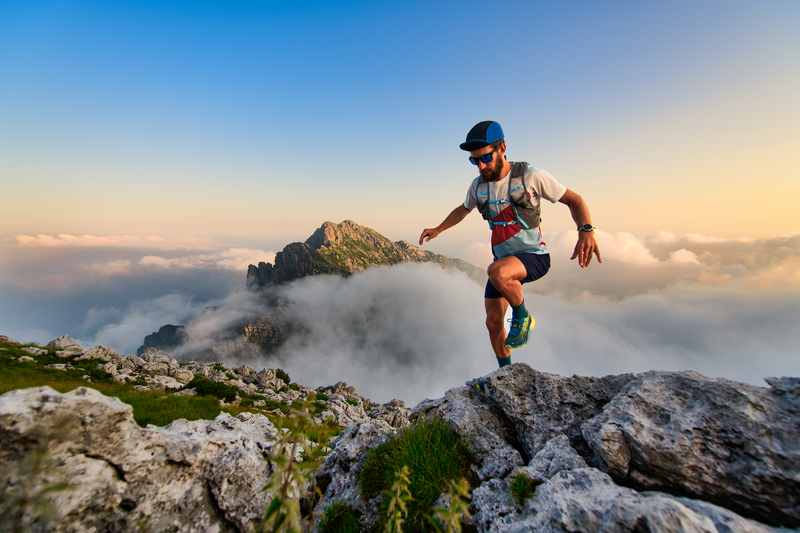
94% of researchers rate our articles as excellent or good
Learn more about the work of our research integrity team to safeguard the quality of each article we publish.
Find out more
REVIEW article
Front. Pharmacol. , 23 September 2021
Sec. Inflammation Pharmacology
Volume 12 - 2021 | https://doi.org/10.3389/fphar.2021.750857
This article is part of the Research Topic Inflammatory immune disease: Molecular Mechanisms, Translational Approaches and Therapeutics View all 97 articles
Gliomas are the most common primary tumors of the central nervous system. Due to the existence of the blood-brain barrier and its unique regional immune characteristics, the study of the immune microenvironment of gliomas is particularly important. Glioma stem cells are an important cause of initiating glioma, promoting tumor progression and leading to tumor recurrence. Immunotherapeutic strategies targeting glioma stem cells have become the focus of current research. This paper will focus on the research progress of glioma stem cells in the immune microenvironment of glioma to provide the basis for the immunotherapy of glioma.
Glioma is one of the most common malignant tumors of the central nervous system. According to the statistics of brain Tumor Registry in the United States, glioma accounts for about 80% of all malignant tumors of the central nervous system, and more than half of gliomas are glioblastoma (GBM) (Ostrom et al., 2015). GBM growth is aggressive, causes tumor recurrence, migration, so that it is difficult to cure. Currently, the standardized treatment plan for glioma, namely, the maximum safe surgical resection of the tumor supplemented by postoperative concurrent chemoradiotherapy or adjuvant chemotherapy, has a very poor prognosis with a median survival of only 15 months due to its rapid growth, high invasiity and multi-tolerance of chemoradiotherapy (Eder and Kalman, 2014; Wang et al., 2016). Even emerging molecular targeted therapy, electric field therapy and other methods, but these can only alleviate the disease to a limited extent, there is no significant improvement in overall survival. Recent studies have shown that the brain can connect to the peripheral immune system through meningeal lymphatic vessels. This subversive finding reconsiders the relationship between the brain immune microenvironment and neurological diseases (Louveau et al., 2016). Immunotargeted therapy is a hot topic in glioma treatment (Agarwal et al., 2011). Glioma stem cells (GSCs) play an important role in initiating glioma, promoting tumor progression and leading to tumor recurrence (Singh et al., 2004; Sundar et al., 2014). In the microenvironment of glioma, GSCs can not only cause reprogramming of related cells in the microenvironment, but also have a high inhibitory effect on the antitumor activity of immune cells (Laterra et al., 2021). The study of GSCs in glioma immune microenvironment and targeting GSCs therapy have become the forefront focus of neurooncology. In this paper, we will review and analyze the research progress of glioma immune microenvironment and targeted immunotherapy for GSCs.
The microenvironment of glioma tissue is composed of tumor cells, immune cells and various cytokines secreted by them. These cytokines, including pro-inflammatory factors, anti-inflammatory factors and chemokines, constitute a microenvironmental network, which interact with each other to jointly regulate the regional immune effect and ultimately determine the outcome of the disease (Grabowski et al., 2021).
Glioma highly inhibits tumor immunity, and glioma cells produce immunosuppressive factors such as TGF-β, IL-10 and indoleamine–pyrrole 2, 3-dioxygenase (IDO) (Tran et al., 2007; Preusser et al., 2015) Glioma also expresses immune checkpoint ligand to inhibit immune response (Garber et al., 2016; Hodges et al., 2017), so it is difficult to use immunotherapy to treat glioma. In addition, Glioma tumor microenvironment is filled with a large number of regulatory T cells (Tregs), M2 tumor-associated macrophages (TAMs), and myeloid inhibitory cells (MDSCs), which are closely related to the poor prognosis of glioma (Yue et al., 2014). Many clinical trials have been conducted to suppress immune checkpoints by reinvigorating the body’s immune system against glioma (Preusser et al., 2015).
Macrophages infiltrated by gliomas mainly come from monocytes in peripheral blood circulation, which are recruited to tumor regions and differentiated into tumor infiltrating macrophages (Sica and Bronte, 2007). Gliomas associated macrophages are usually immunosuppressive (Grabowski et al., 2021). Promote the fine direct contact between macrophages and tumors to create conditions for tumor cells to induce immunosuppression of macrophages (Zheng et al., 2013). Antitumor drugs targeting macrophages (Yu et al., 2021), such as colony-stimulating factor-1 receptor (CSF-1R), have been used to create glioma models in mice (Pyonteck et al., 2013; Sun et al., 2019; Akkari et al., 2020) found that targeting these Gliomas associated macrophages populations using a colony-stimulating factor-1 receptor (CSF-1R) inhibitor combined with radiotherapy substantially enhanced survival in preclinical models.
Microglia are both CNS-resident myeloid cells and the most important form of defense in the central nervous system. Ye et al. found that a large number of microglial cell infiltrates in gliomas, and the degree of infiltration is positively correlated with the degree of malignancy of gliomas (Ye et al., 2012). Glioma-derived factors can enhance the migration and continuous proliferation of microglia and promote the local aggregation of microglia in tumors by enhancing the adhesion kinase and PI3K/Akt pathway (Ellert-Miklaszewska et al., 2013). Microglia not only express CSF1R, but also GABA receptors (Beppu et al., 2013; Liu et al., 2016). Glutamate induces targeted chemotaxis of microglias to glioma margins and releases ligands in an AMPAR-dependent manner, promoting invasion of glioma cells and stemness activation of GSCs (Guo et al., 2009). In synaptic studies (Parkhurst et al., 2013; So-Hee et al., 2013; Miyamoto et al., 2016), microglia have been found to mediate synaptic formation through direct contact or secretion of soluble proteins BDNF or IL-10. However, GSCs induce IL-10 secretion through microglia (Wu et al., 2010), suggesting that GSCs secrete IL-10 through a bidirectional signaling axis by microglia, leading to abnormal synaptic formation, which also provides potential research direction for immunotherapy targeting GSCs.
Macrophages and microglias showed mutual activation and counterbalance in glioma immune microenvironment and tumor progression. The specific and unique roles of microglia and macrophages depend on their localization in and around the tumor (Radin and Tsirka, 2020). Studies on IDH mutated gliomas have found that macrophage transcription programs on microglias increase with tumor grade (Venteicher et al., 2017). Studies in mouse glioma models have found that microglias are predominantly located in the margins of gliomas, where they may promote spatially related behaviors such as invasion, proliferation, and stemness (Hambardzumyan et al., 2015; Shen et al., 2016; Caponegro et al., 2020). Caponegro et al. found that microglias promote the recruitment of anti-inflammatory macrophages and T regulatory cells from systemic circulation by releasing chemokines such as CCL2 (Caponegro et al., 2020). Their study also found that the enrichment of associated microglias around gliomas was associated with reduced patient survival. Microglia can also promote stem cell differentiation by releasing IL-6 (Wang et al., 2009; Zhang et al., 2012), which in turn induces GSCs to recruit anti-inflammatory macrophages by releasing osteoperioprotein (Zhou et al., 2015). An increased proportion of macrophages was found in studies of recurrent gliomas, which may indicate that macrophages play a potential role in mediating the recurrence of gliomas after radiation (Akkari et al., 2020).
