- 1Department of Neurology, First Affiliated Hospital of China Medical University, Shenyang, Liaoning, China
- 2Department of Cell Biology, Key Laboratory of Cell Biology, Ministry of Public Health, Shenyang, Liaoning, China
- 3Key Laboratory of Medical Cell Biology, Ministry of Education, China Medical University, Shenyang, Liaoning, China
Cerebral ischemia-reperfusion (I/R) injury is the predominant causes for the poor prognosis of ischemic stroke patients after reperfusion therapy. Currently, potent therapeutic interventions for cerebral I/R injury are still very limited. Melatonin, an endogenous hormone, was found to be valid in preventing I/R injury in a variety of organs. However, a systematic review covering all neuroprotective effects of melatonin in cerebral I/R injury has not been reported yet. Thus, we perform a comprehensive overview of the influence of melatonin on cerebral I/R injury by collecting all available literature exploring the latent effect of melatonin on cerebral I/R injury as well as ischemic stroke. In this systematic review, we outline the extensive scientific studies and summarize the beneficial functions of melatonin, including reducing infarct volume, decreasing brain edema, improving neurological functions and attenuating blood-brain barrier breakdown, as well as its key protective mechanisms on almost every aspect of cerebral I/R injury, including inhibiting oxidative stress, neuroinflammation, apoptosis, excessive autophagy, glutamate excitotoxicity and mitochondrial dysfunction. Subsequently, we also review the predictive and therapeutic implications of melatonin on ischemic stroke reported in clinical studies. We hope that our systematic review can provide the most comprehensive introduction of current advancements on melatonin in cerebral I/R injury and new insights into personalized diagnosis and treatment of ischemic stroke.
1 Introduction
Ischemic stroke is one of the main causes of mortality and the leading cause of adult disability worldwide. It affects the lives of millions of patients and imposes a heavy financial burden on the society (Regenhardt et al., 2018; Zhou et al., 2018; Datta et al., 2020). According to estimates in 2020, the average costs of hospitalization on ischemic stroke per person was amounted to $18,154 and the 3-year follow-up cost was $44,347 in the United States (Yousufuddin et al., 2020). Currently, therapeutic options for ischemic stroke are still very limited and the only drug authorized by FDA is recombinant tissue plasminogen activator (t-PA), which can accelerate clot dissolution and blood reperfusion. However, administration of t-PA is only suitable for about 5% of ischemic stroke patients due to the narrow therapeutic window (Zhou et al., 2018; Powers et al., 2019). Moreover, it may paradoxically result in cerebral I/R injury owing to blood flow restoration, which can lead to reactive oxygen free radical accumulation and thus cause brain edema, neurological deficits as well as individual death through a series of mechanisms, including oxidative stress, neuroinflammation, apoptosis, excessive autophagy, glutamate excitotoxicity, and mitochondrial dysfunction (Duehrkop and Rieben, 2014; Sarmah et al., 2018; Datta et al., 2020). Nowadays, a valid treatment for cerebral I/R injury has not been determined. Despite several substances have shown neuroprotective effects on cerebral I/R injury models, consistent results are not be obtained in further clinical trials. Thus, more potentially effective substances are being actively studied, among which melatonin is the most concerned.
Melatonin (N-acetyl-5-methoxytrytamine), an amine hormone discovered by Aaron Lerner half a century ago, is mostly secreted by the pineal gland and exists in extra-pineal sites including the retina, gastrointestinal tract, and bone marrow as well (Zawilska JolantaB. et al., 2009; Samantaray et al., 2009; Venegas et al., 2012). The timing of melatonin secretion adapts to the light-dark cycle (Zawilska, Skene, and Arendt, 2009a). Therefore, it is originally considered to be responsible for controlling circadian rhythms, including sleep-wake rhythm, neuroendocrine rhythm as well as seasonal response, by providing night information and mediating dark signals (Claustrat, Brun, and Chazot, 2005). Moreover, melatonin is involved in lowering core body temperature at night and promotes sleep tendency, whereas its secretion is disrupted upon exposure to light, thus causing wakefulness (Akerstedt et al., 1979). Additionally, melatonin plays important physiological roles in multiple other systems: cardiovascular system, respiratory system, reproductive system, immune system, and endocrine system, etc., For example, recent studies have indicated that melatonin could take participate in the regulation of blood pressure and heart rate via endothelium-dependent vasodilation and sympathovagal autonomic modulation (Girouard et al., 2001; Baker and Kimpinski, 2018). Besides, when combing with melatonin receptors on pancreatic islets, melatonin could induce the production of insulin growth factors, and subsequently promote insulin receptor tyrosine phosphorylation, thereby modulating insulin sensitivity and glucose homeostasis (Sharma et al., 2015).
To date, melatonin is thought to exert various physiological effects primarily through its action on two specific membrane receptors, MT1 and MT2. As members of the seven-transmembrane G protein-coupled receptor family, these two MT receptors are widely distributed throughout the central nervous system, including cerebral cortex, hippocampus, cerebellum, midbrain and especially hypothalamus and suprachiasmatic nucleus (Ekmekcioglu, 2006; Ng et al., 2017). Moreover, MT1 receptors are more abundant than MT2 receptors in most brain regions. Recent researches have demonstrated that in the various CNS regions, melatonin may exert multiple neuroprotective effects by direct binding to MT1 and MT2 receptors. In mouse primary neurons, Liu et al. observed that melatonin supplementation could activate Akt/GSK-3β/CRMP-2 signaling by acting on MT2 receptors, subsequently enhance excitatory synaptic transmission and thus promote functional and synaptic formation (Liu et al., 2015). Similarly, in APP/PS1 transgenic mice, melatonin administration could reverse mitochondrial dysfunction and decrease abnormal Aβ deposition in the cortex, striatum, and hippocampus brain region via MT2 receptor signaling, eventually improving cognitive and behavior deficits (Dragicevic et al., 2011). Additionally, in a transgenic mouse model of Huntington’s disease (HD), MT1 receptor levels in mice brain were much lower compared with wild-type controls. However, melatonin treatment could inhibit mutant huntingtin-induced caspase-3 activation and preserve MT1 receptor expression. Furthermore, melatonin would delay disease onset and mortality of HD mice, which was dependent on the presence and activation of MT1 receptor (Wang et al., 2011).
More importantly, emerging studies have confirmed that melatonin also plays an important effect in preventing I/R injury in a variety of organs including heart, liver, and brain (Tai et al., 2010; Kang et al., 2011; Zhang et al., 2019). Zhang et al. observed that melatonin administration contributed to improve mitochondrial fusion/mitophagy through inhibiting I/R-mediated Optic atrophy 1 (OPA1) downregulation, thereby maintaining myocardial function and cardiomyocyte viability in myocardial I/R injury (Zhang et al., 2019). Additionally, Kang et al. confirmed that melatonin could protect against hepatic I/R injury via mitigating Toll-like receptor 3 (TLR3) and Toll-like receptor 4 (TLR4) overexpression, which further inhibited TLR-mediated downstream inflammatory signaling cascades, including myeloid differentiation primary response 88 (MyD88)-dependent and toll-receptor-associated activator of interferon (TRIF)-dependent pathways (Kang, Koh, and Lee, 2011). As for cerebral I/R injury, Tai et al. demonstrated that melatonin reduced endothelial damage and preserved blood-brain barrier (BBB) integrity via attenuating matrix metalloproteinase-9 (MMP-9) protein expression and activity, thereby exerting neuroprotective effects (Tai et al., 2010). Nowadays, growing studies have demonstrated that melatonin-induced protective effects exert an important role in the brain. Thus, melatonin is expected to become a latent therapeutic agent in brain I/R injury. However, almost no systematic review that covering all protective effects of melatonin in cerebral I/R injury has been reported. Based on existing evidence, we conduct this comprehensive review aiming to study the neuroprotective effect of melatonin and the underlying molecular mechanisms, and explore the latent clinical application prospects of melatonin in cerebral I/R injury together with ischemic stroke.
2 Article search strategy process and study selection
A total of 920 articles are identified in PubMed database by using the following terms: melatonin and cerebral ischemia/brain ischemia/cerebral infarction/brain infarction/stroke/ischemia-reperfusion. Among them, by screening the titles and abstracts, 503 apparently irrelevant articles are excluded. 293 articles are further removed after reviewing the full-text articles for eligibility, among which 142 articles are reviews and meta-analyses, 8 articles are not related to melatonin, 90 articles are not related to cerebral I/R injury model or ischemic stroke model, including traumatic brain injury (TBI), neurodegenerative diseases, neonatal encephalopathy, 51 articles are not published in English, and 2 articles are with incomplete information. At last, 124 eligible studies are included in the review. Specifically, 32 studies are focusing on in vitro cerebral I/R injury model (Table 1), 98 studies on in vivo cerebral I/R injury model or ischemic stroke model (Table 2), 6 clinical studies are related to cerebral I/R injury or ischemic stroke. The flow of screening process is shown in Figure 1.
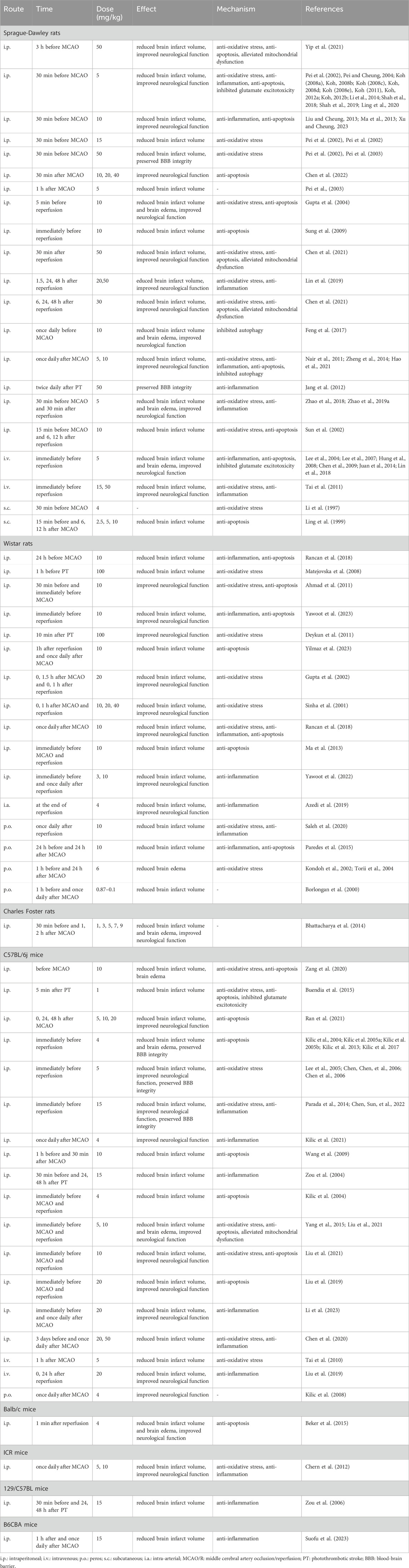
TABLE 2. Protective effects of melatonin on in vivo cerebral I/R injury model or ischemic stroke model.
3 Protective effects of melatonin in experimental I/R injury models
During ischemic stroke, blood supply for the brain is interrupted, which can cause irretrievable tissue damage. Ischemic tissue injury is commonly attributed to tissue hypoxia and the subsequent cellular ATP depletion, which may in turn cause death of neurons and glia and then lead to the brain tissue infarction as well as neurological deficits. After reperfusion, the generation of reactive oxygen species (ROS) is at an accelerated rate in tissues (Granger and Kvietys, 2015). The central nervous system (CNS) is particularly sensitive to ROS because it contains high concentrations of substances that promote oxidative processes, including iron, ascorbic acid, and polyunsaturated fatty acids, while low amounts of endogenous antioxidants (Watson et al., 2016). ROS accumulation can cause reperfusion injury, including programmed cell death and necrosis in the brain through triggering a cascade characterized by ionic imbalance, mitochondrial disturbances, oxidative stress and endoplasmic reticulum (ER) stress (Murphy and Steenbergen, 2008; Bagheri et al., 2016; Lin et al., 2018). Thus, protecting CNS cells from I/R injury is considered as an important therapeutic strategy for ischemic stroke treatment. For investigations of cerebral I/R injury, the preferred in vivo model is middle cerebral artery occlusion (MCAO), including transient MCAO and permanent MCAO (Lemmerman et al., 2022). Besides, neurons and glial cells subjected to oxygen-glucose deprivation/reoxygenation (OGD/R) are common in vitro models, allowing us to investigate the specific feature of different cell types.
As a free radical scavenger and antioxidant, melatonin has been inferred to exert a neuroprotective effect in cerebral I/R injury. Up till now, large amounts of studies have indicated that treatment with melatonin before and after OGD can exert neuroprotective effects in CNS cells. Detailed characteristics of included studies are shown in Table 1 (Vlkolinsky and Stolc, 1999; Pei and Cheung, 2003; Andrabi et al., 2004; Uchida et al., 2004; Duan et al., 2006; Gasparova, Stolc, and Snirc, 2006; Han et al., 2006; Wang et al., 2009; Guo et al., 2010; Tai et al., 2011; Parada et al., 2014; Buendia et al., 2015; Huang et al., 2015; Patino et al., 2016; Suofu et al., 2017; Carloni et al., 2018; Lin et al., 2018; Azedi et al., 2019; Beker et al., 2019; Liu, Ran, et al., 2019; Wei et al., 2019; Xing et al., 2019; Xiang et al., 2020; Yang, Zang, et al., 2020; Zhi et al., 2020; Liu, Cao, Gao, Li, Xia, et al., 2021; Liu, Cao, Gao, Li, Zeng, et al., 2021; Nasoni et al., 2021; Ran et al., 2021; Luchetti et al., 2022; Sun et al., 2022; Su et al., 2023). Lin et al. reported that exposure of cultured neurons obtained from the cerebral cortices of Sprague-Dawley rats to 30 min of OGD and 1, 2, 4, and 24 h of reoxygenation caused the increase of cell apoptosis, while pretreatment with melatonin (10–500 µM) before OGD/R for 30 min could promote the survival of neurons (Y.W. Lin et al., 2018). Subsequently, Yang et al. confirmed that compared with control groups, pretreatment with melatonin (50 µM) in the PC12 cell line exposed to OGD for 30 min and reoxygenation for 24 h had a lower neuronal apoptosis rate (Yang, Zang, et al., 2020). In addition, in cultured neurons from CA1 and CA3 hippocampal regions, administration of melatonin (1, 3, 10, and 30 µM) after OGD/R could lead to a decrease in OGD/R-induced neuronal injury and death compared to control groups (Patino et al., 2016). Similarly, consistent results were also observed in a study using melatonin-treated SH-SY5Y cell line models performed by Zhi et al. (Zhi et al., 2020). Apart from neuronal cells, the neuroprotective effect of melatonin has also been confirmed in glial cells. In BV-2 cell line, pretreatment with melatonin (10, 100, and 300 µM) at 24 h prior to OGD/R was found to increase the viability of cells exposed to 3 h of OGD and 3, 6, and 24 h of reoxygenation (Azedi et al., 2019).
