). Antiplatelet effects and mechanisms of action of Atractylenolide III. Int. J. Pharm. Res. 43 (03), 514–517. doi:10.13220/j.cnki.jipr.2016.03.021
Cho, H. D., Kim, U., Suh, J. H., Eom, H. Y., Kim, J., Lee, S. G., et al. (2016). Classification of the medicinal plants of the genus Atractylodes using high-performance liquid chromatography with diode array and tandem mass spectrometry detection combined with multivariate statistical analysis. J. Sep. Sci. 39 (7), 1286–1294. doi:10.1002/jssc.201501279
Cunningham, K. E., Vincent, G., Sodhi, C. P., Novak, E. A., Ranganathan, S., Egan, C. E., et al. (2016). Peroxisome proliferator-activated receptor-γ coactivator 1-α (PGC1α) protects against experimental murine colitis. J. Biol. Chem. 291 (19), 10184–10200. doi:10.1074/jbc.M115.688812
Deng, M., Chen, H., Long, J., Song, J., Xie, L., and Li, X. (2021). Atractylenolides (I, II, and III): a review of their pharmacology and pharmacokinetics. Arch. Pharm. Res. 44 (7), 633–654. doi:10.1007/s12272-021-01342-6
Deng, S., Wang, A., Chen, X., Du, Q., Wu, Y., Chen, G., et al. (2019a). HBD inhibits the development of colitis-associated cancer in mice via the IL-6R/STAT3 signaling pathway. Int. J. Mol. Sci. 20 (5), 1069. doi:10.3390/ijms20051069
Deng, S., Wang, A. P., Chen, X., Wu, Y. L., and Li, Y. W. (2019b). Huangqi Baizhu decoction prevents CAC process by inhibiting MIR-31-5p/STAT3 positive circle. Chin. Pharmacol. Bull. 35 (9), 1308–1313. doi:10.3969/j.issn.1001-1978.2019.09.023
D'Errico, I., Salvatore, L., Murzilli, S., Lo Sasso, G., Latorre, D., Martelli, N., et al. (2011). Peroxisome proliferator-activated receptor-gamma coactivator 1-alpha (PGC1alpha) is a metabolic regulator of intestinal epithelial cell fate. Proc. Natl. Acad. Sci. U. S. A. 108 (16), 6603–6608. doi:10.1073/pnas.1016354108
Ekhtiar, M., Ghasemi-Dehnoo, M., Mirzaei, Y., Azadegan-Dehkordi, F., Amini-Khoei, H., Lorigooini, Z., et al. (2023). The coumaric acid and syringic acid ameliorate acetic acid-induced ulcerative colitis in rats via modulator of Nrf2/HO-1 and pro-inflammatory cytokines. Int. Immunopharmacol. 120, 110309. doi:10.1016/j.intimp.2023.110309
Feng, W. W., Ao, H., Yue, S. J., and Peng, C. (2018). Systems pharmacology reveals the unique mechanism features of Shenzhu Capsule for treatment of ulcerative colitis in comparison with synthetic drugs. Sci. Rep. 8, 16160. doi:10.1038/s41598-018-34509-1
Feuerstein, J. D., and Cheifetz, A. S. (2017). Crohn disease: epidemiology, diagnosis, and management. Mayo Clin. Proc. 92 (7), 1088–1103. doi:10.1016/j.mayocp.2017.04.010
Gao, X. X., Feng, J. Y., Yang, L., Wang, P., Jia, J. P., and Qin, X. M. (2018). Investigation on pharmacochemistry and pharmacokinetics of atractylenolides from Atractylodes in vivo based on UPLC-MS combined with everted gut sac model in vitro. J. Liq. Chromatogr. Relat. Technol. 41 (15-16), 892–902. doi:10.1080/10826076.2018.1537286
Geremia, A., Biancheri, P., Allan, P., Corazza, G. R., and Di Sabatino, A. (2014). Innate and adaptive immunity in inflammatory bowel disease. Autoimmun. Rev. 13 (1), 3–10. doi:10.1016/j.autrev.2013.06.004
Guo, Y., Xiao, Y., Zhu, H., Guo, H., Zhou, Y., Shentu, Y., et al. (2021). Inhibition of proliferation-linked signaling cascades with atractylenolide I reduces myofibroblastic phenotype and renal fibrosis. Biochem. Pharmacol. 183, 114344. doi:10.1016/j.bcp.2020.114344
