- 1Department of Integrative Pharmacology, Mie University Graduate School of Medicine, Tsu, Japan
- 2Division of Medical Genetics, Kanagawa Children’s Medical Center, Yokohama, Japan
- 3Department of Clinical Dysmorphology, Mie University Graduate School of Medicine, Tsu, Japan
Various genetic and environmental factors are associated with developmental disorders (DDs). It has been suggested that interaction between genetic and environmental factors (G
Introduction
Developmental toxicity linked to early-life chemical exposure can have a crucial impact on the development of various tissues and is associated with developmental disorders (DDs) such as fetal alcohol syndrome (FAS), autism spectrum disorder (ASD), attention deficit hyperactivity disorder (ADHD), craniofacial anomalies, and congenital heart defects (De la Monte and Kril, 2014; Bölte et al., 2019; Beames and Lipinski, 2020; Hollander et al., 2020; Kalisch-Smith et al., 2020; Martinelli et al., 2020). The susceptibility to these chemicals may be determined by genetic factors (Lovely et al., 2017; Musci et al., 2019; Beames and Lipinski, 2020; Boyce et al., 2020; Gomes et al., 2021) (Figure 1). The gene–environment interaction (G
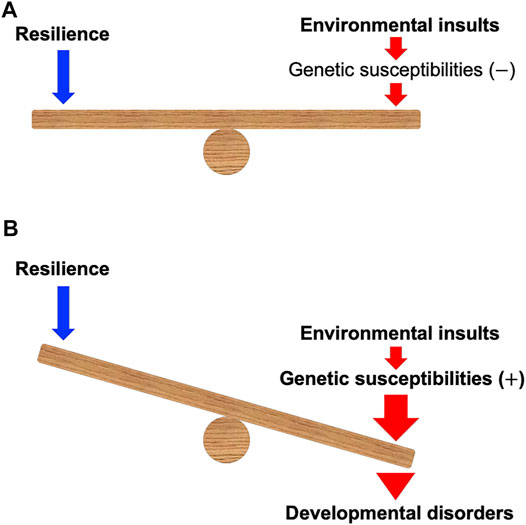
FIGURE 1. A model of gene–environment interaction proposed in this mini-review. (A) When environmental insults during development can be counterbalanced by the resilience in the individuals without the genetic susceptibilities, the insults may not cause developmental disorders. (B) Environmental insults during development can be enhanced and defeat the resilience of the individuals with the genetic susceptibilities, which may cause developmental disorders. Note that genetic susceptibilities may modulate the power of resilience.
G
Genetic Susceptibilities to Developmental Ethanol Exposure
Genome-Wide Approaches
Zebrafish have been successfully used to identify the genes involved in disease development through unbiased forward genetic screening with chemical mutagenesis (Mullins et al., 1994; Kelsh et al., 1996; Amsterdam and Hopkins, 2006; Swartz et al., 2020). The signaling pathways involved in cranial neural crest development are impaired in FAS, leading to various craniofacial anomalies such as cleft palate and holoprosencephaly (Smith et al., 2014; Nasreddine et al., 2021). To examine this, an N-ethyl-N-nitrosourea (ENU)-based random mutagenesis was performed to identify novel ethanol-sensitive zebrafish mutants, wherein F3 embryos from 126 inbred F2 families were exposed to 1% ethanol in the medium from 6 h post-fertilization (hpf) until they were screened. Alcian Blue/Alizarin Red staining was performed 4–7 days post-fertilization (dpf) to examine alterations in the craniofacial skeleton. The screening identified a novel ethanol-sensitive mutant in which the splice donor of exon 15 in si:dkey-88l16.3, a previously uncharacterized gene, was mutated (Swartz et al., 2020). The mechanisms of how the impairment of si:dkey-88l16.3 is involved in the craniofacial defects remain to be clarified.
Candidate Gene-Based Approaches
Genes involved in sonic hedgehog (SHH) signaling pathways have been intensively analyzed in studies on G
A screen of zebrafish mutants found that VANGL planar cell polarity protein 2 (vangl2) is involved in the genetic susceptibility to craniofacial defects induced by developmental ethanol exposure (Swartz et al., 2014). VANGL2 is a transmembrane protein that regulates the Wnt-mediated planar cell polarity (PCP) pathway (Yang and Mlodzik, 2015; Bailly et al., 2018; Bell et al., 2021). Zebrafish with mutations of vangl2 show slightly shortened craniofacial elements when there is no exposure to ethanol, whereas severe craniofacial anomalies such as synophthalmia, rod-like ethmoid plate, and disrupted axon projections, are observed in the vangl2 mutant exposed to ethanol during development (Swartz et al., 2014).
Impairment of platelet-derived growth factor (PDGF) receptor α (PDGFRA) and the resultant mutation in the 3′ untranslated region (UTR) of the PDGFRA gene (c.*34G > A) is associated with cleft palate in humans, mice, and zebrafish (Xu et al., 2005; Eberhart et al., 2008; Rattanasopha et al., 2012). MicroRNA (miRNA) 140 (miR-140) binds to the 3′-UTR of pdgfra and suppresses the expression of Pdgfra in zebrafish (Eberhart et al., 2008). The suppression of PDGFRA by miR-140 is also observed in cultured mouse palate cells (Li et al., 2019a). The c.*34G > A mutation is located 10 bp away from a predicted binding site of miR-140 (Rattanasopha et al., 2012). Ethanol exposure increases miR-140 levels in the extracellular vesicles of fetal neural stem cells (Tseng et al., 2019). Pdgfra is protective against ethanol-induced craniofacial anomalies in zebrafish (Mccarthy et al., 2013). These findings suggest that miR-140-mediated PDGFRA expression may be involved in the susceptibility to ethanol that is associated with craniofacial anomalies.