More importantly, emerging studies have indicated that melatonin exert neuroprotective effects in stroke animals (Table 2) (Li et al., 1997; Kilic et al., 1999; Ling et al., 1999; Borlongan et al., 2000; Sinha et al., 2001; Gupta et al., 2002; Kondoh et al., 2002; Pei et al., 2002; Pei et al., 2002; Sun et al., 2002; Pe and Cheung, 2003; Pei et al., 2003; Andrabi et al., 2004; Gupta et al., 2004; Lee et al., 2004; Pei and Cheung, 2004; Torii et al., 2004; Zou et al., 2004; Kilic E. et al., 2005; Kilic U. et al., 2005; Lee et al., 2005; Chen et al., 2006; Chen et al., 2006; Zou et al., 2006; Lee et al., 2007; Koh, 2008a; Koh, 2008b; Koh, 2008c; Koh, 2008d; Koh, 2008e; Hung et al., 2008; Kilic et al., 2008; Matejovska et al., 2008; Chen et al., 2009; Sung et al., 2009; Wang et al., 2009; Tai et al., 2010; Ahmad et al., 2011; Deykun et al., 2011; Koh, 2011; Nair et al., 2011; Tai et al., 2011; Koh, 2012a; Koh, 2012b; Chern et al., 2012; Jang et al., 2012; Kilic et al., 2012; Kilic et al., 2013; Liu and Cheung, 2013; Ma et al., 2013; Bhattacharya et al., 2014; Juan et al., 2014; Li et al., 2014; Zheng et al., 2014; Beker et al., 2015; Buendia et al., 2015; Paredes et al., 2015; Yang et al., 2015; Chumboatong et al., 2017; Feng et al., 2017; Kilic et al., 2017; Lin et al., 2018; Rancan et al., 2018; Shah et al., 2018; Zhao et al., 2018; Azedi et al., 2019; Zhao et al., 2019a; Lin et al., 2019; Liu, Chen, et al., 2019; Shah et al., 2019; Wei et al., 2019; Chen et al., 2020; Chumboatong et al., 2020; Ling et al., 2020; Saleh et al., 2020; Yang et al., 2020; Zang et al., 2020; Chen et al., 2021; Hao et al., 2021; Kilic et al., 2021; Liu et al., 2021; Liu et al., 2021; Ran et al., 2021; Shao et al., 2021; Yip et al., 2021; Cambiaghi, Cherchi, and Comai, 2022; Chen et al., 2022; Chen et al., 2022; Kilic et al., 2022; Yawoot et al., 2022; Li et al., 2023; Su et al., 2023; Suofu et al., 2023; Xu and Cheung, 2023; Yawoot et al., 2023; Yilmaz et al., 2023). For example, mice that exposed to focal cerebral ischemia for 30 min and reperfusion for 72 h exhibited brain damage and neurological dysfunction, while intraperitoneal administration of melatonin (4 mg/kg) prior to MCAO could decrease the neurological deficits by maintaining the integrity of BBB and reducing brain edema formation as well as infarct volume (Kilic et al., 2017). Besides, in a rat MCAO/R model, treatment with melatonin (5 mg/kg) intraperitoneally at the 30 min after I/R significantly reduced cerebral infarct volumes, promoted myelination as well as alleviated white matter damage (Zhao et al., 2019a). Furthermore, Liu et al. demonstrated that in C57BL/6J mice receiving MCAO/R, not only the cerebral infarct size was reduced, but the sensorimotor function was also improved after intraperitoneal injection of melatonin (Liu et al., 2019).
4 The mechanism of melatonin’s neuroprotective effect in cerebral I/R injury
Melatonin could exert neuroprotective effects through various mechanisms in cerebral I/R injury (Figure 2).
4.1 Oxidative stress
Oxidative stress is characterized by excessive ROS and reactive nitrogen species (RNS) production under harmful stimuli. During cerebral I/R injury, ROS overproduction causes immense damage to brain (Manzanero et al., 2013). Compelling evidence suggests that, as a powerful scavenger against ROS/RNS, melatonin can inhibit oxidative stress in cerebral I/R injury via multiple mechanisms, among which nuclear factor E2-related factor-2 (Nrf2) occupies a vital position.
Melatonin can exert neuroprotective effects based on the upregulation of Nrf2 and Nrf2-related antioxidants during cerebral oxidative stress injury. Specifically, melatonin could reduce the ubiquitination of Nrf2 via modifying Kelch-like ECH-associated protein 1 (Keap1) cysteine residues, thereby reducing Nrf2 degradation by proteasome and enhancing Nrf2 phosphorylation and nuclear translocation to the nucleus (Zhao et al., 2018). In addition, melatonin can promote Ca2+ influx and induce phosphorylation of protein kinase C (PKC), an upstream mediator of Nrf2, which further leads to the downstream Nrf2 nuclear translocation (Romero et al., 2010). Moreover, melatonin can directly serve as a proteasome inhibitor to decrease the degradation of Nrf2 (Vriend and Reiter, 2014).
Nrf2, an antioxidative transcription factor, can positively regulate the expression of a series of antioxidant response element (ARE)-dependent genes. Melatonin induced Nrf2 translocation and upregulation could increase antioxidant enzymes expression levels that comprise glutathione (GSH), glutathione s-transferase (GST), superoxide dismutase (SOD), catalase (CAT), heme oxygenase-1(HO-1) and glutathione peroxidase (GPx), etc (Rodriguez et al., 2004; Ma, 2013; Zhang et al., 2013). These upregulated enzymes effectively scavenge free radicals as well as related reactants and ultimately reduce the cerebral damage during the oxidative stress process (Deng et al., 2015; Liu et al., 2019).
As shown in a former study by Parada et al., in hippocampal cultures exposed to OGD/R, administration with melatonin could induce the Nrf2 nuclear accumulation as well as the combination of Nrf2 and ARE sequences within HO-1 promoter, thereby elevating HO-1 expression and decreasing the production of OGD-induced ROS to basal levels (Parada et al., 2014). During heme metabolic process, HO-1 can reduce the ROS by degrading free heme. Meanwhile, the by-products of heme catabolism such as carbon monoxide (CO) and biliverdin (BV)/bilirubin (BR) can not only directly scavenge OGD-induced ROS production, but also inhibit NADPH oxidase activity, further alleviating oxidative stress (Kim et al., 2011). Additionally, Nrf2α subunit was found to bind with sirtuin-3 (SIRT3) promoter directly, leading to the upregulation of SIRT3 expression. And the elevation of SIRT3 further activated SOD through promoting SOD deacetylation and then reduced mitochondrial oxidative stress (Satterstrom et al., 2015; He et al., 2019). Moreover, another possible mechanism by which melatonin suppresses oxidative stress involves increasing GSH content (Paterniti et al., 2016). Nrf2 can promote GSH biosynthesis enzymes and GSH reductase (GSR) expression, and thus exerts a valid effect in preserving the mitochondrial GSH pool (Ryoo and Kwak, 2018). High concentrations of GSH are oxidized to glutathione disulfide (GSSG) by GPx, thereby reducing peroxides to non-toxic substances and alleviating oxidative stress (Harvey et al., 2009).
In addition to activating Nrf2, studies have also demonstrated that administration with melatonin can play protective effects via inhibiting NADPH oxidase, which can produce ROS upon activation (Wilkinson and Landreth, 2006; Li et al., 2014). Specifically, in a rat MCAO/R model, by hindering phosphatidylinositol 3-kinase (PI3K)/Akt signaling pathway, melatonin can suppress the p47phox subunit phosphorylation, and thus block the combination of p47phox with gp91phox subunit. This process disturbs the assembly and activation of NADPH oxidase, ultimately alleviating oxidative stress (Li et al., 2014).
4.2 Neuroinflammation
Neuroinflammation is the inflammatory response concentrated in the CNS and might be initiated from ischemia. It is featured as the accumulation of pro-inflammatory factors, comprise IL-1β, IL-6, TNF-α and inducible nitric oxide synthase (iNOS) that are generated from activated microglia and astrocytes after ischemic stroke (Jin et al., 2010; DiSabato et al., 2016; Yang and Zhou, 2019). Melatonin can hinder microglia and astrocytes phenotype switching through multiple mechanisms, further attenuating neuroinflammation.
During the I/R process, two different forms of microglia are involved, including pro-inflammatory microglia (M1) and anti-inflammatory microglia (M2) phenotypes. Under the ischemic conditions, M2 phenotype transforms into the active M1 phenotype, which promotes further pro-inflammatory cytokines release and eventually accelerates stroke-induced secondary brain injury (Jin, Yang, and Li, 2010). The switch and balance between the M1/M2 phenotype can be modulated by melatonin through various mechanisms, including inhibiting nuclear factor kappa-B (NF-κΒ), activating signal transducer and activator of transcription 3 (STAT3) and interaction with MT2 receptor. Detailly, Zhao et al. demonstrated that in MCAO/R rats, melatonin could inhibit TLR4 expression, further hindering downstream NF-κΒ activation. NF-κΒ inactivation can initiate the transformation of microglia from M1 to M2 phenotype, thereby down-regulating the release of M1 phenotype-related pro-inflammatory factors (Y. Zhao et al., 2019; J. Sun et al., 2019; Ji et al., 2017). In addition, treatment with melatonin was also confirmed to significantly upregulate the expression of phosphorylated STAT3 in microglial cells (Z.J. Liu, Ran, et al., 2019). The phosphorylation of STAT3 has been demonstrated to exert a crucial effect in IL-10-induced anti-inflammatory effects. Specifically, removing the docking sites of STAT3 from IL-10R1 would render the receptor unable to transduce the signal for suppressing cytokine synthesis. Thus, the activated STAT3 may contribute to the anti-inflammatory cytokines expression which induces microglia transforming from M1 to M2 phenotype and further inhibits neuroinflammation (Williams et al., 2004). Besides, in a rat MCAO/R model, melatonin was found to act on MT2 and increase the ratio of triggering receptor expressed on myeloid cells 2 (TREM2)/iNOS, a marker for the transformation of M1 to M2, which eventually resulted in the suppression of neuroinflammation (Azedi et al., 2019).
Melatonin also exerts anti-inflammatory effect through suppressing the proliferation and activation of astrocytes. Regarding astrocytes, they are involved in BBB formation and maintenance, synaptogenesis, neurotransmission, and metabolic regulation. Under ischemic conditions, cytokines produced by activated microglia cause astrocyte reactivity hyperplasia and transform into neurotoxic reactive astrocytes, which further result in cerebral damage (Liu and Chopp, 2016; Liddelow et al., 2017; Yang et al., 2020). Chen et al. reported that administration with melatonin attenuated TRIF expression, an adapter molecule of TLRs, and ultimately prevented the conversion of astrocytes from anti-inflammatory (A2) to pro-inflammatory (A1) phenotype (Chen et al., 2020). In addition, in rats subjected to MCAO/R, melatonin administration could effectively decrease glial fibrillary acid protein (GFAP) expression, C3, and S100A10, suggesting that melatonin inhibited reactive astrogliosis and A1 astrocyte polarization (Yawoot et al., 2022). Besides, melatonin was also shown to reduce astrocyte-mediated inflammatory response by inhibiting glycogen synthase kinase-3 beta (GSK-3β) expression levels and receptor-interacting serine/threonine-protein 1 kinase (RIP1K) activities, consequently enhanced axonal regeneration and promoted neurobehavioral recovery (Yawoot et al., 2022). Mechanistically, suppression of GSK-3β can reduce NF-κB nuclear translocation and upregulate cyclic AMP response element-binding protein (CREB) transcription. These cause a relative increase in CREB compared to NF-κB in the nucleus, resulting in increased binding of CREB Binding Protein (CBP) with CREB and decreased interaction between CBP and NF-κB p65. The increased combination of CBP and CREB produces high levels of IL-10, a critical anti-inflammatory factor (Maixner and Weng, 2013). Meanwhile, the reduced interaction of CBP with NF-κB p65 inhibits the pro-inflammatory factors, and thus alleviates neuroinflammation (Martin et al., 2005).
4.3 Apoptosis
Apoptosis is an ATP-dependent death program characterized by fragmentation of chromosomal DNA, degradation of cytoskeletal, and generation of apoptotic bodies (Elmore, 2007; Xu et al., 2019). Regarding the apoptosis pathways, two major patterns are widely accepted including the extrinsic pathway and the intrinsic pathway. The extrinsic pathway refers to receptor-mediated apoptosis, and the activation of death receptors involves in two main ligands, including tumor necrosis factor (TNF) and Fas. Ischemia can induce the combination of the ligands with death receptors which lead to apoptosis and eventually cerebral injury. While in the intrinsic pathway, ischemia could directly induce the generation of intracellular signals and the subsequent formation of mitochondrial permeability transition pore, induce cytochrome C release into cytoplasm and activate caspase-3, and eventually lead to apoptosis (Broughton et al., 2009). In addition, members of Bcl-2 protein family, such as Bim, Bad, Bid, Bcl-x (L), and Bcl-2, are responsible for regulation of apoptosis via modifying the permeability of mitochondrial membrane (Cory and Adams, 2002; Elmore, 2007).
Previous studies have reported that melatonin could play anti-apoptotic functions during cerebral I/R injury through multiple biological mechanisms, including activation of the PI3K/Akt, Raf/MEK/extracellular-regulated kinase (ERK) signaling pathway as well as inactivation of Jun N-terminal kinases (JNK) pathway (Koh, 2008c). In 2008, Koh et al. elucidated that pretreatment with melatonin in the rat model of MCAO-induced cerebral ischemia could significantly reduce the infarct volume by activating Akt. Specifically, melatonin administration can promote the phosphorylation of specific residues on forkhead rhabdomyosarcoma transcription factors (FKHR) by activating PI3K/Akt. Then, 14-3-3 protein, an anti-apoptotic factor, would directly interact with the phosphorylated FKHR and anchor the phosphorylated FKHR within the cytoplasm. These FKHR are blocked from further translocation into the nucleus, therefore inhibiting downstream target genes transcription, including Fas ligand (Koh, 2008d). In 2021, Ran et al. elucidated that both in MCAO/R mice model and N2a cells exposed to OGD/R, treatment with melatonin could significantly reduce the brain infarct volume and neuronal cells apoptosis by activating Akt. Specifically, melatonin administration can decrease phosphatase and tensin homolog (PTEN) deleted on chromosome 10 activity through promoting its phosphorylation. PTEN is a major up-stream negative regulator of the PI3K/Akt signal transduction which can promote the dephosphorylation of PIP3. In the presence of melatonin, phosphorylated PTEN levels were increased, thereby decreasing PTEN activity and activating PI3K/Akt pathway (Ran et al., 2021). Similarly, in a MCAO/R mouse model, melatonin was shown to activate the phosphorylation of Akt at Thr308 via PI3K/pyruvate dehydrogenase kinase-1 (PDK-1) signaling, which further inactivate GSK-3α/β, two critical players in the activation of apoptosis (Kilic et al., 2017).
Besides, in the cerebral MCAO model, melatonin was found to increase the phosphorylation of pro-apoptotic protein Bad through activating PI3K/Akt, further preventing Bad from interacting with Bcl-x(L) and blocking the activation of downstream apoptosis pathway (Koh, 2008b).
