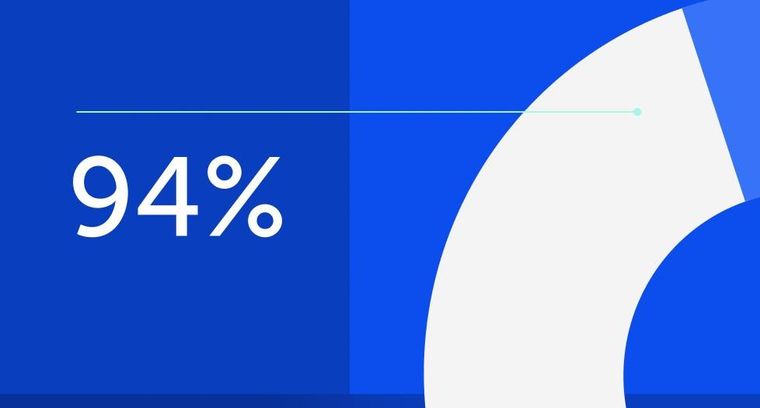
94% of researchers rate our articles as excellent or good
Learn more about the work of our research integrity team to safeguard the quality of each article we publish.
Find out more
REVIEW article
Front. Oncol., 25 July 2024
Sec. Gastrointestinal Cancers: Hepato Pancreatic Biliary Cancers
Volume 14 - 2024 | https://doi.org/10.3389/fonc.2024.1421067
Pancreatic ductal adenocarcinoma (PDAC) is an aggressive malignant tumor with a high metastatic potential. Perineural invasion (PNI) occurs in the early stages of PDAC with a high incidence rate and is directly associated with a poor prognosis. It involves close interaction among PDAC cells, nerves and the tumor microenvironment. In this review, we detailed discuss PNI-related pain, six specific steps of PNI, and treatment of PDAC with PNI and emphasize the importance of novel technologies for further investigation.
Pancreatic ductal adenocarcinoma (PDAC) is the fourth-leading cause of cancer-related death in the United States of America, with a 5-year overall survival rate of 13% (1). In 2024, 66440 new PDAC cases and 51750 PDAC-related deaths were reported in the United States of America. Metastasis is the leading cause of PDAC-related death, and patients with metastatic PDAC have a 5-year survival rate of <8%. Local metastasis occurs more frequently than distant metastasis in PDAC (2).
Perineural invasion (PNI) is the most common type of local metastasis in PDAC, with a high incidence, a special invasion routine and life-threatening clinical manifestations. Patients with PNI have a poor quality of life and prognosis (Table 1) (3). PNI is defined as the presence of cancer cells along the pancreatic nerve and peripheral nerve plexus within the epineural, perineural and endoneurial spaces of the neuronal sheath, and independently meet the situation that cancer cells envelop at least 33% of the nerves (9). It may result in inflammation, pain, sensory abnormalities, numbness and paralysis (3). Treatment of PDAC with PNI is difficult, and the rate of local or regional recurrence is as high as 60% (10), which may be attributed to the presence of residual cancer cells in the nerve sheath. PNI occurs as a result of the interaction among cancer cells, nerve cells and the tumor microenvironment (TME). However, the precise mechanisms underlying PNI in PDAC remain unclear (11).
In this review, we focus on the key steps of PNI and associated pain in PDAC. PNI is a continuous multistep process that involves various factors. The complex process of PNI is divided into six steps as follows: mutual chemotaxis between cancer and nerve cells, extracellular matrix (ECM) remodeling, cancer cell adhesion and invasion, immune evasion and nerve remodeling and regeneration. In addition to sumarising these steps, we discuss the recent research progress of PNI and novel strategies for targeting PNI in the treatment of PDAC.