Genetic Susceptibilities to Other Developmental Toxicants
Genome-Wide Approaches
Genetic diversity in zebrafish populations can be used to analyze G
Candidate Gene-Based Approaches
Cranial neural crest cells regulate craniofacial development through multiple pathways, including SHH, and Wnt/PCP signaling pathways (Bush and Jiang, 2012; Suzuki et al., 2016). Candidate gene-based approaches that target genes involved in these pathways have successfully identified that G
There are global chemicals and therapeutic drugs that can affect SHH signaling. For example, piperonyl butoxide (PBO), a semisynthetic pesticide synergist present in hundreds of commercial products, can inhibit SHH signaling (Wang et al., 2012). In mice and zebrafish with haploinsufficiency of SHH, the embryos are sensitized to craniofacial defects induced by PBO (Everson et al., 2019; Everson et al., 2020).
Cholesterol is required in the SHH signaling cascade (Haas and Muenke, 2010). Statins, therapeutic drugs for hypercholesterolemia, negatively affect SHH signaling through the inhibition of 3-hydroxy-3-methyl-glutaryl-CoA reductase (HMGCR) that is a key enzyme in cholesterol synthesis (Haas and Muenke, 2010; Abramyan, 2019). In zebrafish, orofacial defects are induced by the developmental exposure to statins or mutation of hmgcr (Signore et al., 2016). Mutation of 7-dehydrocholesterol reductase gene that encodes an enzyme involved in cholesterol metabolism, is the cause of Smith-Lemli-Opitz syndrome (SLOS), a DD with multiple congenital anomalies including cleft palate and holoprosencephaly (Kelley and Hennekam, 2000). The severity of SLOS depends on the maternal apo E genotype (Witsch-Baumgartner et al., 2004). Apo E, a protein regulating the transport of cholesterol and other lipids in the blood and the brain, includes three common isoforms: ApoE2, ApoE3, and ApoE4 (Villeneuve et al., 2014). Because ApoE2 is defective in binding to low-density lipoprotein receptors, plasma total cholesterol level tends to be low in individuals with the ApoE2 genotype (Witsch-Baumgartner et al., 2004). The severity score of SLOS is higher in children from mothers with the ApoE2 genotype than from those without it (Witsch-Baumgartner et al., 2004). Individuals with the ApoE2 genotype are more sensitive to statin therapy than those with the ApoE4 genotype (Mega et al., 2009). A link between cholesterol metabolism and ASD has been suggested (Gillberg et al., 2017). These studies suggest that G
Mice with single-allele Gli2 mutation show an increased incidence of holoprosencephaly induced by vismodegib, a hedgehog pathway inhibitor (Heyne et al., 2016). Mice with a null mutation of Cdon, which encodes an SHH co-receptor, are sensitized to prenatal ethanol exposure to produce holoprosencephaly with defective expression of genes targeted by SHH (Hong and Krauss, 2012). Apart from the susceptibility to these teratogens, mice with haploinsufficiency of Shh or Gli2, or null allele of Cdon, are phenotypically indistinguishable from the wild-type littermates (Hong and Krauss, 2012; Kietzman et al., 2014; Heyne et al., 2016). In contrast, mice with a null mutation of Mosmo, which encodes a component of a membrane protein complex called MMM that promotes degradation of the Hedgehog signal transducer Smoothened, show multiple birth defects with increased SHH signaling (Kong et al., 2021). These birth defects can be suppressed by in utero treatment with vismodegib to inhibit SHH signaling (Kong et al., 2021). These studies suggest that individuals with mutations involved in the SHH pathway may be susceptible to chemicals that affect SHH signaling.
