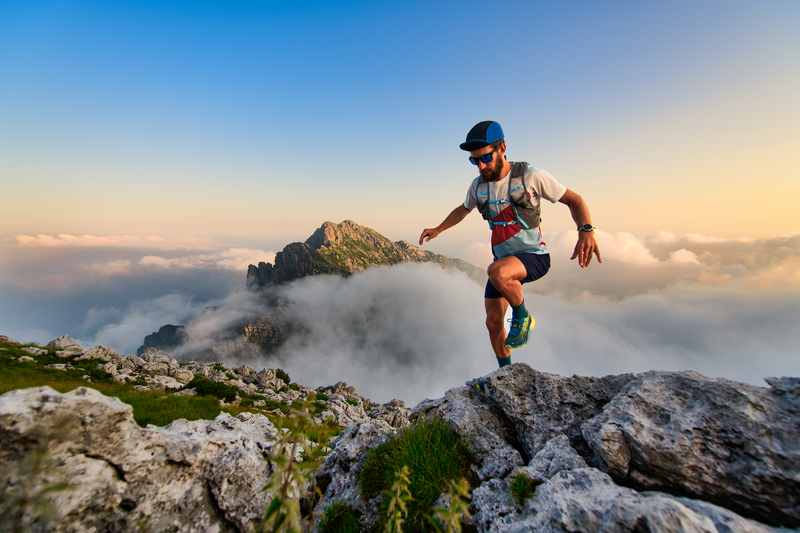
94% of researchers rate our articles as excellent or good
Learn more about the work of our research integrity team to safeguard the quality of each article we publish.
Find out more
REVIEW article
Front. Pharmacol. , 24 August 2022
Sec. Pharmacology of Anti-Cancer Drugs
Volume 13 - 2022 | https://doi.org/10.3389/fphar.2022.933655
This article is part of the Research Topic Application of Next-generation Sequencing for Cancer Drug Repositioning View all 10 articles
Gliomas are the most common malignant brain tumors. High-grade gliomas, represented by glioblastoma multiforme (GBM), have a poor prognosis and are prone to recurrence. The standard treatment strategy is tumor removal combined with radiotherapy and chemotherapy, such as temozolomide (TMZ). However, even after conventional treatment, they still have a high recurrence rate, resulting in an increasing demand for effective anti-glioma drugs. Drug repurposing is a method of reusing drugs that have already been widely approved for new indication. It has the advantages of reduced research cost, safety, and increased efficiency. Disulfiram (DSF), originally approved for alcohol dependence, has been repurposed for adjuvant chemotherapy in glioma. This article reviews the drug repurposing method and the progress of research on disulfiram reuse for glioma treatment.
Glioma is a central nervous system (CNS) tumor and is one of the most common malignant brain tumors (Özcan et al., 2021), accounting for approximately 80% of all brain-related malignancies (Ostrom et al., 2019). According to the World Health Organization (WHO) classification, gliomas are divided into four grades based on their malignancy. Grades I–II are low-grade gliomas (LGGs), whereas grades III–IV are called high-grade gliomas (HGGs) (Özcan et al., 2021). Glioblastomas (GBMs) are WHO grade IV tumors with a high degree of malignancy and a median overall survival of approximately 15–26 months (Seliger and Hau, 2018).
Conventional treatment only modestly prolongs survival (Jakola et al., 2018). The growing demand for effective anticancer drugs has led researchers to search for Food and Drug Administration (FDA)-approved drugs that can be reused as chemotherapeutic agents (McMahon et al., 2020). Drug repurposing, also known as drug repositioning, drug reprofiling, drug redirecting, drug rediscovery, and others (Langedijk et al., 2015), is a promising treatment strategy for reusing drugs with known formulations, pharmacokinetics, toxicity, clinical trials, and post-marketing surveillance safety data that offer increased scope for use (Ashburn and Thor, 2004; Turanli et al., 2021).
Disulfiram (DSF), also known as antabuse, is an FDA-approved acetaldehyde dehydrogenase inhibitor (Shirley et al., 2021; Turanli et al., 2021). Figure 1 shows the molecular structure of DSF. It has been used for treating alcohol dependence for the past 70 years with good pharmacokinetic properties, safety, and tolerability owing to the flu-like symptoms that patients experience when consuming alcohol (Seliger and Hau, 2018; Shirley et al., 2021). Preclinical and clinical studies have shown that DSF exhibits broad-spectrum anticancer activity against a variety of cancer types when administered with copper (Cu)-containing supplements (McMahon et al., 2020). Therefore, this review describes the drug repurposing of DSF in gliomas.
The standard initial treatment for GBM is extensive neurosurgical resection, followed by postoperative segmental radiotherapy, temozolomide (TMZ) chemotherapy, and combined adjuvant therapy (Jakola et al., 2018; Stylli, 2020). The therapy using some natural and synthetic anti-glioma agents, such as medicinal cannabis or cannabinoids, bipolaris setariae fungi, oncolytic viruses, neurostatin, and fatty acid synthase (FAS) inhibitors, can also help combat gliomas (Anjum et al., 2017). Despite comprehensive strategies, residual GBM cells can develop treatment resistance (TR), resulting in GBM recurrence within a median time of approximately 7 months (Louis et al., 2016; Liu C. C. et al., 2021).
TMZ is a chemotherapeutic agent specifically used for brain cancer and was approved for recurrent mesenchymal astrocytoma in the United States in 1999 and recurrent mesenchymal astrocytoma and glioblastoma in Europe in 2000 (Mutter and Stupp, 2006). However, mitozolomide, the essential compound of TMZ, entered the clinic in 1983 (Newlands et al., 1997) and a phase I trial was completed in 1985 (Newlands et al., 1985), indicating that TMZ was developed over 16 years. A randomized clinical study conducted by Stupp in 2005 showed that adding TMZ to radiotherapy improved the 2-year survival rate of patients with GBM from 10.9% to 27.2% with minimal toxicity (Stupp et al., 2005). Since then, TMZ has been used as the first-line chemotherapeutic agent for gliomas (Stylli, 2020).
However, it is estimated that at least 50% of patients treated with TMZ do not respond to it, exhibiting innate or acquired chemoresistance, which ultimately leads to tumor recurrence (Lee, 2016). Presumably, during the window of chemotherapy with TMZ, most tumor cells are vulnerable and killed and may not have acquired full resistance (Osuka and Van Meir, 2017). Eventually, tumor cells resistant to multiple therapies persist in the brain parenchyma surrounding the tumor cavity and become the basis of tumor regeneration and recurrence (Osuka and Van Meir, 2017).
Given the slow and costly development of new therapies, drug repurposing has become an attractive strategy (Stylli, 2020). For example, a trial on metformin for GBM in 2015 showed that metformin prolonged the survival of patients with glioblastoma and diabetes (Adeberg et al., 2015). However, a pooled analysis questioned this finding and showed that metformin did not prolong survival in patients with GBM (Seliger et al., 2020). Similarly, celecoxib, as an adjuvant to TMZ chemotherapy, has shown good tolerability, but its efficacy in terms of survival benefits for patients remains uncertain (Stockhammer et al., 2010). The classical antimalarial drug, chloroquine, has been found to kill various cancer types, including GBM, through drug repurposing (Weyerhäuser et al., 2018). However, its concentration threshold for killing tumor cells under laboratory conditions is much higher than the clinically tolerable dose; therefore, its clinical value remains unclear (Weyerhäuser et al., 2018).
TMZ has shown great therapeutic value; however, the rate of drug resistance and recurrence of glioma remains reasonably high. Drug repurposing has contributed to the discovery of new therapies, but clinical application remains slow. Thus, there is an urgent need for developing new adjuvant chemotherapeutic agents.
Drug repurposing can shorten development times by 5–7 years, reduce research investment costs by accounting for less than 10% of new compounds, and avoid risks such as a constant weight of generics (Cha et al., 2018). Moreover, a strict and burdensome regulatory process for new drugs forces developers to explore novel therapeutic uses for existing drugs (Turanli et al., 2021). Drug repurposing provides sufficient assurance regarding the safety, efficacy, and administration route of existing drugs (Parvathaneni et al., 2019; Juárez-López and Schcolnik-Cabrera, 2021; Turanli et al., 2021). Studies have shown that the cost and time to develop DSF as an anticancer drug are reduced by over 40% with an estimated annual cost of approximately $550 with DSF 500 mg per day (Cvek, 2012).
Drug repurposing facilitates the passage of projects and improves development efficiency. Only a minority of drug development projects can obtain FDA approval among newly developed drugs, compared with over 65% of drug repurposing projects (Masoudi-Sobhanzadeh et al., 2019). Recently, drug repurposing projects have accounted for approximately 30% of all newly approved drugs by the FDA (Parvathaneni et al., 2019). Additionally, drug repurposing can allow the revaluation of drugs that failed in the development phase for other uses and the change of application settings for better use (Masoudi-Sobhanzadeh et al., 2019).
As high-throughput screening and computational biology methods advance, accumulated data lay the foundation for new approaches to rational drug repurposing (Turanli et al., 2021). Currently, numerous drug repurposing databases are available, allowing easy access to drug repurposing research, and systematic analysis is now accessible on platforms or screening systems dedicated to identifying repurposable drug candidates (Juárez-López and Schcolnik-Cabrera, 2021).
There are multiple information-gathering methods for drug repurposing. Electronic drug repurposing involves using various public databases to gather information from research, clinical trials, utilization reports, and other published data, and then to identify drug targets and networks of drug-drug interactions with the help of bioinformatics tools and artificial intelligence (Kumar et al., 2019). Text mining methods are used to discover new information by extracting aggregated new information from multiple published resources with the help of a computer, and by obtaining a large amount of data on conceptual relationships in biology from publications (Kumar et al., 2019). Clustering methods are applied to display and discover new drug targets or drug-disease relationships through various modules, groups, or subnetworks using clustering algorithms (Kumar et al., 2019). Propagation methods are based on information transmission from the source node to the network nodes and individual subnetwork nodes to determine the relationship between disease genes and target diseases (Emig et al., 2013). Semantic approaches seek biological entity relationships from medical databases, build semantic networks based on existing ontology networks, develop algorithms to discover the relationships, and extract medical information and image resources for drug reuse (Xue et al., 2018). Biological approaches include using systems and network biology to develop various models for drug reutilization studies that mimic the physiological environment of the target protein and modulate the outcome of its action, particularly targeting multifactorial complex diseases (Pujol et al., 2010). Knowledge-based empirical approaches are methods based on the knowledge of researchers and physicians and their ability, experience, and skills to interpret observations, with the opportunity for the serendipitous discovery of new drug utilization pathways (Kumar et al., 2019). Experimental methods include target screening, cellular analysis, animal models, and clinical trials (Kumar et al., 2019). These various methods of drug repurposing have been developed to evaluate the clinical efficacy for glioma treatment.