The high incidence of PNI in PDAC is mainly attributed to the specific anatomical location of the pancreas and the unique nerve plexus surrounding the organ (12). As an extraperitoneal organ, the pancreas lies transversely in the upper abdomen between the duodenum on the right and the spleen on the left and is enriched with the nerve plexus. The pancreas can be divided into the head, neck, body and tail (13). According to the peripancreatic nerve division standard established by the Japan Pancreas Society (JPS), the peripancreatic nerve plexus is divided into six subgroups, namely, pancreas head nerve plexus I (PLX- I) and II (PLX-II), hepatoduodenal ligament-surrounding nerve plexus (PLX-hdl), superior mesenteric artery-surrounding nerve plexus (PLX-sma), right celiac ganglion plexus (Rcg) and left celiac ganglion plexus (Lcg). PLX- I extends from the celiac ganglion to the medial and superior uncinate processes, whereas PLX-II extends from the superior mesenteric artery nerve plexus to the medial and superior uncinate processes of the pancreas (14). Based on the subregions of the nerve plexus, PNI can occur through the following pathways (15): (1) PPC1 pathway, in which cancer cells in the pancreatic uncinate process (middle and upper regions) disseminate posterior to the portal vein to the right celiac ganglion; (2) PPC2 pathway, in which cancer cells in the pancreatic uncinate process (the tail region) travel along the inferior posterior pancreaticoduodenal artery or the jejunal trunk of the superior mesenteric vein to the mesenteric ganglion; PNI occurs through the PPC2 pathway in approximately 74%–81% of PDAC cases; (3) mesentery pathway, in which cancer cells in the pancreatic uncinate process disseminate posterior to the inferior pancreaticoduodenal artery to the mesentery of the small intestine or the transverse mesocolon; (4) anterior pathway, in which head and neck pancreatic cancer cells invade the right celiac ganglion through the gastroduodenal artery or the common hepatic artery. Moreover, pancreatic cancer cells may spread along the lymphatic capillaries and invade the superior mesenteric artery nerve plexus, eventually leading to PNI.
In addition to the specific anatomical location of the pancreas, the characteristic distribution of nerve fibers in the pancreas significantly contributes to PNI. Zuo et al. reported that nerve fibers surrounding the pancreas are rich in blood supply and form a network, facilitating the interaction between cancer cells and nerves (14). The pancreas is innervated by two types of peripheral nerves, the autonomic nerve and the sensory nerve (16). The sympathetic and parasympathetic nerves form the autonomic nervous system. The sympathetic nervous system is distributed in areas such as the pancreatic ganglia, vascular system, endocrine islands, ducts, and lymph nodes (16). The function of the parasympathetic nervous system is related to the secretion of insulin. Mainly by increasing the release of digestive enzymes to reduce insulin secretion. In terms of sensory nerves, different functional nerve fibers are distributed in various parts of the pancreas. Their relative density decreases from the head to the tail, with the head being the highest. Differently, in the pancreatic parenchyma, the postganglionic sympathetic nerve fibers are evenly distributed (17). In addition, nerve fibers capable of releasing calcitonin gene related peptide (CGRP) and substance P (SP) are distributed in the exocrine tissue of the pancreas and the islets (18).
The clinical symptoms of PDAC mainly include abdominal pain, emaciation, diarrhea, jaundice and even bleeding (19). In addition, most patients with pancreatic cancer experience abdominal or back pain. Pancreatic cancer-related pain occurs through various mechanisms involving physical and chemical factors (20). From the physical aspect, pancreatic cancer cells invade the nerve plexus and destroy the nerve sheath, increasing the susceptibility of nerves to harmful stimulation by the ECM and eventually leading to pain. The positive feedback loop between pancreatic cancer cells and nerves may accelerate this process (21). In addition, invasion of the perineural space may promote the proliferation of pancreatic cancer cells in the limited space, resulting in pain (22). From the chemical aspect, various cytokines and growth factors derived from pancreatic cancer cells and nerves are involved in PNI-related pain (Figure 1).