Regulatory T cells (Treg) and Myeloid-derived suppressor cells (MDSC) play an immunosuppressive role in glioma and are important reasons for mediating immune escape. Treg does not exist in normal human brain tissue, but there are a large number of immunosuppressive Treg cells in GBM microenvironment, and the degree of Treg infiltration in glioma is closely related to tumor origin and pathological grade (Heimberger et al., 2015). Treg not only inhibits the activation of effector T cells by presenting surface antigens (Mccoy and Gros, 1999), but also inhibits the functions of other immune cells by secreting cytokines (IL-10, TGF-β and so on) and induces the transformation of T cells into Treg (Câmara et al., 2003; Cantini et al., 2011). Liu et al. found that Treg infiltrated densely in gliomas induced the expression of stemness related genes CD133, SOX2, NESTIN and so on, activated GSCs and promoted tumor growth by secreting TGF-β mediated NF-κB-IL6-STAT3 signaling pathway (Liu et al., 2021). In addition, their results showed that blocking the IL-6 receptor with tocilizumab eliminated this effect in in vitro and in vivo models.
MDSC are a type of heterogeneous cells, including immature macrophages, granulocytes, dendritic cell (DC), and other myeloid cells in the early stage of differentiation. Its main functions include promoting the increased expression of immunosuppressive molecules (IL-10, TGF-β and so on), inhibiting DC differentiation, inhibiting phagocytosis, reducing NK cell cytotoxicity and inducing T cell apoptosis (Rodrigues et al., 2010). Clinical studies have shown that COX-2 inhibitors (such as aspirin) can inhibit gliomas (Fujita et al., 2011). The mechanism may be that the COX-2 pathway can promote the formation of MDSC and the microenvironment of its polymerization, inhibit the infiltration of cytotoxic T lymphocytes, and thus promote the growth of glioma. The infiltration and accumulation of MDSC and Treg in GBM drives the local immunosuppression influenced by other immune cells. Chang et al. found that macrophage and microglia in the glioma microenvironment produce chemokine CCL2, which is crucial for recruiting Treg and MDSC (Chang et al., 2016). Their results showed that in a mouse glioma model, tumors growing in CCL2-deficient mice did not maximize the accumulation of Treg and MDSC.
Natural killer (NK) cells can secrete perforin and granase to induce apoptosis or necrosis of target cells. In gliomas, NK cells are the first to be recruited to the glioma region, but their function is inhibited, and the degree of functional inhibition is positively correlated with the degree of malignancy of gliomas (Dix et al., 1999). Glioma cells can inhibit NK cells’ function not only by direct contact, but also by secreting inhibitory factors (Aldemir et al., 2005; Hao et al., 2011; Wolpert et al., 2012). In addition, (Orozco-Morales et al., 2015) found that the specific changes of tumor suppressor factor (Rb) and proto-oncogene (Ras) were also an important reason for the resistance of glioma cells to NK cell-mediated cytotoxicity. Therefore, adoptive immunotherapy based on NK cells is a promising immunotherapeutic strategy for glioma. In vitro studies have shown that GSCs can express high levels of PVR and adhesin 2, and NK cells activated receptor DNAM⁃1 can specifically recognize the above two ligands, thus killing GSCs (Castriconi et al., 2009). They also found that GSCs derived from GBM were highly sensitive to NK cell-mediated live lysates stimulated by IL⁃2 or IL⁃15 (Castriconi et al., 2009).
In the tumor microenvironment, cytokines are communication mediators between cells and tissues, and are closely related to the differentiation and activation of immune cells in the tumor microenvironment. Immunoregulatory cytokines such as pro-inflammatory factors (IL-1, IL-2, TNF-α, IFN-γ), anti-inflammatory factors (IL-10, TGF-β), and chemokines (CCL-2, CCL-5, CCL-7, CX3CL1) are synthesized and secreted in the microenvironment of glioma. These cytokines and their receptors form a comprehensive regulatory network in the local tumor, and play an important role in tumor progression and tumor immune response. Here we introduce some of the latest and most popular factors.
Transforming growth factor⁃β (TGF-β) signal transducing pathway is involved in multiple links of glioma genesis and malignant progression, and the change of TGF-β expression in serum of patients with malignant glioma is positively correlated with tumor grade and prognosis. TGF-β can induce monocyte recruitment, macrophage phagocytosis inhibition, tumor local T cell proliferation inhibition and other effects (Wu et al., 2010). TGF-β signal transduction pathway mainly regulates stem cell-related genes (such as SOX2 and SOX4) to achieve self-renewal and inhibit differentiation of GSCs (Ikushima et al., 2009; Peñuelas et al., 2009). Activation receptor NKG2D expressed by NK cells and CD8+T cells plays a role in specific killing of transformed cells. Crane et al. found that GBM could down-regulate NKG2D by secreting TGF-β, thus inhibiting the killing function of NK cells and CD8+ T cells (Crane et al., 2010).
Chemokines play an important role in the local migration of immune cells such as microglias and macrophages to tumors. Studies have shown that the high expression of inflammatory chemokines ligand 1 (CXCL1) and ligand 2 (CXCL2) is closely related to tumor invasion, metastasis and poor prognosis (Miyake et al., 2014; Seifert et al., 2016). CXCR2 is the only receptor for CXCL1 and CXCL2, and is highly expressed mainly in myeloid cells, including neutrophils, monocytes and macrophages. These receptors guide the migration of myeloid derived cells from the bone marrow to tumor regions where CXCL1 and CXCL2 are highly expressed, inhibit the activity and proliferation of effector T cells, and stimulate the growth of regulatory T cells, thereby promoting tumor immune escape (Oppenheim et al., 1991; Gabrilovich et al., 2012). In addition to suppressing immunity, CXCL1 and CXCL2 also recruit myeloid cells to produce paracrine factors such as S100A9 and promote tumor cell survival (Acharyya et al., 2012; Alafate et al., 2020) found that silencing CXCL1 can down-regulate NF-κB and mesenchymal cell transformation, and inhibit the growth of human glioma xenograft (Alafate et al., 2020).
Hu et al. found that the high expression of CXCL1 and CXCL2 was closely related to the invasiveness of glioma. The high expression of CXCL1/CXCL2 promoted the migration of myeloid cells and disrupted the accumulation of CD8+T cells in the tumor microenvironment, leading to accelerated tumor proliferation. Inhibition of CXCL1/2 significantly prevented myeloid inhibitory cell migration and increased CD8+T cell accumulation in vitro and in vivo. CXCL1/2 also promoted the expression of paracrine factor S100A9, activated ERK1/2 and p70S60k, and promoted tumor growth, while blocking CXCL1/2 down-regulated the expression of these pro-survival factors and slowed tumor growth. Targeting CXCL1/2 with standard chemotherapy can improve the chemotherapy efficiency of glioma and prolong the survival of glioma mice (Hu et al., 2021).