Apart from activating PI3K/Akt pathway, Koh et al. also demonstrated that in a rat MCAO model, treatment with melatonin can protect brain tissue from ischemic injury through Raf/MEK/ERK signaling pathway by modulating the pro-apoptotic protein phosphorylation. To some extent, melatonin can increase the phosphorylation of Raf-1 as well as MEK. And the activation of these two proteins kinases can phosphorylate ERK1/2 which results in the phosphorylation of its downstream target 90 ribosomal S6 kinase (90RSK) (Koh, 2008a). The phosphorylated 90RSK may further phosphorylate the Ser112 residue of Bad and inactivate the pro-apoptotic effect of Bad (Zha et al., 1996; Koh, 2008a). Moreover, Chen et al. reported that melatonin can effectively suppress fork-head box O3a (FoxO3a) activity by inhibiting JNK, thereby hindering the binding of FoxO3a to Bim promoters and the subsequent expression of Bim proapoptotic protein (Chen et al., 2022). Besides, JNK has been shown to regulate cytochrome C release from mitochondria through inducing Bid cleavage. Melatonin could attenuate JNK activation, thereby hindering cytochrome C release and suppressing cytochrome C-induced apoptosis cascades (Madesh et al., 2002; Song et al., 2014).
4.4 Autophagy
Autophagy is a lysosome-mediated self-digestion and recycling process that contributes to clearing folded or aggregated proteins and promoting the degradation of damaged organelles. It plays a key role in preserving cellular homeostasis under physiological conditions (Glick et al., 2010; Boga et al., 2019). However, under pathological circumstances including cerebral I/R injury, ischemia-mediated excessive autophagy activation may be harmful and further lead to cell death. Autophagy begins with nucleation of the phagophore induced by the unc-51-like autophagy activating kinase 1/2 (ULK1/2) complex. Then, the phagophore elongates with the aid of autophagy-related (ATG) proteins and finally the expanding membrane forms an autophagosome which closes around its cargo. The outer membrane of the autophagosome subsequently fuses with lysosomal membrane, and further generate an autolysosome, lead to autophagic cargo degradation (Itakura and Mizushima, 2010; Wong et al., 2013; Parzych and Klionsky, 2014).
Accumulating evidences have demonstrated that melatonin could promote physiological autophagy, while inhibiting excessive autophagy to exert neuroprotective effects. On the one hand, in hippocampal HT22 cells exposed to OGD/R, melatonin was shown to increase the expression of both Ras-related protein 7 (Rab7) and transcription factor Forkhead box class O1 (FoxO1), subsequently promoting autophagosome maturation and attenuating ischemic-like injury (Luchetti et al., 2022). Furthermore, both in a MCAO/R model and HT22 cell line exposed to OGD/R, treatment with melatonin upregulated SIRT1, a histone deacetylase that can cause the deacetylation of brain and muscle ARNT-like protein 1 (BMAL1) and decrease its degradation. The upregulation of BMAL1 could promote the expression of ATG14 through directly binding to the E-box elements in the ATG14 promoter, subsequently increasing the level of Beclin-1 and the ratio of LC3II/LC3I, enhancing autophagy and improving neurological function (Liu et al., 2021). On the other hand, melatonin could activate mammalian target of rapamycin complex1 (mTORC1) through multiple pathways, thereby hindering excessive autophagy and exerting neuroprotective effects. Specifically, in a rat MCAO/R model, melatonin was observed to decrease the phosphorylation of tuberous sclerosis complex 2 (TSC2) through PI3K/Akt signaling pathway. Therefore, the downstream protein complex TSC1/TSC2 was disrupted, leading to stabilization of the downstream Rheb-GTPase and subsequent activation of mTORC1 (Yang and Klionsky, 2010; Zheng et al., 2014). The mTORC1 can inactivate the autophagy initiators ATG13 and ULK1/2 through combination and phosphorylation, regulate the class III PI3K complex, and thus exerts an important effect in inhibiting autophagy (Rabanal-Ruiz, Otten, and Korolchuk, 2017). Likewise, mTORC1 can also phosphorylate transcription factor EB (TFEB) and TFE3 which facilitates the interaction between these two transcription factors and cytosolic chaperone 14-3-3 and retains them in cytoplasm (Martina et al., 2012). Therefore, the expression of several autophagy-related genes including PIK3C3 and ATG16L1, etc., are inhibited, thereby suppressing excessive autophagy and reducing cerebral injury (Settembre et al., 2011).
In addition to activating mTORC1 signaling pathway, melatonin could also attenuate excessive autophagy through hindering JNK signaling pathway, according to previous studies. Melatonin can inactivate JNK1, which further inhibits the separation of Bcl-2 from Beclin-1. Beclin-1 acts as a part of class III PI3K complex, and the combination of Bcl-2 and Beclin-1 can hinder class III PI3K complex activation, thereby inhibiting autophagy (H.D. Xu and Qin, 2019; Zheng et al., 2014). Similarly, in a rat MCAO/R model, Feng et al. reported that pretreatment with melatonin could inhibit pancreatic ER kinase (PKR)-like kinase (PERK)/Inositol-requiring enzyme 1 (IRE1) pathway, further suppressing the activation of the JNK, subsequently preventing the uncoupling of Bcl-2 with Beclin-1 and excessive autophagy (Feng et al., 2017). Moreover, in a rat MCAO/R model and PC12 cell OGD/R model, pretreatment with melatonin was shown to inhibit excessive autophagy through upregulating miR-26a-5p expression and then downregulating downstream neuron-restrictive silence factor (NRSF) expression (Yang et al., 2020).
4.5 Glutamate excitotoxicity
Glutamate acts as the predominant excitatory neurotransmitter in the CNS (Teichberg et al., 2009). During cerebral I/R injury, ischemia can lead to the excessive glutamate release, which triggers the rapid influx of calcium into the cell cytoplasm through the overstimulation of N-methyl-D-aspartate receptors (NMDARs), thereby resulting in a succession of harmful signaling cascades and subsequent CNS cells death (Tehse and Taghibiglou, 2019; M. Zhou and Baudry, 2006; Sattler and Tymianski, 2000). Moreover, glutamate can also cause the overproduction of nitric oxide (NO) and the decrease of downstream mediator protein phosphatase 2A (PP2A) which result in neurotoxicity and cerebral injury (Koh, 2012a; Jia et al., 2015). Melatonin can reduce glutamate excitotoxicity during cerebral I/R injury through modulating glutamate as well as its receptor pathways.
Detailly, Patino et al. confirmed that in rat hippocampal slices exposed to OGD/R, melatonin could directly reduce the release of glutamate and prevent a persistent activation of NMDARs, thereby reducing intracellular Ca2+ levels (Patino et al., 2016). Similarly, in hippocampal slices exposed to OGD/R, melatonin reduced a surge of synaptic glutamate release and neuronal cell death after ischemia-reperfusion, which further strongly suppressed the increase in the intracellular Ca2+ concentration by downregulating the NMDAR activity (Furuta et al., 2022). Besides, in hippocampal HT22 cell lines exposed to glutamate excitotoxicity, melatonin pretreatment could prevent glutamate-mediated reduction in calcium-buffering proteins such as parvalbumin and hippocalcin and thus downregulate Ca2+ levels (Koh, 2012b). Similarly, in Sprague-Dawley rat model subjected to MCAO, melatonin has also been documented to reduce intracellular Ca2+ levels through increasing the expression of parvalbumin and hippocalcin (Koh, 2012b). Additionally, melatonin can directly bind with calreticulin and further reduce the level of Ca2+ by hindering the release of Ca2+ from endoplasmic reticulum, in rat C6 astroglial cells exposed to glutamate excitotoxicity (Das et al., 2008; Venkatesan et al., 2021). With the decrease of Ca2+ levels, calpain expression and caspase-3 activation are inhibited. Calpain inhibition subsequently reduces the degradation of cytoskeletal proteins as well as axon-myelin structural unit, thus maintaining the structural integrity of CNS cells and preventing glutamate excitotoxicity (Samantaray et al., 2008; Doshi and Lynch, 2009).
Moreover, in a MCAO rat model, melatonin was found to interact with calmodulin, further preventing the binding of calmodulin to NOS, and thus inhibited calmodulin-dependent NOS activation (Koh, 2008e). Apart from inhibiting NOS activity, Koh et al. suggested that in a rat focal cerebral ischemia model, treatment with melatonin could mitigate glutamate-induced decrease of PP2A subunit B, a critical subunit in facilitating various functions of PP2A. As an essential protein phosphatase, PP2A can promote DNA repair, cellular proliferation and differentiation. From this point of view, melatonin can maintain PP2A levels, thereby promoting neuronal survival and alleviating glutamate excitotoxicity (Koh, 2012a).
4.6 Mitochondrial dysfunction
Mitochondria serve as essential organelles that exert a crucial effect in maintaining energy metabolism and cellular homeostasis (Anderson and Sims, 1999; Lesnefsky et al., 2017). Under normal circumstances, mitochondria produce ATP through the electron transport in the respiratory chain to keep cellular function and integrity (Newmeyer and Ferguson-Miller, 2003). Meanwhile, mitochondria could release pro-apoptotic factors, and thus control cell survival (Ott et al., 2002). However, under pathological conditions such as cerebral I/R injury, ischemia can lead to mitochondrial dysfunction, including impaired ability to generate ATP, accumulation of ROS, transition of the mitochondrial permeability and increased release of pro-apoptotic factors (Sims and Anderson, 2002; Ham and Raju, 2017). Melatonin has been found to play neuroprotective effects by improving ischemia-induced disturbance in mitochondrial redox state, fusion and fission, biogenesis, mitophagy and mitochondrial transfer.
Firstly, as a potent free radical scavenger, melatonin has the capacity to directly counteract mitochondrial oxidative injury. In a MCAO/R mice model, melatonin pretreatment significantly increased SIRT1 expression and thus reduced the expression of acetylated p53 and NF-κB. Subsequently, the deacetylated p53 and NF-κB maintained mitochondrial membrane potential, elevated the activity of mitochondrial Complex I and reduced mitochondrial ROS levels (Yang et al., 2015). Apart from alleviating mitochondrial oxidative damage, in N2a neuroblastoma cells exposed to OGD/R as well as a MCAO/R rat model, melatonin was shown to upregulate OPA1 expression, one of the mitochondrial fusion-related proteins, by activating Hippo/Yap pathway. Then, the mitochondrial cristae junctions are tightened and cytochrome c release is restricted, thereby improving OPA1-related mitochondrial fusion and inhibiting mitochondrial fission (Wei et al., 2019; Nasoni et al., 2021). Moreover, Nasoni et al. reported that in hippocampal HT22 cells with excessive mitochondrial oxidative stress, melatonin was found to enhance the levels of peroxisome proliferator-activated receptor gamma coactivator 1 alpha (PGC-1α) expression. Subsequently, PGC-1α can further enhance the combination of estrogen-related receptor (ERR) α with ERR binding element. Thus, SIRT3 expression is initiated which further induces mitochondrial biogenesis through activating nuclear respiratory factors-1 (NRF-1) and nuclear respiratory factors-2 (NRF-2) and subsequently increasing expression of mitochondrial transcription factor A (mtTFA) (Kong et al., 2010; Nasoni et al., 2021). Besides, in HT22 cells exposed to OGD/R, melatonin was shown to promote mitochondrial transfer and reshape mitochondrial network via increasing the number of tunneling nanotubes (TNTs). TNTs are dynamic structures that connect cells, and intercellular mitochondria could transfer through these structures, which further compensate for damaged organelles and promote cell recovery (Nasoni et al., 2021). Lastly, in HT22 neuronal cell line with glutamate injury, melatonin could upregulate the levels of Bcl-2 expression and downregulate the levels of Beclin-1 expression, thereby further reducing glutamate-induced mitophagy and restoring mitochondrial function (Wang et al., 2019). In addition, in PC12 cell line treated with ropivacaine, melatonin was shown to reduce the expression of two mitophagy-related proteins, PTEN-induced kinase I (PINK1) and Parkin, which may hinder the occurrence of mitophagy (Yu et al., 2018; Wang et al., 2019; Zeng et al., 2022).
5 Clinical prospects
In recent years, numerous clinical investigations exploring the influence of melatonin on ischemic stroke and the sequelae of ischemic stroke have been reported. Firstly, a series of preclinical studies have shown melatonin was an extremely safe neurotherapeutic agent even at high concentrations (Romero et al., 2016). So far, melatonin has been applied to treat a variety of diseases, involving sleep disorders, depression, etc., and no clinical studies on melatonin therapy have shown serious side effects (Cardinali et al., 2012; Hassell et al., 2015; Riha, 2018). Secondly, pineal calcifications diagnosed by applying multi-spiral computer and/or magnetic resonance tomography has been described as having a significant impact on the risk of ischemic stroke (Kitkhuandee et al., 2014). Besides, subsequent studies confirmed a positive relationship between pineal calcifications and decrease of melatonin synthesis (Schmid et al., 1994). Thus, human clinical trials of ischemic stroke to evaluate potential clinical applications of melatonin seemed to be necessary. Current studies have found that endogenous melatonin, mainly produced by the pineal gland, might be a good predictor for the prognosis of ischemic stroke. In a prospective observational study published in 2018, Lorente et al. found that in patients with severe middle cerebral artery infarction, serum melatonin concentrations were associated with total antioxidant capacity as well as malondialdehyde levels (used to assess lipid peroxidation). Furthermore, results of this research also illustrated a positive correlation between serum melatonin levels and the severity or even mortality of stroke patients during the 30-day follow-up period (Lorente et al., 2018). For this point of view, we speculated that patients with more severe ischemic stroke would produce high levels of ROS, which might result in higher concentrations of serum melatonin to compensate for the increase in oxidation products. Among them, when the attempts to maintain a balance between oxidation and antioxidant status were insufficient, the patients with severe ischemic stroke would eventually die. Additionally, a case-control study involving 42 patients with ischemic stroke demonstrated that the levels of melatonin in the urine was remarkably decreased in contrast to the control value. It was reasonable to suspect that due to the excessive free radical production, the catabolism of melatonin might be speeded up during acute ischemic stroke, indicating the vital role of melatonin in scavenging free radicals and neuroprotection (Ritzenthaler et al., 2013).