Advances in biotechnology, bioinformatics, and histological techniques (proteomics, genomics, metabolomics, etc.) have facilitated the development of several databases in biology, chemistry, medicine, and pharmacology (Kumar et al., 2019).
Basic databases for drug repurposing, such as DrugBank (Wishart et al., 2018), DGIdb (Cotto et al., 2018), and KEGG (Kanehisa et al., 2017), can provide information on drugs, targets, and pathways (Masoudi-Sobhanzadeh et al., 2019; Turanli et al., 2021). Recently, the newly developed DrugR + database based on DrugBank and KEGG provides a new source of information for the single application and reuse of drugs (Masoudi-Sobhanzadeh et al., 2019). The DrugR + database supports not only specialist users with structured query language (SQL) query functions, but also nonspecialist users with different options for targeted functions (Masoudi-Sobhanzadeh et al., 2019). The DrugR + database can also be readjusted to include drug use and provide a list of potential drugs for certain uses (Masoudi-Sobhanzadeh et al., 2019). The DrugR + database has several advantages, such as 1) providing a suitable and simple way to search for and obtain information about drugs with no technical problems, 2) providing information on the reusability of drugs, 3) allowing the selection of different types of research targets to obtain lists of drugs for diseases, and 4) providing up-to-date information on new drugs and the latest research (Masoudi-Sobhanzadeh et al., 2019).
Drug repurposing databases contain news, articles, and results obtained from drug repurposing studies (Masoudi-Sobhanzadeh et al., 2019), such as RepoDB (Brown and Patel, 2017b), Excelra (Arora et al., 2017), Drug Repurposing Hub (DRH) (Corsello et al., 2017), and TTD (Yang et al., 2016). Databases such as PREDICT (Gottlieb et al., 2011) and RepurposeDB (Shameer et al., 2018) summarize similarities between one drug or target and another (Masoudi-Sobhanzadeh et al., 2019). ChemMapper and iDrug Target are databases that assess ligand similarity (Sam and Athri, 2019). Drug target-based databases, such as DMAP (Huang et al., 2015), DrugSig (Wu et al., 2017), DDW (Holland, 2016), PDID, and idTarget (Sam and Athri, 2019), summarize drugs and their various targets, based on which new drug application pathways is proposed (Masoudi-Sobhanzadeh et al., 2019).
There are other databases such as ConnectivityMap (Lamb et al., 2006) for drug-induced gene expression studies, LINCS (Koleti et al., 2018) and GEO (Barrett et al., 2013) for transcriptomic characterization of tumor tissue from patients with cancer, CSNAP (Gaulton et al., 2012) and STITCH (Kuhn et al., 2012) for biological networks, RE:fine drugs (Moosavinasab et al., 2016) and MeSHDD (Brown and Patel, 2017a) linking drugs to disease databases, and PubChem (Wang et al., 2017) for comprehensive chemical and structural information on active ingredient components.
These databases cover an amount of information on drug targets, pathways, characteristics, and recent advances, allowing for exploring potentially efficient drugs.
The urgent need for glioma treatment and the application of DSF to cancer through drug repurposing has led to a steady stream of studies in recent years that have shown good results in treating glioma (Huang J. et al., 2016; Huang et al., 2018; Huang et al., 2019; Halatsch et al., 2021). Table 1 shows the information on DSF in the drug repurposing database.
In 1937, factory workers who were regularly exposed to DSF developed flu-like symptoms when they ingested alcohol (Triscott et al., 2015). DSF has been used to treat alcohol dependence since 1947 (Chick, 1999).
In the last 40 years, the anticancer effects of DSF have been discovered in vitro and in cancer xenografts (Conticello et al., 2012; Paranjpe et al., 2014). In 2003, Wang et al. suggested the possible clinical use of DSF in rectal cancer using cellular assays (Wang et al., 2003). In 2006, Chen et al. demonstrated through animal testing that DSF promotes selective apoptosis of tumor cells by inhibiting proteasome activity (Chen et al., 2006). In 2009, Iljin et al. systematically investigated the efficacy of most drugs and drug-like molecules already on the market against prostate cancer cells, and ultimately showed that DSF reduced tumor growth, induced metallothionein expression, and reduced DNA replication in vivo, indicating its potential as a therapeutic agent for prostate cancer (Iljin et al., 2009).
In 2009, Richard et al. speculated that DSF should be studied as an adjuvant to chemotherapy for glioblastoma based on its effect on acetaldehyde dehydrogenase (ALDH) in glioma (Kast and Belda-Iniesta, 2009), and since then the value of DSF in glioma has been focused. In 2012, Joanna et al. used the database approach for drug repurposing and selected DSF from numerous drugs that inhibit tumor-initiating cells using the Prestwick database (Triscott et al., 2012). They found that DSF inhibited PLK1 expression in GBM cells, suggesting that DSF could be repurposed for the treatment of refractory GBM (Triscott et al., 2012). In 2015, high ALDH1A1 expression was found to be associated with highly aggressive tumor cells and high-grade gliomas (Chen et al., 2006). ALDH1A1 promoted glioma progression, invasion, and proliferation, and led to poor prognosis (Chen et al., 2006). This finding brought the anti-glioma effects of DSF as an ALDH inhibitor back into the spotlight (Chen et al., 2006). In 2016, Huang et al. conducted the first phase I clinical trial using DSF in combination with TMZ for GBM treatment (Huang J. et al., 2016). In 2017, Karamanakos et al. combined DSF with standard treatment modalities to treat a patient with GBM and ultimately improved his prognosis, thereby confirming the clinical value of DSF (Karamanakos et al., 2017). In 2019, DSF was first reported to preferentially enhance radiosensitivity in GBM cells, particularly in radioresistant cells (Koh et al., 2019). In 2021, Meier et al. demonstrated the therapeutic value of DSF as a repurposed drug for treating gliomas in children (Meier et al., 2021). To date, relevant clinical trials are ongoing (NCT03363659, NCT03151772, NCT02715609, etc.).
Huang et al. (2016) conducted a phase I clinical trial. Twelve patients newly diagnosed with supratentorial primary GBM were studied using concomitant adjuvant DSF and TMZ chemotherapy after radiotherapy. Patients were divided into two groups and received 500 mg/day or 1000 mg/day of DSF in combination with TMZ chemotherapy. The results showed that the maximum tolerated dose of DSF was 500 mg/day. Although some associated adverse effects, such as fatigue, delirium, ataxia, dizziness, and peripheral motor/sensory neuropathy, existed, these adverse reactions were self-limiting and resolved within 30 days after DSF discontinuation (Huang J. et al., 2016).
In 2018, Huang et al. updated data from a phase I clinical trial. The study population comprised 18 patients newly diagnosed with GBM after standard radiotherapy. DSF was also administered during the chemotherapy phase of TMZ. Seven patients received DSF at 500 mg/day, five patients received DSF at 1000 mg/day, and six patients received DSF/Cu at 500 mg/day. The results showed that a maximum dose of 500 mg/day of DSF was well tolerated with or without combined Cu, while 1000 mg/day was poorly tolerated. Of the patients receiving 500 mg DSF with a combination of Cu per day, one patient suffered from nausea and diarrhea in the first 30 days, which was relieved after the reduction of DSF to 250 mg per day. Additionally, without the combination of Cu, one patient developed delirium after 1.6 months and one developed motor neuropathy after 2.6 months. All adverse reactions resolved rapidly after dose reduction or DSF discontinuation. Notably, a 40-year-old woman in the study who received 500 mg DSF per day discontinued DSF therapy after 2.6 months of treatment due to motor neuropathy. At 33 months after DSF treatment, the patient survived in good health with no signs of tumor recurrence (Huang et al., 2018).
Based on a previous study, Huang et al. conducted a phase II clinical trial in 2019. Twenty-three patients with recurrent TMZ-resistant GBM were enrolled in the study. DSF (80 mg) and Cu gluconate (1.5 mg) were administered orally thrice daily at approximately 4–8 hourly intervals in conjunction with TMZ chemotherapy. The results showed that 14% of the patients achieved clinical benefit over a stabilization period of over 6 months. The most common adverse effects were nausea and vomiting in 17% of patients, followed by dizziness (Huang et al., 2019).
In 2021, Marc et al. conducted a phase Ib/IIa clinical trial using a CUSP9 treatment regimen in combination with TMZ. Ten patients with GBM were included in this study. CUSP9 was gradually added at increasing doses during uninterrupted TMZ chemotherapy. When all drugs reached the target dose, the drug was maintained until side effects or tumor progression occurred. The results showed that the regimen was safe under clinical, laboratory, and electrocardiographic monitoring and that the side effects were mild and disappeared after discontinuation (Halatsch et al., 2021).
Since 2016, there have been updates on clinical trials using DSF as an adjuvant in the treatment of glioma. Evidence suggests that DSF has great therapeutic potential but with mild side effects; however, clinical studies are still inadequate.
DSF can act as an anticancer agent through various Cu- and zinc-dependent processes, including the inhibition of nuclear factor kappa B (NF-κB), NPL4, and phosphoglycerol dehydrogenase (Spillier et al., 2019). DSF produces oxidative stress by inhibiting NF-κB activation and superoxide dismutase (SOD) and inducing an increased ratio of oxidized glutathione to its reduced form (Tesson et al., 2017). DSF cytotoxicity is dependent on Cu (Ye et al., 2011). Cu plays a key role in redox reactions and triggers the generation of reactive oxygen species (ROS) in human cells (Liu et al., 2012). Many cancer types, including GBM, have significantly higher intracellular Cu levels than normal tissues, and DSF penetrates and chelates Cu intracellularly (Liu et al., 2012). As DSF cytotoxicity appears to be Cu-dependent, high Cu concentrations in cancer cells produce cytotoxicity through oxidative stress generated by the Fenton reaction (production of Cu ions and hydroxyl radicals) or through inhibiting enzymes that bind Cu to peptide bonds, allowing DSF to specifically target cancer cells and preserve normal tissue (Liu et al., 2012; Tesson et al., 2017).
Approximately 80–95% of the orally administered DSF is absorbed and the unabsorbed portion is excreted (Shirley et al., 2021). In the body, DSF is rapidly metabolized to DTC acid and then rapidly formed as diethylthiocarbamic acid methyl ester (diethyldithiomethylcarbamate, MeDTC) or broken down into carbon disulphide and dimethylamine (Shirley et al., 2021). MeDTC inhibits ALDH and is a strong metal chelate that can form complexes with metal ions (Shirley et al., 2021). DSF is also reduced to DTC in the stomach and forms metal-ion complexes in the gastrointestinal tract (Shirley et al., 2021). DTC metal complexes have a relatively long half-life, are widely distributed throughout the body, and penetrate the blood-brain barrier (BBM) (Shirley et al., 2021). This pharmacological mechanism makes it possible for DSF to kill glioma cells specifically. During DSF treatment, alcohol ingestion leads to acetaldehyde accumulation due to ALDH inhibition, causing the DSF-ethanol reaction (Jørgensen et al., 2011). This reaction manifests as tachypnea, tachycardia, facial flushing, nausea, vomiting, hypotension, and even cardiovascular collapse (Jørgensen et al., 2011).