Oncostatin M (OSM) is another cytokine with important biological significance. Its main function is to inhibit the growth of various tumor cells and induce the differentiation of some tumor cells. Oncostatin M receptor (OSMR) is widely distributed on the surface of many tumor cells, endothelial cells and epithelial cells. The inhibitory effect of tumor cell growth and differentiation induced by statin M is exerted by specific high-affinity receptors (Auguste et al., 1997; Liu et al., 1998). Previous single-cell RNA sequencing by researchers found that malignant glioma cells had four states: neural progenitor cell-like (NPC-like), oligodendrocyte progenitor cell-like (OPC-like), astrocyte like (AC-like) and mesenchymal like (MES-like), indicating that tumor cells have potential state plasticity (Tanay and Regev, 2017; Neftel et al., 2019). The frequency of the first three states in tumors is affected by some driving genes (such as CDK4, PDGFRA, EGFR, etc.). Mesenchymal tumor cells are almost nonexistent in normal human brain tissue and are associated only with genetic changes, and they have been poorly studied. Previous studies have shown that TCGA-MES subtype is related to the abundance of macrophages, and NF1 mutation or deletion in TCGA -MES rich tumors increases the recruitment of macrophages (Wang et al., 2017). Therefore, TCGA-MES is related to the increased abundance of macrophages in tumors, which may explain its advantage in recurrent diseases (Qzawa et al., 2014; Hara et al., 2021) found that MES status of GBM was closely related to macrophage expression, T cell abundance, and increased cytotoxicity in both the mouse model and GBM globule model. The results of this study show that OSM secreted on macrophages induces the transition of MES like glioma cells to MES like state. In addition, OSM-mediated induction mainly comes from the regulation of STAT3, and MES-like tumor cells are also associated with increased mesenchymal expression of macrophages and increased cytotoxicity of T cells. This study highlights the influence of changes in immune microenvironment on tumor cell state, and the combination of changes in tumor cell state and activation of the immune system has a good potential therapeutic effect.
The local aggregation of the above immune cell subsets and cytokines in glioma fully demonstrates that they jointly form an immunosuppressive microenvironment in glioma and hinder the production of anti-tumor immune response. Therefore, how to reduce or reverse this state of inhibition, will continue to receive attention.
Tumor stem cells account for a small proportion of tumor cells, and have the biological characteristics of self-renewal, multilineage differentiation and tolerance to conventional therapy. GSCs are the cells that play a tumor initiation role in glioma (Sundar et al., 2014). With high heterogeneity, they can induce tumor angiogenesis, promote tumor invasion and dissemination, and are highly tolerant to radiotherapy and chemotherapy (Folkins et al., 2007). Under the pressure of conventional treatment, they can rapidly rebuild tumors, leading to rapid recurrence of glioma (Zhi et al., 2010). The surface of GSCs can express different proteins, which are closely related to the maintenance of their homeostasis. These cell surface proteins are ideal markers for screening and targeting GSCs, among which CD133 is the most classic (Sundar et al., 2014). Other well-studied surface markers include SOX2, KLF4, C-myC, Nestin, Oct4 and A2B5, etc. Early studies targeted GSCs by preparing specific monoclonal antibodies targeting these markers. Cells in the GSCs microenvironment can secret a variety of cytokines, such as vascular endothelial growth factor (VEGF), hypoxia-inducing factor (HIF), and fibroblast growth factor 2 (FGF2), to stimulate GSCs to self-renew, induce angiogenesis, recruit immune cells, and promote tumor cell invasion and metastasis (Hambardzumyan and Bergers, 2015).
Oncolytic viruses (OVs) are therapeutics designed to selectively multiply and kill tumor cells. An important design principle is to weaken or delete virulence factors so that the oncolytic virus cannot replicate in normal tissues, but still retain the ability to replicate and kill tumor cells in tumor cells, at the same time it also can stimulate the immune response, attract more immune cells to continue to kill residual cancer cells (Muik et al., 2014; Fukuhara et al., 2016). However, the ability of oncolytic viruses to spread, cross the host tumor and penetrate the blood-brain barrier is very limited, which limits their application in glioma. Neural Stem Cells (NSCs) are pluripotent progenitor Cells derived from the developing and adult central nervous system (Conti and Cattaneo, 2010). Preclinical experiments have shown that NSC can cross the blood-brain barrier, reach the tumor region, surround the tumor boundary, and migrate within the brain to target glioma cells (Aboody et al., 2000). The ability of NSCs to migrate and spread freely within tumors can be used to deliver therapeutic molecules across the blood-brain barrier to target glioma cells. Kim et al. modified an oncolytic adenovirus (CRAd-S-pk7), which improved viral replication and targeting to glioma cells, and increased the anti-tumor activity and survival rate of mice (Kim et al., 2016). By combining the tumor propensity of NSCs with the ability of CRAd-S-pk7 to target GSCs, they engineered an NSC-provided engineered oncolytic virus (NSC-CRAd-S-pk7), which extended the median survival of mice treated with it by 50%. In a recently published phase one trial, NSC-CRAd-S-pk7 injection was shown to be safe and effective in patients with newly diagnosed GBM during surgery (Fares et al., 2021). The results also suggest that multi-site injection in the brain can increase virus coverage and improve treatment efficiency, and that concurrent use of chemoradiotherapy can enhance the replication capacity of oncolytic adenovirus, which is expected to further improve treatment effectiveness. Neurotropic viruses as oncolytic viruses in the treatment of brain tumors have become the focus of attention because of their ability to cross the blood-brain barrier. The Zika virus (ZIKV) outbreak in 2015 became a global public health emergency. This latest study shows that ZIKA virus can target glioblastoma stem cells through the SOX2-integrin αVβ5 axis to exert antitumor effect (Zhu et al., 2020). They found that ZIKV was more likely to infect GSCs from the patient’s source. At the same time, ZIKV could significantly induce apoptosis and inhibit proliferation of GSCs. SOX2 is an important regulator of GSCs with high expression, which can induce cell pluripotency and maintain the characteristics of stem cells. After knocking down SOX2, GSCs are less susceptible to ZIKV infection. Integrin αVβ5 was associated with the expression of SOX2 and other GSC markers. Inhibition of αVβ5 significantly reduced viral infection and inhibited the effect of ZIKV on neuroglobulogenesis. Integrin αVβ5 plays an important role in ZIKV infection and cell damage. Therefore, the results of this study confirmed the oncolytic activity of ZIKV against GSCs and correlated with αVβ5 expression. Friedman et al. found that the modified HSV-1 oncolytic virus (G207) is highly sensitive to treating high-grade gliomas in children. The results showed that G207 could transform immunologically “cold” tumors into “hot” tumors (Friedman et al., 2021). In addition, because the GSCs are highly enriched in integrins such as αVβ3 or αVβ5, the oncolytic viruses designed by genetic engineering technology can enter the glioma stem cells through these integrins and play an anti-tumor role, of which DNX⁃2401 is an oncolytic virus with tumor specificity and strong infectivity. It has been used in phase I clinical trials with good safety, significantly reduced tumor volume and prolonged patient survival (Jiang et al., 2007; Lang et al., 2018).
Tumor vaccines can activate the host immune system to recognize and kill tumor cells, which is an active immunotherapy. The best way to activate the immune system is to stimulate multifunctional antigen presenting cells (APC), such as DC, macrophages, and B lymphocytes (B cells), among which DC is the best choice. GSCs have the same ability to sensitize DC, so the direct targeting of this tumor cell subpopulation and the establishment of tumor vaccines against glioma are expected to be effective therapeutic programs (Finocchiaro and Pellegatta, 2015). In recent years, SOX2, as an important transcription factor for maintaining glioma stem cells, has been considered as a new target for active immunotherapy. SOX2 peptide vaccine can significantly enhance systemic and local immune responses, and prolong the survival of tumor-bearing animals in tumor models, with definite efficacy observed regardless of whether combined with chemotherapy (Favaro et al., 2014). Therefore, glioma stem cell-related vaccines targeting SOX2 and other glioma stem cell-related genes may provide a new regimen for active immunotherapy. Epidermal growth factor receptor variant III (EGFRvIII), the most common EGFR gene specific mutation in glioma and GSCs. In recent glioma studies, it has been found that the EGFRvIII resulting from the in-frame deletion of the EGF receptor gene is not only overexpressed and carcinogenic, but also exists in tumor stem cells with high immunogenicity. Therefore, it has great potential as a target for immunotherapy. As a synthetic peptide that can specifically recognize EGFRvIII, pepVIII has shown a good application prospect in the test of glioma. In particular, in the latest glioma study, a variant peptide vaccine (Y6-pepVIII) designed by the researchers was found to significantly enhance the immune response and improve survival in mice (Fidanza et al., 2021). Their results showed an increased proportion of CD8+ T cells in tumors of mice receiving Y6-pepVIII vaccine, and both CD4+ and CD8+ T subsets were involved in antitumor responses.