Haep, L., Britzen-Laurent, N., Weber, T. G., Naschberger, E., Schaefer, A., Kremmer, E., et al. (2015). Interferon gamma counteracts the angiogenic switch and induces vascular permeability in dextran sulfate sodium colitis in mice. Inflamm. Bowel Dis. 21 (10), 2360–2371. doi:10.1097/MIB.0000000000000490
Hai, C. T., Luyen, N. T., Giang, D. H., Minh, B. Q., Trung, N. Q., Chinh, P. T., et al. (2023). Atractylodes macrocephala rhizomes contain anti-inflammatory sesquiterpenes. Chem. Pharm. Bull. (Tokyo) 71 (76), 451–453. doi:10.1248/cpb.c22-00779
Han, J., Li, W., Shi, G., Huang, Y., Sun, X., Sun, N., et al. (2022). Atractylenolide III improves mitochondrial function and protects against ulcerative colitis by activating AMPK/SIRT1/PGC-1α. Mediat. Inflamm. 2022, 9129984. doi:10.1155/2022/9129984
Han, K. H., Park, J. M., Jeong, M., Hang, Y. M., Go, E. J., Park, J., et al. (2017). Heme oxygenase-1 induction and anti-inflammatory actions of Atractylodes macrocephala and Taraxacum herba extracts prevented colitis and was more effective than sulfasalazine in preventing relapse. Gut Liver 11 (5), 655–666. doi:10.5009/gnl16496
Henderson, N. C., Rieder, F., and Wynn, T. A. (2020). Fibrosis: from mechanisms to medicines. Nature 587 (7835), 555–566. doi:10.1038/s41586-020-2938-9
Herrera-Gómez, R. G., Grecea, M., Gallois, C., Boige, V., Pautier, P., Pistilli, B., et al. (2022). Safety and efficacy of bevacizumab in cancer patients with inflammatory bowel disease. Cancers (Basel) 14 (12), 2914. doi:10.3390/cancers14122914
Ho, G. T., Aird, R. E., Liu, B., Boyapati, R. K., Kennedy, N. A., Dorward, D. A., et al. (2018). MDR1 deficiency impairs mitochondrial homeostasis and promotes intestinal inflammation. Mucosal Immunol. 11 (1), 120–130. doi:10.1038/mi.2017.31
Ho, G. T., and Theiss, A. L. (2022). Mitochondria and inflammatory bowel diseases: toward a stratified therapeutic intervention. Annu. Rev. Physiol. 84, 435–459. doi:10.1146/annurev-physiol-060821-083306
Hoang le, S., Tran, M. H., Lee, J. S., Ngo, Q. M., Woo, M. H., and Min, B. S. (2016). Inflammatory inhibitory activity of sesquiterpenoids from Atractylodes macrocephala rhizomes. Chem. Pharm. Bull. (Tokyo) 64 (5), 507–511. doi:10.1248/cpb.c15-00805
Huai, B., and Ding, J. (2020). Atractylenolide III attenuates bleomycin-induced experimental pulmonary fibrosis and oxidative stress in rat model via Nrf2/NQO1/HO-1 pathway activation. Immunopharmacol. Immunotoxicol. 42 (5), 436–444. doi:10.1080/08923973.2020.1806871
Huang, M., Jiang, W., Luo, C., Yang, M., and Ren, Y. (2022). Atractylenolide III inhibits epithelial-mesenchymal transition in small intestine epithelial cells by activating the AMPK signaling pathway. Mol. Med. Rep. 25 (3), 98. doi:10.3892/mmr.2022.12614
Iboshi, Y., Nakamura, K., Fukaura, K., Iwasa, T., Ogino, H., Sumida, Y., et al. (2017). Increased IL-17A/IL-17F expression ratio represents the key mucosal T helper/regulatory cell-related gene signature paralleling disease activity in ulcerative colitis. J. Gastroenterology 52 (3), 315–326. doi:10.1007/s00535-016-1221-1
Ji, G., Chen, R., and Zheng, J. (2014). Atractylenolide I inhibits lipopolysaccharide-induced inflammatory responses via mitogen-activated protein kinase pathways in RAW264.7 cells. Immunopharmacol. Immunotoxicol. 36 (6), 420–425. doi:10.3109/08923973.2014.968256