DSF has a symmetric structure, and its first metabolic step is reducing the disulfide bond at the center of the molecule to produce two diethyldithiocarbamate (DTC) fractions (Lipsky et al., 2001a). DTC is further converted to its methyl ester and other metabolites (Lipsky et al., 2001a). DTC is a potent ALDH inhibitor, which forms a mixed disulfide bond with the key cysteine near the active site (Lipsky et al., 2001a). DSF significantly alters alcohol metabolism and treats chronic alcohol dependence by irreversibly inhibiting ALDH and causing acetaldehyde accumulation (Jørgensen et al., 2011). ALDH belongs to a family of metabolic enzymes that catalyze the oxidation of aldehydes, a toxic alcohol metabolism product (Lipsky et al., 2001b). ALDH promotes cell survival by protecting DNA from genotoxic damage and providing resistance to a wide range of anticancer drugs (Hothi et al., 2012). Therefore, ALDH inhibition is an effective way to sensitize resistant cell populations to the cytotoxic effects of chemotherapeutic drugs (Hothi et al., 2012). The strong expression of ALDH is a prominent feature of normal and cancer stem cells, including the stem cell subpopulation of glioblastoma (Kast and Belda-Iniesta, 2009). In GBM and other cancers, increased ALDH expression is observed in a small subpopulation of tumor cells with stem cell properties (Rappa et al., 2013). ALDH expression is associated with the anti-apoptotic capacity of glioma stem cells (GSCs) and the protection of DNA against damage by ROS and aldehydes (Kast and Belda-Iniesta, 2009). DSF and its metabolites form mixed disulfide bonds with key cysteines (Cys302) near the active site of ALDH to inactivate it (Paranjpe et al., 2014). With ALDH inhibition, the division of stem cells into non-stem daughter cells is blocked (Kast and Belda-Iniesta, 2009). However, such inhibitory effect of DSF on ALDH does not affect normal neural stem cells or fibroblasts (Choi et al., 2015).
MLL1 and MLL2 are human homologs of the Drosophila epigenetic regulator Trithorax (Trx) (Schuettengruber et al., 2017). MLL1 promotes tumor stem cell characteristics, cell growth, and tumorigenicity in adult glioblastomas (Meier et al., 2021). MLL2 mutations are found in 14% of patients with medulloblastoma (Parsons et al., 2011). Studies have shown that DSF can effectively kill both childhood glioma stem cells at low concentrations and glioma cell lines at slightly higher concentrations by inducing MLL degradation (Meier et al., 2021).
DSF-mediated cytotoxicity is partially due to increased ROS production. Elevated ROS levels are the major mode of DSF-mediated cell death (Kast et al., 2014). DSF/Cu induces ROS in GBM cell lines, which activates the JNK and p38 pathways, and inhibits NF-κB activity (Liu et al., 2012). DSF/Cu eliminates stem cell-like cell populations in GBM cell lines by modulating the Bcl2 family to trigger the intrinsic apoptotic pathway (Liu et al., 2012). DSF rapidly inhibits superoxide dismutase 1 (SOD1) in murine microglia to induce neurotoxic microglia activation (Dimayuga et al., 2007). ROS production by activated microglia directs redox-sensitive inflammatory signaling and initiates neurotoxic inflammation (Dimayuga et al., 2007). The inhibition of activated microglia might lower the neurological side effects of DSF during the treatment.
O6-methylguanine-DNA methyltransferase (MGMT) is a DNA repair protein and chemotherapeutic target that is highly expressed in approximately 80% of brain tumors and other cancers (Gerson, 2002). MGMT, as an anti-mutagenic DNA repair protein, can remove mutagenic O6-alkyl groups from guanine and interfere with the cytotoxic effects of alkylating agents to make tumors resistant (Gerson, 2002). The survival of patients with GBM depends on the MGMT promoter methylation status (Koh et al., 2019). Those with methylated MGMT promoter (MGMT meth) had a higher survival rate than those with the wild-type (MGMT wt) (Paranjpe et al., 2014; Koh et al., 2019). Thus, MGMT has become a central determinant of tumor resistance to alkylating agents (Paranjpe et al., 2014). DSF causes MGMT degradation (Srivenugopal et al., 2016) and synergistically inhibits the growth and renewal of TMZ-resistant GBM cells (Triscott et al., 2012). Compared to normal astrocytes, DSF induces a preferential increase in radiosensitivity in GBM cells, causing increased apoptosis and delayed DNA damage repair (Koh et al., 2019). DSF-induced radiosensitization is more pronounced in radioresistant cells, especially drug-resistant GBM cells with wild-type non-methylated MGMT promoters (Koh et al., 2019). In brain tumor cell lines, DSF reduced MGMT activity in a rapid and dose-dependent manner (Paranjpe et al., 2014). Of these, DSF/Cu was approximately five times more potent than DSF in inhibiting MGMT activity in cultured brain tumor cells (Paranjpe et al., 2014). There are no reports about adverse effects on normal neuronal cells caused by this mechanism.
NF-κB promotes disease progression by increasing tumor cell proliferation, inducing the transcription of anti-apoptotic genes and genes involved in the DNA damage response, and promoting angiogenesis (Voorhees and Orlowski, 2006). NF-κB activation is associated with radioresistance, particularly through inducing anti-apoptotic and antioxidant gene expression (Tesson et al., 2017). DSF is an NF-κB inhibitor, which interferes with TGF-β-induced epithelial-mesenchymal transition in cancer (Han et al., 2015). The blockade of NF-κB activation by DSF reduces tumor volume and cell invasion (Westhoff et al., 2013). The aggressiveness of GBM is associated with the secretion and processing of fibronectin by GBM cells via fibrinogen and matrix metallopeptidases (Mettang et al., 2018). GBM causes reduced intercellular interactions by creating this new extracellular matrix (ECM)-based microenvironment, and generating a stress response that triggers NF-κB activation and enhances cell-matrix adhesion of GBM (Mettang et al., 2018). DSF blockade of NF-κB activation inhibits cell-matrix adhesion between GBM and the brain tissue microenvironment, and reduces tumor volume and cell invasion (Mettang et al., 2018). Other drugs that modulate fibrinogen, metallopeptidases, and fibronectin in the microenvironment of glioma cells might synergistically enhance the anti-invasive effects of DSF.
Proteasome activity promotes tumorigenesis by enhancing tumor cell proliferation, down-regulating apoptosis, and promoting angiogenesis (Voorhees and Orlowski, 2006). Therefore, the ubiquitin-proteasome pathway is an important target for cancer therapy (Voorhees and Orlowski, 2006). DSF inhibits proteasome activation (Voorhees and Orlowski, 2006), leading to the accumulation of misfolded proteins and potentially toxic protein aggregates (Hothi et al., 2012), subsequently inducing tumor cell death (Hothi et al., 2012). The DSF activity depends on the presence of Cu ions, and Cu thiocarbamate complexes act as proteasome inhibitors (Hothi et al., 2012). As a potent proteasome inhibitor, DSF/Cu can functionally impair the DNA repair pathways and enhance the effects of DNA alkylating agents and radiation (Lun et al., 2016). DSF/Cu inhibits the chymotrypsin-like proteasome activity in cultured glioma stem cells (GSC), consistent with the inactivation of the ubiquitin-proteasome pathway and subsequent tumor cell death induction (Hothi et al., 2012).
Polo-like kinase 1 (PLK1), which is highly expressed in tumor cells, is a key serine/threonine kinase involved in many important cell cycle functions such as mitotic entry, centrosome maturation, cell cycle progression, and cytoplasmic division (Triscott et al., 2012). As GBM tumors with higher levels of PLK1 expression have a higher incidence and poorer prognosis, PLK1 could be a promising therapeutic target for brain tumors (Triscott et al., 2012). DSF leads to downregulation of cell cycle kinase PLK1 in GBM cells (Triscott et al., 2012).
DSF is involved in the regulation of complicated molecular mechanisms. These molecular mechanisms of DSF are shown in Figure 2.
The standard dosage following FDA-approved indications for managing chronic alcohol use is 250 mg/day orally, with a maximum dose of 500 mg/day (Ekinci et al., 2019). Additionally, the nasal-brain pathway is considered a safe and effective alternative for direct drug delivery to the CNS (Landis et al., 2012). Administration via the nasal cavity to the CNS bypasses the blood-brain barrier and avoids hepatic first-pass effects (Qu et al., 2021). The clinical application of DSF as an anticancer drug is limited by its poor oral bioavailability and rapid metabolism in vivo (Shergill et al., 2016). It has been shown that DSF encapsulation in hydroxypropyl-β-cyclodextrin (HP-β-CD) produces a DSF complex with enhanced solubility (Qu et al., 2021). In vitro anti-GBM activity and safety (DSF/HP-β-CD/Cu) can increase the aqueous solubility of DSF by approximately 2,450-fold and may be a promising intranasal agent to treat (Qu et al., 2021). Animal studies have shown that DSF/HP-β-CD/Cu significantly inhibited tumor growth and migration, promoted tumor apoptosis, and prolonged the median survival time in male glioma rats in the intranasal administration group (Qu et al., 2021). Besides oral and nasal-brain access, 1,2-distearoyl-sn-glycero-3-phosphocholine (DSPC)/cholesterol liposomes to prepare DSF as an injectable Cu(DDC)2 formulation are also therapeutically active (Wehbe et al., 2017).
Combinations of two or more drugs with different mechanisms of action, also referred to as combination therapy, is an alternative strategy for improving the success of drug repurposing (Masoudi-Sobhanzadeh et al., 2019). Compared with single-drug therapy, it reduces the incidence/emergence of resistance mechanisms, doses of drugs, and adverse effects, and improves the synergistic effects and success of treatment modalities (Masoudi-Sobhanzadeh et al., 2019). Combination therapy allows simultaneous targeting of multiple therapeutic genes, and is currently the most effective treatment for aggressive tumors, such as GBM (Ghosh et al., 2018). Combination therapy of DSF with other modalities holds great promise.