Figure 1 Perineural invasion related pain in PDAC. This figure shows the known major molecules in the pain generation process of PDAC. Nerve growth factor (NGF) interacts with TrkA and/or p75NTR through autocrine and paracrine ways to directly activate and sensitize sensory nerves closely related to pancreatic cancer. Transient receptor potential cationic channel V subfamily 1 (TRPV1) acted as an ion channel, causing neurons to release pain related neurotransmitters such as Calcitonin-related peptide (CGRP) and substance P, which in turn emit pain signals through the central nervous system. The increased expression of Artemin (ARTN) increased the expression of TRPV1.
Among the molecular factors involved in PNI, nerve-expressed transient receptor potential cationic channel V subfamily 1 (TRPV1) and Nerve growth factor (NGF) have been intensively investigated in studies on PDAC (23).
NGF is secreted by pancreatic cancer cells and TME-resident stromal cells, including tumor-associated immune cells and cancer-associated fibroblasts (24). It can directly bind to and activate sensory nerves expressing TrkA, p75NTR and TRPV1, resulting in neurogenic inflammation and an elevated pain response (25, 26). TRPV1 (27), a non-selective cation channel (with a preference for sodium and calcium ions), is expressed in the central nervous system and sensory ganglia and participates in the regulation of pain response (28). It can be activated by various physical (thermal and mechanical stimulation) and chemical (capsaicin [an ingredient of chilli] and neurotrophic factors such as NGF (29), GDNF (30) and ARTN) factors (31, 32). Activation of TRPV1 may result in the depolarization of neurons and release of pain-related neurotransmitters, such as calcitonin-related peptide (CGRP) and substance P, which transport pain signals to the central nervous system (33). Li et al. reported that overexpression of ARTN in pancreatic cancer cells and Schwann cells increased the expression of TRPV1, thereby triggering capsaicin-related pain (34). The formation of the ARTN–GFRα3–TRPV1 complex in neurons may enhance TRPV1 function and pain response. Qin et al. reported that honokiol (HNK), a natural compound extracted from Magnolia spp, efficiently downregulated TRPV1 and relieved PNI-related pain in a mouse model of PDAC (35, 36). In addition, PNI-related pain can be alleviated by inhibiting CCL2–CXCR10. Although the L1-CAM–p38MAPK pathway has been reported to be involved in PNI-related pain in PDAC, the precise underlying mechanism warrants further investigation (25).
As the accompany process of vascularization new nerve fibers invasion during tumor progression, primary experiment data revealed the closely relationship between pain with the vascular endothelial cell derived molecules, such as vascular endothelial growth factor (VEGF) (37), ARTN (38), interleukin-1 (39), epinephrine B2 (40) and prostaglandin (41). Therefore, we speculate that tumor neovascularization, which promotes the growth of new capillaries and nerve fibers, may be involved in PNI-related pain in PDAC.
PNI involves a close and complex interaction among cancer cells, nerve cells and TME, which results in the dissemination of cancer cells within the perineural space. The dynamic communication between nerve and PDAC cells further facilitates the immune escape, growth and metastasis of tumors. Ayala and her colleagues found that DU-145 cells co cultured with neurons showed high expression of axon guiding molecule signaling protein 4F (S4F), and increased S4F induced neuronal axon length increase (42). A decrease in S4F expression led to a decrease in axonal growth. Proved that cancer cells could drive the generation of nerve axons (42). In addition, the removal of parasympathetic and sympathetic nerve innervation resulted in tumor inhibition in mice. The gene deletion of β2- and β3-adrenergic receptors in stromal cells could also inhibit early tumor progression (43). On the contrary, the stimulation generated by the parasympathetic nervous system contributes to the progression, invasion, and metastasis of tumors in the later stage. Indicating that cancer-related neurogenesis could also drive tumor progression. Amit et al. and Chen et al. firmly believed that PNI was mainly triggered through a closed-loop system, involving direct communication between nerve cells and the tumor microenvironment, improving tumor survival rate, avoiding cancer cell apoptosis and neuronal adhesion (44, 45). Based on previous studies and reviews, the process of PNI in PDAC can be divided into the following six steps: mutual chemotaxis between cancer and nerve cells, ECM remodeling, cancer cell adhesion and invasion, immune evasion of cancer cells and nerve remodeling and regeneration (Figure 2). Identifying specific and sensitive regulators of PNI and understanding the molecular mechanisms underlying PNI may guide the development of efficient diagnostic and therapeutic strategies (Figure 3).