Ji, G. Q., Chen, R. Q., and Wang, L. (2016). Anti-inflammatory activity of atractylenolide III through inhibition of nuclear factor-κB and mitogen-activated protein kinase pathways in mouse macrophages. Immunopharmacol. Immunotoxicol. 38 (2), 98–102. doi:10.3109/08923973.2015.1122617
Jiang, Z., Peng, C., Huang, W., Wu, B., Zhang, D., Ouyang, H., et al. (2019). A high throughput three-step ultra-performance liquid chromatography tandem mass spectrometry method to study metabolites of atractylenolide-III. J. Chromatogr. Sci. 57 (2), 163–176. doi:10.1093/chromsci/bmy098
Kang, T. H., Han, N. R., Kim, H. M., and Jeong, H. J. (2011). Blockade of IL-6 secretion pathway by the sesquiterpenoid atractylenolide III. J. Nat. Prod. 74 (2), 223–227. doi:10.1021/np100686a
Keller, D. S., Windsor, A., Cohen, R., and Chand, M. (2019). Colorectal cancer in inflammatory bowel disease: review of the evidence. Tech. Coloproctol. 23 (1), 3–13. doi:10.1007/s10151-019-1926-2
Kim, J.-H., Lee, Y., Lee, G., Doh, E.-J., and Hong, S. (2018). Quantitative interrelation between atractylenolide I, II, and III in Atractylodes japonica koidzumi rhizomes, and evaluation of their oxidative transformation using a biomimetic kinetic model. ACS Omega 3 (11), 14833–14840. doi:10.1021/acsomega.8b02005
Kong, D., Mai, Z., Chen, Y., Luo, L., Liu, H., Zhao, L., et al. (2022). ATL I, acts as a SIRT6 activator to alleviate hepatic steatosis in mice via suppression of NLRP3 inflammasome formation. Pharm. (Basel) 15 (12), 1526. doi:10.3390/ph15121526
Langer, V., Vivi, E., Regensburger, D., Winkler, T. H., Waldner, M. J., Rath, T., et al. (2019). IFN-γ drives inflammatory bowel disease pathogenesis through VE-cadherin–directed vascular barrier disruption. J. Clin. Investigation 129 (11), 4691–4707. doi:10.1172/JCI124884
Laudadio, I., Bastianelli, A., Fulci, V., Carissimi, C., Colantoni, E., Palone, F., et al. (2022). ZNF281 promotes colon fibroblast activation in tgfβ1-induced gut fibrosis. Int. J. Mol. Sci. 23 (18), 10261. doi:10.3390/ijms231810261
Le Berre, C., Honap, S., and Peyrin-Biroulet, L. (2023). Ulcerative colitis. Lancet 402 (10401), 571–584. doi:10.1016/s0140-6736(23)00966-2
Li, C., Wang, J., Ma, R., Li, L., Wu, W., Cai, D., et al. (2022). Natural-derived alkaloids exhibit great potential in the treatment of ulcerative colitis. Pharmacol. Res. 175, 105972. doi:10.1016/j.phrs.2021.105972
Li, C. Q., He, L. c., and Den, T. (2006). Pharmacokinetics and tissue distribution of atractylenolide III in rats. Chin. Herb. Med. 29, 807–809. doi:10.13863/j.issn1001-4454.2006.08.024
Li, C. Q., He, L. C., Dong, H. Y., and Jin, J. Q. (2007a). Screening for the anti-inflammatory activity of fractions and compounds from Atractylodes macrocephala koidz. J. Ethnopharmacol. 114 (2), 212–217. doi:10.1016/j.jep.2007.08.002
Li, C. Q., He, L. C., and Jin, J. Q. (2007b). Atractylenolide I and atractylenolide III inhibit Lipopolysaccharide-induced TNF-alpha and NO production in macrophages. Phytother. Res. 21 (4), 347–353. doi:10.1002/ptr.2040
Li, Y., Liu, J., and Yang, X. W. (2013). Four new eudesmane-type sesquiterpenoid lactones from atractylenolide II by biotransformation of rat hepatic microsomes. J. Asian Nat. Prod. Res. 15 (4), 344–356. doi:10.1080/10286020.2013.764867