Preclinical and clinical studies have shown that DSF has broad-spectrum anticancer activity against a variety of cancer types when combined with Cu-containing supplements, such as Cu gluconate (McMahon et al., 2020). The combination regimen comprised 500 mg of DSF daily plus 50 mg of zinc gluconate or 2 mg of Cu gluconate three times daily (Ekinci et al., 2019). It has been shown that DSF/Cu sulphide (CuS) nanocomplexes (Tf-DSF/CuS) modified with transferrin (Tf) exhibit high cytotoxic effects in vitro (Lan et al., 2021). The CuS nanoparticles enable them to accumulate specifically and within tumor tissue by enhancing the permeability and retention (EPR) effect of the tumor tissue (Lan et al., 2021). The metallic nature of the nanoparticles enhanced the drug-loading capacity by forming Cu complexes on the CuS surface (Lan et al., 2021). Additionally, the use of a long-wavelength laser in the near-infrared (700–1000 nm) region activated the photothermal effect of the nanoparticles, resulting in minimal damage to normal tissue from tumor ablation (Lan et al., 2021). Besides Cu, DSF can act through zinc chelation to inhibit the activities of MMP-2 and MMP-9 (Kast and Halatsch, 2012).
In vitro cytotoxicity is enhanced by the addition of DSF to standard chemotherapeutic agents (Rappa et al., 2013) such as cisplatin (O'Brien et al., 2012), TMZ (Triscott et al., 2012), paclitaxel (Yip et al., 2011), gemcitabine (Cunningham and Chaiton, 2018), doxorubicin (Budman and Calabro, 2002), cyclophosphamide (Moreb et al., 2012), 5-fluorouracil (Wang et al., 2003), and adriamycin (Xu et al., 2011). The combination regimens for DSF against gliomas are described below.
The 5-year survival rate of patients with GBM treated with radiation alone was 1.9%, and TMZ, in conjunction with radiation therapy, only increased the rate to 9.8% (Triscott et al., 2015). Additionally, TMZ toxicity is often not well tolerated by patients, and other modalities of TMZ resistance, such as MGMT expression, make it complicated (Triscott et al., 2015).
DSF is highly effective in cases where cells develop TMZ resistance (Triscott et al., 2012). DSF has synergistic activity when combined with TMZ, which is highly effective against TMZ-resistant cells (Lun et al., 2016). In the presence of low Cu doses, DSF significantly enhanced TMZ activity in vitro (Lun et al., 2016). In vivo studies have confirmed that DSF and Cu have synergistic effects with TMZ and improve survival in mice with in situ GBM tumors (Lun et al., 2016).
DSF can be safely used in combination with TMZ, although it causes reversible neurotoxicity (Huang J. et al., 2016). At a maximum tolerated dose (MTD) of 500 mg/day of DSF with TMZ without concomitant Cu administration, slight proteasomal inhibition of peripheral blood cells was observed after 4 weeks, with an average reduction of approximately 5% (Huang J. et al., 2016). A dose-response trend appeared with doubling proteasome inhibition at a dose of 1000 mg/day (Huang J. et al., 2016). The results of a phase II clinical study in 23 patients showed that adding DSF/Cu to TMZ was safe and well tolerated in TMZ-resistant IDH wild-type GBM (Huang et al., 2019).
DSF enhances cisplatin-induced cytotoxicity by directly damaging DNA (O'Brien et al., 2012). Activating transcription factor 3 (ATF3) exerts a pro-apoptotic effect in response to cisplatin by directly binding to and activating vascular endothelial cells (O'Brien et al., 2012). It has been shown that the combination of cisplatin and DSF plays a synergistic role in inducing ATF3 protein expression and promoting tumor cell death (O'Brien et al., 2012). Adding DSF to the combination regimen of cisplatin and vincristine is well tolerated in antitumor therapy (Nechushtan et al., 2015). A dose of 40 mg DSF, with a half-life of approximately 7 hours, can be administered three times daily to improve its therapeutic effect with minimal side effects (Nechushtan et al., 2015). Significant neurotoxicity has not been reported with this combination therapy, besides fatigue (Nechushtan et al., 2015).
DSF may assist cisplatin in enhancing cytotoxic effects. This strategy appears to be safe, but the in vivo efficacy in glioma patients remains unclear.
A study of glioma stem cells in children found that DSF killed glioma stem cells at low concentrations and killed cell lines at slightly higher concentrations (Meier et al., 2021). The addition of auranofin increased DSF efficiency, and the synergistic effect was more pronounced in differentiated cells than in undifferentiated cells (Meier et al., 2021). Auranofin was also used in combination with DSF in the CUSP9 treatment program (Skaga et al., 2019).
DSF with aprepitant, auranofin, captopril, celecoxib, itraconazole, minocycline, quetiapine, and sertraline constituted a CUSP9 regimen that was used in combination with TMZ to synergistically disrupt the active survival pathway in GBM, block multiple signaling pathways, and make GBM vulnerable to the cytotoxic effects of TMZ (Skaga et al., 2019). A study of patient-derived glioblastoma stem cell (GSC) cultures from 15 patients with GBM showed that combining CUSP9 with TMZ produced a synergistic effect compared to the single drug (Skaga et al., 2019). CUSP9, combined with TMZ, was more effective than TMZ monotherapy in terms of the clinical plasma concentrations (Skaga et al., 2019). The CUSP9* regimen was generated based on the CUSP9 regimen, comprising DSF in combination with aprepitant, artesunate, auranofin, captopril, celecoxib, itraconazole, sertraline, and ritonavir (Kast et al., 2014). All nine drugs in the CUSP9* regimen were FDA-approved, each inhibiting one or more of the important GBM growth pathways (Kast et al., 2014).
DSF and auranofin have synergistic effect even without the other components of a CUSP9 regimen. All these might be the powerful adjuncts to TMZ chemotherapy. However, the side effects of CUSP9 or CUSP9* regimens combined with multiple agents deserve further clinical study.
Gemcitabine (2,2′-difluorodeoxycytidine, dFdC) is a deoxyribonucleic acid analogue that can be used as a single agent or combined with other anticancer drugs (Metro et al., 2010). Gemcitabine is active against a wide range of hematological and solid cancers. It is one of the few classical anticancer drugs that can pass through the BBB, penetrate the tumor mass, and be effectively converted to its active form in GBM tissue (Metro et al., 2010). However, its use in GBM chemotherapy is limited by the high resistance of GBM cells to dFdCs (Liu et al., 2012). Therefore, it is currently used mainly in combination with radiotherapy as a radiosensitizer for GBM treatment (Liu et al., 2012). DSF can synergistically enhance gemcitabine cytotoxicity and reverse gemcitabine resistance in cancer cell lines through ROS induction and inhibition of the ALDH and NF-κB pathways (Liu et al., 2012; O'Brien et al., 2012). In preclinical studies, DSF and its derivative pyrrolidine dithiocarbamate have been successfully used to enhance gemcitabine efficacy, particularly through NF-κB inhibition and oxidative stress generation (Iljin et al., 2009; Tesson et al., 2017). Increased oxidative stress due to DSF/Cu interactions with glutathione sensitizes cancer cells to gemcitabine treatment (Iljin et al., 2009; Tesson et al., 2017).
Carbenoxolone is mainly used to treat gastric ulcers and other types of inflammation (Connors, 2012) and can play a role in inhibiting tumor cell growth by interfering with intracellular signaling through inhibiting ligand proteins (Zhang et al., 2003). DSF and carbenoxolone inhibit distinct interactions of GBM with the brain tissue microenvironment and stress-induced GBM cell-matrix adhesion with gap junction-mediated intercellular communication (Mettang et al., 2018). Animal experiments have shown that the combined use of DSF, carbenoxolone, and TMZ reduces tumor size in an in situ mouse model (Mettang et al., 2018). Tumor-initiating and adherent differentiated cells form gap junctions, and carbenoxolone can block adherent differentiated cells and affect intercellular communication (Mettang et al., 2018). Adherent differentiated cells are more sensitive to DSF treatment, and DSF interferes with cell-matrix adhesion by modulating NF-κB signaling (Mettang et al., 2018).
Table 2 shows the candidate chemicals used in combination with DSF for cancer treatment.
DSF has a radiosensitizing effect on GBM cells (Koh et al., 2019) and enhances the radiosensitivity of AT/RT cell lines by increasing DNA damage, apoptosis, and autophagy (Lee et al., 2017). Combining DSF and Cu enhanced radiosensitivity by inducing cell death or interfering with DNA repair (Liu C. C. et al., 2021).
Thus, the efficacy of both chemotherapy and radiotherapy might be enhanced by DSF.
DSF is a safe and well-tolerated drug, with mild side effects (Shirley et al., 2021). With chronic lymphocytic leukemia and normal lymphocytes (Wickström et al., 2007), invasive cancer and normal endothelial cells (Shian et al., 2003), and glioblastoma and normal astrocytes (Hothi et al., 2012), DSF is selectively toxic and kills human cancer cells (Paranjpe et al., 2014). The ability of different organs to resist endogenous and environmentally derived alkylating agents may be compromised, and this unrepaired DNA damage, particularly in regulatory oncogenes, may manifest as deleterious mutations and promote genomic instability (Paranjpe et al., 2014). DSF combined with Cu has an enhanced role in killing cancer cells; however, Cu-mediated cytotoxicity is also significantly increased in normal cells (Choi et al., 2015). The combined use of Cu and Zn in therapy is potentially dangerous because they are teratogenic and may lead to developmental defects (Choi et al., 2015). High DSF doses are hepatotoxic (Triscott et al., 2015), and rare cases of severe atopic hepatitis may occur, along with a risk of neuropathy; however, these symptoms are reversible after discontinuation (Huang J. et al., 2016). The mechanisms of neurological side effects of DSF remain unclear and may involve free acid radicals, inhibition of certain enzymes, calcium-induced neuronal toxicity, synergistic activity of neurotoxic drugs/chemicals, and other mechanisms (Kulkarni et al., 2013). If ethanol is ingested during DSF treatment, the large amounts of acetaldehyde produced from ethanol can cause severe nausea, headache, vomiting, flushing, and physical discomfort (Kast and Belda-Iniesta, 2009).
DSF is considered a relatively safe treatment since most of its adverse effects resolve after discontinuation. The mechanism of neuropathy caused by DSF still requires further research.
Malignant gliomas have a poor prognosis and high recurrence rate, posing a major threat to global public health. Current conventional treatment modalities hardly eradicate gliomas; therefore, new therapeutic approaches are urgently needed. Drug repurposing approaches have provided new research ideas for glioma treatment. This can help the pharmaceutical industry and researchers identify new uses for existing drugs. The expanding research on gliomas through drug repurposing approaches has made DSF a potential adjuvant for glioma treatment. DSF has a good safety profile and is an economical drug that is expected to play a broader role in the future treatment of gliomas. Further epidemiological studies should be performed to investigate the relationship between DSF and the risk to or survival of patients with gliomas. More clinical trials are needed to further refine treatment options for DSF. However, drug repurposing may not yet reach its full potential in the field of glioma, and new therapeutic agents through drug repurposing deserve further exploration.