Monoclonal antibodies (Mabs) play an important role in tumor immunotherapy due to their direct cell-killing and immunomodulatory effects (Guo et al., 2011). Previous clinical studies on anti-EGFR antibody in glioma have found that antibody-mediated therapy has a potential application prospect (Herbst and Shin, 2002). However, bevacizumab, the first monoclonal antibody against endothelial production factor (VEGF) approved by the US Food and Drug Administration (FDA) for the treatment of high-grade gliomas, has significant anti-tumor angiogenesis. It will greatly improve the killing and inhibition of glioma stem cells by other therapies (Calabrese et al., 2007), but interestingly, no significant survival benefit has been observed in subsequent clinical trials (Zhuang et al., 2016). Recent studies have found that Semaphorin3A (SEMA3A), as an axon guide factor, has carcinogenic effect in several cancers (Kigel et al., 2008). Lee et al. found that SEMA3A was highly expressed in human glioma specimens. The SEMA3A antibody developed by them significantly inhibited the migration and proliferation of GBM patient-derived cells and U87-MG cells in vitro, and inhibited tumor by down-regulating cell proliferation dynamics and tumor-associated macrophage recruitment in PDX model (Lee et al., 2018). These different findings need to be further explored.
Recent studies have shown that there is a mechanism in the immune microenvironment of tumor stem cells that inhibits the immune response and interferes with the surveillance role of the immune system. Immune checkpoints play a key role in the immunosuppressed tumor stem cell microenvironment, and the intervention of these immune checkpoints has become an important target of tumor immunotherapy (Pardoll and Drew, 2012; Topalian et al., 2015). During tumorigenesis, the immune checkpoint interaction between GSCs and immune cells changes from stimulating to inhibiting (Zhai et al., 2020).
The most representative immune checkpoints are cytotoxic T lymphocyte-associated protein antigen-4 (CTLA-4) and programmed cell death-1 (PD-1) (Buchbinder and Desai, 2016). CTLA-4 is a negative regulator of T cells and has a high affinity with T cells, which leads to depletion and inhibits their activation (Four et al., 2016; Chikuma, 2017). Anti-CTLA-4 treatment can remove the inhibitory effect of T cells, thus supporting the anti-tumor immune response (Fecci et al., 2014). PD-1 is expressed in various immune cells (Alsaab et al., 2017) and is highly expressed in malignant tumor tissues, which is associated with T cell depletion (Ohaegbulam et al., 2015). The interaction between CTLA-4 and PD-1 significantly inhibited the secretion of IFN⁃γ by activated T cells and impaired the function of T cells (Que et al., 2017). Therefore, joint blocking of CTLA-4 and PD-1 signal transduction pathway may be an effective way to rescue immunosuppression of malignant tumors including glioma and maintain anti-tumor immune response (Castro et al., 2014). Microrna (miRNA) regulate multiple gene transcripts and may involve multiple immune checkpoints, so they have potential as immunotherapies. Wei et al. found that mir-138 could bind the 3′ untranslated regions of CTLA-4 and PD-1, and mir-138 transfected human CD4+ T cells could inhibit the expression of CTLA-4 and PD-1 in transfected human CD4+ T cells (Wei et al., 2016). Immune checkpoint inhibitors that block the molecule CTLA-4 (2011) and PD-1 (2014) have been approved by the FDA. Among the immunotherapy drugs against PD-1 antibody, Nivolumab has attracted the most attention. In clinical efficacy observation, it was found that combined radiotherapy, chemotherapy and electric field therapy can improve the survival of some glioma patients to a certain extent (Preusser et al., 2015), improve prognosis and reduce adverse drug reactions (Chandran et al., 2017).
In addition, GSCs reduce T cell cognition and evade systemic immune monitoring by downregulation or defect of major histocompatibility complex I (MHC-I) molecules and antigen-processing mechanism (APM) components. Improving tumor surface antigens of GSCs may be an effective strategy for triggering adaptive immune responses and activating cytotoxic T cells (CTLs) to inhibit gliomas. A new research found that inhibition of histone deacetylase (HDAC) increased the expression of MHC-I and APM components, and enhanced the antitumor effect of tumor lysate vaccine by activating the destruction of CTLs (Yang et al., 2020). The enhanced T cell immune response induced by HDAC inhibition may provide a new direction for targeting GSCs-based immunotherapy.
GSCs and glioma immune microenvironment are the key factors in the development and progression of glioma. The complex multidirectional interactions between GSCs, various immune cells and cytokines eventually form a tumor-supporting immune microenvironment that promotes tumorigenesis, proliferation and invasion initiated by GSCs. The study on the immunosuppression mechanism of GSCs and glioma microenvironment will help us to further explore the immunotherapy strategies targeting GSCs and point out new directions for establishing effective therapeutic targets. We always believe that immunotherapy is the “ultimate killer” of glioma. Oncolytic virus immunotherapy targeting GSCs is the blade of this “sharp weapon” (Figure 1). In the context of the global COVID-19 pandemic, while we conquer it, its research in tumor and even glioma is worth paying close attention and thinking.
FIGURE 1. Schematic diagram of a simple simulation of the mechanism by which oncolytic viruses (OVs) targeting glioma stem cells (GSCs) exert glioma-inhibiting action. GSCs infected by genetically engineered oncolytic viruses that target dry-associated genes (e.g., SOX2-integrin αVβ5 axis) can be cleaved directly to destroy GSCs, and additional immune cells are recruited and chemotaxis around gliomas through the release of inflammatory factors to kill and inhibit gliomas.
XW and LZ initiated the work, designed the idea. XY, TR, and XW prepared and collected material and data. XL, ML, and JZ wrote the paper. All authors reviewed the article. All authors read and approved the final authors.
This work is supported by grants from Liaoning Provincial Natural Science Foundation of China (No.2019-BS-056), Science and Technology Innovation Fund Project of Dalian (No.2021JJ13SN55), and the Youth Scientific Research guiding Project of Fujian Provincial Health Commission (No.2018-ZQN-80).
The authors declare that the research was conducted in the absence of any commercial or financial relationships that could be construed as a potential conflict of interest.
All claims expressed in this article are solely those of the authors and do not necessarily represent those of their affiliated organizations, or those of the publisher, the editors and the reviewers. Any product that may be evaluated in this article, or claim that may be made by its manufacturer, is not guaranteed or endorsed by the publisher.