A previous systematic review focusing on clinical trials of melatonin on various brain injury provided evidence that melatonin treatment improved sleep disturbance following traumatic brain injury and increased survival rate in intubated patients with hemorrhagic stroke and asphyxiated newborns (Ramos et al., 2020). Regarding the clinical potential of exogenous melatonin therapy on cerebral I/R injury and ischemic stroke, a double-blind randomized controlled trial recruited 60 patients, who orally received melatonin at 6 mg/day for 3 days before and after carotid endarterectomy, to explore the therapeutic effect of melatonin on cerebral I/R injury (Yang et al., 2018). The circulating levels of inflammatory cytokines in patients of the melatonin group were significantly reduced, implying that melatonin could decrease the inflammatory damage and ameliorate subsequent brain I/R injury. Furthermore, Mehrpooya et al. recruited 65 patients with acute ischemic stroke who were not eligible for reperfusion treatment in a double-blind placebo-controlled trial. They observed that melatonin supplementation within 24 h after stroke at a dose of 20 mg once daily for 5 days had a higher reduction in modified Rankin Scale score (mRS) at 30 and 90 days after treatment, indicating melatonin had beneficial effects on neurological recovery after ischemic stroke (Mehrpooya et al., 2022). As one of the holy grails for acute ischemic stroke treatment, neuroprotection could theoretically improve the neurological disability of stroke survivors, which makes it attract widespread attention. According to previous studies, therapeutic hypothermia can maintain organ vitality and is one of the most powerful neuroprotective treatments (Perlman et al., 2010; Kurisu and Yenari, 2018). Although no clinical researches involving in the neuroprotective effect of melatonin combined with hypothermic therapy in cerebral I/R injury treatment following ischemic stroke, a clinical trial recruiting 30 newborns with confirmed hypoxic-ischemic encephalopathy demonstrated that early use of melatonin combined with hypothermia could lower plasma free radicals generation, protect against the subsequent CNS injury and ultimately improve survival rate of patients (Aly et al., 2015). Additionally, as a common and severe complication of ischemic stroke, poststroke delirium (PSD) has attracted wide attention from researchers due to it was related to increased mortality, longer hospital stays, and lower functional outcome (Siddiqi et al., 2006; Shaw et al., 2019). A propensity score-matched analysis involving 573 patients with acute ischemic stroke showed that patients who received prophylactic treatment with melatonin within 24 h after stroke onset had a lower risk of PSD compared with patients receiving standard treatment (Mengel et al., 2021). Although the underlying mechanism of melatonin for preventing PSD was still unclear, the evidence of this propensity score-matched analysis implied that supplementation with melatonin could reduce PSD susceptibility, and further supported the neuroprotective effect of melatonin on acute ischemic stroke. Taken together, recent clinical studies have partially clarified the predictive and therapeutic potential of melatonin for ischemic stroke. Nevertheless, further studies, ideally enrolling more clinical subjects, are still needed to evaluate the clinical safety and effectiveness of melatonin to better understand its medical effects in cerebral I/R injury and ischemic stroke.
6 Conclusion
In the past decades, ischemic stroke has been one of the most threatening diseases for human health. Due to its high morbidity and mortality, efforts have been made for detecting appropriate alternative or complementary drugs on ischemic stroke therapy. Currently, accumulated lines of evidence suggested that melatonin could reduce cell death and increase cell viability, and display an obvious neuroprotective effect on various CNS cells in vitro. Meanwhile, experimental stroke models in vivo have confirmed that melatonin would reduce infarct size and brain edema, and improve neurological function. Subsequently, we also outlined the extensive studies and performed a comprehensive introduction of various functions of melatonin in cerebral I/R injury, including regulating oxidative stress, neuroinflammation, apoptosis, autophagy, glutamate excitotoxicity and mitochondrial dysfunction. Meanwhile, some key signaling pathways has been demonstrated to play important roles in melatonin neuroprotection, such as Nrf2, NF-κΒ, PI3K/Akt, Raf/MEK/ERK, JNK, and Hippo/Yap pathways. In future studies, the biomolecular mechanism of melatonin in cerebral I/R damage still needs in-depth research, which is necessary for its clinical application in ischemic stroke.
Given its multiple roles against cerebral I/R damage, it is not surprisingly that melatonin has been considered as a promising candidate, both as a diagnostic biomarker for stroke prognosis and as a drug target for stroke therapy. Recent studies have demonstrated a potential relationship between circulating melatonin levels and the severity and mortality of stroke patients. Regarding exogenous melatonin therapy, oral melatonin in patients undergoing carotid endarterectomy could reduce serum pro-inflammatory cytokines levels and alleviate cerebral I/R injury to a certain extent. Moreover, early treatment with melatonin following stroke may help improve functional recovery in patients ineligible for reperfusion therapy. However, there are still some limitations to the clinical application of melatonin, and some potential issues should be considered in future clinical trials. First, high-quality randomized controlled trials with larger sample sizes and more ethnic groups are needed to further explore the clinical efficacy and side effects of melatonin in patients with ischemic stroke. Second, inappropriate treatment time windows, insufficient dosage or treatment duration may be responsible for the lack of positive results in clinical trials. Thus, the dosage, timing, and duration of melatonin require further reconsideration to determine the optimal application strategy in stroke patients. Third, melatonin might be more effective when combined with other types of stroke treatments, such as hypothermia or hyperbaric oxygen therapy, and the effectiveness of combination therapy should be fully investigated in the future.
In summary, based on the information reviewed above, as a low toxicity and well-tolerated agent, melatonin supplement was supposed to be a new and prospective treatment method for cerebral I/R injury. More randomized and multiple-center clinical trials were need to be designed to confirm predictive and therapeutic role of melatonin in ischemic stroke.
Author contributions
CZ: Visualization, Writing–original draft. YM: Visualization, Writing–original draft. YZ: Visualization, Writing–original draft. NG: Visualization, Writing–original draft. CH: Visualization, Writing–original draft. QW: Visualization, Writing–original draft. CM: Visualization, Writing–original draft. YZ: Investigation, Supervision, Writing–review and editing. ST: Investigation, Supervision, Writing–review and editing. JZ: Conceptualization, Investigation, Project administration, Supervision, Visualization, Writing–original draft, Writing–review and editing. XL: Conceptualization, Investigation, Project administration, Supervision, Visualization, Writing–original draft, Writing–review and editing.
Funding
The author(s) declare financial support was received for the research, authorship, and/or publication of this article. This work was supported by the National Natural Science Foundation of China (Grant No. 81400950), Natural Science Foundation of Liaoning Province (Grant No. 2019-MS-365).
Conflict of interest
The authors declare that the research was conducted in the absence of any commercial or financial relationships that could be construed as a potential conflict of interest.
The author(s) declared that they were an editorial board member of Frontiers, at the time of submission. This had no impact on the peer review process and the final decision.
Publisher’s note
All claims expressed in this article are solely those of the authors and do not necessarily represent those of their affiliated organizations, or those of the publisher, the editors and the reviewers. Any product that may be evaluated in this article, or claim that may be made by its manufacturer, is not guaranteed or endorsed by the publisher.
References
Ahmad, A., Khan, M. M., Ishrat, T., Khan, M. B., Khuwaja, G., Raza, S. S., et al. (2011). Synergistic effect of selenium and melatonin on neuroprotection in cerebral ischemia in rats. Biol. Trace Elem. Res. 139 (1), 81–96. doi:10.1007/s12011-010-8643-z
Akerstedt, T., Froberg, J. E., Friberg, Y., and Wetterberg, L. (1979). Melatonin excretion, body temperature and subjective arousal during 64 hours of sleep deprivation. Psychoneuroendocrinology 4 (3), 219–225. doi:10.1016/0306-4530(79)90005-2
Aly, H., Elmahdy, H., El-Dib, M., Rowisha, M., Awny, M., El-Gohary, T., et al. (2015). Melatonin use for neuroprotection in perinatal asphyxia: a randomized controlled pilot study. J. Perinatol. 35 (3), 186–191. doi:10.1038/jp.2014.186
Anderson, M. F., and Sims, N. R. (1999). Mitochondrial respiratory function and cell death in focal cerebral ischemia. J. Neurochem. 73 (3), 1189–1199. doi:10.1046/j.1471-4159.1999.0731189.x
Andrabi, S. A., Sayeed, I., Siemen, D., Wolf, G., and Horn, T. F. (2004). Direct inhibition of the mitochondrial permeability transition pore: a possible mechanism responsible for anti-apoptotic effects of melatonin. FASEB J. 18 (7), 869–871. doi:10.1096/fj.03-1031fje
Azedi, F., Mehrpour, M., Talebi, S., Zendedel, A., Kazemnejad, S., Mousavizadeh, K., et al. (2019). Melatonin regulates neuroinflammation ischemic stroke damage through interactions with microglia in reperfusion phase. Brain Res. 1723, 146401. doi:10.1016/j.brainres.2019.146401
Bagheri, F., Khori, V., Alizadeh, A. M., Khalighfard, S., Khodayari, S., and Khodayari, H. (2016). Reactive oxygen species-mediated cardiac-reperfusion injury: mechanisms and therapies. Life Sci. 165 (2), 43–55. doi:10.1016/j.lfs.2016.09.013
Baker, J., and Kimpinski, K. (2018). Role of melatonin in blood pressure regulation: an adjunct anti-hypertensive agent. Clin. Exp. Pharmacol. Physiol. 45 (8), 755–766. doi:10.1111/1440-1681.12942
Beker, M. C., Caglayan, A. B., Kelestemur, T., Caglayan, B., Yalcin, E., Yulug, B., et al. (2015). Effects of normobaric oxygen and melatonin on reperfusion injury: role of cerebral microcirculation. Oncotarget 6 (31), 30604–30614. doi:10.18632/oncotarget.5773
Beker, M. C., Caglayan, B., Caglayan, A. B., Kelestemur, T., Yalcin, E., Caglayan, A., et al. (2019). Interaction of melatonin and Bmal1 in the regulation of PI3K/AKT pathway components and cellular survival. Sci. Rep. 9 (1), 19082. doi:10.1038/s41598-019-55663-0
Bhattacharya, P., Pandey, A. K., Paul, S., and Patnaik, R. (2014). Melatonin renders neuroprotection by protein kinase C mediated aquaporin-4 inhibition in animal model of focal cerebral ischemia. Life Sci. 100 (2), 97–109. doi:10.1016/j.lfs.2014.01.085
Boga, J. A., Caballero, B., Potes, Y., Perez-Martinez, Z., Reiter, R. J., Vega-Naredo, I., et al. (2019). Therapeutic potential of melatonin related to its role as an autophagy regulator: a review. J. Pineal Res. 66 (1), e12534. doi:10.1111/jpi.12534
Borlongan, C. V., Yamamoto, M., Takei, N., Kumazaki, M., Ungsuparkorn, C., Hida, H., et al. (2000). Glial cell survival is enhanced during melatonin-induced neuroprotection against cerebral ischemia. FASEB J. 14 (10), 1307–1317. doi:10.1096/fj.14.10.1307
Broughton, B. R., Reutens, D. C., and Sobey, C. G. (2009). Apoptotic mechanisms after cerebral ischemia. Stroke 40 (5), e331–e339. doi:10.1161/STROKEAHA.108.531632
Buendia, I., Gomez-Rangel, V., Gonzalez-Lafuente, L., Parada, E., Leon, R., Gameiro, I., et al. (2015). Neuroprotective mechanism of the novel melatonin derivative Neu-P11 in brain ischemia related models. Neuropharmacology 99, 187–195. doi:10.1016/j.neuropharm.2015.07.014
Cambiaghi, M., Cherchi, L., and Comai, S. (2022). Photothrombotic mouse models for the study of melatonin as a therapeutic tool after ischemic stroke. Methods Mol. Biol. 2550, 433–441. doi:10.1007/978-1-0716-2593-4_42
Cardinali, D. P., Srinivasan, V., Brzezinski, A., and Brown, G. M. (2012). Melatonin and its analogs in insomnia and depression. J. Pineal Res. 52 (4), 365–375. doi:10.1111/j.1600-079X.2011.00962.x
Carloni, S., Facchinetti, F., Pelizzi, N., Buonocore, G., and Balduini, W. (2018). Melatonin acts in synergy with hypothermia to reduce oxygen-glucose deprivation-induced cell death in rat Hippocampus organotypic slice cultures. Neonatology 114 (4), 364–371. doi:10.1159/000491859
Chen, H. Y., Chen, T. Y., Lee, M. Y., Chen, S. T., Hsu, Y. S., Kuo, Y. L., et al. (2006). Melatonin decreases neurovascular oxidative/nitrosative damage and protects against early increases in the blood-brain barrier permeability after transient focal cerebral ischemia in mice. J. Pineal Res. 41 (2), 175–182. doi:10.1111/j.1600-079X.2006.00351.x