Conceptualization, YB and SZ; methodology, SZ; critical revision of the manuscript for important intellectual content, YB, YW, and SL; analysis and interpretation of data, XZ and SL; investigation, XS, GL, KL, XZ, LL, LR, ST, QZ, BZ, WS, ZY, and QX; reference collection and data acquisition, SZ and SL; original draft preparation, SZ; review and editing, SZ, YB, and YW; visualization, SZ; supervision, YB and YW; project administration, SZ, SL, YB, and YW; funding acquisition, YB and XS. All authors have read and agreed to the published version of the manuscript.
This study was funded by Liaoning Provincial Natural Science Foundation (2020-MS-155), China Medical University novel coronavirus pneumonia prevention and control research project (2020-12-11), Shenyang Planning Foundation for Science and Technology (21-173-9-38), the first batch of medical education scientific research project of China Medical University for the 14th Five-Year Plan (YDJK2021011), the Scientific Research Funding Project of Education Department of Liaoning Province (JCZR2020010), National Science Foundation of China (72074104), Immersion Technology and Evaluation Shandong Engineering Research Center (2022), and Immersion Technology and Evaluation Shandong Engineering Research Center (2022). The researchers are grateful for the support of several organizations.
The authors declare that the research was conducted in the absence of any commercial or financial relationships that could be construed as a potential conflict of interest.
All claims expressed in this article are solely those of the authors and do not necessarily represent those of their affiliated organizations, or those of the publisher, the editors, and the reviewers. Any product that may be evaluated in this article, or claim that may be made by its manufacturer, is not guaranteed or endorsed by the publisher.
Abidin, I. Z., Rezoagli, E., Simonassi-Paiva, B., Fehrenbach, G. W., Masterson, K., Pogue, R., et al. (2020). A bilayer vaginal tablet for the localized delivery of disulfiram and 5-fluorouracil to the cervix. Pharmaceutics 12, E1185. doi:10.3390/pharmaceutics12121185
Adeberg, S., Bernhardt, D., Ben Harrabi, S., Bostel, T., Mohr, A., Koelsche, C., et al. (2015). Metformin influences progression in diabetic glioblastoma patients. Strahlenther. Onkol. 191, 928–935. doi:10.1007/s00066-015-0884-5
Anjum, K., Shagufta, B. I., Abbas, S. Q., Patel, S., Khan, I., Shah, S. A. A., et al. (2017). Current status and future therapeutic perspectives of glioblastoma multiforme (GBM) therapy: A review. Biomed. Pharmacother. 92, 681–689. doi:10.1016/j.biopha.2017.05.125
Arora, N., Kacker, P. K. M., and Kacker, P. (2017). Translational research in drug discovery and development. Translational Bioinformatics and its Application.
Ashburn, T. T., and Thor, K. B. (2004). Drug repositioning: Identifying and developing new uses for existing drugs. Nat. Rev. Drug Discov. 3, 673–683. doi:10.1038/nrd1468
Bai, M. Y., Yu, M. H., Wang, T. T., Chen, S. H., and Wang, Y. C. (2021). Plate-like alginate microparticles with disulfiram-SPIO-coencapsulation: An in vivo study for combined therapy on ovarian cancer. Pharmaceutics 13, 1348. doi:10.3390/pharmaceutics13091348
Barrett, T., Wilhite, S. E., Ledoux, P., Evangelista, C., Kim, I. F., Tomashevsky, M., et al. (2013). NCBI GEO: Archive for functional genomics data sets--update. Nucleic Acids Res. 41, D991–D995. doi:10.1093/nar/gks1193
Brown, A. S., and Patel, C. J. (2017b). A standard database for drug repositioning. Sci. Data 4, 170029. doi:10.1038/sdata.2017.29
Brown, A. S., and Patel, C. J. (2017a). MeSHDD: Literature-based drug-drug similarity for drug repositioning. J. Am. Med. Inf. Assoc. 24, 614–618. doi:10.1093/jamia/ocw142
Budman, D. R., and Calabro, A. (2002). In vitro search for synergy and antagonism: Evaluation of docetaxel combinations in breast cancer cell lines. Breast Cancer Res. Treat. 74, 41–46. doi:10.1023/a:1016070230538
Cha, Y., Erez, T., Reynolds, I. J., Kumar, D., Ross, J., Koytiger, G., et al. (2018). Drug repurposing from the perspective of pharmaceutical companies. Br. J. Pharmacol. 175, 168–180. doi:10.1111/bph.13798
Chen, D., Cui, Q. C., Yang, H., and Dou, Q. P. (2006). Disulfiram, a clinically used anti-alcoholism drug and copper-binding agent, induces apoptotic cell death in breast cancer cultures and xenografts via inhibition of the proteasome activity. Cancer Res. 66, 10425–10433. doi:10.1158/0008-5472.Can-06-2126
Chick, J. (1999). Safety issues concerning the use of disulfiram in treating alcohol dependence. Drug Saf. 20, 427–435. doi:10.2165/00002018-199920050-00003
Choi, S. A., Choi, J. W., Wang, K. C., Phi, J. H., Lee, J. Y., Park, K. D., et al. (2015). Disulfiram modulates stemness and metabolism of brain tumor initiating cells in atypical teratoid/rhabdoid tumors. Neuro. Oncol. 17, 810–821. doi:10.1093/neuonc/nou305
Cong, J., Wang, Y., Zhang, X., Zhang, N., Liu, L., Soukup, K., et al. (2017). A novel chemoradiation targeting stem and nonstem pancreatic cancer cells by repurposing disulfiram. Cancer Lett. 409, 9–19. doi:10.1016/j.canlet.2017.08.028
Connors, B. W. (2012). Tales of a dirty drug: Carbenoxolone, gap junctions, and seizures. Epilepsy Curr. 12, 66–68. doi:10.5698/1535-7511-12.2.66
Conticello, C., Martinetti, D., Adamo, L., Buccheri, S., Giuffrida, R., Parrinello, N., et al. (2012). Disulfiram, an old drug with new potential therapeutic uses for human hematological malignancies. Int. J. Cancer 131, 2197–2203. doi:10.1002/ijc.27482
Corsello, S. M., Bittker, J. A., Liu, Z., Gould, J., Mccarren, P., Hirschman, J. E., et al. (2017). The drug repurposing hub: A next-generation drug library and information resource. Nat. Med. 23, 405–408. doi:10.1038/nm.4306
Cotto, K. C., Wagner, A. H., Feng, Y. Y., Kiwala, S., Coffman, A. C., Spies, G., et al. (2018). DGIdb 3.0: A redesign and expansion of the drug-gene interaction database. Nucleic Acids Res. 46, D1068-D1073–d1073. doi:10.1093/nar/gkx1143
Cunningham, J. A., and Chaiton, M. (2018). Has there been an increase in the frequency with which people who drink in a risky fashion receive advice to cut down on their drinking from 1998 to 2015? Subst. Abus. 39, 449–451. doi:10.1080/08897077.2018.1449048
Cvek, B. (2012). Nonprofit drugs as the salvation of the world's healthcare systems: The case of antabuse (disulfiram). Drug Discov. Today 17, 409–412. doi:10.1016/j.drudis.2011.12.010
Dimayuga, F. O., Wang, C., Clark, J. M., Dimayuga, E. R., Dimayuga, V. M., and Bruce-Keller, A. J. (2007). SOD1 overexpression alters ROS production and reduces neurotoxic inflammatory signaling in microglial cells. J. Neuroimmunol. 182, 89–99. doi:10.1016/j.jneuroim.2006.10.003
Dinnen, R. D., Mao, Y., Qiu, W., Cassai, N., Slavkovich, V. N., Nichols, G., et al. (2013). Redirecting apoptosis to aponecrosis induces selective cytotoxicity to pancreatic cancer cells through increased ROS, decline in ATP levels, and VDAC. Mol. Cancer Ther. 12, 2792–2803. doi:10.1158/1535-7163.Mct-13-0234
Ekinci, E., Rohondia, S., Khan, R., and Dou, Q. P. (2019). Repurposing disulfiram as an anti-cancer agent: Updated review on literature and patents. Recent Pat. anticancer. Drug Discov. 14, 113–132. doi:10.2174/1574892814666190514104035
Emig, D., Ivliev, A., Pustovalova, O., Lancashire, L., Bureeva, S., Nikolsky, Y., et al. (2013). Drug target prediction and repositioning using an integrated network-based approach. PLoS One 8, e60618. doi:10.1371/journal.pone.0060618
Gaulton, A., Bellis, L. J., Bento, A. P., Chambers, J., Davies, M., Hersey, A., et al. (2012). ChEMBL: A large-scale bioactivity database for drug discovery. Nucleic Acids Res. 40, D1100–D1107. doi:10.1093/nar/gkr777
Gerson, S. L. (2002). Clinical relevance of MGMT in the treatment of cancer. J. Clin. Oncol. 20, 2388–2399. doi:10.1200/jco.2002.06.110
Ghosh, D., Nandi, S., and Bhattacharjee, S. (2018). Combination therapy to checkmate glioblastoma: Clinical challenges and advances. Clin. Transl. Med. 7, 33. doi:10.1186/s40169-018-0211-8
Gottlieb, A., Stein, G. Y., Ruppin, E., and Sharan, R. (2011). Predict: A method for inferring novel drug indications with application to personalized medicine. Mol. Syst. Biol. 7, 496. doi:10.1038/msb.2011.26
Guo, X., Xu, B., Pandey, S., Goessl, E., Brown, J., Armesilla, A. L., et al. (2010). Disulfiram/copper complex inhibiting NFkappaB activity and potentiating cytotoxic effect of gemcitabine on colon and breast cancer cell lines. Cancer Lett. 290, 104–113. doi:10.1016/j.canlet.2009.09.002
Halatsch, M. E., Kast, R. E., Karpel-Massler, G., Mayer, B., Zolk, O., Schmitz, B., et al. (2021). A phase ib/IIa trial of 9 repurposed drugs combined with temozolomide for the treatment of recurrent glioblastoma: CUSP9v3. Neurooncol. Adv. 3, vdab075. vdab075. doi:10.1093/noajnl/vdab075
Han, D., Wu, G., Chang, C., Zhu, F., Xiao, Y., Li, Q., et al. (2015). Disulfiram inhibits TGF-β-induced epithelial-mesenchymal transition and stem-like features in breast cancer via ERK/NF-κB/Snail pathway. Oncotarget 6, 40907–40919. doi:10.18632/oncotarget.5723