Aboody, K. S., Brown, A., Rainov, N. G., Bower, K. A., and Liu, S. (2000). Neural Stem Cells Display Extensive Tropism for Pathology in Adult Brain: Evidence from Intracranial Gliomas. Proc. Natl. Acad. Sci. 97 (23), 12846–12851. doi:10.1073/pnas.97.23.12846
Acharyya, S., Oskarsson, T., Vanharanta, S., Malladi, S., Kim, J., Morris, P., et al. (2012). A CXCL1 Paracrine Network Links Cancer Chemoresistance and Metastasis. Cell 150 (1), 165–178. doi:10.1016/j.cell.2012.04.042
Agarwal, S., Sane, R., Oberoi, R., Ohlfest, J. R., and Elmquist, W. F. (2011). Delivery of Molecularly Targeted Therapy to Malignant Glioma, a Disease of the Whole Brain. Expert Rev. Mol. Med. 13, e17. doi:10.1017/S1462399411001888
Akkari, L., Bowman, R., Tessier, J., Klemm, F., Handgraaf, S., de Groot, M., et al. (2020). Dynamic Changes in Glioma Macrophage Populations after Radiotherapy Reveal CSF-1R Inhibition as a Strategy to Overcome Resistance. Sci. Transl. Med. 12 (552), eaaw7843. doi:10.1126/scitranslmed.aaw7843
Alafate, W., Li, X., Zuo, J., Zhang, H., Xiang, J., Wu, W., et al. (2020). Elevation of CXCL1 Indicates Poor Prognosis and Radioresistance by Inducing Mesenchymal Transition in Glioblastoma. CNS Neurosci. Ther. 26 (4), 475–485. doi:10.1111/cns.13297
Aldemir, H., Prod'homme, V., Dumaurier, M., Retiere, C., Poupon, G., Cazareth, J., et al. (2005). Cutting Edge: Lectin-like Transcript 1 Is a Ligand for the CD161 Receptor. J. Immunol. 175 (12), 7791–7795. doi:10.4049/jimmunol.175.12.7791
Alsaab, H. O., Samaresh, S., Rami, A., Katyayani, T., Ketki, B., Kashaw, S. K., et al. (2017). PD-1 and PD-L1 Checkpoint Signaling Inhibition for Cancer Immunotherapy: Mechanism, Combinations, and Clinical Outcome. Front. Pharmacol. 8, 561. doi:10.3389/fphar.2017.00561
Auguste, P., Guillet, C., Fourcin, M., Olivier, C., Veziers, J., Pouplard-Barthelaix, A., et al. (1997). Signaling of Type II Oncostatin M Receptor. J. Biol. Chem. 272 (25), 15760–15764. doi:10.1074/jbc.272.25.15760
Beppu, K., Kosai, Y., Kido, M., Akimoto, N., Mori, Y., Kojima, Y., et al. (2013). Expression, Subunit Composition, and Function of AMPA-type Glutamate Receptors Are Changed in Activated Microglia; Possible Contribution of GluA2 (GluR-B)-Deficiency under Pathological Conditions. Glia 61 (6), 881–891. doi:10.1002/glia.22481
Buchbinder, E., and Desai, A. (2016). CTLA-4 and PD-1 Pathways: Similarities, Differences, and Implications of Their Inhibition. Am. J. Clin. Oncol. 39 (1), 98–106. doi:10.1097/COC.0000000000000239
Calabrese, C., Poppleton, H., Kocak, M., Hogg, T. L., Fuller, C., Hamner, B., et al. (2007). A Perivascular Niche for Brain Tumor Stem Cells. Cancer Cell 11 (1), 69–82. doi:10.1016/j.ccr.2006.11.020
Câmara, N. O., Sebille, F., and Lechler, R. I. (2003). Human CD4+CD25+ Regulatory Cells Have Marked and Sustained Effects on CD8+ T Cell Activation. Eur. J. Immunol. 33 (12), 3473–3483. doi:10.1002/eji.200323966
Cantini, G., Pisati, F., Mastropietro, A., Frattini, V., Iwakura, Y., Finocchiaro, G., et al. (2011). A Critical Role for Regulatory T Cells in Driving Cytokine Profiles of Th17 Cells and Their Modulation of Glioma Microenvironment. Cancer Immunol. Immunother. 60 (12), 1739–1750. doi:10.1007/s00262-011-1069-4
Caponegro, M., Oh, K., Madeira, M., Radin, D., and Tsirka, S. E. (2020). A Distinct Microglial Subset at the Tumor-Stroma Interface of Glioma. Glia 69, 176. doi:10.1002/glia.23991
Castriconi, R., Daga, A., Dondero, A., Zona, G., Poliani, P. L., Melotti, A., et al. (2009). NK Cells Recognize and Kill Human Glioblastoma Cells with Stem Cell-like Properties. J. Immunol. 182 (6), 3530–3539. doi:10.4049/jimmunol.0802845
Castro, M., Baker, G., and Lowenstein, P. (2014). Blocking Immunosuppressive Checkpoints for Glioma Therapy: the More the Merrier! Clin. Cancer Res. 20 (20), 5147–5149. doi:10.1158/1078-0432.CCR-14-0820
Chandran, M., Candolfi, M., Shah, D., Mineharu, Y., Yadav, V., Koschmann, C., et al. (2017). Single vs. Combination Immunotherapeutic Strategies for Glioma. Expert Opin. Biol. Ther. 17 (5), 543–554. doi:10.1080/14712598.2017.1305353
Chang, A., Miska, J., Wainwright, D., Dey, M., Rivetta, C., Yu, D., et al. (2016). CCL2 Produced by the Glioma Microenvironment Is Essential for the Recruitment of Regulatory T Cells and Myeloid-Derived Suppressor Cells. Cancer Res. 76 (19), 5671–5682. doi:10.1158/0008-5472.CAN-16-0144
Chikuma, S. (2017). CTLA-4, an Essential Immune-Checkpoint for T-Cell Activation: Emerging Concepts Targeting Immune Checkpoints in Cancer and Autoimmunity. Berlin: Springer Verlag.
Conti, L., and Cattaneo, E. (2010). Neural Stem Cell Systems: Physiological Players or In Vitro Entities? Nat. Rev. Neurosci. 11 (11), 176–187. doi:10.1038/nrn2761
Crane, C., Han, S., Barry, J., Ahn, B., Lanier, L., and Parsa, A. (2010). TGF-beta Downregulates the Activating Receptor NKG2D on NK Cells and CD8+ T Cells in Glioma Patients. Neuro Oncol. 12 (1), 7–13. doi:10.1093/neuonc/nop009
Dix, A. R., Brooks, W. H., Roszman, T. L., and Morford, L. A. (1999). Immune Defects Observed in Patients with Primary Malignant Brain Tumors. J. Neuroimmunol. 100 (1-2), 216–232. doi:10.1016/s0165-5728(99)00203-9
Eder, K., and Kalman, B. (2014). Molecular Heterogeneity of Glioblastoma and its Clinical Relevance. Pathol. Oncol. Res. 20 (4), 777–787. doi:10.1007/s12253-014-9833-3
Ellert-Miklaszewska, A., Dabrowski, M., Lipko, M., Sliwa, M., Maleszewska, M., and Kaminska, B. (2013). Molecular Definition of the Pro-tumorigenic Phenotype of Glioma-Activated Microglia. Glia 61 (7), 1178–1190. doi:10.1002/glia.22510
Fares, J., Ahmed, A., Ulasov, I., Sonabend, A., Miska, J., Lee-Chang, C., et al. (2021). Neural Stem Cell Delivery of an Oncolytic Adenovirus in Newly Diagnosed Malignant Glioma: a First-In-Human, Phase 1, Dose-Escalation Trial. Lancet Oncol. 22, 1103. doi:10.1016/s1470-2045(21)00245-x
Favaro, R., Appolloni, P., Malatesta, F., Gambini, O., Finocchiaro, F., and Nicolis, S. K. (2014). Sox2 Is Required to Maintain Cancer Stem Cells in a Mouse Model of High-Grade Oligodendroglioma. Cancer Res. 74 (6), 1833–1844. doi:10.1158/0008-5472.CAN-13-1942
Fecci, P. E., Heimberger, A., and Sampson, J. H. (2014). Immunotherapy for Primary Brain Tumors: No Longer a Matter of Privilege. Clin. Cancer Res. 20 (22), 5620–5629. doi:10.1158/1078-0432.CCR-14-0832
Fidanza, M., Gupta, P., Sayana, A., Shanker, V., Pahlke, S., Vu, B., et al. (2021). Enhancing Proteasomal Processing Improves Survival for a Peptide Vaccine Used to Treat Glioblastoma. Sci. Transl. Med. 13 (598), eaax4100. doi:10.1126/scitranslmed.aax4100
Finocchiaro, G., and Pellegatta, S. (2015). Immunotherapy with Dendritic Cells Loaded with Glioblastoma Stem Cells: from Preclinical to Clinical Studies. Cancer Immunol. Immunother. 65 (1), 1–9. doi:10.1007/s00262-015-1754-9
Folkins, C., Man, S., Xu, P., Shake D, Y., Hicklin, D. J., and Kerbel, R. S. (2007). Anticancer Therapies Combining Antiangiogenic and Tumor Cell Cytotoxic Effects Reduce the Tumor Stem-like Cell Fraction in Glioma Xenograft Tumors. Cancer Res. 67 (8), 3560. doi:10.1158/0008-5472.CAN-06-4238
Four, S. D., Maenhout, S. K., Niclou, S. P., Thielemans, K., and Aerts, J. L. (2016). Combined VEGFR and CTLA-4 Blockade Increases the Antigen-Presenting Function of Intratumoral DCs and Reduces the Suppressive Capacity of Intratumoral MDSCs. Am. J. Cancer Res. 6 (11), 2514–2531.