Chen, H. Y., Hung, Y. C., Chen, T. Y., Huang, S. Y., Wang, Y. H., Lee, W. T., et al. (2009). Melatonin improves presynaptic protein, SNAP-25, expression and dendritic spine density and enhances functional and electrophysiological recovery following transient focal cerebral ischemia in rats. J. Pineal Res. 47 (3), 260–270. doi:10.1111/j.1600-079X.2009.00709.x
Chen, K. H., Chai, H. T., Chen, C. H., Huang, C. R., Chiang, J. Y., Sung, P. H., et al. (2021). Synergic effect of combined cyclosporin and melatonin protects the brain against acute ischemic reperfusion injury. Biomed. Pharmacother. 136, 111266. doi:10.1016/j.biopha.2021.111266
Chen, K. H., Lin, K. C., Ko, S. F., Chiang, J. Y., Guo, J., and Yip, H. K. (2020). Melatonin against acute ischaemic stroke dependently via suppressing both inflammatory and oxidative stress downstream signallings. J. Cell. Mol. Med. 24 (18), 10402–10419. doi:10.1111/jcmm.15654
Chen, S., Sun, Y., Li, F., Zhang, X., Hu, X., Zhao, X., et al. (2022). Modulation of α7nAchR by melatonin alleviates ischemia and reperfusion-compromised integrity of blood-brain barrier through inhibiting HMGB1-mediated microglia activation and CRTC1-mediated neuronal loss. Cell. Mol. Neurobiol. 42 (7), 2407–2422. doi:10.1007/s10571-021-01122-2
Chen, T. Y., Lee, M. Y., Chen, H. Y., Kuo, Y. L., Lin, S. C., Wu, T. S., et al. (2006). Melatonin attenuates the postischemic increase in blood-brain barrier permeability and decreases hemorrhagic transformation of tissue-plasminogen activator therapy following ischemic stroke in mice. J. Pineal Res. 40 (3), 242–250. doi:10.1111/j.1600-079X.2005.00307.x
Chen, X., Shen, X., Lai, J., Yao, Z., Peng, X., Wu, L., et al. (2022). Influence of melatonin on behavioral and neurological function of rats with focal cerebral ischemia-reperfusion injury via the JNK/FoxO3a/Bim pathway. Comput. Math. Methods Med. 2022, 8202975. doi:10.1155/2022/8202975
Chern, C. M., Liao, J. F., Wang, Y. H., and Shen, Y. C. (2012). Melatonin ameliorates neural function by promoting endogenous neurogenesis through the MT2 melatonin receptor in ischemic-stroke mice. Free Radic. Biol. Med. 52 (9), 1634–1647. doi:10.1016/j.freeradbiomed.2012.01.030
Chumboatong, W., Khamchai, S., Tocharus, C., Govitrapong, P., and Tocharus, J. (2020). Agomelatine protects against permanent cerebral ischaemia via the Nrf2-HO-1 pathway. Eur. J. Pharmacol. 874, 173028. doi:10.1016/j.ejphar.2020.173028
Chumboatong, W., Thummayot, S., Govitrapong, P., Tocharus, C., Jittiwat, J., and Tocharus, J. (2017). Neuroprotection of agomelatine against cerebral ischemia/reperfusion injury through an antiapoptotic pathway in rat. Neurochem. Int. 102, 114–122. doi:10.1016/j.neuint.2016.12.011
Claustrat, B., Brun, J., and Chazot, G. (2005). The basic physiology and pathophysiology of melatonin. Sleep. Med. Rev. 9 (1), 11–24. doi:10.1016/j.smrv.2004.08.001
Cory, S., and Adams, J. M. (2002). The Bcl2 family: regulators of the cellular life-or-death switch. Nat. Rev. Cancer 2 (9), 647–656. doi:10.1038/nrc883
Das, A., Belagodu, A., Reiter, R. J., Ray, S. K., and Banik, N. L. (2008). Cytoprotective effects of melatonin on C6 astroglial cells exposed to glutamate excitotoxicity and oxidative stress. J. Pineal Res. 45 (2), 117–124. doi:10.1111/j.1600-079X.2008.00582.x
Datta, A., Sarmah, D., Mounica, L., Kaur, H., Kesharwani, R., Verma, G., et al. (2020). Cell death pathways in ischemic stroke and targeted pharmacotherapy. Translational stroke research. doi:10.1007/s12975-020-00806-z
Deng, Y., Zhu, J., Mi, C., Xu, B., Jiao, C., Li, Y., et al. (2015). Melatonin antagonizes Mn-induced oxidative injury through the activation of keap1-Nrf2-ARE signaling pathway in the striatum of mice. Neurotox. Res. 27 (2), 156–171. doi:10.1007/s12640-014-9489-5
Deykun, K., Pometlova, M., Schutova, B., and Mares, J. (2011). Modulations of behavioral consequences of minor cortical ischemic lesion by application of free radicals scavengers. Gen. Physiol. Biophys. 30 (3), 263–270. doi:10.4149/gpb_2011_03_263
DiSabato, D. J., Quan, N., and Godbout, J. P. (2016). Neuroinflammation: the devil is in the details. J. Neurochem. 139 (Suppl. 2), 136–153. doi:10.1111/jnc.13607
Doshi, S., and Lynch, D. R. (2009). Calpain and the glutamatergic synapse. Front. Biosci. Sch. Ed. 1 (2), 466–476. doi:10.2741/s38
Dragicevic, N., Copes, N., O'Neal-Moffitt, G., Jin, J., Buzzeo, R., Mamcarz, M., et al. (2011). Melatonin treatment restores mitochondrial function in Alzheimer's mice: a mitochondrial protective role of melatonin membrane receptor signaling. J. Pineal Res. 51 (1), 75–86. doi:10.1111/j.1600-079X.2011.00864.x
Duan, Q., Wang, Z., Lu, T., Chen, J., and Wang, X. (2006). Comparison of 6-hydroxylmelatonin or melatonin in protecting neurons against ischemia/reperfusion-mediated injury. J. Pineal Res. 41 (4), 351–357. doi:10.1111/j.1600-079X.2006.00374.x
Duehrkop, C., and Rieben, R. (2014). Ischemia/reperfusion injury: effect of simultaneous inhibition of plasma cascade systems versus specific complement inhibition. Biochem. Pharmacol. 88 (1), 12–22. doi:10.1016/j.bcp.2013.12.013
Ekmekcioglu, C. (2006). Melatonin receptors in humans: biological role and clinical relevance. Biomed. Pharmacother. 60 (3), 97–108. doi:10.1016/j.biopha.2006.01.002
Feng, D., Wang, B., Wang, L., Abraham, N., Tao, K., Huang, L., et al. (2017). Pre-ischemia melatonin treatment alleviated acute neuronal injury after ischemic stroke by inhibiting endoplasmic reticulum stress-dependent autophagy via PERK and IRE1 signalings. J. Pineal Res. 62 (3). doi:10.1111/jpi.12395
Furuta, T., Nakagawa, I., Yokoyama, S., Morisaki, Y., Saito, Y., and Nakase, H. (2022). Melatonin-induced postconditioning suppresses NMDA receptor through opening of the mitochondrial permeability transition pore via melatonin receptor in mouse neurons. Int. J. Mol. Sci. 23 (7), 3822. doi:10.3390/ijms23073822
Gasparova, Z., Stolc, S., and Snirc, V. (2006). In vitro physiological evidence of enhanced antioxidant and neuroprotective action of 2,3-dihydromelatonin, a melatonin analogue. Pharmacol. Res. 53 (1), 22–27. doi:10.1016/j.phrs.2005.08.004
Girouard, H., Chulak, C., Lejossec, M., Lamontagne, D., and de Champlain, J. (2001). Vasorelaxant effects of the chronic treatment with melatonin on mesenteric artery and aorta of spontaneously hypertensive rats. J. Hypertens. 19 (8), 1369–1377. doi:10.1097/00004872-200108000-00004
Glick, D., Barth, S., and Macleod, K. F. (2010). Autophagy: cellular and molecular mechanisms. J. Pathol. 221 (1), 3–12. doi:10.1002/path.2697
Granger, D. N., and Kvietys, P. R. (2015). Reperfusion injury and reactive oxygen species: the evolution of a concept. Redox Biol. 6, 524–551. doi:10.1016/j.redox.2015.08.020
Guo, Y., Wang, J., Wang, Z., Yang, Y., Wang, X., and Duan, Q. (2010). Melatonin protects N2a against ischemia/reperfusion injury through autophagy enhancement. J. Huazhong Univ. Sci. Technol. Med. Sci. 30 (1), 1–7. doi:10.1007/s11596-010-0101-9
Gupta, S., Kaul, C. L., and Sharma, S. S. (2004). Neuroprotective effect of combination of poly (ADP-ribose) polymerase inhibitor and antioxidant in middle cerebral artery occlusion induced focal ischemia in rats. Neurol. Res. 26 (1), 103–107. doi:10.1179/016164104773026624
Gupta, Y. K., Chaudhary, G., and Sinha, K. (2002). Enhanced protection by melatonin and meloxicam combination in a middle cerebral artery occlusion model of acute ischemic stroke in rat. Can. J. Physiol. Pharmacol. 80 (3), 210–217. doi:10.1139/y02-052
Ham, P. B., and Raju, R. (2017). Mitochondrial function in hypoxic ischemic injury and influence of aging. Prog. Neurobiol. 157, 92–116. doi:10.1016/j.pneurobio.2016.06.006
Han, Y. X., Zhang, S. H., Wang, X. M., and Wu, J. B. (2006). Inhibition of mitochondria responsible for the anti-apoptotic effects of melatonin during ischemia-reperfusion. J. Zhejiang Univ. Sci. B 7 (2), 142–147. doi:10.1631/jzus.2006.B0142
Hao, S. M., Zhong, Z. G., Qu, W. M., Huang, Z. L., Sun, F. Y., and Qiu, M. H. (2021). Melatonin supplementation in the subacute phase after ischemia alleviates postischemic sleep disturbances in rats. Brain Behav. 11 (10), e2366. doi:10.1002/brb3.2366
Harvey, C. J., Thimmulappa, R. K., Singh, A., Blake, D. J., Ling, G., Wakabayashi, N., et al. (2009). Nrf2-regulated glutathione recycling independent of biosynthesis is critical for cell survival during oxidative stress. Free Radic. Biol. Med. 46 (4), 443–453. doi:10.1016/j.freeradbiomed.2008.10.040
Hassell, K. J., Ezzati, M., Alonso-Alconada, D., Hausenloy, D. J., and Robertson, N. J. (2015). New horizons for newborn brain protection: enhancing endogenous neuroprotection. Arch. Dis. Child. Fetal Neonatal 100 (6), F541–F552. doi:10.1136/archdischild-2014-306284
He, J., Liu, X., Su, C., Wu, F., Sun, J., Zhang, J., et al. (2019). Inhibition of mitochondrial oxidative damage improves reendothelialization capacity of endothelial progenitor cells via SIRT3 (sirtuin 3)-enhanced SOD2 (superoxide dismutase 2) deacetylation in hypertension. Arterioscler. Thromb. Vasc. Biol. 39 (8), 1682–1698. doi:10.1161/ATVBAHA.119.312613
Huang, C. C., Lai, C. J., Tsai, M. H., Wu, Y. C., Chen, K. T., Jou, M. J., et al. (2015). Effects of melatonin on the nitric oxide system and protein nitration in the hypobaric hypoxic rat hippocampus. BMC Neurosci. 16, 61. doi:10.1186/s12868-015-0199-6
Hung, Y. C., Chen, T. Y., Lee, E. J., Chen, W. L., Huang, S. Y., Lee, W. T., et al. (2008). Melatonin decreases matrix metalloproteinase-9 activation and expression and attenuates reperfusion-induced hemorrhage following transient focal cerebral ischemia in rats. J. Pineal Res. 45 (4), 459–467. doi:10.1111/j.1600-079X.2008.00617.x
Itakura, E., and Mizushima, N. (2010). Characterization of autophagosome formation site by a hierarchical analysis of mammalian Atg proteins. Autophagy 6 (6), 764–776. doi:10.4161/auto.6.6.12709
Jang, J. W., Lee, J. K., Lee, M. C., Piao, M. S., Kim, S. H., and Kim, H. S. (2012). Melatonin reduced the elevated matrix metalloproteinase-9 level in a rat photothrombotic stroke model. J. Neurol. Sci. 323 (1-2), 221–227. doi:10.1016/j.jns.2012.09.021
Ji, J., Xiang, P., Li, T., Lan, L., Xu, X., Lu, G., et al. (2017). NOSH-NBP, a novel nitric oxide and hydrogen sulfide- releasing hybrid, attenuates ischemic stroke-induced neuroinflammatory injury by modulating microglia polarization. Front. Cell. Neurosci. 11, 154. doi:10.3389/fncel.2017.00154
Jia, M., Njapo, S. A., Rastogi, V., and Hedna, V. S. (2015). Taming glutamate excitotoxicity: strategic pathway modulation for neuroprotection. CNS Drugs 29 (2), 153–162. doi:10.1007/s40263-015-0225-3
Jin, R., Yang, G., and Li, G. (2010). Inflammatory mechanisms in ischemic stroke: role of inflammatory cells. J. Leukoc. Biol. 87 (5), 779–789. doi:10.1189/jlb.1109766
Juan, W. S., Huang, S. Y., Chang, C. C., Hung, Y. C., Lin, Y. W., Chen, T. Y., et al. (2014). Melatonin improves neuroplasticity by upregulating the growth-associated protein-43 (GAP-43) and NMDAR postsynaptic density-95 (PSD-95) proteins in cultured neurons exposed to glutamate excitotoxicity and in rats subjected to transient focal cerebral ischemia even during a long-term recovery period. J. Pineal Res. 56 (2), 213–223. doi:10.1111/jpi.12114
Kang, J. W., Koh, E. J., and Lee, S. M. (2011). Melatonin protects liver against ischemia and reperfusion injury through inhibition of toll-like receptor signaling pathway. J. Pineal Res. 50 (4), 403–411. doi:10.1111/j.1600-079X.2011.00858.x
Kilic, E., Kilic, U., Bacigaluppi, M., Guo, Z., Abdallah, N. B., Wolfer, D. P., et al. (2008). Delayed melatonin administration promotes neuronal survival, neurogenesis and motor recovery, and attenuates hyperactivity and anxiety after mild focal cerebral ischemia in mice. J. Pineal Res. 45 (2), 142–148. doi:10.1111/j.1600-079X.2008.00568.x
Kilic, E., Kilic, U., Reiter, R. J., Bassetti, C. L., and Hermann, D. M. (2004a). Prophylactic use of melatonin protects against focal cerebral ischemia in mice: role of endothelin converting enzyme-1. J. Pineal Res. 37 (4), 247–251. doi:10.1111/j.1600-079X.2004.00162.x
Kilic, E., Kilic, U., Reiter, R. J., Bassetti, C. L., and Hermann, D. M. (2005a). Tissue-plasminogen activator-induced ischemic brain injury is reversed by melatonin: role of iNOS and Akt. J. Pineal Res. 39 (2), 151–155. doi:10.1111/j.1600-079X.2005.00228.x
Kilic, E., Kilic, U., Yulug, B., Hermann, D. M., and Reiter, R. J. (2004b). Melatonin reduces disseminate neuronal death after mild focal ischemia in mice via inhibition of caspase-3 and is suitable as an add-on treatment to tissue-plasminogen activator. J. Pineal Res. 36 (3), 171–176. doi:10.1046/j.1600-079x.2003.00115.x
Kilic, E., Ozdemir, Y. G., Bolay, H., Kelestimur, H., and Dalkara, T. (1999). Pinealectomy aggravates and melatonin administration attenuates brain damage in focal ischemia. J. Cereb. Blood Flow. Metab. 19 (5), 511–516. doi:10.1097/00004647-199905000-00005
Kilic, U., Caglayan, A. B., Beker, M. C., Gunal, M. Y., Caglayan, B., Yalcin, E., et al. (2017). Particular phosphorylation of PI3K/Akt on Thr308 via PDK-1 and PTEN mediates melatonin's neuroprotective activity after focal cerebral ischemia in mice. Redox Biol. 12, 657–665. doi:10.1016/j.redox.2017.04.006