Hothi, P., Martins, T. J., Chen, L., Deleyrolle, L., Yoon, J. G., Reynolds, B., et al. (2012). High-throughput chemical screens identify disulfiram as an inhibitor of human glioblastoma stem cells. Oncotarget 3, 1124–1136. doi:10.18632/oncotarget.707
Huang, H., Liao, Y., Liu, N., Hua, X., Cai, J., Yang, C., et al. (2016a). Two clinical drugs deubiquitinase inhibitor auranofin and aldehyde dehydrogenase inhibitor disulfiram trigger synergistic anti-tumor effects in vitro and in vivo. Oncotarget 7, 2796–2808. doi:10.18632/oncotarget.6425
Huang, H., Nguyen, T., Ibrahim, S., Shantharam, S., Yue, Z., and Chen, J. Y. (2015). Dmap: A connectivity map database to enable identification of novel drug repositioning candidates. BMC Bioinforma. 16 (13), S4. doi:10.1186/1471-2105-16-s13-s4
Huang, J., Campian, J. L., Gujar, A. D., Tran, D. D., Lockhart, A. C., Dewees, T. A., et al. (2016b). A phase I study to repurpose disulfiram in combination with temozolomide to treat newly diagnosed glioblastoma after chemoradiotherapy. J. Neurooncol. 128, 259–266. doi:10.1007/s11060-016-2104-2
Huang, J., Campian, J. L., Gujar, A. D., Tsien, C., Ansstas, G., Tran, D. D., et al. (2018). Final results of a phase I dose-escalation, dose-expansion study of adding disulfiram with or without copper to adjuvant temozolomide for newly diagnosed glioblastoma. J. Neurooncol. 138, 105–111. doi:10.1007/s11060-018-2775-y
Huang, J., Chaudhary, R., Cohen, A. L., Fink, K., Goldlust, S., Boockvar, J., et al. (2019). A multicenter phase II study of temozolomide plus disulfiram and copper for recurrent temozolomide-resistant glioblastoma. J. Neurooncol. 142, 537–544. doi:10.1007/s11060-019-03125-y
Iljin, K., Ketola, K., Vainio, P., Halonen, P., Kohonen, P., Fey, V., et al. (2009). High-throughput cell-based screening of 4910 known drugs and drug-like small molecules identifies disulfiram as an inhibitor of prostate cancer cell growth. Clin. Cancer Res. 15, 6070–6078. doi:10.1158/1078-0432.Ccr-09-1035
Jakola, A. S., Werlenius, K., Mudaisi, M., Hylin, S., Kinhult, S., Bartek, J., et al. (2018). Disulfiram repurposing combined with nutritional copper supplement as add-on to chemotherapy in recurrent glioblastoma (DIRECT): Study protocol for a randomized controlled trial. F1000Res. 7, 1797. doi:10.12688/f1000research.16786.1
Jangra, A., Choi, S. A., Yang, J., Koh, E. J., Phi, J. H., Lee, J. Y., et al. (2020). Disulfiram potentiates the anticancer effect of cisplatin in atypical teratoid/rhabdoid tumors (AT/RT). Cancer Lett. 486, 38–45. doi:10.1016/j.canlet.2020.05.006
Jivan, R., Peres, J., Damelin, L. H., Wadee, R., Veale, R. B., Prince, S., et al. (2018). Disulfiram with or without metformin inhibits oesophageal squamous cell carcinoma in vivo. Cancer Lett. 417, 1–10. doi:10.1016/j.canlet.2017.12.026
Jørgensen, C. H., Pedersen, B., and TøNNESEN, H. (2011). The efficacy of disulfiram for the treatment of alcohol use disorder. Alcohol. Clin. Exp. Res. 35, 1749–1758. doi:10.1111/j.1530-0277.2011.01523.x
Juárez-López, D., and Schcolnik-Cabrera, A. (2021). Drug repurposing: Considerations to surpass while Re-directing old compounds for new treatments. Arch. Med. Res. 52, 243–251. doi:10.1016/j.arcmed.2020.10.021
Kanehisa, M., Furumichi, M., Tanabe, M., Sato, Y., and Morishima, K. (2017). Kegg: New perspectives on genomes, pathways, diseases and drugs. Nucleic Acids Res. 45, D353-D361–d361. doi:10.1093/nar/gkw1092
Karamanakos, P. N., Trafalis, D. T., Papachristou, D. J., Panteli, E. S., Papavasilopoulou, M., Karatzas, A., et al. (2017). Evidence for the efficacy of disulfiram and copper combination in glioblastoma multiforme - a propos of a case. J. buon 22, 1227–1232.
Kast, R. E., and Belda-Iniesta, C. (2009). Suppressing glioblastoma stem cell function by aldehyde dehydrogenase inhibition with chloramphenicol or disulfiram as a new treatment adjunct: An hypothesis. Curr. Stem Cell. Res. Ther. 4, 314–317. doi:10.2174/157488809789649241
Kast, R. E., and Halatsch, M. E. (2012). Matrix metalloproteinase-2 and -9 in glioblastoma: A trio of old drugs-captopril, disulfiram and nelfinavir-are inhibitors with potential as adjunctive treatments in glioblastoma. Arch. Med. Res. 43, 243–247. doi:10.1016/j.arcmed.2012.04.005
Kast, R. E., Karpel-Massler, G., and Halatsch, M. E. (2014). CUSP9* treatment protocol for recurrent glioblastoma: Aprepitant, artesunate, auranofin, captopril, celecoxib, disulfiram, itraconazole, ritonavir, sertraline augmenting continuous low dose temozolomide. Oncotarget 5, 8052–8082. doi:10.18632/oncotarget.2408
Kim, S. K., Kim, H., Lee, D. H., Kim, T. S., Kim, T., Chung, C., et al. (2013). Reversing the intractable nature of pancreatic cancer by selectively targeting ALDH-high, therapy-resistant cancer cells. PLoS One 8, e78130. doi:10.1371/journal.pone.0078130
Kita, Y., Hamada, A., Saito, R., Teramoto, Y., Tanaka, R., Takano, K., et al. (2019). Systematic chemical screening identifies disulfiram as a repurposed drug that enhances sensitivity to cisplatin in bladder cancer: A summary of preclinical studies. Br. J. Cancer 121, 1027–1038. doi:10.1038/s41416-019-0609-0
Koh, H. K., Seo, S. Y., Kim, J. H., Kim, H. J., Chie, E. K., Kim, S. K., et al. (2019). Disulfiram, a Re-positioned aldehyde dehydrogenase inhibitor, enhances radiosensitivity of human glioblastoma cells in vitro. Cancer Res. Treat. 51, 696–705. doi:10.4143/crt.2018.249
Koleti, A., Terryn, R., Stathias, V., Chung, C., Cooper, D. J., Turner, J. P., et al. (2018). Data portal for the library of integrated network-based cellular signatures (LINCS) program: Integrated access to diverse large-scale cellular perturbation response data. Nucleic Acids Res. 46, D558-D566–d566. doi:10.1093/nar/gkx1063
Kuhn, M., Szklarczyk, D., Franceschini, A., von Mering, C., Jensen, L. J., and Bork, P. (2012). Stitch 3: Zooming in on protein-chemical interactions. Nucleic Acids Res. 40, D876–D880. doi:10.1093/nar/gkr1011
Kulkarni, R. R., Pradeep, A. V., and Bairy, B. K. (2013). Disulfiram-induced combined irreversible anterior ischemic optic neuropathy and reversible peripheral neuropathy: A prospective case report and review of the literature. J. Neuropsychiatry Clin. Neurosci. 25, 339–342. doi:10.1176/appi.neuropsych.12120410
Kumar, R., Harilal, S., Gupta, S. V., Jose, J., Thomas Parambi, D. G., Uddin, M. S., et al. (2019). Exploring the new horizons of drug repurposing: A vital tool for turning hard work into smart work. Eur. J. Med. Chem. 182, 111602. doi:10.1016/j.ejmech.2019.111602
Lamb, J., Crawford, E. D., Peck, D., Modell, J. W., Blat, I. C., Wrobel, M. J., et al. (2006). The connectivity map: Using gene-expression signatures to connect small molecules, genes, and disease. Science 313, 1929–1935. doi:10.1126/science.1132939
Lan, Q. H., du, C. C., Yu, R. J., Zhai, J., Shi, Y., Kou, L., et al. (2021). Disulfiram-loaded copper sulfide nanoparticles for potential anti-glioma therapy. Int. J. Pharm. 607, 120978. doi:10.1016/j.ijpharm.2021.120978
Landis, M. S., Boyden, T., and Pegg, S. (2012). Nasal-to-CNS drug delivery: Where are we now and where are we heading? An industrial perspective. Ther. Deliv. 3, 195–208. doi:10.4155/tde.11.149
Langedijk, J., Mantel-Teeuwisse, A. K., Slijkerman, D. S., and Schutjens, M. H. (2015). Drug repositioning and repurposing: Terminology and definitions in literature. Drug Discov. Today 20, 1027–1034. doi:10.1016/j.drudis.2015.05.001
Lee, S. Y. (2016). Temozolomide resistance in glioblastoma multiforme. Genes. Dis. 3, 198–210. doi:10.1016/j.gendis.2016.04.007
Lee, Y. E., Choi, S. A., Kwack, P. A., Kim, H. J., Kim, I. H., Wang, K. C., et al. (2017). Repositioning disulfiram as a radiosensitizer against atypical teratoid/rhabdoid tumor. Neuro. Oncol. 19, 1079–1087. doi:10.1093/neuonc/now300
Li, Y., Chen, F., Chen, J., Chan, S., He, Y., Liu, W., et al. (2020). Disulfiram/copper induces antitumor activity against both nasopharyngeal cancer cells and cancer-associated fibroblasts through ROS/MAPK and ferroptosis pathways. Cancers (Basel) 12, E138. doi:10.3390/cancers12010138
Lipsky, J. J., Shen, M. L., and Naylor, S. (2001a). In vivo inhibition of aldehyde dehydrogenase by disulfiram. Chem. Biol. Interact. 130-132, 93–102. doi:10.1016/s0009-2797(00)00225-8
Lipsky, J. J., Shen, M. L., and Naylor, S. (2001b). Overview--in vitro inhibition of aldehyde dehydrogenase by disulfiram and metabolites. Chem. Biol. Interact. 130-132, 81–91. doi:10.1016/s0009-2797(00)00224-6
Liu, C. C., Wu, C. L., Lin, M. X., Sze, C. I., and Gean, P. W. (2021b). Disulfiram sensitizes a therapeutic-resistant glioblastoma to the TGF-β receptor inhibitor. Int. J. Mol. Sci. 22, 10496. doi:10.3390/ijms221910496
Liu, C., Qiang, J., Deng, Q., Xia, J., Deng, L., Zhou, L., et al. (2021a). ALDH1A1 activity in tumor-initiating cells remodels myeloid-derived suppressor cells to promote breast cancer progression. Cancer Res. 81, 5919–5934. doi:10.1158/0008-5472.Can-21-1337