Figure 2 Signaling molecules in the perineural invasion of pancreatic cancer. The interactions between molecules expressed on cancer cells and nerve cells play an important role in PNI. These signaling molecules include secreted neurotrophins (such as nerve growth factor (NGF), which binds to tropomyosin-receptor kinase A (TRKA). Glial cell line-derived neurotrophic factor (GDNF) family: GDNF binds to RET, and downstream is RAF-MAPK. Annexin A2 (ANXA2) in nerve cells regulates the secretion of axonal SEMA3D, which binds to and activates PLXND1. The interaction between slit-guiding ligand 2 (SLIT2) and its receptor, roundabout guidance receptor 1(ROBO1), enhanced the motility and invasiveness of PDAC cells.
In PDAC, PNI results from the coordinated activity of both cancer and nerve cells. Both cell types release various factors that promote their mutual chemotaxis (46). In the following sections, we discuss the precise roles of cancer and nerve cells in PNI (Figure 4A).
Figure 4 PDAC PNI progression. (A), Cancer cells and nerve cells chemotaxis. The interactions between molecules that are expressed on the cancer cells and the peripheral nerves have an important role in PNI. TAMs and PSCs promoted the chemotaxis. TAMs (Tumor associated macrophages). PSCs (Pancreatic stellate cells). (B), Cancer cells remodel extracellular matrix, adhesive and invade nerve cells. Cancer cells downregulated epithelial characteristics, and activate mesenchymal characteristics. Experienced EMT cancer cells released matrix metalloproteinase (MMPs), degraded and broken through the basement membrane, remodel the ECM and form a low-resistance corridor between the cancer cells and nerve cells. MUC1 promotes cancer cell invasion by activating β-catenin and inducing epithelial-mesenchymal transformation. (C), Immune evasion of cancer cells. The increase of acetylcholine in the pancreatic vagus nerve downregulated the expression of CCL5 in cancer cells, inhibits the aggregation of CD8+T cells, and inactivated CD8+T cells, promoting cancer cell survival. (D), Nerve remodeling and regeneration. The Schwann cells recruited after nerve injury arrived at the damaged site for repair. In this process, macrophages release anti-inflammatory substances to help with axonal repair.
Cancer cells secrete various growth factors (NGF and hepatocyte growth factor [HGF]), chemokines and metabolites in an autocrine and/or paracrine manner. These molecules are involved in the initiation of PNI in PDAC. Upregulated NGF can bind to its receptors TrkA and p75NTR on pancreatic cancer cells and nerve cells (47), facilitating cancer cell migration and invasion as well as nerve cell survival, growth and development (48). The interaction of HGF family members with the proto-oncogene receptor c-Met promotes PNI either directly by activating mTOR/NGF or indirectly by enhancing NGF secretion. Inhibition of c-Met suppresses the migration of cancer cells along the nerves, attenuates the damage caused by cancer cells to the sciatic nerve and protects the function of the sciatic nerve in vivo (49, 50).
Cancer cell-derived C-X-C-based chemokine ligand (CXCL) and its related receptor (CXCR) on nerve cells can facilitate PNI in PDAC. The interaction of CX3CL1 with CX3CR1 promotes the migration of PDAC cells (MiaPACA2, T3M4, PANC1 and ASPC1 cells) to nerve cells (51). In addition, Nodal, which plays a key role in embryonic neural development, can promote the initiation of PNI in PDAC. Overexpression of Nodal in pancreatic cells upregulates the expression of NGF, BDNF and GDNF, enhancing the migratory and invasive abilities of cancer cells. A study showed that Nodal promoted the nerve sphere-forming ability in a 3D nerve invasion model involving the coculture of nerve and pancreatic cancer cells. Therefore, inhibiting Nodal expression may represent a promising strategy for targeting PNI in the treatment of pancreatic cancer (52).