Kilic, U., Elibol, B., Beker, M., Altug-Tasa, B., Caglayan, A. B., Beker, M. C., et al. (2021). Inflammatory cytokines are in action: brain plasticity and recovery after brain ischemia due to delayed melatonin administration. J. Stroke Cerebrovasc. Dis. 30 (12), 106105. doi:10.1016/j.jstrokecerebrovasdis.2021.106105
Kilic, U., Elibol, B., Caglayan, A. B., Beker, M. C., Beker, M., Altug-Tasa, B., et al. (2022). Delayed therapeutic administration of melatonin enhances neuronal survival through AKT and MAPK signaling pathways following focal brain ischemia in mice. J. Mol. Neurosci. 72 (5), 994–1007. doi:10.1007/s12031-022-01995-y
Kilic, U., Kilic, E., Reiter, R. J., Bassetti, C. L., and Hermann, D. M. (2005b). Signal transduction pathways involved in melatonin-induced neuroprotection after focal cerebral ischemia in mice. J. Pineal Res. 38 (1), 67–71. doi:10.1111/j.1600-079X.2004.00178.x
Kilic, U., Yilmaz, B., Reiter, R. J., Yuksel, A., and Kilic, E. (2013). Effects of memantine and melatonin on signal transduction pathways vascular leakage and brain injury after focal cerebral ischemia in mice. Neuroscience 237, 268–276. doi:10.1016/j.neuroscience.2013.01.059
Kilic, U., Yilmaz, B., Ugur, M., Yuksel, A., Reiter, R. J., Hermann, D. M., et al. (2012). Evidence that membrane-bound G protein-coupled melatonin receptors MT1 and MT2 are not involved in the neuroprotective effects of melatonin in focal cerebral ischemia. J. Pineal Res. 52 (2), 228–235. doi:10.1111/j.1600-079X.2011.00932.x
Kim, Y. M., Pae, H. O., Park, J. E., Lee, Y. C., Woo, J. M., Kim, N. H., et al. (2011). Heme oxygenase in the regulation of vascular biology: from molecular mechanisms to therapeutic opportunities. Antioxid. Redox Signal 14 (1), 137–167. doi:10.1089/ars.2010.3153
Kitkhuandee, A., Sawanyawisuth, K., Johns, N. P., Kanpittaya, J., and Johns, J. (2014). Pineal calcification is associated with symptomatic cerebral infarction. J. Stroke Cerebrovasc. Dis. 23 (2), 249–253. doi:10.1016/j.jstrokecerebrovasdis.2013.01.009
Koh, P. O. (2008a). Melatonin attenuates the cerebral ischemic injury via the MEK/ERK/p90RSK/bad signaling cascade. J. Vet. Med. Sci. 70 (11), 1219–1223. doi:10.1292/jvms.70.1219
Koh, P. O. (2008b). Melatonin attenuates the focal cerebral ischemic injury by inhibiting the dissociation of pBad from 14-3-3. J. Pineal Res. 44 (1), 101–106. doi:10.1111/j.1600-079X.2007.00495.x
Koh, P. O. (2008c). Melatonin prevents ischemic brain injury through activation of the mTOR/p70S6 kinase signaling pathway. Neurosci. Lett. 444 (1), 74–78. doi:10.1016/j.neulet.2008.08.024
Koh, P. O. (2008d). Melatonin prevents the injury-induced decline of Akt/forkhead transcription factors phosphorylation. J. Pineal Res. 45 (2), 199–203. doi:10.1111/j.1600-079X.2008.00577.x
Koh, P. O. (2008e). Melatonin regulates nitric oxide synthase expression in ischemic brain injury. J. Vet. Med. Sci. 70 (7), 747–750. doi:10.1292/jvms.70.747
Koh, P. O. (2011). Melatonin prevents down-regulation of astrocytic phosphoprotein PEA-15 in ischemic brain injury. J. Pineal Res. 51 (4), 381–386. doi:10.1111/j.1600-079X.2011.00900.x
Koh, P. O. (2012a). Melatonin attenuates decrease of protein phosphatase 2A subunit B in ischemic brain injury. J. Pineal Res. 52 (1), 57–61. doi:10.1111/j.1600-079X.2011.00918.x
Koh, P. O. (2012b). Melatonin regulates the calcium-buffering proteins, parvalbumin and hippocalcin, in ischemic brain injury. J. Pineal Res. 53 (4), 358–365. doi:10.1111/j.1600-079X.2012.01005.x
Kondoh, T., Uneyama, H., Nishino, H., and Torii, K. (2002). Melatonin reduces cerebral edema formation caused by transient forebrain ischemia in rats. Life Sci. 72 (4-5), 583–590. doi:10.1016/s0024-3205(02)02256-7
Kong, X., Wang, R., Xue, Y., Liu, X., Zhang, H., Chen, Y., et al. (2010). Sirtuin 3, a new target of PGC-1alpha, plays an important role in the suppression of ROS and mitochondrial biogenesis. PLoS One 5 (7), e11707. doi:10.1371/journal.pone.0011707
Kurisu, K., and Yenari, M. A. (2018). Therapeutic hypothermia for ischemic stroke; pathophysiology and future promise. Neuropharmacology 134 (Pt B), 302–309. doi:10.1016/j.neuropharm.2017.08.025
Lee, E. J., Lee, M. Y., Chen, H. Y., Hsu, Y. S., Wu, T. S., Chen, S. T., et al. (2005). Melatonin attenuates gray and white matter damage in a mouse model of transient focal cerebral ischemia. J. Pineal Res. 38 (1), 42–52. doi:10.1111/j.1600-079X.2004.00173.x
Lee, E. J., Wu, T. S., Lee, M. Y., Chen, T. Y., Tsai, Y. Y., Chuang, J. I., et al. (2004). Delayed treatment with melatonin enhances electrophysiological recovery following transient focal cerebral ischemia in rats. J. Pineal Res. 36 (1), 33–42. doi:10.1046/j.1600-079x.2003.00093.x
Lee, M. Y., Kuan, Y. H., Chen, H. Y., Chen, T. Y., Chen, S. T., Huang, C. C., et al. (2007). Intravenous administration of melatonin reduces the intracerebral cellular inflammatory response following transient focal cerebral ischemia in rats. J. Pineal Res. 42 (3), 297–309. doi:10.1111/j.1600-079X.2007.00420.x
Lemmerman, L. R., Harris, H. N., Balch, M. H. H., Rincon-Benavides, M. A., Higuita-Castro, N., Arnold, D. W., et al. (2022). Transient middle cerebral artery occlusion with an intraluminal suture enables reproducible induction of ischemic stroke in mice. Bio Protoc. 12 (3), e4305. doi:10.21769/BioProtoc.4305
Lesnefsky, E. J., Chen, Q., Tandler, B., and Hoppel, C. L. (2017). Mitochondrial dysfunction and myocardial ischemia-reperfusion: implications for novel therapies. Annu. Rev. Pharmacol. Toxicol. 57, 535–565. doi:10.1146/annurev-pharmtox-010715-103335
Li, D., He, T., Zhang, Y., Liu, J., Zhao, H., Wang, D., et al. (2023). Melatonin regulates microglial polarization and protects against ischemic stroke-induced brain injury in mice. Exp. Neurol. 367, 114464. doi:10.1016/j.expneurol.2023.114464
Li, H., Wang, Y., Feng, D., Liu, Y., Xu, M., Gao, A., et al. (2014). Alterations in the time course of expression of the Nox family in the brain in a rat experimental cerebral ischemia and reperfusion model: effects of melatonin. J. Pineal Res. 57 (1), 110–119. doi:10.1111/jpi.12148
Li, X. J., Zhang, L. M., Gu, J., Zhang, A. Z., and Sun, F. Y. (1997). Melatonin decreases production of hydroxyl radical during cerebral ischemia-reperfusion. Zhongguo Yao Li Xue Bao 18 (5), 394–396.
Liddelow, S. A., Guttenplan, K. A., Clarke, L. E., Bennett, F. C., Bohlen, C. J., Schirmer, L., et al. (2017). Neurotoxic reactive astrocytes are induced by activated microglia. Nature 541 (7638), 481–487. doi:10.1038/nature21029
Lin, K. C., Chen, K. H., Wallace, C. G., Chen, Y. L., Ko, S. F., Lee, M. S., et al. (2019). Combined therapy with hyperbaric oxygen and melatonin effectively reduce brain infarct volume and preserve neurological function after acute ischemic infarct in rat. J. Neuropathol. Exp. Neurol. 78 (10), 949–960. doi:10.1093/jnen/nlz076
Lin, Y. W., Chen, T. Y., Hung, C. Y., Tai, S. H., Huang, S. Y., Chang, C. C., et al. (2018). Melatonin protects brain against ischemia/reperfusion injury by attenuating endoplasmic reticulum stress. Int. J. Mol. Med. 42 (1), 182–192. doi:10.3892/ijmm.2018.3607
Ling, L., Alattar, A., Tan, Z., Shah, F. A., Ali, T., Alshaman, R., et al. (2020). A potent antioxidant endogenous neurohormone melatonin, rescued MCAO by attenuating oxidative stress-associated neuroinflammation. Front. Pharmacol. 11, 1220. doi:10.3389/fphar.2020.01220
Ling, X., Zhang, L. M., Lu, S. D., Li, X. J., and Sun, F. Y. (1999). Protective effect of melatonin on injuried cerebral neurons is associated with bcl-2 protein over-expression. Zhongguo Yao Li Xue Bao 20 (5), 409–414.
Liu, D., Wei, N., Man, H. Y., Lu, Y., Zhu, L. Q., and Wang, J. Z. (2015). The MT2 receptor stimulates axonogenesis and enhances synaptic transmission by activating Akt signaling. Cell. Death Differ. 22 (4), 583–596. doi:10.1038/cdd.2014.195
Liu, L., Cao, Q., Gao, W., Li, B., Xia, Z., and Zhao, B. (2021). Melatonin protects against focal cerebral ischemia-reperfusion injury in diabetic mice by ameliorating mitochondrial impairments: involvement of the Akt-SIRT3-SOD2 signaling pathway. Aging (Albany NY) 13 (12), 16105–16123. doi:10.18632/aging.203137
Liu, L., Cao, Q., Gao, W., Li, B. Y., Zeng, C., Xia, Z., et al. (2021). Melatonin ameliorates cerebral ischemia-reperfusion injury in diabetic mice by enhancing autophagy via the SIRT1-BMAL1 pathway. FASEB J. 35 (12), e22040. doi:10.1096/fj.202002718RR
Liu, L., Chen, H., Jin, J., Tang, Z., Yin, P., Zhong, D., et al. (2019). Melatonin ameliorates cerebral ischemia/reperfusion injury through SIRT3 activation. Life Sci. 239, 117036. doi:10.1016/j.lfs.2019.117036
Liu, L., and Cheung, R. T. (2013). Effects of pretreatment with a combination of melatonin and electroacupuncture in a rat model of transient focal cerebral ischemia. Evid. Based Complement. Altern. Med. 2013, 953162. doi:10.1155/2013/953162
Liu, L., Locascio, L. M., and Dore, S. (2019). Critical role of Nrf2 in experimental ischemic stroke. Front. Pharmacol. 10, 153. doi:10.3389/fphar.2019.00153
Liu, Z., and Chopp, M. (2016). Astrocytes, therapeutic targets for neuroprotection and neurorestoration in ischemic stroke. Prog. Neurobiol. 144, 103–120. doi:10.1016/j.pneurobio.2015.09.008
Liu, Z. J., Ran, Y. Y., Qie, S. Y., Gong, W. J., Gao, F. H., Ding, Z. T., et al. (2019). Melatonin protects against ischemic stroke by modulating microglia/macrophage polarization toward anti-inflammatory phenotype through STAT3 pathway. CNS Neurosci. Ther. 25 (12), 1353–1362. doi:10.1111/cns.13261
Lorente, L., Martín, M. M., Abreu-González, P., Pérez-Cejas, A., Ramos, L., Argueso, M., et al. (2018). Serum melatonin levels are associated with mortality in patients with malignant middle cerebral artery infarction. J. Int. Med. Res. 46 (8), 3268–3277. doi:10.1177/0300060518775008
Luchetti, F., Nasoni, M. G., Burattini, S., Mohammadi, A., Pagliarini, M., Canonico, B., et al. (2022). Melatonin attenuates ischemic-like cell injury by promoting autophagosome maturation via the sirt1/FoxO1/rab7 Axis in hippocampal HT22 cells and in organotypic cultures. Cells 11 (22), 3701. doi:10.3390/cells11223701
Ma, Q. (2013). Role of nrf2 in oxidative stress and toxicity. Annu. Rev. Pharmacol. Toxicol. 53, 401–426. doi:10.1146/annurev-pharmtox-011112-140320
Ma, Y., Feng, Q., Ma, J., Feng, Z., Zhan, M., Ouyang, L., et al. (2013). Melatonin ameliorates injury and specific responses of ischemic striatal neurons in rats. J. Histochem Cytochem 61 (8), 591–605. doi:10.1369/0022155413492159
Madesh, M., Antonsson, B., Srinivasula, S. M., Alnemri, E. S., and Hajnoczky, G. (2002). Rapid kinetics of tBid-induced cytochrome c and Smac/DIABLO release and mitochondrial depolarization. J. Biol. Chem. 277 (7), 5651–5659. doi:10.1074/jbc.M108171200
Maixner, D. W., and Weng, H. R. (2013). The role of glycogen synthase kinase 3 beta in neuroinflammation and pain. J. Pharm. Pharmacol. (Los Angel) 1 (1), 001. doi:10.13188/2327-204X.1000001
Manzanero, S., Santro, T., and Arumugam, T. V. (2013). Neuronal oxidative stress in acute ischemic stroke: sources and contribution to cell injury. Neurochem. Int. 62 (5), 712–718. doi:10.1016/j.neuint.2012.11.009
Martin, M., Rehani, K., Jope, R. S., and Michalek, S. M. (2005). Toll-like receptor-mediated cytokine production is differentially regulated by glycogen synthase kinase 3. Nat. Immunol. 6 (8), 777–784. doi:10.1038/ni1221
Martina, J. A., Chen, Y., Gucek, M., and Puertollano, R. (2012). MTORC1 functions as a transcriptional regulator of autophagy by preventing nuclear transport of TFEB. Autophagy 8 (6), 903–914. doi:10.4161/auto.19653
Matejovska, I., Bernaskova, K., Krysl, D., and Mares, J. (2008). Influence of melatonin pretreatment and preconditioning by hypobaric hypoxia on the development of cortical photothrombotic ischemic lesion. Physiol. Res. 57 (2), 283–288. doi:10.33549/physiolres.931143
Mehrpooya, M., Mazdeh, M., Rahmani, E., Khazaie, M., and Ahmadimoghaddam, D. (2022). Melatonin supplementation may benefit patients with acute ischemic stroke not eligible for reperfusion therapies: results of a pilot study. J. Clin. Neurosci. 106, 66–75. doi:10.1016/j.jocn.2022.10.006
Mengel, A., Zurloh, J., Boßelmann, C., Brendel, B., Stadler, V., Sartor-Pfeiffer, J., et al. (2021). Delirium REduction after administration of melatonin in acute ischemic stroke (DREAMS): a propensity score-matched analysis. Eur. J. Neurol. 28 (6), 1958–1966. doi:10.1111/ene.14792
Murphy, E., and Steenbergen, C. (2008). Mechanisms underlying acute protection from cardiac ischemia-reperfusion injury. Physiol. Rev. 88 (2), 581–609. doi:10.1152/physrev.00024.2007
Nair, S. M., Rahman, R. M., Clarkson, A. N., Sutherland, B. A., Taurin, S., Sammut, I. A., et al. (2011). Melatonin treatment following stroke induction modulates L-arginine metabolism. J. Pineal Res. 51 (3), 313–323. doi:10.1111/j.1600-079X.2011.00891.x
Nasoni, M. G., Carloni, S., Canonico, B., Burattini, S., Cesarini, E., Papa, S., et al. (2021). Melatonin reshapes the mitochondrial network and promotes intercellular mitochondrial transfer via tunneling nanotubes after ischemic-like injury in hippocampal HT22 cells. J. Pineal Res. 71 (1), e12747. doi:10.1111/jpi.12747
Newmeyer, D. D., and Ferguson-Miller, S. (2003). Mitochondria: releasing power for life and unleashing the machineries of death. Cell. 112 (4), 481–490. doi:10.1016/s0092-8674(03)00116-8