Mutation of vangl2 sensitizes the zebrafish to craniofacial anomalies induced by blebbistatin, an inhibitor of the Wnt/PCP pathway (Sidik et al., 2021). Genes involved in the PCP pathway have also emerged as susceptibility-inducing genes in ASD and other DDs (Sans et al., 2016; Milgrom-Hoffman and Humbert, 2018). Therefore, G
Non-coding RNA such as miRNA and long non-coding RNA (lncRNA) have also attracted attention as important mediators in response to environmental stressors (Miguel et al., 2020). For example, lncRNA is involved in the toxic response to dioxins, such as jaw malformation and pericardiac edema, by downregulating the expression of sox9b (Mathew et al., 2008; Xiong et al., 2008; Garcia et al., 2018). The roles of non-coding RNA in G
Discussion
Advances in genome editing technologies have enabled us to edit any gene of interest in various experimental models, including zebrafish and induced pluripotent stem (iPS) cells generated from human samples (Adachi et al., 2022; Whiteley et al., 2022). Public databases focusing on genes, biological samples, and chemicals related to various diseases including DDs, have been expanding (Al-Jawahiri and Milne, 2017; Reilly et al., 2017; Lombardo et al., 2019; Davis et al., 2021). These resources have accelerated G
Brain organoids derived from human iPS cells have emerged as a powerful tool to study the G
Public databases such as the Comparative Toxicogenomic Database (CTD) (Davis et al., 2021), Gene Expression Omnibus (GEO) (Clough and Barrett, 2016), Simons Simplex Collection (SSC) (Fischbach and Lord, 2010), and Autism Sequencing Consortium (ASC) (Buxbaum et al., 2012) can be used to discover novel interactions between chemicals and genes associated with DDs. For example, an integrative analysis using CTD, SSC, and ASC revealed a total of 212 gene–environment interaction pairs putatively relevant for ASD, and provided a list of candidate genes susceptible to chemicals associated with ASD, such as valproic acid, benzo(a)pyrene, bisphenol A, particulate matter, and perfluorooctane sulfonic acid (Santos et al., 2019). A novel in silico approach using GEO identified tumor suppressors: p53, retinoblastoma 1, and Krüppel-like factor 8 as leading nodes in the network of developmental neurotoxicity of selective serotonin reuptake inhibitors and antidepressants associated with ASD (Li et al., 2021). A study using CTD and a database of ASD gene networks (Nelson et al., 2012) found that ASD-associated genes are selectively targeted by environmental pollutants such as pesticides, heavy metals, and phthalates (Carter and Blizard, 2016). Novel disease-associated genes can be identified using whole exome sequencing of biological samples from patients with DDs (Kuroda et al., 2019a; Kuroda et al., 2019b). CTD can be used to examine whether the novel genes are targeted by environmental chemicals and thereby confirm the role of these genes in the susceptibility to the chemicals (Davis et al., 2021). A database named Human Tissue-specific Exposure Atlas (TExAs) has been developed by compilation of various databases, including CTD, Exposome-Explorer (Neveu et al., 2020), PubChem (Kim et al., 2021), ToxCast (Dix et al., 2007), and DisGeNET (Piñero et al., 2019). Using TExAs, one can retrieve the information about tissue-specific target genes of the chemicals and diseases associated with these genes (Ravichandran et al., 2021). The integration of databases combined with new approach methodologies may provide novel insights into the G
Author Contributions
YN and KK conceived the study and wrote the manuscript.
Funding
This work was supported in part by JSPS KAKENHI (19K07318 to YN), Long-range Research Initiative of the Japan Chemical Industrial Association (20-3-08 to YN), and Takeda Science Foundation (to YN and KK).
Conflict of Interest
The authors declare that the research was conducted in the absence of any commercial or financial relationships that could be construed as a potential conflict of interest.
Publisher’s Note
All claims expressed in this article are solely those of the authors and do not necessarily represent those of their affiliated organizations, or those of the publisher, the editors and the reviewers. Any product that may be evaluated in this article, or claim that may be made by its manufacturer, is not guaranteed or endorsed by the publisher.
References
Abramyan, J. (2019). Hedgehog Signaling and Embryonic Craniofacial Disorders. J. Dev. Biol. 7, 9. doi:10.3390/jdb7020009
Ackerman, S., Schoenbrun, S., Hudac, C., and Bernier, R. (2017). Erratum to: Interactive Effects of Prenatal Antidepressant Exposure and Likely Gene Disrupting Mutations on the Severity of Autism Spectrum Disorder. J. Autism Dev. Disord. 47, 3497–3496. doi:10.1007/s10803-017-3301-3
Adachi, Y., Higuchi, A., Wakai, E., Shiromizu, T., Koiwa, J., and Nishimura, Y. (2022). Involvement of Homeobox Transcription Factor Mohawk in Palatogenesis. Congenit. Anom. (Kyoto) 62, 27–37. doi:10.1111/cga.12451
Al-Jawahiri, R., and Milne, E. (2017). Resources Available for Autism Research in the Big Data Era: a Systematic Review. PeerJ 5, e2880. doi:10.7717/peerj.2880
Amsterdam, A., and Hopkins, N. (2006). Mutagenesis Strategies in Zebrafish for Identifying Genes Involved in Development and Disease. Trends Genet. 22, 473–478. doi:10.1016/j.tig.2006.06.011
Arzua, T., Yan, Y., Jiang, C., Logan, S., Allison, R. L., Wells, C., et al. (2020). Modeling Alcohol-Induced Neurotoxicity Using Human Induced Pluripotent Stem Cell-Derived Three-Dimensional Cerebral Organoids. Transl Psychiatry 10, 347. doi:10.1038/s41398-020-01029-4
Bailly, E., Walton, A., and Borg, J. P. (2018). The Planar Cell Polarity Vangl2 Protein: From Genetics to Cellular and Molecular Functions. Semin. Cel Dev Biol 81, 62–70. doi:10.1016/j.semcdb.2017.10.030
Balik-Meisner, M., Truong, L., Scholl, E. H., La Du, J. K., Tanguay, R. L., and Reif, D. M. (2018). Elucidating Gene-By-Environment Interactions Associated with Differential Susceptibility to Chemical Exposure. Environ. Health Perspect. 126, 067010. doi:10.1289/EHP2662
Beames, T. G., and Lipinski, R. J. (2020). Gene-environment Interactions: Aligning Birth Defects Research with Complex Etiology. Development 147, dev191064. doi:10.1242/dev.191064
Bell, I. J., Horn, M. S., and Van Raay, T. J. (2021). Bridging the gap between Non-canonical and Canonical Wnt Signaling through Vangl2. Semin. Cel Develop. Biol. doi:10.1016/j.semcdb.2021.10.004
Beversdorf, D. Q., Shah, A., Jhin, A., Noel-Macdonnell, J., Hecht, P., Ferguson, B. J., et al. (2021). microRNAs and Gene-Environment Interactions in Autism: Effects of Prenatal Maternal Stress and the SERT Gene on Maternal microRNA Expression. Front. Psychiatry 12, 668577. doi:10.3389/fpsyt.2021.668577
Bölte, S., Girdler, S., and Marschik, P. B. (2019). The Contribution of Environmental Exposure to the Etiology of Autism Spectrum Disorder. Cell Mol Life Sci 76, 1275–1297.