Nerve cells secrete growth factors (NGF and GDNF), chemokines and metabolites in a paracrine manner. These molecules have been reported to be involved in PNI in PDAC. GDNF accelerates the migration of Kras-mutated pancreatic cancer cells to nerve cells through the RET–phosphatidylinositol 4,5-diphosphate-3-kinase catalytic pathway. Inhibiting the release of GDNF from nerve cells has been shown to prevent PNI in a mouse model of PDAC (53). Soluble GFRα1 secreted by nerve cells may enhance PNI (54). Chernichenko et al. used siRNA library screening and found that the GDNF–RET–βPix–Cdc42 pathway guided the migration of pancreatic cancer cells to nerve cells. This pathway may serve as a promising therapeutic target for PDAC (55). Artemin, a GDNF family member, can initiate PNI through the GFRα3–NF-κB/CXCR4 signaling pathway (56). CXCL12 secreted by DRG in a paracrine manner can attract PDAC cells to the periphery of DRG (57). Norepinephrine activates adrenaline receptor β2 (ADRβ2), cAMP-dependent protein kinase and activating transcription factor 3 to induce the chemotaxis of cancer cells toward nerves (58). Glutamate is an important excitatory neurotransmitter in the nervous system, accounting for 60% -70% of the total number of synapses. Glutamate has two types of receptors: ion affinity receptors (NMDA) and metabolic receptors (59). The downstream signals of NMDA, MEK-MAPK and CaMK, induce tumor invasion in a mouse model of pancreatic neuroendocrine tumors (60). A recent study by Li et al. showed that glutamate in nerve cells can induce calcium influx into PDAC cells through N-methyl-D-aspartate receptor (NMDAR), thereby activating the downstream calcium-dependent protein kinase CaMKII/ERK–MAPK pathway and promoting the transcription of METTL3. Subsequently, METTL3 upregulates the mRNA expression of hexokinase 2 (HK2) through N6-methyladenosine (m6A) modification, thereby promoting glycolysis and PNI in PDAC (61). The interaction between Schwann cells and cancer cells can be regulated at multiple levels. Schwann cells can communicate with cancer cells by secreting proteins, including L1 cell adhesion molecule (L1-CAM) (62) and TGFβ (63). In a study, immunohistochemical analysis of PDAC tissue samples showed that L1-CAM was highly expressed in cancer cells and adjacent Schwann cells of the affected nerves. L1-CAM secreted by Schwann cells affected the chemotaxis of cancer cells by activating the MAP kinase signaling pathway (62).
Stromal cells present in TME and factors secreted by them are involved in the chemotaxis of cancer and nerve cells. Pancreatic stellate cells (PSCs), which account for 50% of all stromal cells in the PDAC microenvironment, release the ECM glycoprotein tenascin C, which enhances the chemotaxis of cancer and nerve cells (64). Aberrant activation of hedgehog signaling is involved in the communication among tumor cells, PSCs and nerve cells (65). Pancreatic cancer cells release hedgehog signaling molecules to activate the hedgehog pathway in PSCs, facilitating the chemotaxis of cancer cells toward nerve cells. Tumor-associated macrophages (TAMs) result in the formation of an immunosuppressive TME in PDAC. Studies have shown that TAMs contribute to a poor prognosis in PDAC with PNI (66). Colony-stimulating factor 1 (CSF1) secreted by PDAC cells can recruit and stimulate macrophages to promote the chemotaxis of cancer cells (67).
Not all neurotrophins are highly expressed in PDAC cells. For example, Göhrig A et al. showed that the mRNA expression of slit-guiding ligand 2 (SLIT2), a neurotrophic protein related to cell migration, was low or absent in PDAC cell lines and tumor tissues from patients with PDAC (68). Functional studies have shown that overexpression of SLIT2 in human PDAC cell lines (MiaPACA2 and PANC1) suppresses cell migration and invasion (68). Conversely, blocking the interaction between SLIT2 and its receptor roundabout guidance receptor 1 (ROBO1) enhances the motility and invasiveness of PDAC cells, further supporting the inhibitory effects of SLIT2 on cancer cell migration and invasion (68).