Li, Y., Wang, Y., Liu, Z., Guo, X., Miao, Z., and Ma, S. (2020). Atractylenolide I induces apoptosis and suppresses glycolysis by blocking the JAK2/STAT3 signaling pathway in colorectal cancer cells. Front. Pharmacol. 11, 273. doi:10.3389/fphar.2020.00273
Li, Y., and Yang, X.-W. (2013). Five new eudesmane-type sesquiterpenoid lactones biotransformed from atractylenolide I by rat hepatic microsomes. Fitoterapia 85, 95–100. doi:10.1016/j.fitote.2012.12.033
Li, Y., Zhang, Y., Wang, Z., Zhu, J., Tian, Y., and Chen, B. (2012). Quantitative analysis of atractylenolide I in rat plasma by LC–MS/MS method and its application to pharmacokinetic study. J. Pharm. Biomed. Analysis 58, 172–176. doi:10.1016/j.jpba.2011.09.023
Lin, Y., Zheng, X., Chen, J., Luo, D., Xie, J., Su, Z., et al. (2020). Protective effect of Bruguiera gymnorrhiza (L.) lam. Fruit on dextran sulfate sodium-induced ulcerative colitis in mice: role of keap1/nrf2 pathway and gut microbiota. Front. Pharmacol. 10, 1602. doi:10.3389/fphar.2019.01602
Liu, P., Zhao, G., Zhang, L., Gong, Y., and Gu, Y. (2021). Atractylenolide I inhibits antibiotic-induced dysbiosis of the intestinal microbiome. Ann. Transl. Med. 9 (20), 1539. doi:10.21037/atm-21-4656
Liu, Q., Zhang, S., Yang, X., Wang, R., Guo, W., Kong, W., et al. (2016). Differentiation of essential oils in Atractylodes lancea and Atractylodes koreana by gas chromatography with mass spectrometry. J. Sep. Sci. 39 (24), 4773–4780. doi:10.1002/jssc.201600574
Liu, Y., Jia, Z., Dong, L., Wang, R., and Qiu, G. (2008). A randomized pilot study of atractylenolide I on gastric cancer cachexia patients. Evid. Based Complement. Altern. Med. 5 (3), 337–344. doi:10.1093/ecam/nem031
Lu, Q., Xie, Y., Luo, J., Gong, Q., and Li, C. (2023). Natural flavones from edible and medicinal plants exhibit enormous potential to treat ulcerative colitis. Front. Pharmacol. 14, 1168990. doi:10.3389/fphar.2023.1168990
Maloy, K. J., and Powrie, F. (2011). Intestinal homeostasis and its breakdown in inflammatory bowel disease. Nature 474 (7351), 298–306. doi:10.1038/nature10208
Mirkov, M. U., Verstockt, B., and Cleynen, I. (2017). Genetics of inflammatory bowel disease: beyond NOD2. Lancet Gastroenterol. Hepatol. 2 (3), 224–234. doi:10.1016/s2468-1253(16)30111-x
Nakase, H., Sato, N., Mizuno, N., and Ikawa, Y. (2022). The influence of cytokines on the complex pathology of ulcerative colitis. Autoimmun. Rev. 21 (3), 103017. doi:10.1016/j.autrev.2021.103017
Nelson, A. D., Fluxá, D., Caldera, F., Farraye, F. A., Hashash, J. G., and Kröner, P. T. (2023). Thromboembolic events in hospitalized patients with inflammatory bowel disease. Dig. Dis. Sci. 68 (6), 2597–2603. doi:10.1007/s10620-023-07920-6
Neurath, M. F. (2014). Cytokines in inflammatory bowel disease. Nat. Rev. Immunol. 14 (5), 329–342. doi:10.1038/nri3661
Nguyen, G. C., Bernstein, C. N., Bitton, A., Chan, A. K., Griffiths, A. M., Leontiadis, G. I., et al. (2014). Consensus statements on the risk, prevention, and treatment of venous thromboembolism in inflammatory bowel disease: Canadian Association of Gastroenterology. Gastroenterology 146 (3), 835–848. doi:10.1053/j.gastro.2014.01.042
Ni, H. M., Williams, J. A., and Ding, W. X. (2015). Mitochondrial dynamics and mitochondrial quality control. Redox Biol. 4, 6–13. doi:10.1016/j.redox.2014.11.006