Friedman, G., Johnston, J., Bag, A., Bernstock, J., Li, R., Aban, I., et al. (2021). Oncolytic HSV-1 G207 Immunovirotherapy for Pediatric High-Grade Gliomas. New Engl. J. Med. 384 (17), 1613–1622. doi:10.1056/NEJMoa2024947
Fujita, M., Kohanbash, G., Fellows-Mayle, W., Hamilton, R. L., and Okada, H. (2011). COX-2 Blockade Suppresses Gliomagenesis by Inhibiting Myeloid-Derived Suppressor Cells. Cancer Res. 71 (7), 2664. doi:10.1158/0008-5472.CAN-10-3055
Fukuhara, H., Ino, Y., and Todo, T. (2016). Oncolytic Virus Therapy: A new era of Cancer Treatment at Dawn. Cancer Sci. 107 (10), 1373–1379. doi:10.1111/cas.13027
Gabrilovich, D. I., Ostrand-Rosenberg, S., and Bronte, V. (2012). Coordinated Regulation of Myeloid Cells by Tumours. Nat. Rev. Immunol. 12 (4), 253–268. doi:10.1038/nri3175
Garber, S., Hashimoto, Y., Weathers, S., Xiu, J., Gatalica, Z., Verhaak, R., et al. (2016). Immune Checkpoint Blockade as a Potential Therapeutic Target: Surveying CNS Malignancies. Neuro Oncol. 18 (10), 1357–1366. doi:10.1093/neuonc/now132
Grabowski, M., Sankey, E., Ryan, K., Chongsathidkiet, P., Lorrey, S., Wilkinson, D., et al. (2021). Immune Suppression in Gliomas. J. Neurooncol. 151 (1), 3–12. doi:10.1007/s11060-020-03483-y
Guo, J. L., Nagarajah, R., Banati, R. B., and Bennett, M. R. (2009). Glutamate Induces Directed Chemotaxis of Microglia. Eur. J. Neurosci. 29 (6), 1108–1118. doi:10.1111/j.1460-9568.2009.06659.x
Guo, Y. T., Hou, Q. Y., and Wang, N. (2011). Monoclonal Antibodies in Cancer Therapy. Clin. Oncol. Cancer Res. English Edition 2 (4), 215–219. doi:10.1007/s11805-011-0583-7
Hambardzumyan, D., and Bergers, G. (2015). Glioblastoma: Defining Tumor Niches. Trends Cancer 1 (4), 252–265. doi:10.1016/j.trecan.2015.10.009
Hambardzumyan, D., Gutmann, D. H., and Kettenmann, H. (2015). The Role of Microglia and Macrophages in Glioma Maintenance and Progression. Nat. Neurosci. 19 (1), 20. doi:10.1038/nn.4185
Hao, J., Campagnolo, D., Liu, R., Piao, W., Shi, S., Hu, B., et al. (2011). Interleukin‐2/interleukin‐2 Antibody Therapy Induces Target Organ Natural Killer Cells that Inhibit central Nervous System Inflammation. Ann. Neurol. 69 (4), 721. doi:10.1002/ana.22339
Hara, T., Chanoch-Myers, R., Mathewson, N. D., Myskiw, C., and Tirosh, I. (2021). Interactions between Cancer Cells and Immune Cells Drive Transitions to Mesenchymal-like States in Glioblastoma. Cancer Cell 39, 779. doi:10.1016/j.ccell.2021.05.002
Heimberger, A. B., Abou-Ghazal, M., Reina-Ortiz, C., Yang, D. S., Sun, W., Qiao, W., et al. (2015). Incidence and Prognostic Impact of FoxP3+ Regulatory T Cells in Human Gliomas. Clin. Cancer Res. 14 (16), 5166–5172. doi:10.1158/1078-0432.CCR-08-0320
Herbst, R., and Shin, D. (2002). Monoclonal Antibodies to Target Epidermal Growth Factor Receptor-Positive Tumors: a New Paradigm for Cancer Therapy. Cancer 94 (5), 1593–1611. doi:10.1002/cncr.10372
Hodges, T., Ott, M., Xiu, J., Gatalica, Z., Swensen, J., Zhou, S., et al. (2017). Mutational burden, Immune Checkpoint Expression, and Mismatch Repair in Glioma: Implications for Immune Checkpoint Immunotherapy. Neuro Oncol. 19 (8), 1047–1057. doi:10.1093/neuonc/nox026
Hu, J., Zhao, Q., Kong, L., Wang, J., Yan, J., Xia, X., et al. (2021). Regulation of Tumor Immune Suppression and Cancer Cell Survival by CXCL1/2 Elevation in Glioblastoma Multiforme. Sci. Adv. 7 (5), eabc2511. doi:10.1126/sciadv.abc2511
Ikushima, H., Todo, T., Ino, Y., Takahashi, M., Miyazawa, K., and Miyazono, K. (2009). Autocrine TGF-Beta Signaling Maintains Tumorigenicity of Glioma-Initiating Cells through Sry-Related HMG-Box Factors. Cell Stem cell 5 (5), 504–514. doi:10.1016/j.stem.2009.08.018
Jiang, H., Candelaria, G. M., Hiroshi, A., Alonso, M. M., Seiji, K., Frank, M. C., et al. (2007). Examination of the Therapeutic Potential of Delta-24-RGD in Brain Tumor Stem Cells: Role of Autophagic Cell Death. J. Natl. Cancer Inst. 18, 1410–1414. doi:10.1093/jnci/djm102
Kigel, B., Varshavsky, A., Kessler, O., and Neufeld, G. (2008). Successful Inhibition of Tumor Development by Specific Class-3 Semaphorins Is Associated with Expression of Appropriate Semaphorin Receptors by Tumor Cells. PloS one 3 (9), e3287. doi:10.1371/journal.pone.0003287
Kim, J. W., Auffinger, B., Spencer, D. A., Miska, J., Chang, A. L., Kane, J. R., et al. (2016). Single Dose GLP Toxicity and Biodistribution Study of a Conditionally Replicative Adenovirus Vector, CRAd-S-Pk7, Administered by Intracerebral Injection to Syrian Hamsters. J. Transl. Med. 14 (1), 134. doi:10.1186/s12967-016-0895-8
Lang, F. F., Conrad, C., Gomez-Manzano, C., Yung, W. K. A., Sawaya, R., Weinberg, J. S., et al. (2018). Phase I Study of DNX-2401 (Delta-24-RGD) Oncolytic Adenovirus: Replication and Immunotherapeutic Effects in Recurrent Malignant Glioma. J. Clin. Oncol. 36 (14), 1419. doi:10.1200/JCO.2017.75.8219
Laterra, J., Ma, T., Hu, C., Lal, B., Li, Y., Zhou, W., et al. (2021). Reprogramming Transcription Factors Oct4 and Sox2 Induce a BRD-dependent Immunosuppressive Transcriptome in GBM-Propagating Cells. Cancer Res. 81, 2457. doi:10.1158/0008-5472.CAN-20-2489
Lee, J., Shin, Y., Lee, K., Cho, H., Sa, J., Lee, S., et al. (2018). Anti-SEMA3A Antibody: A Novel Therapeutic Agent to Suppress Glioblastoma Tumor Growth. Cancer Res. Treat. 50 (3), 1009–1022. doi:10.4143/crt.2017.315
Liu, H., Leak, R. K., and Hu, X. (2016). Neurotransmitter Receptors on Microglia. Stroke Vasc. Neurol. 1 (2), 52–58. doi:10.1136/svn-2016-000012
Liu, J., Hadjokas, N., Mosley, B., Estrov, Z., Spence, M., and Vestal, R. (1998). Oncostatin M-specific Receptor Expression and Function in Regulating Cell Proliferation of normal and Malignant Mammary Epithelial Cells. Cytokine 10 (4), 295–302. doi:10.1006/cyto.1997.0283
Liu, S., Zhang, C., Wang, B., Zhang, H., Qin, G., Li, C., et al. (2021). Regulatory T Cells Promote Glioma Cell Stemness through TGF-β-NF-κB-IL6-STAT3 Signaling. Cancer Immunol. Immunother. 70, 2601. doi:10.1007/s00262-021-02872-0