Under the chemotactic action of nerve cells, cancer cells initially undergo EMT and subsequently remodel the ECM. During EMT, cancer cells lose epithelial characteristics and acquire mesenchymal characteristics. ECM is a polymeric network of proteins, glycoproteins, proteoglycans and glycosaminoglycans that supports and compartmentalizes tissues while regulating cell fate and function (69). It is roughly divided into two parts, namely, the interstitial connective tissue matrix and the basement membrane (BM). After undergoing EMT, cancer cells release matrix metalloproteinases (MMPs), degrade and penetrate the BM (70–72) and remodel the ECM to create a low-resistance corridor between cancer and nerve cells, thereby crossing the ECM (Figure 4B).
BM is composed of an interrelated network of type IV collagen, laminins, nidogen and sulphated proteoglycans that lies beneath epithelial and endothelial cells and surrounds muscles, nerves, adipocytes and smooth muscle cells (73). Molecules secreted by cancer and stromal cells participate in the penetration of BM. Proteolysis of MMPs disrupts BM, which is necessary for pancreatic cancer cells to pass through the barrier. MMP2 and MMP9 lead to polysaccharide deficiency and interrupt the activity of extracellular fibronectin and type IV collagen, which play an important role in the penetration of BM by cancer cells (74). GDNF activates MMP9 by binding to the RET tyrosine kinase receptor and activating two downstream signaling pathways: PI3K–AKT and RAS/RAF–MEK-1–ERK1/2 pathways, which are essential for the activation and expression of MMP9 (53, 75). Stromal cells secrete various factors that indirectly regulate the expression and function of MMPs. Schwann cells surrounding nerves can release L1-CAM, activate downstream STAT3, upregulate the expression of MMP2 and MMP9 and promote the degradation of ECM (62). The expression of MMPs is remarkably high in cocultured PSCs and PDAC cells (76). Upregulated galactose agglutinin 1 (LGALS1) in stellate cells activates SRC signaling in PDAC cells, leading to the transcription of MMPs, which is necessary for the degradation of ECM and the passage of cancer cells through BM (71). In vitro studies have shown that activated macrophages can stimulate human PDAC cells (PANC1 and MiaPACA2) to secrete MMP1 (77). Soluble MMP1 induces DRG to release substance P (SP); activates killer cell lectin-like receptor B1 (KLRB1) and consequently leads to ECM degradation through the SP, KLRB1 and MAPK pathways (77).
In an in vivo setting, ECM degradation involves not only the disruption and penetration of BM by cancer cells but also the formation of a low-resistance corridor in ECM to facilitate cancer cell migration. Cancer cells follow a certain concentration gradient, and aggregation results in mechanical pressure (78). PDAC cells migrate toward never cells along the GDNF concentration gradient. Cancer-associated fibroblasts (CAFs) are highly differentiated stromal cells that promote ECM remodeling in tumors (79). CAF-derived TGFβ stimulates the synthesis of collagen in neighboring fibroblasts, thereby increasing the stiffness of the tissue. The resulting mechanical stress in the connective tissue leads to the collapse of neighboring structures. Subsequently, the dense structure formed through fibrosis surrounding a group of PDAC cells increases the internal pressure and leads to the rupture of glandular structures (69). The formation of high-density fibers helps to track the movement of cancer cells.