Ni, J., Wu, G. D., Albenberg, L., and Tomov, V. T. (2017). Gut microbiota and IBD: causation or correlation? Nat. Rev. Gastroenterol. Hepatol. 14 (10), 573–584. doi:10.1038/nrgastro.2017.88
Qin, Y., Yu, Y., Yang, C., Wang, Z., Yang, Y., Wang, C., et al. (2021). Atractylenolide I inhibits NLRP3 inflammasome activation in colitis-associated colorectal cancer via suppressing drp1-mediated mitochondrial fission. Front. Pharmacol. 12, 674340. doi:10.3389/fphar.2021.674340
Qu, L., Shi, K., Xu, J., Liu, C., Ke, C., Zhan, X., et al. (2022). Atractylenolide-1 targets SPHK1 and B4GALT2 to regulate intestinal metabolism and flora composition to improve inflammation in mice with colitis. Phytomedicine 98, 153945. doi:10.1016/j.phymed.2022.153945
Ren, Y., Jiang, W., Luo, C., Zhang, X., and Huang, M. (2021a). Atractylenolide III ameliorates TNBS-induced intestinal inflammation in mice by reducing oxidative stress and regulating intestinal flora. Chem. Biodivers. 18 (8), e2001001. doi:10.1002/cbdv.202001001
Ren, Y., Jiang, W., Luo, C., Zhang, X., and Huang, M. (2021b). The promotive effect of the active ingredients of Atractylodes macrocephala on intestinal epithelial repair through activating Ca2+ pathway. Nat. Product. Commun. 16 (11), 1934578X2110403. doi:10.1177/1934578X211040357
Rieder, F., Fiocchi, C., and Rogler, G. (2017). Mechanisms, management, and treatment of fibrosis in patients with inflammatory bowel diseases. Gastroenterology 152 (2), 340–350. doi:10.1053/j.gastro.2016.09.047
Sandor, Z., Deng, X. M., Khomenko, T., Tarnawski, A. S., and Szabo, S. (2006). Altered angiogenic balance in ulcerative colitis: a key to impaired healing? Biochem. Biophysical Res. Commun. 350 (1), 147–150. doi:10.1016/j.bbrc.2006.09.021
Santhanam, S., Rajamanickam, S., Motamarry, A., Ramakrishna, B. S., Amirtharaj, J. G., Ramachandran, A., et al. (2012). Mitochondrial electron transport chain complex dysfunction in the colonic mucosa in ulcerative colitis. Inflamm. Bowel Dis. 18 (11), 2158–2168. doi:10.1002/ibd.22926
Schwab, R., Lim, R., and Goldberg, R. (2019). Resolving intestinal fibrosis through regenerative medicine. Curr. Opin. Pharmacol. 49, 90–94. doi:10.1016/j.coph.2019.09.011
Shi, Y. Y., Guan, S. H., Tang, R. N., Tao, S. J., and Guo, D. A. (2012). Simultaneous determination of atractylenolide II and atractylenolide III by liquid chromatography-tandem mass spectrometry in rat plasma and its application in a pharmacokinetic study after oral administration of Atractylodes Macrocephala Rhizoma extract. Biomed. Chromatogr. 26 (11), 1386–1392. doi:10.1002/bmc.2709
Shimada, K., Crother, T. R., Karlin, J., Dagvadorj, J., Chiba, N., Chen, S., et al. (2012). Oxidized mitochondrial DNA activates the NLRP3 inflammasome during apoptosis. Immunity 36 (3), 401–414. doi:10.1016/j.immuni.2012.01.009
Song, H. P., Hou, X. Q., Li, R. Y., Yu, R., Li, X., Zhou, S. N., et al. (2017). Atractylenolide I stimulates intestinal epithelial repair through polyamine-mediated Ca(2+) signaling pathway. Phytomedicine 28, 27–35. doi:10.1016/j.phymed.2017.03.001
Terzić, J., Grivennikov, S., Karin, E., and Karin, M. (2010). Inflammation and colon cancer. Gastroenterology 138 (6), 2101–2114. doi:10.1053/j.gastro.2010.01.058
Tong, X., Zheng, Y., Li, Y., Xiong, Y., and Chen, D. (2021). Soluble ligands as drug targets for treatment of inflammatory bowel disease. Pharmacol. Ther. 226, 107859. doi:10.1016/j.pharmthera.2021.107859