Boyce, W. T., Sokolowski, M. B., and Robinson, G. E. (2020). Genes and Environments, Development and Time. Proc. Natl. Acad. Sci. U S A. 117, 23235–23241. doi:10.1073/pnas.2016710117
Bush, J. O., and Jiang, R. (2012). Palatogenesis: Morphogenetic and Molecular Mechanisms of Secondary Palate Development. Development 139, 231–243. doi:10.1242/dev.067082
Buxbaum, J. D., Daly, M. J., Devlin, B., Lehner, T., Roeder, K., and State, M. W. (2012). The Autism Sequencing Consortium: Large-Scale, High-Throughput Sequencing in Autism Spectrum Disorders. Neuron 76, 1052–1056. doi:10.1016/j.neuron.2012.12.008
Carter, C. J., and Blizard, R. A. (2016). Autism Genes Are Selectively Targeted by Environmental Pollutants Including Pesticides, Heavy Metals, Bisphenol A, Phthalates and many Others in Food, Cosmetics or Household Products. Neurochem. Int. 101, 83–109doi:10.1016/j.neuint.2016.10.011
Cheroni, C., Caporale, N., and Testa, G. (2020). Autism Spectrum Disorder at the Crossroad between Genes and Environment: Contributions, Convergences, and Interactions in ASD Developmental Pathophysiology. Mol. Autism 11, 69. doi:10.1186/s13229-020-00370-1
Cicchetti, D., and Rogosch, F. A. (2012). Gene × Environment Interaction and Resilience: Effects of Child Maltreatment and Serotonin, Corticotropin Releasing Hormone, Dopamine, and Oxytocin Genes. Dev. Psychopathol 24, 411–427. doi:10.1017/S0954579412000077
Clough, E., and Barrett, T. (2016). The Gene Expression Omnibus Database. Methods Mol. Biol. 1418, 93–110. doi:10.1007/978-1-4939-3578-9_5
Cui, L., Du, W., Xu, N., Dong, J., Xia, B., Ma, J., et al. (2021). Impact of MicroRNAs in Interaction with Environmental Factors on Autism Spectrum Disorder: An Exploratory Pilot Study. Front. Psychiatry 12, 715481. doi:10.3389/fpsyt.2021.715481
Davis, A. P., Grondin, C. J., Johnson, R. J., Sciaky, D., Wiegers, J., Wiegers, T. C., et al. (2021). Comparative Toxicogenomics Database (CTD): Update 2021. Nucleic Acids Res. 49, D1138–d1143. doi:10.1093/nar/gkaa891
De La Monte, S. M., and Kril, J. J. (2014). Human Alcohol-Related Neuropathology. Acta Neuropathol. 127, 71–90. doi:10.1007/s00401-013-1233-3
Dix, D. J., Houck, K. A., Martin, M. T., Richard, A. M., Setzer, R. W., and Kavlock, R. J. (2007). The ToxCast Program for Prioritizing Toxicity Testing of Environmental Chemicals. Toxicol. Sci. 95, 5–12. doi:10.1093/toxsci/kfl103
Eberhart, J. K., He, X., Swartz, M. E., Yan, Y. L., Song, H., Boling, T. C., et al. (2008). MicroRNA Mirn140 Modulates Pdgf Signaling during Palatogenesis. Nat. Genet. 40, 290–298. doi:10.1038/ng.82
Eberhart, J. K., and Parnell, S. E. (2016). The Genetics of Fetal Alcohol Spectrum Disorders. Alcohol. Clin. Exp. Res. 40, 1154–1165. doi:10.1111/acer.13066
Elbau, I. G., Cruceanu, C., and Binder, E. B. (2019). Genetics of Resilience: Gene-By-Environment Interaction Studies as a Tool to Dissect Mechanisms of Resilience. Biol. Psychiatry 86, 433–442. doi:10.1016/j.biopsych.2019.04.025
Engel, S. M., Wetmur, J., Chen, J., Zhu, C., Barr, D. B., Canfield, R. L., et al. (2011). Prenatal Exposure to Organophosphates, Paraoxonase 1, and Cognitive Development in Childhood. Environ. Health Perspect. 119, 1182–1188. doi:10.1289/ehp.1003183
Esposito, G., Azhari, A., and Borelli, J. L. (2018). Gene × Environment Interaction in Developmental Disorders: Where Do We Stand and What's Next? Front. Psychol. 9, 2036. doi:10.3389/fpsyg.2018.02036
Everson, J. L., Batchu, R., and Eberhart, J. K. (2020). Multifactorial Genetic and Environmental Hedgehog Pathway Disruption Sensitizes Embryos to Alcohol-Induced Craniofacial Defects. Alcohol. Clin. Exp. Res. 44, 1988–1996. doi:10.1111/acer.14427