After they migrate to nerve cells, cancer cells adhere to the neurilemma by binding to cell adhesion molecules and their related receptors (Figure 4B). Overexpressed Mucin 1 (MUC1), a type I transmembrane protein, on pancreatic cancer cells can selectively bind to MAG on peripheral nerve cells through the abnormal glycosylation extracellular domain, contributing to selective adhesion between the two types of cells (80). In addition, overexpressed MUC1 promotes cancer cell invasion by activating β-catenin and inducing EMT. GDNF treated pancreatic cancer cell line displayed silk foot and tablet lipid formation enhanced extracellular matrix proteins adhesion ability, which played an important role in the PDAC-related PNI (81). In a study, high-throughput genetic analysis of a neurotrophin sorting model showed that kinesin family member 14 (KIF14) and Rho GDP dissociation inhibitor β (ARHGDI β) were involved in the recruitment of adhesion molecules to the cell membrane, however, the exact underlying mechanism warrants further investigation (82). The interaction between CXCR1 and CX3CL1 facilitates the co-localization of β1 integrin and focal adhesion kinase (FAK) to stabilize adhesion between nerve and cancer cells (51). Nerve cell adhesion molecule 1 (NCAM1) expressed on Schwann cells can enhance cell–cell adhesion through cadherins; however, modification of NCAM1 by polysialic acid counteracts these effects (83, 84).
The nerve bundle membrane, which acts as a protective barrier, contains laminin, fibronectin and type IV collagen. After cancer cells infiltrate the nerve bundle membrane, cancer cells and neighboring cells secrete numerous factors that promote the proliferation and migration of cancer cells along the nerve fibers (Figure 4B) (85).
Damaged nerve fibers produce abundant neurotrophic hormones and attract more cancer cells, creating a vicious cycle. PAP/REG3A (PAP/REG3β), a type C lectin-like secretory protein, can promote the growth of cancer cells in the nerve space, resulting in an increase in mechanical pressure in nerve fibers (86, 87). Upregulated SDC3 in nerve cells may lead to the aggregation of PTN+ PDAC cells around nerve cells, thereby promoting cancer cell invasion (88, 89). In the nerve space, cancer cells require more nutrients or energy to support their growth and metastasis by altering their metabolism. Axons and dorsal root ganglia (DRG) secrete serine to provide additional energy to cancer cells. In the case of serine deprivation, PDAC cells express and secrete more NGF by upregulating its translation, thereby enhancing the movement of axons toward the tumor nest.
In addition to the direct communication between cancer cells and nerve fibers, stomal cells, such as CAFs, participate in and enhance cancer cell invasion. In PDAC, CCL2 secreted by Schwann cells can recruit CCR2 monocytes, which further differentiate into macrophages, release protease B and contribute to the degradation of nerve bundle membrane proteins (90). TGFβ produced and released by Schwann cells can enhance the invasive ability of PDAC cells. Ferdoushi et al. showed through proteomic analysis that TGFβ (TGFβ-1, TGFβ-2, TGFβ-BI) played an important role in Schwann cells stimulating pancreatic cancer cell migration, invasion and metastasis, but the specific functional analysis is unknown (91). Roger et al. also reported that TGFβ rich in Schwann cell culture medium could promote the migration and invasion of Capan-2 cells (63). In addition, tumor-activated Schwann cell trajectories (TASTs) can promote the migratory and invasive abilities of PDAC cells. Non-myelinated Schwann cells form TASTs, resulting in a poor prognosis in PDAC. In TASTs, dynamic SCs form trajectories as cancer-related pathways and enhance the migratory ability of cancer cells (92). Activation of CAF-derived MMP2 by membrane type I matrix metalloproteinases (MT1-MMPs) contributes to the degradation of nerve bundle collagen and facilitates the diffusion of cancer cells in the perineural space (93, 94).
Abnormalities in mitochondrial function and glucose metabolism have been associated with cancer cell invasion, as these metabolic changes may be related to the aetiology of neurodegeneration and nerve injury. Oxidative stress can induce chronic neuroinflammation and the transformation of astrocytes from the neurotrophic to neurotoxic phenotype, which may inhibit nerve cell proliferation. In addition, the binding of NGF to TrkA receptors can induce the Warburg effect in PDAC and DRG cells. Goetze et al. showed that lactate can promote cancer cell invasion (95). The nervous system contains abundant lactate, which is produced by Schwann cells through aerobic glycolysis to support the propagation of action potentials along axons (96).