Louveau, A., Smirnov, I., Keyes, T. J., Eccles, J. D., Rouhani, S. J., Peske, J. D., et al. (2016). Structural and Functional Features of central Nervous System Lymphatic Vessels (Vol 523, Pg 337, 2015). Nature 533, 278. doi:10.1038/nature16999
Mccoy, K. D., and Gros, G. L. (1999). The Role of CTLA‐4 in the Regulation of T Cell Immune Responses. Immunol. Cel Biol. 77 (1), 1–10. doi:10.1046/j.1440-1711.1999.00795.x
Miyake, M., Lawton, A., Goodison, S., Urquidi, V., and Rosser, C. J. (2014). Chemokine (C-X-C Motif) Ligand 1 (CXCL1) Protein Expression Is Increased in High-Grade Prostate Cancer. Pathol. Res. Pract. 210 (2), 74–78. doi:10.1016/j.prp.2013.08.013
Miyamoto, A., Wake, H., Ishikawa, A. W., Eto, K., Shibata, K., Murakoshi, H., et al. (2016). Microglia Contact Induces Synapse Formation in Developing Somatosensory Cortex. Nat. Commun. 7, 12540. doi:10.1038/ncomms12540
Muik, A., Stubbert, L. J., Jahedi, R. Z., Geiss, Y., Kimpel, J., Dold, C., et al. (2014). Re-engineering Vesicular Stomatitis Virus to Abrogate Neurotoxicity, Circumvent Humoral Immunity, and Enhance Oncolytic Potency. Cancer Res. 50 (13), S199. doi:10.1158/0008-5472.CAN-13-3306
Neftel, C., Laffy, J., Filbin, M., Hara, T., Shore, M., Rahme, G., et al. (2019). An Integrative Model of Cellular States, Plasticity, and Genetics for Glioblastoma. Cell 178 (4), 835–849. doi:10.1016/j.cell.2019.06.024
Ohaegbulam, K. C., Assal, A., Lazar-Molnar, E., Yao, Y., and Zang, X. (2015). Human Cancer Immunotherapy with Antibodies to the PD-1 and PD-L1 Pathway. Trends Mol. Med. 21 (1), 24–33. doi:10.1016/j.molmed.2014.10.009
Oppenheim, J., Zachariae, C., Mukaida, N., and Matsushima, K. (1991). Properties of the Novel Proinflammatory Supergene "intercrine" Cytokine Family. Annu. Rev. Immunol. 9, 617–648. doi:10.1146/annurev.iy.09.040191.003153
Orozco-Morales, M., Sánchez-García, F., Golán-Cancela, I., Hernández-Pedro, N., Costoya, J., de la Cruz, V., et al. (2015). RB Mutation and RAS Overexpression Induce Resistance to NK Cell-Mediated Cytotoxicity in Glioma Cells. Cancer Cel. Int. 15, 57. doi:10.1186/s12935-015-0209-x
Ostrom, Q., Gittleman, H., Fulop, J., Liu, M., Blanda, R., Kromer, C., et al. (2015). CBTRUS Statistical Report: Primary Brain and Central Nervous System Tumors Diagnosed in the United States in 2008-2012. Neuro Oncol. 17, iv1–iv62. doi:10.1093/neuonc/nov189
Ozawa, T., Riester, M., Cheng, Y., Huse, J., Squatrito, M., Helmy, K., et al. (2014). Most Human Non-GCIMP Glioblastoma Subtypes Evolve from a Common Proneural-like Precursor Glioma. Cancer Cell 26 (2), 288–300. doi:10.1016/j.ccr.2014.06.005
Pardolland, , , and Drew, M. (2012). The Blockade of Immune Checkpoints in Cancer Immunotherapy. Nat. Rev. Cancer 12 (4), 252–264. doi:10.1038/nrc3239
Parkhurst, C. N., Yang, G., Ninan, I., Savas, J. N., Yates, J. R., Lafaille, J. J., et al. (2013). Microglia Promote Learning-dependent Synapse Formation through Brain-Derived Neurotrophic Factor. Cell 155 (7), 1596–1609. doi:10.1016/j.cell.2013.11.030
Peñuelas, S., Anido, J., Prieto-Sánchez, R., Folch, G., Barba, I., Cuartas, I., et al. (2009). TGF-beta Increases Glioma-Initiating Cell Self-Renewal through the Induction of LIF in Human Glioblastoma. Cancer Cell 15 (4), 315–327. doi:10.1016/j.ccr.2009.02.011
Preusser, M., Lim, M., Hafler, D., Reardon, D., and Sampson, J. (2015). Prospects of Immune Checkpoint Modulators in the Treatment of Glioblastoma. Nat. Rev. Neurol. 11 (9), 504–514. doi:10.1038/nrneurol.2015.139
Pyonteck, S. M., Akkari, L., Schuhmacher, A. J., Bowman, R. L., Sevenich, L., Quail, D. F., et al. (2013). CSF-1R Inhibition Alters Macrophage Polarization and Blocks Glioma Progression. Nat. Med. 19 (10), 1264. doi:10.1038/nm.3337
Que, Y., Xiao, W., Guan, Y. X., Liang, Y., Yan, S. M., Chen, H. Y., et al. (2017). PD-L1 Expression Is Associated with FOXP3+ Regulatory T-Cell Infiltration of Soft Tissue Sarcoma and Poor Patient Prognosis. J. Cancer 8 (11), 2018. doi:10.7150/jca.18683
Radin, D. P., and Tsirka, S. E. (2020). Interactions between Tumor Cells, Neurons, and Microglia in the Glioma Microenvironment. Int. J. Mol. Sci. 21 (22), 1–17. doi:10.3390/ijms21228476
Rodrigues, J. C., Gonzalez, G. C., Lei, Z., Ibrahim, G., Kelly, J. J., Gustafson, M. P., et al. (2010). Normal Human Monocytes Exposed to Glioma Cells Acquire Myeloid-Derived Suppressor Cell-like Properties. Neuro Oncol. 12 (4), 351. doi:10.1093/neuonc/nop023
Seifert, L., Werba, G., Tiwari, S., Giao Ly, N., Alothman, S., Alqunaibit, D., et al. (2016). The Necrosome Promotes Pancreatic Oncogenesis via CXCL1 and Mincle-Induced Immune Suppression. Nature 532 (7598), 245–249. doi:10.1038/nature17403
Shen, X., Burguillos, M., Osman, A., Frijhoff, J., Carrillo-Jiménez, A., Kanatani, S., et al. (2016). Glioma-induced Inhibition of Caspase-3 in Microglia Promotes a Tumor-Supportive Phenotype. Nat. Immunol. 17 (11), 1282–1290. doi:10.1038/ni.3545
Sica, A., and Bronte, V. (2007). Altered Macrophage Differentiation and Immune Dysfunction in Tumor Development. J. Clin. Invest. 117 (5), 1156–1166. doi:10.1172/JCI31422
Singh, S. K., Hawkins, C., Clarke, I. D., Squire, J. A., Bayani, J., Hide, T., et al. (2004). Identification of Human Brain Tumour Initiating Cells. Nature 432 (7015), 396–401. doi:10.1038/nature03128
So-Hee, L., Eunha, P., You, B., Youngseob, J., A-Reum, P., Goo, P. S., et al. (2013). Neuronal Synapse Formation Induced by Microglia and Interleukin 10. Plos One 8 (11), 65. doi:10.1371/journal.pone.0081218
Sun, L., Liang, H., Yu, W., and Jin, X. (2019). Increased Invasive Phenotype of CSF-1R Expression in Glioma Cells via the ERK1/2 Signaling Pathway. Cancer Gene Ther. 26, 136–144. doi:10.1038/s41417-018-0053-y
Sundar, S. J., Hsieh, J. K., Manjila, S., Lathia, J. D., and Sloan, A. (2014). The Role of Cancer Stem Cells in Glioblastoma. Neurosurg. Focus 37 (6), E6. doi:10.3171/2014.9.FOCUS14494
Tanay, A., and Regev, A. (2017). Scaling Single-Cell Genomics from Phenomenology to Mechanism. Nature 541 (7637), 331–338. doi:10.1038/nature21350