Everson, J. L., Sun, M. R., Fink, D. M., Heyne, G. W., Melberg, C. G., Nelson, K. F., et al. (2019). Developmental Toxicity Assessment of Piperonyl Butoxide Exposure Targeting Sonic Hedgehog Signaling and Forebrain and Face Morphogenesis in the Mouse: An In Vitro and In Vivo Study. Environ. Health Perspect. 127, 107006. doi:10.1289/EHP5260
Fernandes, Y., and Lovely, C. B. (2021). Zebrafish Models of Fetal Alcohol Spectrum Disorders. Genesis 59, e23460. doi:10.1002/dvg.23460
Fischbach, G. D., and Lord, C. (2010). The Simons Simplex Collection: a Resource for Identification of Autism Genetic Risk Factors. Neuron 68, 192–195. doi:10.1016/j.neuron.2010.10.006
Garcia, G. R., Shankar, P., Dunham, C. L., Garcia, A., La Du, J. K., Truong, L., et al. (2018). Signaling Events Downstream of AHR Activation that Contribute to Toxic Responses: The Functional Role of an AHR-dependent Long Noncoding RNA (slincR) Using the Zebrafish Model. Environ. Health Perspect. 126, 117002. doi:10.1289/EHP3281
Gillberg, C., Fernell, E., Kočovská, E., Minnis, H., Bourgeron, T., Thompson, L., et al. (2017). The Role of Cholesterol Metabolism and Various Steroid Abnormalities in Autism Spectrum Disorders: A Hypothesis Paper. Autism Res. 10, 1022–1044. doi:10.1002/aur.1777
Gomes, J. D. A., Olstad, E. W., Kowalski, T. W., Gervin, K., Vianna, F. S. L., Schüler-Faccini, L., et al. (2021). Genetic Susceptibility to Drug Teratogenicity: A Systematic Literature Review. Front. Genet. 12, 645555. doi:10.3389/fgene.2021.645555
Haas, D., and Muenke, M. (2010). Abnormal Sterol Metabolism in Holoprosencephaly. Am. J. Med. Genet. C Semin. Med. Genet. 154c, 102–108. doi:10.1002/ajmg.c.30243
Harrill, A. H., and Mcallister, K. A. (2017). New Rodent Population Models May Inform Human Health Risk Assessment and Identification of Genetic Susceptibility to Environmental Exposures. Environ. Health Perspect. 125, 086002. doi:10.1289/EHP1274
Hermkens, D. M., Van Impel, A., Urasaki, A., Bussmann, J., Duckers, H. J., and Schulte-Merker, S. (2015). Sox7 Controls Arterial Specification in Conjunction with Hey2 and Efnb2 Function. Development 142, 1695–1704. doi:10.1242/dev.117275
Heyne, G. W., Everson, J. L., Ansen-Wilson, L. J., Melberg, C. G., Fink, D. M., Parins, K. F., et al. (2016). Gli2 Gene-Environment Interactions Contribute to the Etiological Complexity of Holoprosencephaly: Evidence from a Mouse Model. Dis. Model. Mech. 9, 1307–1315. doi:10.1242/dmm.026328
Hollander, J. A., Cory-Slechta, D. A., Jacka, F. N., Szabo, S. T., Guilarte, T. R., Bilbo, S. D., et al. (2020). Beyond the Looking Glass: Recent Advances in Understanding the Impact of Environmental Exposures on Neuropsychiatric Disease. Neuropsychopharmacology 45, 1086–1096. doi:10.1038/s41386-020-0648-5
Hong, M., and Krauss, R. S. (2012). Cdon Mutation and Fetal Ethanol Exposure Synergize to Produce Midline Signaling Defects and Holoprosencephaly Spectrum Disorders in Mice. Plos Genet. 8, e1002999. doi:10.1371/journal.pgen.1002999
Hong, M., and Krauss, R. S. (2018). Modeling the Complex Etiology of Holoprosencephaly in Mice. Am. J. Med. Genet. C Semin. Med. Genet. 178, 140–150. doi:10.1002/ajmg.c.31611
Kalisch-Smith, J. I., Ved, N., and Sparrow, D. B. (2020). Environmental Risk Factors for Congenital Heart Disease. Cold Spring Harb Perspect. Biol. 12, a037234. doi:10.1101/cshperspect.a037234
Kelley, R. I., and Hennekam, R. C. (2000). The Smith-Lemli-Opitz Syndrome. J. Med. Genet. 37, 321–335. doi:10.1136/jmg.37.5.321
Kelsh, R. N., Brand, M., Jiang, Y. J., Heisenberg, C. P., Lin, S., Haffter, P., et al. (1996). Zebrafish Pigmentation Mutations and the Processes of Neural Crest Development. Development 123, 369–389. doi:10.1242/dev.123.1.369
Kietzman, H. W., Everson, J. L., Sulik, K. K., and Lipinski, R. J. (2014). The Teratogenic Effects of Prenatal Ethanol Exposure Are Exacerbated by Sonic Hedgehog or GLI2 Haploinsufficiency in the Mouse. PLoS One 9, e89448. doi:10.1371/journal.pone.0089448