Liu, P., Brown, S., Goktug, T., Channathodiyil, P., Kannappan, V., Hugnot, J. P., et al. (2012). Cytotoxic effect of disulfiram/copper on human glioblastoma cell lines and ALDH-positive cancer-stem-like cells. Br. J. Cancer 107, 1488–1497. doi:10.1038/bjc.2012.442
Liu, P., Kumar, I. S., Brown, S., Kannappan, V., Tawari, P. E., Tang, J. Z., et al. (2013). Disulfiram targets cancer stem-like cells and reverses resistance and cross-resistance in acquired paclitaxel-resistant triple-negative breast cancer cells. Br. J. Cancer 109, 1876–1885. doi:10.1038/bjc.2013.534
Louis, D. N., Perry, A., Reifenberger, G., von Deimling, A., Figarella-Branger, D., Cavenee, W. K., et al. (2016). The 2016 world health organization classification of tumors of the central nervous system: A summary. Acta Neuropathol. 131, 803–820. doi:10.1007/s00401-016-1545-1
Lun, X., Wells, J. C., Grinshtein, N., King, J. C., Hao, X., Dang, N. H., et al. (2016). Disulfiram when combined with copper enhances the therapeutic effects of temozolomide for the treatment of glioblastoma. Clin. Cancer Res. 22, 3860–3875. doi:10.1158/1078-0432.Ccr-15-1798
Mandell, J. B., Douglas, N., Ukani, V., Beumer, J. H., Guo, J., Payne, J., et al. (2022). ALDH1A1 gene expression and cellular copper levels between low and highly metastatic osteosarcoma provide a case for novel repurposing with disulfiram and copper. Sarcoma 2022, 7157507. doi:10.1155/2022/7157507
Masoudi-Sobhanzadeh, Y., Omidi, Y., Amanlou, M., and Masoudi-Nejad, A. (2019). DrugR+: A comprehensive relational database for drug repurposing, combination therapy, and replacement therapy. Comput. Biol. Med. 109, 254–262. doi:10.1016/j.compbiomed.2019.05.006
Mcmahon, A., Chen, W., and Li, F. (2020). Old wine in new bottles: Advanced drug delivery systems for disulfiram-based cancer therapy. J. Control. Release 319, 352–359. doi:10.1016/j.jconrel.2020.01.001
Meier, S., Cantilena, S., Niklison Chirou, M. V., Anderson, J., Hargrave, D., Salomoni, P., et al. (2021). Alcohol-abuse drug disulfiram targets pediatric glioma via MLL degradation. Cell. Death Dis. 12, 785. doi:10.1038/s41419-021-04078-9
Metro, G., Fabi, A., Mirri, M. A., Vidiri, A., Pace, A., Carosi, M., et al. (2010). Phase II study of fixed dose rate gemcitabine as radiosensitizer for newly diagnosed glioblastoma multiforme. Cancer Chemother. Pharmacol. 65, 391–397. doi:10.1007/s00280-009-1155-x
Mettang, M., Meyer-Pannwitt, V., Karpel-Massler, G., Zhou, S., Carragher, N. O., FöHR, K. J., et al. (2018). Blocking distinct interactions between glioblastoma cells and their tissue microenvironment: A novel multi-targeted therapeutic approach. Sci. Rep. 8, 5527. doi:10.1038/s41598-018-23592-z
Mohammad, I. S., Teng, C., Chaurasiya, B., Yin, L., Wu, C., and He, W. (2019). Drug-delivering-drug approach-based codelivery of paclitaxel and disulfiram for treating multidrug-resistant cancer. Int. J. Pharm. 557, 304–313. doi:10.1016/j.ijpharm.2018.12.067
Moosavinasab, S., Patterson, J., Strouse, R., Rastegar-Mojarad, M., Regan, K., Payne, P. R., et al. (2016). 'RE:fine drugs': An interactive dashboard to access drug repurposing opportunities. Oxford): Database. doi:10.1093/database/baw083
Moreb, J. S., Ucar, D., Han, S., Amory, J. K., Goldstein, A. S., Ostmark, B., et al. (2012). The enzymatic activity of human aldehyde dehydrogenases 1A2 and 2 (ALDH1A2 and ALDH2) is detected by Aldefluor, inhibited by diethylaminobenzaldehyde and has significant effects on cell proliferation and drug resistance. Chem. Biol. Interact. 195, 52–60. doi:10.1016/j.cbi.2011.10.007
Mutter, N., and Stupp, R. (2006). Temozolomide: A milestone in neuro-oncology and beyond? Expert Rev. Anticancer Ther. 6, 1187–1204. doi:10.1586/14737140.6.8.1187
Nechushtan, H., Hamamreh, Y., Nidal, S., Gotfried, M., Baron, A., Shalev, Y. I., et al. (2015). A phase IIb trial assessing the addition of disulfiram to chemotherapy for the treatment of metastatic non-small cell lung cancer. Oncologist 20, 366–367. doi:10.1634/theoncologist.2014-0424
Newlands, E. S., Blackledge, G., Slack, J. A., Goddard, C., Brindley, C. J., Holden, L., et al. (1985). Phase I clinical trial of mitozolomide. Cancer Treat. Rep. 69, 801–805.
Newlands, E. S., Stevens, M. F., Wedge, S. R., Wheelhouse, R. T., and Brock, C. (1997). Temozolomide: A review of its discovery, chemical properties, pre-clinical development and clinical trials. Cancer Treat. Rev. 23, 35–61. doi:10.1016/s0305-7372(97)90019-0
O'brien, A., Barber, J. E., Reid, S., Niknejad, N., and Dimitroulakos, J. (2012). Enhancement of cisplatin cytotoxicity by disulfiram involves activating transcription factor 3. Anticancer Res. 32, 2679–2688.
Ostrom, Q. T., Cioffi, G., Gittleman, H., Patil, N., Waite, K., Kruchko, C., et al. (2019). CBTRUS statistical report: Primary brain and other central nervous system tumors diagnosed in the United States in 2012-2016. Neuro. Oncol. 21, v1–v100. doi:10.1093/neuonc/noz150
Osuka, S., and Van Meir, E. G. (2017). Overcoming therapeutic resistance in glioblastoma: The way forward. J. Clin. Investig. 127, 415–426. doi:10.1172/jci89587
Özcan, H., Emiroğlu, B. G., Sabuncuoğlu, H., Özdoğan, S., Soyer, A., and SAYGı, T. (2021). A comparative study for glioma classification using deep convolutional neural networks. Math. Biosci. Eng. 18, 1550–1572. doi:10.3934/mbe.2021080
Papaioannou, M., Mylonas, I., Kast, R. E., and BRüNING, A. (2014). Disulfiram/copper causes redox-related proteotoxicity and concomitant heat shock response in ovarian cancer cells that is augmented by auranofin-mediated thioredoxin inhibition. Oncoscience 1, 21–29. doi:10.18632/oncoscience.5
Paranjpe, A., Zhang, R., Ali-Osman, F., Bobustuc, G. C., and Srivenugopal, K. S. (2014). Disulfiram is a direct and potent inhibitor of human O6-methylguanine-DNA methyltransferase (MGMT) in brain tumor cells and mouse brain and markedly increases the alkylating DNA damage. Carcinogenesis 35, 692–702. doi:10.1093/carcin/bgt366
Parsons, D. W., Li, M., Zhang, X., Jones, S., Leary, R. J., Lin, J. C., et al. (2011). The genetic landscape of the childhood cancer medulloblastoma. Science 331, 435–439. doi:10.1126/science.1198056
Parvathaneni, V., Kulkarni, N. S., Muth, A., and Gupta, V. (2019). Drug repurposing: A promising tool to accelerate the drug discovery process. Drug Discov. Today 24, 2076–2085. doi:10.1016/j.drudis.2019.06.014
Pujol, A., Mosca, R., FARRéS, J., and Aloy, P. (2010). Unveiling the role of network and systems biology in drug discovery. Trends Pharmacol. Sci. 31, 115–123. doi:10.1016/j.tips.2009.11.006
Qu, Y., Sun, X., Ma, L., Li, C., Xu, Z., Ma, W., et al. (2021). Therapeutic effect of disulfiram inclusion complex embedded in hydroxypropyl-β-cyclodextrin on intracranial glioma-bearing male rats via intranasal route. Eur. J. Pharm. Sci. 156, 105590. doi:10.1016/j.ejps.2020.105590
Rappa, F., Cappello, F., Halatsch, M. E., Scheuerle, A., and Kast, R. E. (2013). Aldehyde dehydrogenase and HSP90 co-localize in human glioblastoma biopsy cells. Biochimie 95, 782–786. doi:10.1016/j.biochi.2012.11.007
Rolle, F., Bincoletto, V., Gazzano, E., Rolando, B., Lollo, G., Stella, B., et al. (2020). Coencapsulation of disulfiram and doxorubicin in liposomes strongly reverses multidrug resistance in breast cancer cells. Int. J. Pharm. 580, 119191. doi:10.1016/j.ijpharm.2020.119191
Sam, E., and Athri, P. (2019). Web-based drug repurposing tools: A survey. Brief. Bioinform. 20, 299–316. doi:10.1093/bib/bbx125
Schmidtova, S., Kalavska, K., Gercakova, K., Cierna, Z., Miklikova, S., Smolkova, B., et al. (2019). Disulfiram Overcomes Cisplatin Resistance in Human Embryonal Carcinoma Cells, 11. doi:10.3390/cancers11091224Cancers (Basel)
Schuettengruber, B., Bourbon, H. M., di Croce, L., and Cavalli, G. (2017). Genome regulation by polycomb and Trithorax: 70 Years and counting. Cell. 171, 34–57. doi:10.1016/j.cell.2017.08.002
Seliger, C., Genbrugge, E., Gorlia, T., Chinot, O., Stupp, R., Nabors, B., et al. (2020). Use of metformin and outcome of patients with newly diagnosed glioblastoma: Pooled analysis. Int. J. Cancer 146, 803–809. doi:10.1002/ijc.32337
Seliger, C., and Hau, P. (2018). Drug repurposing of metabolic agents in malignant glioma. Int. J. Mol. Sci. 19, E2768. doi:10.3390/ijms19092768
Shameer, K., Glicksberg, B. S., Hodos, R., Johnson, K. W., Badgeley, M. A., Readhead, B., et al. (2018). Systematic analyses of drugs and disease indications in RepurposeDB reveal pharmacological, biological and epidemiological factors influencing drug repositioning. Brief. Bioinform. 19, 656–678. doi:10.1093/bib/bbw136
Shergill, M., Patel, M., Khan, S., Bashir, A., and Mcconville, C. (2016). Development and characterisation of sustained release solid dispersion oral tablets containing the poorly water soluble drug disulfiram. Int. J. Pharm. 497, 3–11. doi:10.1016/j.ijpharm.2015.11.029