Ng, K. Y., Leong, M. K., Liang, H., and Paxinos, G. (2017). Melatonin receptors: distribution in mammalian brain and their respective putative functions. Brain Struct. Funct. 222 (7), 2921–2939. doi:10.1007/s00429-017-1439-6
Ott, M., Robertson, J. D., Gogvadze, V., Zhivotovsky, B., and Orrenius, S. (2002). Cytochrome c release from mitochondria proceeds by a two-step process. Proc. Natl. Acad. Sci. U. S. A. 99 (3), 1259–1263. doi:10.1073/pnas.241655498
Parada, E., Buendia, I., Leon, R., Negredo, P., Romero, A., Cuadrado, A., et al. (2014). Neuroprotective effect of melatonin against ischemia is partially mediated by alpha-7 nicotinic receptor modulation and HO-1 overexpression. J. Pineal Res. 56 (2), 204–212. doi:10.1111/jpi.12113
Paredes, S. D., Rancan, L., Kireev, R., Gonzalez, A., Louzao, P., Gonzalez, P., et al. (2015). Melatonin counteracts at a transcriptional level the inflammatory and apoptotic response secondary to ischemic brain injury induced by middle cerebral artery blockade in aging rats. Biores Open Access 4 (1), 407–416. doi:10.1089/biores.2015.0032
Parzych, K. R., and Klionsky, D. J. (2014). An overview of autophagy: morphology, mechanism, and regulation. Antioxid. Redox Signal 20 (3), 460–473. doi:10.1089/ars.2013.5371
Paterniti, I., Cordaro, M., Esposito, E., and Cuzzocrea, S. (2016). The antioxidative property of melatonin against brain ischemia. Expert Rev. Neurother. 16 (7), 841–848. doi:10.1080/14737175.2016.1182020
Patino, P., Parada, E., Farre-Alins, V., Molz, S., Cacabelos, R., Marco-Contelles, J., et al. (2016). Melatonin protects against oxygen and glucose deprivation by decreasing extracellular glutamate and Nox-derived ROS in rat hippocampal slices. Neurotoxicology 57, 61–68. doi:10.1016/j.neuro.2016.09.002
Pei, Z., and Cheung, R. T. (2003). Melatonin protects SHSY5Y neuronal cells but not cultured astrocytes from ischemia due to oxygen and glucose deprivation. J. Pineal Res. 34 (3), 194–201. doi:10.1034/j.1600-079x.2003.00026.x
Pei, Z., and Cheung, R. T. (2004). Pretreatment with melatonin exerts anti-inflammatory effects against ischemia/reperfusion injury in a rat middle cerebral artery occlusion stroke model. J. Pineal Res. 37 (2), 85–91. doi:10.1111/j.1600-079X.2004.00138.x
Pei, Z., Fung, P. C., and Cheung, R. T. (2003a). Melatonin reduces nitric oxide level during ischemia but not blood-brain barrier breakdown during reperfusion in a rat middle cerebral artery occlusion stroke model. J. Pineal Res. 34 (2), 110–118. doi:10.1034/j.1600-079x.2003.00014.x
Pei, Z., Ho, H. T., and Cheung, R. T. (2002). Pre-treatment with melatonin reduces volume of cerebral infarction in a permanent middle cerebral artery occlusion stroke model in the rat. Neurosci. Lett. 318 (3), 141–144. doi:10.1016/s0304-3940(01)02503-4
Pei, Z., Pang, S. F., and Cheung, R. T. (2002). Pretreatment with melatonin reduces volume of cerebral infarction in a rat middle cerebral artery occlusion stroke model. J. Pineal Res. 32 (3), 168–172. doi:10.1034/j.1600-079x.2002.1o847.x
Pei, Z., Pang, S. F., and Cheung, R. T. (2003b). Administration of melatonin after onset of ischemia reduces the volume of cerebral infarction in a rat middle cerebral artery occlusion stroke model. Stroke 34 (3), 770–775. doi:10.1161/01.STR.0000057460.14810.3E
Perlman, J. M., Wyllie, J., Kattwinkel, J., Atkins, D. L., Chameides, L., Goldsmith, J. P., et al. (2010). Part 11: neonatal resuscitation: 2010 international consensus on cardiopulmonary resuscitation and emergency cardiovascular care science with treatment recommendations. Circulation 122 (16 Suppl. 2), S516–S538. doi:10.1161/CIRCULATIONAHA.110.971127
Powers, W. J., Rabinstein, A. A., Ackerson, T., Adeoye, O. M., Bambakidis, N. C., Becker, K., et al. (2019). Guidelines for the early management of patients with acute ischemic stroke: 2019 update to the 2018 guidelines for the early management of acute ischemic stroke: a guideline for healthcare professionals from the American heart association/American stroke association. Stroke 50 (12), e344–e418. doi:10.1161/str.0000000000000211
Rabanal-Ruiz, Y., Otten, E. G., and Korolchuk, V. I. (2017). mTORC1 as the main gateway to autophagy. Essays Biochem. 61 (6), 565–584. doi:10.1042/EBC20170027
Ramos, E., Farré-Alins, V., Javier Egea, , López-Muñoz, F., Reiter, R. J., and Romero, A. (2020). Melatonin's efficacy in stroke patients; a matter of dose? A systematic review. Toxicol. Appl. Pharmacol. 392, 114933. doi:10.1016/j.taap.2020.114933
Ran, Y., Ye, L., Ding, Z., Gao, F., Yang, S., Fang, B., et al. (2021). Melatonin protects against ischemic brain injury by modulating PI3K/AKT signaling pathway via suppression of PTEN activity. ASN Neuro 13, 17590914211022888. doi:10.1177/17590914211022888
Rancan, L., Paredes, S. D., Garcia, C., Gonzalez, P., Rodriguez-Bobada, C., Calvo-Soto, M., et al. (2018). Comparison of the effect of melatonin treatment before and after brain ischemic injury in the inflammatory and apoptotic response in aged rats. Int. J. Mol. Sci. 19 (7), 2097. doi:10.3390/ijms19072097
Regenhardt, R. W., Das, A. S., Lo, E. H., and Caplan, L. R. (2018). Advances in understanding the pathophysiology of lacunar stroke: a review. JAMA Neurol. 75 (10), 1273–1281. doi:10.1001/jamaneurol.2018.1073
Riha, R. L. (2018). The use and misuse of exogenous melatonin in the treatment of sleep disorders. Curr. Opin. Pulm. Med. 24 (6), 543–548. doi:10.1097/MCP.0000000000000522
Ritzenthaler, T., Lhommeau, I., Douillard, S., Cho, T. H., Brun, J., Patrice, T., et al. (2013). Dynamics of oxidative stress and urinary excretion of melatonin and its metabolites during acute ischemic stroke. Neurosci. Lett. 544, 1–4. doi:10.1016/j.neulet.2013.02.073
Rodriguez, C., Mayo, J. C., Sainz, R. M., Antolin, I., Herrera, F., Martin, V., et al. (2004). Regulation of antioxidant enzymes: a significant role for melatonin. J. Pineal Res. 36 (1), 1–9. doi:10.1046/j.1600-079x.2003.00092.x
Romero, A., Egea, J., Garcia, A. G., and Lopez, M. G. (2010). Synergistic neuroprotective effect of combined low concentrations of galantamine and melatonin against oxidative stress in SH-SY5Y neuroblastoma cells. J. Pineal Res. 49 (2), 141–148. doi:10.1111/j.1600-079X.2010.00778.x
Romero, A., Ramos, E., Patiño, P., Oset-Gasque, M. J., López-Muñoz, F., Marco-Contelles, J., et al. (2016). Melatonin and nitrones as potential therapeutic agents for stroke. Front. Aging Neurosci. 8, 281. doi:10.3389/fnagi.2016.00281
Ryoo, I. G., and Kwak, M. K. (2018). Regulatory crosstalk between the oxidative stress-related transcription factor Nfe2l2/Nrf2 and mitochondria. Toxicol. Appl. Pharmacol. 359, 24–33. doi:10.1016/j.taap.2018.09.014
Saleh, D. O., Jaleel, G. A. A., Al-Awdan, S. W., Hassan, A., and Asaad, G. F. (2020). Melatonin suppresses the brain injury after cerebral ischemia/reperfusion in hyperglycaemic rats. Res. Pharm. Sci. 15 (5), 418–428. doi:10.4103/1735-5362.297844
Samantaray, S., Das, A., Thakore, N. P., Matzelle, D. D., Reiter, R. J., Ray, S. K., et al. (2009). Therapeutic potential of melatonin in traumatic central nervous system injury. J. Pineal Res. 47 (2), 134–142. doi:10.1111/j.1600-079X.2009.00703.x
Samantaray, S., Sribnick, E. A., Das, A., Knaryan, V. H., Matzelle, D. D., Yallapragada, A. V., et al. (2008). Melatonin attenuates calpain upregulation, axonal damage and neuronal death in spinal cord injury in rats. J. Pineal Res. 44 (4), 348–357. doi:10.1111/j.1600-079X.2007.00534.x
Sarmah, D., Kaur, H., Saraf, J., Vats, K., Pravalika, K., Wanve, M., et al. (2018). Mitochondrial dysfunction in stroke: implications of stem cell therapy. Transl. Stroke Res. 10, 121–136. doi:10.1007/s12975-018-0642-y
Satterstrom, F. K., Swindell, W. R., Laurent, G., Vyas, S., Bulyk, M. L., and Haigis, M. C. (2015). Nuclear respiratory factor 2 induces SIRT3 expression. Aging Cell. 14 (5), 818–825. doi:10.1111/acel.12360
Sattler, R., and Tymianski, M. (2000). Molecular mechanisms of calcium-dependent excitotoxicity. J. Mol. Med. Berl. 78 (1), 3–13. doi:10.1007/s001090000077
Schmid, H. A., Requintina, P. J., Oxenkrug, G. F., and Sturner, W. (1994). Calcium, calcification, and melatonin biosynthesis in the human pineal gland: a postmortem study into age-related factors. J. Pineal Res. 16 (4), 178–183. doi:10.1111/j.1600-079x.1994.tb00098.x
Settembre, C., Di Malta, C., Polito, V. A., Garcia Arencibia, M., Vetrini, F., Erdin, S., et al. (2011). TFEB links autophagy to lysosomal biogenesis. Science 332 (6036), 1429–1433. doi:10.1126/science.1204592
Shah, F. A., Liu, G., Al Kury, L. T., Zeb, A., Abbas, M., Li, T., et al. (2019). Melatonin protects MCAO-induced neuronal loss via NR2A mediated prosurvival pathways. Front. Pharmacol. 10, 297. doi:10.3389/fphar.2019.00297
Shah, F. A., Zeb, A., Ali, T., Muhammad, T., Faheem, M., Alam, S. I., et al. (2018). Identification of proteins differentially expressed in the striatum by melatonin in a middle cerebral artery occlusion rat model-a proteomic and in silico approach. Front. Neurosci. 12, 888. doi:10.3389/fnins.2018.00888
Shao, A., Gao, S., Wu, H., Xu, W., Pan, Y., Fang, Y., et al. (2021). Melatonin ameliorates hemorrhagic transformation via suppression of ROS-induced NLRP3 activation after cerebral ischemia in hyperglycemic rats. Oxid. Med. Cell. Longev. 2021, 6659282. doi:10.1155/2021/6659282
Sharma, S., Singh, H., Ahmad, N., Mishra, P., and Tiwari, A. (2015). The role of melatonin in diabetes: therapeutic implications. Arch. Endocrinol. Metab. 59 (5), 391–399. doi:10.1590/2359-3997000000098
Shaw, R. C., Walker, G., Elliott, E., and Quinn, T. J. (2019). Occurrence rate of delirium in acute stroke settings: systematic review and meta-analysis. Stroke 50 (11), 3028–3036. doi:10.1161/STROKEAHA.119.025015
Siddiqi, N., House, A. O., and Holmes, J. D. (2006). Occurrence and outcome of delirium in medical in-patients: a systematic literature review. Age Ageing 35 (4), 350–364. doi:10.1093/ageing/afl005
Sims, N. R., and Anderson, M. F. (2002). Mitochondrial contributions to tissue damage in stroke. Neurochem. Int. 40 (6), 511–526. doi:10.1016/s0197-0186(01)00122-x
Sinha, K., Degaonkar, M. N., Jagannathan, N. R., and Gupta, Y. K. (2001). Effect of melatonin on ischemia reperfusion injury induced by middle cerebral artery occlusion in rats. Eur. J. Pharmacol. 428 (2), 185–192. doi:10.1016/s0014-2999(01)01253-5
Song, J., Kang, S. M., Lee, W. T., Park, K. A., Lee, K. M., and Lee, J. E. (2014). The beneficial effect of melatonin in brain endothelial cells against oxygen-glucose deprivation followed by reperfusion-induced injury. Oxid. Med. Cell. Longev. 2014, 639531. doi:10.1155/2014/639531
Su, J. H., Wu, G. P., Peng, X., Zhao, G. Q., Peng, Y., Zhang, H. H., et al. (2023). Neuroprotective effects of an N-salicyloyl tryptamine derivative against cerebral ischemia/reperfusion injury. ACS Chem. Neurosci. 14 (11), 2146–2158. doi:10.1021/acschemneuro.3c00149
Sun, F. Y., Lin, X., Mao, L. Z., Ge, W. H., Zhang, L. M., Huang, Y. L., et al. (2002). Neuroprotection by melatonin against ischemic neuronal injury associated with modulation of DNA damage and repair in the rat following a transient cerebral ischemia. J. Pineal Res. 33 (1), 48–56. doi:10.1034/j.1600-079x.2002.01891.x
Sun, J., Chi, L., He, Z., Gao, Y., Gao, Y., Huang, Y., et al. (2019). NLRP3 inflammasome contributes to neurovascular unit damage in stroke. J. Drug Target 27 (8), 866–875. doi:10.1080/1061186X.2018.1564925
Sun, Y., Jin, M. F., Li, L., Liu, Y., Wang, D., and Ni, H. (2022). Genetic inhibition of Plppr5 aggravates hypoxic-ischemie-induced cortical damage and excitotoxic phenotype. Front. Neurosci. 16, 751489. doi:10.3389/fnins.2022.751489
Sung, J. H., Cho, E. H., Kim, M. O., and Koh, P. O. (2009). Identification of proteins differentially expressed by melatonin treatment in cerebral ischemic injury--a proteomics approach. J. Pineal Res. 46 (3), 300–306. doi:10.1111/j.1600-079X.2008.00661.x
Suofu, Y., Jauhari, A., Nirmala, E. S., Mullins, W. A., Wang, X., Li, F., et al. (2023). Neuronal melatonin type 1 receptor overexpression promotes M2 microglia polarization in cerebral ischemia/reperfusion-induced injury. Neurosci. Lett. 795, 137043. doi:10.1016/j.neulet.2022.137043
Suofu, Y., Li, W., Jean-Alphonse, F. G., Jia, J., Khattar, N. K., Li, J., et al. (2017). Dual role of mitochondria in producing melatonin and driving GPCR signaling to block cytochrome c release. Proc. Natl. Acad. Sci. U. S. A. 114 (38), E7997–E8006. doi:10.1073/pnas.1705768114
Tai, S. H., Chen, H. Y., Lee, E. J., Chen, T. Y., Lin, H. W., Hung, Y. C., et al. (2010). Melatonin inhibits postischemic matrix metalloproteinase-9 (MMP-9) activation via dual modulation of plasminogen/plasmin system and endogenous MMP inhibitor in mice subjected to transient focal cerebral ischemia. J. Pineal Res. 49 (4), 332–341. doi:10.1111/j.1600-079X.2010.00797.x
Tai, S. H., Hung, Y. C., Lee, E. J., Lee, A. C., Chen, T. Y., Shen, C. C., et al. (2011). Melatonin protects against transient focal cerebral ischemia in both reproductively active and estrogen-deficient female rats: the impact of circulating estrogen on its hormetic dose-response. J. Pineal Res. 50 (3), 292–303. doi:10.1111/j.1600-079X.2010.00839.x
Tehse, J., and Taghibiglou, C. (2019). The overlooked aspect of excitotoxicity: glutamate-independent excitotoxicity in traumatic brain injuries. Eur. J. Neurosci. 49 (9), 1157–1170. doi:10.1111/ejn.14307