Kim, D., Volk, H., Girirajan, S., Pendergrass, S., Hall, M. A., Verma, S. S., et al. (2017). The Joint Effect of Air Pollution Exposure and Copy Number Variation on Risk for Autism. Autism Res. 10, 1470–1480. doi:10.1002/aur.1799
Kim, S., Chen, J., Cheng, T., Gindulyte, A., He, J., He, S., et al. (2021). PubChem in 2021: New Data Content and Improved Web Interfaces. Nucleic Acids Res. 49, D1388–d1395. doi:10.1093/nar/gkaa971
Kong, J. H., Young, C. B., Pusapati, G. V., Espinoza, F. H., Patel, C. B., Beckert, F., et al. (2021). Gene-teratogen Interactions Influence the Penetrance of Birth Defects by Altering Hedgehog Signaling Strength. Development 148, dev199867. doi:10.1242/dev.199867
Kuroda, Y., Murakami, H., Enomoto, Y., Tsurusaki, Y., Takahashi, K., Mitsuzuka, K., et al. (2019a). A Novel Gene (FAM20B Encoding Glycosaminoglycan Xylosylkinase) for Neonatal Short Limb Dysplasia Resembling Desbuquois Dysplasia. Clin. Genet. 95, 713–717. doi:10.1111/cge.13530
Kuroda, Y., Murakami, H., Yokoi, T., Kumaki, T., Enomoto, Y., Tsurusaki, Y., et al. (2019b). Two Unrelated Girls with Intellectual Disability Associated with a Truncating Mutation in the PPM1D Penultimate Exon. Brain Dev. 41, 538–541. doi:10.1016/j.braindev.2019.02.007
Li, A., Jia, P., Mallik, S., Fei, R., Yoshioka, H., Suzuki, A., et al. (2019a). Critical microRNAs and Regulatory Motifs in Cleft Palate Identified by a Conserved miRNA-TF-Gene Network Approach in Humans and Mice. Brief Bioinform 21, 1465–1478. doi:10.1093/bib/bbz082
Li, J., Li, X., Zhang, S., and Snyder, M. (2019b). Gene-Environment Interaction in the Era of Precision Medicine. Cell 177, 38–44. doi:10.1016/j.cell.2019.03.004
Li, R. A., Talikka, M., Gubian, S., Vom Berg, C., Martin, F., Peitsch, M. C., et al. (2021). Systems Toxicology Approach for Assessing Developmental Neurotoxicity in Larval Zebrafish. Front. Genet. 12, 652632. doi:10.3389/fgene.2021.652632
Lombardo, M. V., Lai, M. C., and Baron-Cohen, S. (2019). Big Data Approaches to Decomposing Heterogeneity across the Autism Spectrum. Mol. Psychiatry 24, 1435–1450. doi:10.1038/s41380-018-0321-0
Lovely, C. B. (2020). Animal Models of Gene-Alcohol Interactions. Birth Defects Res. 112, 367–379. doi:10.1002/bdr2.1623
Lovely, C., Rampersad, M., Fernandes, Y., and Eberhart, J. (2017). Gene-environment Interactions in Development and Disease. Wiley Interdiscip. Rev. Dev. Biol. 6, 247. doi:10.1002/wdev.247
Martinelli, M., Palmieri, A., Carinci, F., and Scapoli, L. (2020). Non-syndromic Cleft Palate: An Overview on Human Genetic and Environmental Risk Factors. Front Cel Dev Biol 8, 592271. doi:10.3389/fcell.2020.592271
Mathew, L. K., Sengupta, S. S., Ladu, J., Andreasen, E. A., and Tanguay, R. L. (2008). Crosstalk between AHR and Wnt Signaling through R-Spondin1 Impairs Tissue Regeneration in Zebrafish. Faseb j 22, 3087–3096. doi:10.1096/fj.08-109009
Mazina, V., Gerdts, J., Trinh, S., Ankenman, K., Ward, T., Dennis, M. Y., et al. (2015). Epigenetics of Autism-Related Impairment: Copy Number Variation and Maternal Infection. J. Dev. Behav. Pediatr. 36, 61–67. doi:10.1097/DBP.0000000000000126
Mcallister, K., Mechanic, L. E., Amos, C., Aschard, H., Blair, I. A., Chatterjee, N., et al. (2017). Current Challenges and New Opportunities for Gene-Environment Interaction Studies of Complex Diseases. Am. J. Epidemiol. 186, 753–761. doi:10.1093/aje/kwx227
Mccarthy, N., Wetherill, L., Lovely, C. B., Swartz, M. E., Foroud, T. M., and Eberhart, J. K. (2013). Pdgfra Protects against Ethanol-Induced Craniofacial Defects in a Zebrafish Model of FASD. Development 140, 3254–3265. doi:10.1242/dev.094938
Mega, J. L., Morrow, D. A., Brown, A., Cannon, C. P., and Sabatine, M. S. (2009). Identification of Genetic Variants Associated with Response to Statin Therapy. Arterioscler Thromb. Vasc. Biol. 29, 1310–1315. doi:10.1161/ATVBAHA.109.188474