Uhlig, H. H., and Powrie, F. (2018). Translating immunology into therapeutic concepts for inflammatory bowel disease. Annu. Rev. Immunol. 36 (1), 755–781. doi:10.1146/annurev-immunol-042617-053055
Uzzan, M., Martin, J. C., Mesin, L., Livanos, A. E., Castro-Dopico, T., Huang, R., et al. (2022). Ulcerative colitis is characterized by a plasmablast-skewed humoral response associated with disease activity. Nat. Med. 28 (4), 766–779. doi:10.1038/s41591-022-01680-y
Wang, C., Duan, H., and He, L. (2009a). Absorption kinetics of atractylenolide I in intestines of rats. Zhongguo Zhong Yao Za Zhi 34 (11), 1430–1434.
Wang, C., Duan, H., and He, L. (2009b). Inhibitory effect of atractylenolide I on angiogenesis in chronic inflammation in vivo and in vitro. Eur. J. Pharmacol. 612 (1-3), 143–152. doi:10.1016/j.ejphar.2009.04.001
Wang, G., Xu, B., Shi, F., Du, M., Li, Y., Yu, T., et al. (2019a). Protective effect of methane-rich saline on acetic acid-induced ulcerative colitis via blocking the TLR4/NF-κB/MAPK pathway and promoting IL-10/JAK1/STAT3-mediated anti-inflammatory response. Oxid. Med. Cell Longev. 2019, 7850324. doi:10.1155/2019/7850324
Wang, K., Huang, W., Sang, X., Wu, X., Shan, Q., Tang, D., et al. (2020). Atractylenolide I inhibits colorectal cancer cell proliferation by affecting metabolism and stemness via AKT/mTOR signaling. Phytomedicine 68, 153191. doi:10.1016/j.phymed.2020.153191
Wang, K. T., Chen, L. G., Wu, C. H., Chang, C. C., and Wang, C. C. (2010). Gastroprotective activity of atractylenolide III from Atractylodes ovata on ethanol-induced gastric ulcer in vitro and in vivo. J. Pharm. Pharmacol. 62 (3), 381–388. doi:10.1211/jpp.62.03.0014
Wang, P., Zhao, Y. N., Xu, R. Z., Zhang, X. W., Sun, Y. R., Feng, Q. M., et al. (2022). Sesquiterpene lactams and lactones with antioxidant potentials from Atractylodes macrocephala discovered by molecular networking strategy. Front. Nutr. 9, 865257. doi:10.3389/fnut.2022.865257
Wang, R., Luo, Y., Lu, Y., Wang, D., Wang, T., Pu, W., et al. (2019b). Maggot extracts alleviate inflammation and oxidative stress in acute experimental colitis via the activation of Nrf2. Oxid. Med. Cell Longev. 2019, 4703253. doi:10.1155/2019/4703253
Wang, R., Wang, D., Wang, H., Wang, T., Weng, Y., Zhang, Y., et al. (2021a). Therapeutic targeting of Nrf2 signaling by maggot extracts ameliorates inflammation-associated intestinal fibrosis in chronic DSS-induced colitis. Front. Immunol. 12, 670159. doi:10.3389/fimmu.2021.670159
Wang, S. Q., Cui, S. X., and Qu, X. J. (2019c). Metformin inhibited colitis and colitis-associated cancer (CAC) through protecting mitochondrial structures of colorectal epithelial cells in mice. Cancer Biol. Ther. 20 (3), 338–348. doi:10.1080/15384047.2018.1529108
Wang, S. S., Zhang, T., Wang, L., Dong, S., Wang, D. H., Li, B., et al. (2023). The dynamic changes in the main substances in Codonopsis pilosula root provide insights into the carbon flux between primary and secondary metabolism during different growth stages. Metabolites 13 (3), 456. doi:10.3390/metabo13030456
Wang, Y. M., Wang, Z. B., Sun, Y. P., Yang, B. Y., and Kuang, H. X. (2021b). Research progress on the chemical structures and biological activities of sesquiterpenoids in Atractylodes plants. Chin. Herb. Med. 52 (01), 299–309.