Teichberg, V. I., Cohen-Kashi-Malina, K., Cooper, I., and Zlotnik, A. (2009). Homeostasis of glutamate in brain fluids: an accelerated brain-to-blood efflux of excess glutamate is produced by blood glutamate scavenging and offers protection from neuropathologies. Neuroscience 158 (1), 301–308. doi:10.1016/j.neuroscience.2008.02.075
Torii, K., Uneyama, H., Nishino, H., and Kondoh, T. (2004). Melatonin suppresses cerebral edema caused by middle cerebral artery occlusion/reperfusion in rats assessed by magnetic resonance imaging. J. Pineal Res. 36 (1), 18–24. doi:10.1046/j.1600-079x.2003.00097.x
Uchida, K., Samejima, M., Okabe, A., and Fukuda, A. (2004). Neuroprotective effects of melatonin against anoxia/aglycemia stress, as assessed by synaptic potentials and superoxide production in rat hippocampal slices. J. Pineal Res. 37 (4), 215–222. doi:10.1111/j.1600-079X.2004.00159.x
Venegas, C., García, J. A., Escames, G., Ortiz, F., López, A., Doerrier, C., et al. (2012). Ana lópez, carolina doerrier, laura garcía-corzo, luis C. López, russel J. Reiter, and darío acuña-castroviejo extrapineal melatonin: analysis of its subcellular distribution and daily fluctuations. J. Pineal Res. 52 (2), 217–227. doi:10.1111/j.1600-079X.2011.00931.x
Venkatesan, A., Satin, L. S., and Raghavan, M. (2021). Roles of calreticulin in protein folding, immunity, calcium signaling and cell transformation. Prog. Mol. Subcell. Biol. 59, 145–162. doi:10.1007/978-3-030-67696-4_7
Vlkolinsky, R., and Stolc, S. (1999). Effects of stobadine, melatonin, and other antioxidants on hypoxia/reoxygenation-induced synaptic transmission failure in rat hippocampal slices. Brain Res. 850 (1-2), 118–126. doi:10.1016/s0006-8993(99)02110-1
Vriend, J., and Reiter, R. J. (2014). Melatonin as a proteasome inhibitor. Is there any clinical evidence? Life Sci. 115 (1-2), 8–14. doi:10.1016/j.lfs.2014.08.024
Wang, D. D., Jin, M. F., Zhao, D. J., and Ni, H. (2019a). Reduction of mitophagy-related oxidative stress and preservation of mitochondria function using melatonin therapy in an HT22 hippocampal neuronal cell model of glutamate-induced excitotoxicity. Front. Endocrinol. (Lausanne) 10, 550. doi:10.3389/fendo.2019.00550
Wang, H., Chen, W., Chen, L., Liu, D., and Wang, X. (2019b). Melatonin attenuates white matter damage after focal brain ischemia in rats by regulating the TLR4/NF-κB pathway. Brain Res. Bull. 150, 168–178. doi:10.1016/j.brainresbull.2019.05.019
Wang, H., Liu, Y., Wang, D., Xu, Y., Dong, R., Yang, Y., et al. (2019c). The upstream pathway of mTOR-mediated autophagy in liver diseases. Cells 8 (12), 1597. doi:10.3390/cells8121597
Wang, X., Figueroa, B. E., Stavrovskaya, I. G., Zhang, Y., Sirianni, A. C., Zhu, S., et al. (2009). Methazolamide and melatonin inhibit mitochondrial cytochrome C release and are neuroprotective in experimental models of ischemic injury. Stroke 40 (5), 1877–1885. doi:10.1161/STROKEAHA.108.540765
Wang, X., Sirianni, A., Pei, Z., Cormier, K., Smith, K., Jiang, J., et al. (2011). The melatonin MT1 receptor axis modulates mutant Huntingtin-mediated toxicity. J. Neurosci. 31 (41), 14496–14507. doi:10.1523/JNEUROSCI.3059-11.2011
Watson, N., Diamandis, T., Gonzales-Portillo, C., Reyes, S., and Borlongan, C. V. (2016). Melatonin as an antioxidant for stroke neuroprotection. Cell. Transpl. 25 (5), 883–891. doi:10.3727/096368915x689749
Wei, N., Pu, Y., Yang, Z., Pan, Y., and Liu, L. (2019). Therapeutic effects of melatonin on cerebral ischemia reperfusion injury: role of Yap-OPA1 signaling pathway and mitochondrial fusion. Biomed. Pharmacother. 110, 203–212. doi:10.1016/j.biopha.2018.11.060
Wilkinson, B. L., and Landreth, G. E. (2006). The microglial NADPH oxidase complex as a source of oxidative stress in Alzheimer's disease. J. Neuroinflammation 3, 30. doi:10.1186/1742-2094-3-30
Williams, L., Bradley, L., Smith, A., and Foxwell, B. (2004). Signal transducer and activator of transcription 3 is the dominant mediator of the anti-inflammatory effects of IL-10 in human macrophages. J. Immunol. 172 (1), 567–576. doi:10.4049/jimmunol.172.1.567
Wong, P. M., Puente, C., Ganley, I. G., and Jiang, X. (2013). The ULK1 complex: sensing nutrient signals for autophagy activation. Autophagy 9 (2), 124–137. doi:10.4161/auto.23323
Xiang, J., Zhu, W., Yang, F., Yu, Z. H., Cai, M., Li, X. T., et al. (2020). Melatonin-induced ApoE expression in mouse astrocytes protects endothelial cells from OGD-R induced injuries. Transl. Psychiatry 10 (1), 181. doi:10.1038/s41398-020-00864-9
Xing, J., Xu, H., Liu, C., Wei, Z., Wang, Z., Zhao, L., et al. (2019). Melatonin ameliorates endoplasmic reticulum stress in N2a neuroblastoma cell hypoxia-reoxygenation injury by activating the AMPK-Pak2 pathway. Cell. Stress Chaperones 24 (3), 621–633. doi:10.1007/s12192-019-00994-0
Xu, H. D., and Qin, Z. H. (2019). Beclin 1, bcl-2 and autophagy. Adv. Exp. Med. Biol. 1206, 109–126. doi:10.1007/978-981-15-0602-4_5
Xu, Q., and Cheung, R. T. F. (2023). Melatonin mitigates type 1 diabetes-aggravated cerebral ischemia-reperfusion injury through anti-inflammatory and anti-apoptotic effects. Brain Behav. 13 (9), e3118. doi:10.1002/brb3.3118
Xu, X., Lai, Y., and Hua, Z. C. (2019). Apoptosis and apoptotic body: disease message and therapeutic target potentials. Biosci. Rep. 39 (1). doi:10.1042/BSR20180992
Yang, B., Zang, L. E., Cui, J. W., Zhang, M. Y., Ma, X., and Wei, L. L. (2020). Melatonin plays a protective role by regulating miR-26a-5p-NRSF and JAK2-STAT3 pathway to improve autophagy, inflammation and oxidative stress of cerebral ischemia-reperfusion injury. Drug Des. Devel Ther. 14, 3177–3188. doi:10.2147/DDDT.S262121
Yang, Q. Q., and Zhou, J. W. (2019). Neuroinflammation in the central nervous system: symphony of glial cells. Glia 67 (6), 1017–1035. doi:10.1002/glia.23571
Yang, Y., Jiang, S., Dong, Y., Fan, C., Zhao, L., Yang, X., et al. (2015). Melatonin prevents cell death and mitochondrial dysfunction via a SIRT1-dependent mechanism during ischemic-stroke in mice. J. Pineal Res. 58 (1), 61–70. doi:10.1111/jpi.12193
Yang, Z., Bao, Y., Chen, W., and He, Y. (2020). Melatonin exerts neuroprotective effects by attenuating astro- and microgliosis and suppressing inflammatory response following spinal cord injury. Neuropeptides 79, 102002. doi:10.1016/j.npep.2019.102002
Yang, Z., and Klionsky, D. J. (2010). Mammalian autophagy: core molecular machinery and signaling regulation. Curr. Opin. Cell. Biol. 22 (2), 124–131. doi:10.1016/j.ceb.2009.11.014
Yang, Z., Wang, Y., Zhang, Y., He, X., Zhong, C. Q., Ni, H., et al. (2018). RIP3 targets pyruvate dehydrogenase complex to increase aerobic respiration in TNF-induced necroptosis. Nat. Cell. Biol. 20 (2), 186–197. doi:10.1038/s41556-017-0022-y
Yawoot, N., Sengking, J., Govitrapong, P., Tocharus, C., and Tocharus, J. (2023). Melatonin modulates the aggravation of pyroptosis, necroptosis, and neuroinflammation following cerebral ischemia and reperfusion injury in obese rats. Biochim. Biophys. Acta Mol. Basis Dis. 1869 (7), 166785. doi:10.1016/j.bbadis.2023.166785
Yawoot, N., Sengking, J., Wicha, P., Govitrapong, P., Tocharus, C., and Tocharus, J. (2022). Melatonin attenuates reactive astrogliosis and glial scar formation following cerebral ischemia and reperfusion injury mediated by GSK-3β and RIP1K. J. Cell. Physiol. 237 (3), 1818–1832. doi:10.1002/jcp.30649
Yilmaz, U., Tanbek, K., Gul, S., Gul, M., Koc, A., and Sandal, S. (2023). Melatonin attenuates cerebral ischemia/reperfusion injury through inducing autophagy. Neuroendocrinology 113 (10), 1035–1050. doi:10.1159/000531567
Yip, H. K., Dubey, N. K., Lin, K. C., Sung, P. H., Chiang, J. Y., Chu, Y. C., et al. (2021). Melatonin rescues cerebral ischemic events through upregulated tunneling nanotube-mediated mitochondrial transfer and downregulated mitochondrial oxidative stress in rat brain. Biomed. Pharmacother. 139, 111593. doi:10.1016/j.biopha.2021.111593
Yousufuddin, M., Moriarty, J. P., Lackore, K. A., Zhu, Y., Peters, J. L., Doyle, T., et al. (2020). Initial and subsequent 3-year cost after hospitalization for first acute ischemic stroke and intracerebral hemorrhage. J. Neurol. Sci. 419, 117181. doi:10.1016/j.jns.2020.117181
Yu, L., Chen, Y., and Tooze, S. A. (2018). Autophagy pathway: cellular and molecular mechanisms. Autophagy 14 (2), 207–215. doi:10.1080/15548627.2017.1378838
Zang, M., Zhao, Y., Gao, L., Zhong, F., Qin, Z., Tong, R., et al. (2020). The circadian nuclear receptor RORα negatively regulates cerebral ischemia-reperfusion injury and mediates the neuroprotective effects of melatonin. Biochim. Biophys. Acta Mol. Basis Dis. 1866 (11), 165890. doi:10.1016/j.bbadis.2020.165890
Zawilska, J. B., Skene, D. J., and Arendt, J. (2009a). Physiology and pharmacology of melatonin in relation to biological rhythms. Pharmacol. Rep. 61 (3), 383–410. doi:10.1016/s1734-1140(09)70081-7
Zawilska, J. B., Skene, D. J., and Arendt., J. (2009b). Physiology and pharmacology of melatonin in relation to biological rhythms. Pharmacol. Rep. 61 (3), 383–410. doi:10.1016/s1734-1140(09)70081-7
Zeng, L., He, J., Liu, C., Zhang, F., Zhang, Z., Chen, H., et al. (2022). Melatonin attenuates ropivacaine-induced apoptosis by inhibiting excessive mitophagy through the Parkin/PINK1 pathway in PC12 and HT22 cells. Inflammation 45 (2), 725–738. doi:10.1007/s10753-021-01579-9
Zha, J., Harada, H., Yang, E., Jockel, J., and Korsmeyer, S. J. (1996). Serine phosphorylation of death agonist BAD in response to survival factor results in binding to 14-3-3 not BCL-X(L). Cell. 87 (4), 619–628. doi:10.1016/s0092-8674(00)81382-3
Zhang, M., An, C., Gao, Y., Leak, R. K., Chen, J., and Zhang, F. (2013). Emerging roles of Nrf2 and phase II antioxidant enzymes in neuroprotection. Prog. Neurobiol. 100, 30–47. doi:10.1016/j.pneurobio.2012.09.003
Zhang, Y., Wang, Y., Xu, J., Tian, F., Hu, S., Chen, Y., et al. (2019). Melatonin attenuates myocardial ischemia-reperfusion injury via improving mitochondrial fusion/mitophagy and activating the AMPK-OPA1 signaling pathways. J. Pineal Res. 66 (2), e12542. doi:10.1111/jpi.12542
Zhao, Y., Wang, H., Chen, W., Chen, L., Liu, D., Wang, X., et al. (2019a). Melatonin attenuates white matter damage after focal brain ischemia in rats by regulating the TLR4/NF-κB pathway. Brain Res. Bull. 150, 168–178. doi:10.1016/j.brainresbull.2019.05.019
Zhao, Z., Lu, C., Li, T., Wang, W., Ye, W., Zeng, R., et al. (2018). The protective effect of melatonin on brain ischemia and reperfusion in rats and humans: in vivo assessment and a randomized controlled trial. J. Pineal Res. 65 (4), e12521. doi:10.1111/jpi.12521
Zheng, Y., Hou, J., Liu, J., Yao, M., Li, L., Zhang, B., et al. (2014). Inhibition of autophagy contributes to melatonin-mediated neuroprotection against transient focal cerebral ischemia in rats. J. Pharmacol. Sci. 124 (3), 354–364. doi:10.1254/jphs.13220fp
Zhi, S. M., Fang, G. X., Xie, X. M., Liu, L. H., Yan, J., Liu, D. B., et al. (2020). Melatonin reduces OGD/R-induced neuron injury by regulating redox/inflammation/apoptosis signaling. Eur. Rev. Med. Pharmacol. Sci. 24 (3), 1524–1536. doi:10.26355/eurrev_202002_20211
Zhou, M., and Baudry, M. (2006). Developmental changes in NMDA neurotoxicity reflect developmental changes in subunit composition of NMDA receptors. J. Neurosci. 26 (11), 2956–2963. doi:10.1523/JNEUROSCI.4299-05.2006
Zhou, Z., Lu, J., Liu, W. W., Manaenko, A., Hou, X., Mei, Q., et al. (2018). Advances in stroke pharmacology. Pharmacol. Ther. 191, 23–42. doi:10.1016/j.pharmthera.2018.05.012
Zou, L. Y., Cheung, R. T., Liu, S., Li, G., and Huang, L. (2006). Melatonin reduces infarction volume in a photothrombotic stroke model in the wild-type but not cyclooxygenase-1-gene knockout mice. J. Pineal Res. 41 (2), 150–156. doi:10.1111/j.1600-079X.2006.00349.x
Keywords: melatonin, cerebral ischemia-reperfusion injury, ischemic stroke, neuroprotection, systematic review
Citation: Zhang C, Ma Y, Zhao Y, Guo N, Han C, Wu Q, Mu C, Zhang Y, Tan S, Zhang J and Liu X (2024) Systematic review of melatonin in cerebral ischemia-reperfusion injury: critical role and therapeutic opportunities. Front. Pharmacol. 15:1356112. doi: 10.3389/fphar.2024.1356112
Received: 15 December 2023; Accepted: 22 January 2024;
Published: 05 February 2024.
Edited by:
Francisco Lopez-Munoz, Camilo José Cela University, SpainReviewed by:
Xiang Xu, Soochow University, ChinaGabriela Del Carmen López-Armas, Centro de Ensenanza Técnica Industrial, Mexico
Xun Wu, Air Force Medical University, China
Copyright © 2024 Zhang, Ma, Zhao, Guo, Han, Wu, Mu, Zhang, Tan, Zhang and Liu. This is an open-access article distributed under the terms of the Creative Commons Attribution License (CC BY). The use, distribution or reproduction in other forums is permitted, provided the original author(s) and the copyright owner(s) are credited and that the original publication in this journal is cited, in accordance with accepted academic practice. No use, distribution or reproduction is permitted which does not comply with these terms.
*Correspondence: Jian Zhang, anpoYW5nQGNtdS5lZHUuY24=; Xu Liu, dmFsZW50aW5lMTEyMEAxMjYuY29t
†These authors have contributed equally to this work and share first authorship