Miguel, V., Lamas, S., and Espinosa-Diez, C. (2020). Role of Non-coding-RNAs in Response to Environmental Stressors and Consequences on Human Health. Redox Biol. 37, 101580. doi:10.1016/j.redox.2020.101580
Milgrom-Hoffman, M., and Humbert, P. O. (2018). Regulation of Cellular and PCP Signalling by the Scribble Polarity Module. Semin. Cel Dev Biol 81, 33–45. doi:10.1016/j.semcdb.2017.11.021
Modafferi, S., Zhong, X., Kleensang, A., Murata, Y., Fagiani, F., Pamies, D., et al. (2021). Gene-Environment Interactions in Developmental Neurotoxicity: a Case Study of Synergy between Chlorpyrifos and CHD8 Knockout in Human BrainSpheres. Environ. Health Perspect. 129, 077001. doi:10.1289/ehp8580
Molnar-Szakacs, I., Kupis, L., and Uddin, L. Q. (2021). Neuroimaging Markers of Risk and Pathways to Resilience in Autism Spectrum Disorder. Biol. Psychiatry Cogn. Neurosci. Neuroimaging 6, 200–210. doi:10.1016/j.bpsc.2020.06.017
Mullins, M. C., Hammerschmidt, M., Haffter, P., and Nüsslein-Volhard, C. (1994). Large-scale Mutagenesis in the Zebrafish: in Search of Genes Controlling Development in a Vertebrate. Curr. Biol. 4, 189–202. doi:10.1016/s0960-9822(00)00048-8
Musci, R. J., Augustinavicius, J. L., and Volk, H. (2019). Gene-Environment Interactions in Psychiatry: Recent Evidence and Clinical Implications. Curr. Psychiatry Rep. 21, 81. doi:10.1007/s11920-019-1065-5
Nasreddine, G., El Hajj, J., and Ghassibe-Sabbagh, M. (2021). Orofacial Clefts Embryology, Classification, Epidemiology, and Genetics. Mutat. Res. Rev. Mutat. Res. 787, 108373. doi:10.1016/j.mrrev.2021.108373
Nelson, T. H., Jung, J. Y., Deluca, T. F., Hinebaugh, B. K., St Gabriel, K. C., and Wall, D. P. (2012). Autworks: a Cross-Disease Network Biology Application for Autism and Related Disorders. BMC Med. Genomics 5, 56. doi:10.1186/1755-8794-5-56
Neveu, V., Nicolas, G., Salek, R. M., Wishart, D. S., and Scalbert, A. (2020). Exposome-Explorer 2.0: an Update Incorporating Candidate Dietary Biomarkers and Dietary Associations with Cancer Risk. Nucleic Acids Res. 48, D908–d912. doi:10.1093/nar/gkz1009
Piñero, J., Ramírez-Anguita, J. M., Saüch-Pitarch, J., Ronzano, F., Centeno, E., Sanz, F., et al. (2019). The DisGeNET Knowledge Platform for Disease Genomics: 2019 Update. Nucleic Acids Res. 48, D845–D855.
Raterman, S. T., Metz, J. R., Wagener, F. A. D. T. G., and Von Den Hoff, J. W. (2020). Zebrafish Models of Craniofacial Malformations: Interactions of Environmental Factors. Front. Cel Dev Biol 8, 600926. doi:10.3389/fcell.2020.600926
Rattanasopha, S., Tongkobpetch, S., Srichomthong, C., Siriwan, P., Suphapeetiporn, K., and Shotelersuk, V. (2012). PDGFRa Mutations in Humans with Isolated Cleft Palate. Eur. J. Hum. Genet. 20, 1058–1062. doi:10.1038/ejhg.2012.55
Ravichandran, J., Karthikeyan, B. S., Aparna, S. R., and Samal, A. (2021). Network Biology Approach to Human Tissue-specific Chemical Exposome. J. Steroid Biochem. Mol. Biol. 214, 105998. doi:10.1016/j.jsbmb.2021.105998
Reilly, J., Gallagher, L., Chen, J. L., Leader, G., and Shen, S. (2017). Bio-collections in Autism Research. Mol. Autism 8, 34. doi:10.1186/s13229-017-0154-8
Sans, N., Ezan, J., Moreau, M. M., and Montcouquiol, M. (2016). “Planar Cell Polarity Gene Mutations in Autism Spectrum Disorder, Intellectual Disabilities, and Related Deletion/Duplication Syndromes,” in Neuronal and Synaptic Dysfunction in Autism Spectrum Disorder and Intellectual Disability. Editors C. Sala, and C. Verpelli (San Diego: Academic Press), 189–219. doi:10.1016/b978-0-12-800109-7.00013-3
Santos, J. X., Rasga, C., Marques, A. R., Martiniano, H. F. M. C., Asif, M., Vilela, J., et al. (2019). A Role for Gene-Environment Interactions in Autism Spectrum Disorder Is Suggested by Variants in Genes Regulating Exposure to Environmental Factors. bioRxiv, 520544.