Wechsler, J. B., Szabo, A., Hsu, C. L., Krier-Burris, R. A., Schroeder, H. A., Wang, M. Y., et al. (2018). Histamine drives severity of innate inflammation via histamine 4 receptor in murine experimental colitis. Mucosal Immunol. 11 (3), 861–870. doi:10.1038/mi.2017.121
Xu, H., Van der Jeught, K., Zhou, Z., Zhang, L., Yu, T., Sun, Y., et al. (2021). Atractylenolide I enhances responsiveness to immune checkpoint blockade therapy by activating tumor antigen presentation. J. Clin. Invest. 131 (10), e146832. doi:10.1172/jci146832
Xu, J., Tang, C., Din, A. U., Lu, Y., Ma, X., Zhang, T., et al. (2023). Oligosaccharides of Polygonatum Cyrtonema Hua ameliorates dextran sulfate sodium-induced colitis and regulates the gut microbiota. Biomed. Pharmacother. 161, 114562. doi:10.1016/j.biopha.2023.114562
Xue, J. C., Yuan, S., Meng, H., Hou, X. T., Li, J., Zhang, H. M., et al. (2023). The role and mechanism of flavonoid herbal natural products in ulcerative colitis. Biomed. Pharmacother. 158, 114086. doi:10.1016/j.biopha.2022.114086
Yang, L., Yu, H., Hou, A., Man, W., Wang, S., Zhang, J., et al. (2021). A review of the Ethnopharmacology, phytochemistry, pharmacology, application, quality control, processing, toxicology, and pharmacokinetics of the dried rhizome of Atractylodes macrocephala. Front. Pharmacol. 12, 727154. doi:10.3389/fphar.2021.727154
Yang, Y., Wang, Y., Wang, T., Jiang, X., and Wang, L. (2017). Screening active components of modified Xiaoyao powder as NRF2 agonists. Cell Biochem. Funct. 35 (8), 518–526. doi:10.1002/cbf.3309
Yao, Z. M., Chen, W. D., Yang, Z. H., and Jiang, C. J. (2019). Research progress on Atractylodes macrocephala and the predictive analysis of its quality markers (Q-markers). Chin. Herb. Med. 50 (19), 4796–4807.