Shian, S. G., Kao, Y. R., Wu, F. Y., and Wu, C. W. (2003). Inhibition of invasion and angiogenesis by zinc-chelating agent disulfiram. Mol. Pharmacol. 64, 1076–1084. doi:10.1124/mol.64.5.1076
Shirley, D. A., Sharma, I., Warren, C. A., and Moonah, S. (2021). Drug repurposing of the alcohol abuse medication disulfiram as an anti-parasitic agent. Front. Cell. Infect. Microbiol. 11, 633194. doi:10.3389/fcimb.2021.633194
Skaga, E., Skaga, I., Grieg, Z., Sandberg, C. J., Langmoen, I. A., and Vik-Mo, E. O. (2019). The efficacy of a coordinated pharmacological blockade in glioblastoma stem cells with nine repurposed drugs using the CUSP9 strategy. J. Cancer Res. Clin. Oncol. 145, 1495–1507. doi:10.1007/s00432-019-02920-4
Spillier, Q., Vertommen, D., Ravez, S., Marteau, R., THéMANS, Q., Corbet, C., et al. (2019). Anti-alcohol abuse drug disulfiram inhibits human PHGDH via disruption of its active tetrameric form through a specific cysteine oxidation. Sci. Rep. 9, 4737. doi:10.1038/s41598-019-41187-0
Srivenugopal, K. S., Rawat, A., Niture, S. K., Paranjpe, A., Velu, C., Venugopal, S. N., et al. (2016). Posttranslational regulation of O(6)-methylguanine-DNA methyltransferase (MGMT) and new opportunities for treatment of brain cancers. Mini Rev. Med. Chem. 16, 455–464. doi:10.2174/1389557515666150722101046
Stockhammer, F., Misch, M., Koch, A., Czabanka, M., Plotkin, M., Blechschmidt, C., et al. (2010). Continuous low-dose temozolomide and celecoxib in recurrent glioblastoma. J. Neurooncol. 100, 407–415. doi:10.1007/s11060-010-0192-y
Stupp, R., Mason, W. P., van Den Bent, M. J., Weller, M., Fisher, B., Taphoorn, M. J., et al. (2005). Radiotherapy plus concomitant and adjuvant temozolomide for glioblastoma. N. Engl. J. Med. 352, 987–996. doi:10.1056/NEJMoa043330
Stylli, S. S. (2020). Novel treatment strategies for glioblastoma. Cancers (Basel) 12, E2883. doi:10.3390/cancers12102883
Swetha, K. L., Sharma, S., Chowdhury, R., and Roy, A. (2020). Disulfiram potentiates docetaxel cytotoxicity in breast cancer cells through enhanced ROS and autophagy. Pharmacol. Rep. 72, 1749–1765. doi:10.1007/s43440-020-00122-1
Tesson, M., Anselmi, G., Bell, C., and Mairs, R. (2017). Cell cycle specific radiosensitisation by the disulfiram and copper complex. Oncotarget 8, 65900–65916. doi:10.18632/oncotarget.19539
Triscott, J., Lee, C., Hu, K., Fotovati, A., Berns, R., Pambid, M., et al. (2012). Disulfiram, a drug widely used to control alcoholism, suppresses the self-renewal of glioblastoma and over-rides resistance to temozolomide. Oncotarget 3, 1112–1123. doi:10.18632/oncotarget.604
Triscott, J., Rose Pambid, M., and Dunn, S. E. (2015). Concise review: Bullseye: Targeting cancer stem cells to improve the treatment of gliomas by repurposing disulfiram. Stem Cells 33, 1042–1046. doi:10.1002/stem.1956
Turanli, B., Altay, O., BORéN, J., Turkez, H., Nielsen, J., Uhlen, M., et al. (2021). Systems biology based drug repositioning for development of cancer therapy. Semin. Cancer Biol. 68, 47–58. doi:10.1016/j.semcancer.2019.09.020
Voorhees, P. M., and Orlowski, R. Z. (2006). The proteasome and proteasome inhibitors in cancer therapy. Annu. Rev. Pharmacol. Toxicol. 46, 189–213. doi:10.1146/annurev.pharmtox.46.120604.141300
Wang, W., Mcleod, H. L., and Cassidy, J. (2003). Disulfiram-mediated inhibition of NF-kappaB activity enhances cytotoxicity of 5-fluorouracil in human colorectal cancer cell lines. Int. J. Cancer 104, 504–511. doi:10.1002/ijc.10972
Wang, Y., Bryant, S. H., Cheng, T., Wang, J., Gindulyte, A., Shoemaker, B. A., et al. (2017). PubChem BioAssay: 2017 update. Nucleic Acids Res. 45, D955-D963–d963. doi:10.1093/nar/gkw1118
Wehbe, M., Anantha, M., Shi, M., Leung, A. W., Dragowska, W. H., Sanche, L., et al. (2017). Development and optimization of an injectable formulation of copper diethyldithiocarbamate, an active anticancer agent. Int. J. Nanomedicine 12, 4129–4146. doi:10.2147/ijn.S137347
Westhoff, M. A., Zhou, S., Nonnenmacher, L., Karpel-Massler, G., Jennewein, C., Schneider, M., et al. (2013). Inhibition of NF-κB signaling ablates the invasive phenotype of glioblastoma. Mol. Cancer Res. 11, 1611–1623. doi:10.1158/1541-7786.Mcr-13-0435-t
Weyerhäuser, P., Kantelhardt, S. R., and Kim, E. L. (2018). Re-Purposing chloroquine for glioblastoma: Potential merits and confounding variables. Front. Oncol. 8, 335. doi:10.3389/fonc.2018.00335
Wickström, M., Danielsson, K., Rickardson, L., Gullbo, J., Nygren, P., Isaksson, A., et al. (2007). Pharmacological profiling of disulfiram using human tumor cell lines and human tumor cells from patients. Biochem. Pharmacol. 73, 25–33. doi:10.1016/j.bcp.2006.08.016
Wishart, D. S., Feunang, Y. D., Guo, A. C., Lo, E. J., Marcu, A., Grant, J. R., et al. (2018). DrugBank 5.0: A major update to the DrugBank database for 2018. Nucleic Acids Res. 46, D1074-D1082–d1082. doi:10.1093/nar/gkx1037
Wu, H., Huang, J., Zhong, Y., and Huang, Q. (2017). DrugSig: A resource for computational drug repositioning utilizing gene expression signatures. PLoS One 12, e0177743. doi:10.1371/journal.pone.0177743
Xu, B., Shi, P., Fombon, I. S., Zhang, Y., Huang, F., Wang, W., et al. (2011). Disulfiram/copper complex activated JNK/c-jun pathway and sensitized cytotoxicity of doxorubicin in doxorubicin resistant leukemia HL60 cells. Blood Cells Mol. Dis. 47, 264–269. doi:10.1016/j.bcmd.2011.08.004
Xue, H., Li, J., Xie, H., and Wang, Y. (2018). Review of drug repositioning approaches and resources. Int. J. Biol. Sci. 14, 1232–1244. doi:10.7150/ijbs.24612
Yang, H., Qin, C., Li, Y. H., Tao, L., Zhou, J., Yu, C. Y., et al. (2016). Therapeutic target database update 2016: Enriched resource for bench to clinical drug target and targeted pathway information. Nucleic Acids Res. 44, D1069–D1074. doi:10.1093/nar/gkv1230
Yang, Z., Guo, F., Albers, A. E., Sehouli, J., and Kaufmann, A. M. (2019). Disulfiram modulates ROS accumulation and overcomes synergistically cisplatin resistance in breast cancer cell lines. Biomed. Pharmacother. 113, 108727. doi:10.1016/j.biopha.2019.108727
Yao, W., Qian, X., Ochsenreither, S., Soldano, F., Deleo, A. B., Sudhoff, H., et al. (2021). Disulfiram acts as a potent radio-chemo sensitizer in head and neck squamous cell carcinoma cell lines and transplanted xenografts. Cells 10, 517. doi:10.3390/cells10030517
Ye, X. Q., Li, Q., Wang, G. H., Sun, F. F., Huang, G. J., Bian, X. W., et al. (2011). Mitochondrial and energy metabolism-related properties as novel indicators of lung cancer stem cells. Int. J. Cancer 129, 820–831. doi:10.1002/ijc.25944
Yip, N. C., Fombon, I. S., Liu, P., Brown, S., Kannappan, V., Armesilla, A. L., et al. (2011). Disulfiram modulated ROS-MAPK and NFκB pathways and targeted breast cancer cells with cancer stem cell-like properties. Br. J. Cancer 104, 1564–1574. doi:10.1038/bjc.2011.126
Zhang, Y. W., Kaneda, M., and Morita, I. (2003). The gap junction-independent tumor-suppressing effect of connexin 43. J. Biol. Chem. 278, 44852–44856. doi:10.1074/jbc.M305072200
Zhao, P., Wang, Y., Kang, X., Wu, A., Yin, W., Tang, Y., et al. (2018). Dual-targeting biomimetic delivery for anti-glioma activity via remodeling the tumor microenvironment and directing macrophage-mediated immunotherapy. Chem. Sci. 9, 2674–2689. doi:10.1039/c7sc04853j
Keywords: gliomas, glioblastoma multiforme, disulfiram, drug repurposing, temozolomide
Citation: Zhong S, Shengyu Liu , Xin Shi , Zhang X, Li K, Liu G, Li L, Tao S, Zheng B, Sheng W, Ye Z, Xing Q, Zhai Q, Ren L, Wu Y and Bao Y (2022) Disulfiram in glioma: Literature review of drug repurposing. Front. Pharmacol. 13:933655. doi: 10.3389/fphar.2022.933655
Received: 01 May 2022; Accepted: 19 July 2022;
Published: 24 August 2022.
Edited by:
Chi Zhang, Indiana University Bloomington, United StatesReviewed by:
Syed Shams Ul Hassan, Shanghai Jiao Tong University, ChinaCopyright © 2022 Zhong, Shengyu Liu, Xin Shi, Zhang, Li, Liu, Li, Tao, Zheng, Sheng, Ye, Xing, Zhai, Ren, Wu and Bao. This is an open-access article distributed under the terms of the Creative Commons Attribution License (CC BY). The use, distribution or reproduction in other forums is permitted, provided the original author(s) and the copyright owner(s) are credited and that the original publication in this journal is cited, in accordance with accepted academic practice. No use, distribution or reproduction is permitted which does not comply with these terms.
*Correspondence: Ying Wu, d3V5aW5nQGNtdS5lZHUuY24=; Yijun Bao, eWpiYW9AY211LmVkdS5jbg==
†These authors have contributed equally to this work and share first authorship
Disclaimer: All claims expressed in this article are solely those of the authors and do not necessarily represent those of their affiliated organizations, or those of the publisher, the editors and the reviewers. Any product that may be evaluated in this article or claim that may be made by its manufacturer is not guaranteed or endorsed by the publisher.
Research integrity at Frontiers
Learn more about the work of our research integrity team to safeguard the quality of each article we publish.