Topalian, S., Drake, C., and Pardoll, D. (2015). Immune Checkpoint Blockade: a Common Denominator Approach to Cancer Therapy. Cancer Cell 27 (4), 450. doi:10.1016/j.ccell.2015.03.001
Tran, T., Uhl, M., Ma, J., Janssen, L., Sriram, V., Aulwurm, S., et al. (2007). Inhibiting TGF-Beta Signaling Restores Immune Surveillance in the SMA-560 Glioma Model. Neuro Oncol. 9 (3), 259–270. doi:10.1215/15228517-2007-010
Venteicher, A., Tirosh, I., Hebert, C., Yizhak, K., Neftel, C., Filbin, M., et al. (2017). Decoupling Genetics, Lineages, and Microenvironment in IDH-Mutant Gliomas by Single-Cell RNA-Seq. Science 355 (6332), eaai8478. doi:10.1126/science.aai8478
Wang, H., Lathia, J., Wu, Q., Wang, J., Li, Z., Heddleston, J., et al. (2009). Targeting Interleukin 6 Signaling Suppresses Glioma Stem Cell Survival and Tumor Growth. Stem Cells 27 (10), 2393–2404. doi:10.1002/stem.188
Wang, J., Yan, Z., Liu, X., Che, S., Wang, C., and Yao, W. (2016). Alpinetin Targets Glioma Stem Cells by Suppressing Notch Pathway. Tumor Biol. 37 (7), 9243–9248. doi:10.1007/s13277-016-4827-2
Wang, Q., Hu, B., Hu, X., Kim, H., Squatrito, M., Scarpace, L., et al. (2017). Tumor Evolution of Glioma-Intrinsic Gene Expression Subtypes Associates with Immunological Changes in the Microenvironment. Cancer Cell 32 (1), 42–56. doi:10.1016/j.ccell.2017.06.003
Wei, J., Nduom, E., Kong, L., Hashimoto, Y., Xu, S., Gabrusiewicz, K., et al. (2016). MiR-138 Exerts Anti-glioma Efficacy by Targeting Immune Checkpoints. Neuro Oncol. 18 (5), 639–648. doi:10.1093/neuonc/nov292
Wolpert, F., Roth, P., Lamszus, K., Tabatabai, G., Weller, M., and Eisele, G. (2012). HLA-E Contributes to an Immune-Inhibitory Phenotype of Glioblastoma Stem-like Cells. J. Neuroimmunol. 250 (1-2), 27–34. doi:10.1016/j.jneuroim.2012.05.010
Wu, A., Wei, J., Kong, L. Y., Wang, Y., Priebe, W., Qiao, W., et al. (2010). Glioma Cancer Stem Cells Induce Immunosuppressive Macrophages/microglia. Neuro Oncol. 12 (11), 1113–1125. doi:10.1093/neuonc/noq082
Yang, W., Li, Y., Gao, R., Xiu, Z., and Sun, T. (2020). MHC Class I Dysfunction of Glioma Stem Cells Escapes from CTL-Mediated Immune Response via Activation of Wnt/β-Catenin Signaling Pathway. Oncogene 39 (5), 1098–1111. doi:10.1038/s41388-019-1045-6
Ye, X. Z., Xu, S. L., Xin, Y. H., Yu, S. C., Ping, Y. F., Chen, L., et al. (2012). Tumor-associated Microglia/macrophages Enhance the Invasion of Glioma Stem-like Cells via TGF-β1 Signaling Pathway. J. Immunol. 189 (1), 444–453. doi:10.4049/jimmunol.1103248
Yu, B., Liang, S. Z., and Hu, M. (2021). The Prognosis and Survival of Integrated Traditional Chinese and Western Medicine on Glioma: a Meta-Analysis. TMR Integr. Med. 5 (9), 11.
Yue, Q., Zhang, X., Ye, H., Wang, Y., Du, Z., Yao, Y., et al. (2014). The Prognostic Value of Foxp3+ Tumor-Infiltrating Lymphocytes in Patients with Glioblastoma. J. Neurooncol. 116 (2), 251–259. doi:10.1007/s11060-013-1314-0
Zhai, Y., Li, G., Li, R., Chang, Y., Feng, Y., Wang, D., et al. (2020). Single-Cell RNA-Sequencing Shift in the Interaction Pattern between Glioma Stem Cells and Immune Cells during Tumorigenesis. Front. Immunol. 11, 581209. doi:10.3389/fimmu.2020.581209
Zhang, J., Sarkar, S., Cua, R., Zhou, Y., Hader, W., and Yong, V. (2012). A Dialog between Glioma and Microglia that Promotes Tumor Invasiveness through the CCL2/CCR2/interleukin-6 axis. Carcinogenesis 33 (2), 312–319. doi:10.1093/carcin/bgr289
Zheng, Y., Yang, W., Aldape, K., He, J., and Lu, Z. (2013). Epidermal Growth Factor (EGF)-enhanced Vascular Cell Adhesion Molecule-1 (VCAM-1) Expression Promotes Macrophage and Glioblastoma Cell Interaction and Tumor Cell Invasion. J. Biol. Chem. 288 (44), 31488–31495. doi:10.1074/jbc.M113.499020
Zhi, H., Lin, C., Guryanova, O. A., Wu, Q., and Ba O, S. (2010). Cancer Stem Cells in Glioblastoma—Molecular Signaling and Therapeutic Targeting. Protein Cell 1 (7), 638–655. doi:10.1007/s13238-010-0078-y
Zhou, W., Ke, S., Huang, Z., Flavahan, W., Fang, X., Paul, J., et al. (2015). Periostin Secreted by Glioblastoma Stem Cells Recruits M2 Tumour-Associated Macrophages and Promotes Malignant Growth. Nat. Cel. Biol. 17 (2), 170–182. doi:10.1038/ncb3090
Zhu, Z., Mesci, P., Bernatchez, J., Gimple, R., Wang, X., Schafer, S., et al. (2020). Zika Virus Targets Glioblastoma Stem Cells through a SOX2-Integrin αβ Axis. Cell Stem Cell 26 (2), 187–204. doi:10.1016/j.stem.2019.11.016
Keywords: glioma, glioma stem cells, microenvironment, immune suppression, treatment
Citation: Li X, Liu M, Zhao J, Ren T, Yan X, Zhang L and Wang X (2021) Research Progress About Glioma Stem Cells in the Immune Microenvironment of Glioma. Front. Pharmacol. 12:750857. doi: 10.3389/fphar.2021.750857
Received: 31 July 2021; Accepted: 13 September 2021;
Published: 23 September 2021.
Edited by:
Jian Gao, Shanghai Children’s Medical Center, ChinaReviewed by:
Yuan Li, First Affiliated Hospital of Zhengzhou University, ChinaCopyright © 2021 Li, Liu, Zhao, Ren, Yan, Zhang and Wang. This is an open-access article distributed under the terms of the Creative Commons Attribution License (CC BY). The use, distribution or reproduction in other forums is permitted, provided the original author(s) and the copyright owner(s) are credited and that the original publication in this journal is cited, in accordance with accepted academic practice. No use, distribution or reproduction is permitted which does not comply with these terms.
*Correspondence: Lijun Zhang, bGlqdW56aGFuZ3dAZ21haWwuY29t; Xun Wang, d2FuZ3h1bjE5ODBAMTI2LmNvbQ==
†These authors have contributed equally to this work
Disclaimer: All claims expressed in this article are solely those of the authors and do not necessarily represent those of their affiliated organizations, or those of the publisher, the editors and the reviewers. Any product that may be evaluated in this article or claim that may be made by its manufacturer is not guaranteed or endorsed by the publisher.
Research integrity at Frontiers
Learn more about the work of our research integrity team to safeguard the quality of each article we publish.