Yeganeh, P. R., Leahy, J., Spahis, S., Patey, N., Desjardins, Y., Roy, D., et al. (2018). Apple peel polyphenols reduce mitochondrial dysfunction in mice with DSS-induced ulcerative colitis. J. Nutr. Biochem. 57, 56–66. doi:10.1016/j.jnutbio.2018.03.008
Yoou, M. S., Nam, S. Y., Jin, M. H., Lee, S. Y., Kim, M. S., Roh, S. S., et al. (2017). Ameliorative effect of atractylenolide III in the mast cell proliferation induced by TSLP. Food Chem. Toxicol. 106 (Pt A), 78–85. doi:10.1016/j.fct.2017.05.042
Yu, W., Wang, G., Lu, C., Liu, C., Jiang, L., Jiang, Z., et al. (2022). Pharmacological mechanism of Shenlingbaizhu formula against experimental colitis. Phytomedicine 98, 153961. doi:10.1016/j.phymed.2022.153961
Zhang, D., Li, X., Song, D., Chen, S., Zhang, Z., Cao, S., et al. (2022). Atractylenolide III induces apoptosis by regulating the Bax/Bcl-2 signaling pathway in human colorectal cancer HCT-116 Cells in vitro and in vivo. Anticancer Drugs 33 (1), 30–47. doi:10.1097/cad.0000000000001136
Zhao, Y. Y., Zhang, J. Y., Zheng, K. Y., Gu, X., Wang, Q., Guo, L., et al. (2022). Chemical pattern recognition of Atractylodes chinensis from different producing areas and establishment of quantitative analysis of multi-components by single marker (QAMS) method for four components. Zhongguo Zhong Yao Za Zhi 47 (16), 4395–4402. doi:10.19540/j.cnki.cjcmm.20211217.201
Zhou, G. X., and Liu, Z. J. (2017). Potential roles of neutrophils in regulating intestinal mucosal inflammation of inflammatory bowel disease. J. Dig. Dis. 18 (9), 495–503. doi:10.1111/1751-2980.12540
Zhou, Y., Lu, X., Chen, L., Zhang, P., Zhou, J., Xiong, Q., et al. (2021). Polysaccharides from Chrysanthemun indicum L. enhance the accumulation of polysaccharide and atractylenolide in Atractylodes macrocephala Koidz. Int. J. Biol. Macromol. 190, 649–659. doi:10.1016/j.ijbiomac.2021.09.010
Zhu, H., and Li, Y. R. (2012). Oxidative stress and redox signaling mechanisms of inflammatory bowel disease: updated experimental and clinical evidence. Exp. Biol. Med. (Maywood) 237 (5), 474–480. doi:10.1258/ebm.2011.011358
Zou, M., and Zhu, Y. (2022). Exploring the molecular mechanism of tong xie yao fang in treating ulcerative colitis using network pharmacology and molecular docking. Evidence-based Complementary Altern. Med. 2022, 8141443. doi:10.1155/2022/8141443
Keywords: Atractylodes macrocephala Koidz., atractylenolides, ulcerative colitis, colitis-associated colorectal cancer, natural products
Citation: Qian H, Ye Z, Hu Y, Wu M, Chen L, Li L, Hu Z, Zhao Q, Zhang C, Yang M, Xudong W, Ye Q and Qin K (2024) Molecular targets associated with ulcerative colitis and the benefits of atractylenolides-based therapy. Front. Pharmacol. 15:1398294. doi: 10.3389/fphar.2024.1398294
Received: 09 March 2024; Accepted: 08 May 2024;
Published: 27 May 2024.
Edited by:
William Chi-Shing Tai, Hong Kong Polytechnic University, Hong Kong SAR, ChinaReviewed by:
Wei-Ting Kuo, National Taiwan University, TaiwanCopyright © 2024 Qian, Ye, Hu, Wu, Chen, Li, Hu, Zhao, Zhang, Yang, Xudong, Ye and Qin. This is an open-access article distributed under the terms of the Creative Commons Attribution License (CC BY). The use, distribution or reproduction in other forums is permitted, provided the original author(s) and the copyright owner(s) are credited and that the original publication in this journal is cited, in accordance with accepted academic practice. No use, distribution or reproduction is permitted which does not comply with these terms.
*Correspondence: Qiaobo Ye, eWVxaWFvYm9AY2R1dGNtLmVkdS5jbg==; Kaihua Qin, cWtoMDEzNUAxNjMuY29t
†These authors have contributed equally to this work and share first authorship
Disclaimer: All claims expressed in this article are solely those of the authors and do not necessarily represent those of their affiliated organizations, or those of the publisher, the editors and the reviewers. Any product that may be evaluated in this article or claim that may be made by its manufacturer is not guaranteed or endorsed by the publisher.
Research integrity at Frontiers
Learn more about the work of our research integrity team to safeguard the quality of each article we publish.