Schmidt, S. (2021). Autism in Three Dimensions: Using Brain Organoids to Study Potential Gene-Environment Interactions. Environ. Health Perspect. 129, 104003. doi:10.1289/EHP10301
Sidik, A., Dixon, G., Buckley, D. M., Kirby, H. G., Sun, S., and Eberhart, J. K. (2021). Exposure to Ethanol Leads to Midfacial Hypoplasia in a Zebrafish Model of FASD via Indirect Interactions with the Shh Pathway. BMC Biol. 19, 134. doi:10.1186/s12915-021-01062-9
Signore, I. A., Jerez, C., Figueroa, D., Suazo, J., Marcelain, K., Cerda, O., et al. (2016). Inhibition of the 3-Hydroxy-3-Methyl-Glutaryl-CoA Reductase Induces Orofacial Defects in Zebrafish. Birth Defects Res. A. Clin. Mol. Teratol 106, 814–830. doi:10.1002/bdra.23546
Smith, S. M., Garic, A., Flentke, G. R., and Berres, M. E. (2014). Neural Crest Development in Fetal Alcohol Syndrome. Birth Defects Res. C Embryo Today 102, 210–220. doi:10.1002/bdrc.21078
Suzuki, A., Sangani, D. R., Ansari, A., and Iwata, J. (2016). Molecular Mechanisms of Midfacial Developmental Defects. Dev. Dyn. 245, 276–293. doi:10.1002/dvdy.24368
Swartz, M. E., Lovely, C. B., Mccarthy, N., Kuka, T., and Eberhart, J. K. (2020). Novel Ethanol-Sensitive Mutants Identified in an F3 Forward Genetic Screen. Alcohol. Clin. Exp. Res. 44, 56–65. doi:10.1111/acer.14240
Swartz, M. E., Wells, M. B., Griffin, M., Mccarthy, N., Lovely, C. B., Mcgurk, P., et al. (2014). A Screen of Zebrafish Mutants Identifies Ethanol-Sensitive Genetic Loci. Alcohol. Clin. Exp. Res. 38, 694–703. doi:10.1111/acer.12286
Tseng, A. M., Chung, D. D., Pinson, M. R., Salem, N. A., Eaves, S. E., and Miranda, R. C. (2019). Ethanol Exposure Increases miR-140 in Extracellular Vesicles: Implications for Fetal Neural Stem Cell Proliferation and Maturation. Alcohol. Clin. Exp. Res. 43, 1414–1426. doi:10.1111/acer.14066
Villeneuve, S., Brisson, D., Marchant, N. L., and Gaudet, D. (2014). The Potential Applications of Apolipoprotein E in Personalized Medicine. Front. Aging Neurosci. 6, 154. doi:10.3389/fnagi.2014.00154
Volk, H. E., Kerin, T., Lurmann, F., Hertz-Picciotto, I., Mcconnell, R., and Campbell, D. B. (2014). Autism Spectrum Disorder: Interaction of Air Pollution with the MET Receptor Tyrosine Kinase Gene. Epidemiology 25, 44–47. doi:10.1097/EDE.0000000000000030
Wang, J., Lu, J., Mook, R. A., Zhang, M., Zhao, S., Barak, L. S., et al. (2012). The Insecticide Synergist Piperonyl Butoxide Inhibits Hedgehog Signaling: Assessing Chemical Risks. Toxicol. Sci. 128, 517–523. doi:10.1093/toxsci/kfs165
Wat, M. J., Beck, T. F., Hernández-García, A., Yu, Z., Veenma, D., Garcia, M., et al. (2012). Mouse Model Reveals the Role of SOX7 in the Development of Congenital Diaphragmatic Hernia Associated with Recurrent Deletions of 8p23.1. Hum. Mol. Genet. 21, 4115–4125. doi:10.1093/hmg/dds241
Whiteley, J. T., Fernandes, S., Sharma, A., Mendes, A. P. D., Racha, V., Benassi, S. K., et al. (2022). Reaching into the Toolbox: Stem Cell Models to Study Neuropsychiatric Disorders. Stem Cel Rep. 17, 187–210. doi:10.1016/j.stemcr.2021.12.015
Witsch-Baumgartner, M., Gruber, M., Kraft, H. G., Rossi, M., Clayton, P., Giros, M., et al. (2004). Maternal Apo E Genotype Is a Modifier of the Smith-Lemli-Opitz Syndrome. J. Med. Genet. 41, 577–584. doi:10.1136/jmg.2004.018085
Xiong, K. M., Peterson, R. E., and Heideman, W. (2008). Aryl Hydrocarbon Receptor-Mediated Down-Regulation of Sox9b Causes Jaw Malformation in Zebrafish Embryos. Mol. Pharmacol. 74, 1544–1553. doi:10.1124/mol.108.050435
Xu, X., Bringas, P., Soriano, P., and Chai, Y. (2005). PDGFR-alpha Signaling Is Critical for Tooth Cusp and Palate Morphogenesis. Dev. Dyn. 232, 75–84. doi:10.1002/dvdy.20197
Yang, Y., and Mlodzik, M. (2015). Wnt-Frizzled/planar Cell Polarity Signaling: Cellular Orientation by Facing the Wind (Wnt). Annu. Rev. Cel Dev Biol 31, 623–646. doi:10.1146/annurev-cellbio-100814-125315
Keywords: developmental toxicity, gene-environment interaction, susceptibility, resilience, organoid, clinical genetics
Citation: Nishimura Y and Kurosawa K (2022) Analysis of Gene-Environment Interactions Related to Developmental Disorders. Front. Pharmacol. 13:863664. doi: 10.3389/fphar.2022.863664
Received: 27 January 2022; Accepted: 03 March 2022;
Published: 17 March 2022.
Edited by:
Aramandla Ramesh, Meharry Medical College, United StatesReviewed by:
James Alan Marrs, Indiana University—Purdue University Indianapolis, United StatesCopyright © 2022 Nishimura and Kurosawa. This is an open-access article distributed under the terms of the Creative Commons Attribution License (CC BY). The use, distribution or reproduction in other forums is permitted, provided the original author(s) and the copyright owner(s) are credited and that the original publication in this journal is cited, in accordance with accepted academic practice. No use, distribution or reproduction is permitted which does not comply with these terms.
*Correspondence: Yuhei Nishimura, eXVoZWlAbWVkLm1pZS11LmFjLmpw