After invasion, cancer cells attempt to evade elimination by neighboring immune cells. Neurotransmitters regulate anti-immune responses. In particular, sympathetic and parasympathetic nerve fibers release norepinephrine (NE) and acetylcholine (ACh) in tumors to reduce the anti-tumor immune response (Figure 4C) (97, 98). Catecholamine triggers immunosuppression in the TME of lung cancer, leading to the re-polarization of M1 macrophages to the M2 phenotype and the aggregation of M2-polarized macrophages and MDSCs. In addition, it reduces the proportion of anti-tumor dendritic cells (DCs), resulting in the synthesis and release of IL-10 and VEGF, inhibition of immune responses and promotion of angiogenesis (99). The impact of adrenergic stress and subsequent catecholamine release on T lymphocytes in the TME was also studied in mouse models of colon cancer and melanoma (100, 101). However, role of catecholamine in PNI in PDAC remains unclear. Catecholamines (adrenaline, NE, DE), also known as stress mediators, have been recognized for their role in cognitive, emotional changes, stress-related diseases, cancer occurrence, and tumor metastasis (102). Based on the above reports, we assumed that immune checkpoint blockade in PDAC is driven by adrenergic stress, leading to increased expression of certain immune checkpoint molecules such as PD-1, FOXP3, and LAG3, thereby triggering T cell depletion.
Macrophages typically had a high content in the TME immune cell population and mainly exerting immunosuppressive effects and participating in multiple stages of PDAC PNI progression (4, 9, 103). TAMs were mainly differentiated from inflammatory monocytes. As mentioned above, myeloid progenitor cells in bone marrow differentiated into inflammatory monocytes under the mobilization of the CCL2/CCR2 axis (104). In the progress of PDAC PNI, inflammatory monocytes at the injured site differentiated into TAMs (105), which participated in the inhibition of various immune responses. Played an important role in dealing with nerve injury and various environmental disturbances (106). TAMs could be divided into M1 and M2 subtypes (106). Type M1 usually played an anti-tumor function, while type M2 played an immunosuppressive function (103). TAMs were usually considered M2 type and secreted various cytokines such as CCL5 and TNF to enhance PNI (107). In the PDAC PNI progression, nuclear factor kappaB (NFκB) could promote the repolarization of macrophages from M1 to M2 and exert the immunosuppressive function of macrophages (108, 109). In addition, OC et al. reported that endoneurial macrophages could be transformed into microglia/macrophage subsets, which could promote nerve regeneration and anti-tumor (67). Endoneurial macrophages were recruited to the front of the tumor by colony stimulating factor-1 secreted by tumors. Activated endoneurial macrophages secreted high levels of GDNF, promote RET phosphorylation on PDAC cell membrane, activated downstream MAPK and PI3K pathways in cancer cells, and enhance PNI (67).
Cancer-associated fibroblasts (CAF) were highly differentiated stromal cells that supported tumor invasion by stimulating angiogenesis, cancer cell proliferation and matrix remodeling (110). In PDAC, CAF was thought to differentiate from pancreatic stellate cells (111). CAF has been proved to be the source of paracrine growth factors, which affected the growth of cancer cells through paracrine, so that some cancer cells could survive (112). For example, M.J et al. reported that TGFβ from CAF induced fibroblasts to react and synthesize collagen, which hardened the surrounding tissue, and the resulting mechanical stress destroyed nearby blood vessels, leading to hypoxia (113). At this time, Schwann cells were activated by interleukin-6 (IL-6) produced by cancer cells, facilitated PNI, which was accompanied by changes in hypoxia-related signaling pathways, such as HIF1α (114). In addition, the interruption of glandular structure is induced by the internal high pressure of CAF fibrosis. At the same time, the changes of fiber structure also make it easier for cancer cells to spread and metastasize (112). CAF and cancer cells work together in PDAC PNI: CAF makes it easier for cancer cells to invade, while cancer cells polarize to promote the spread of CAF. CAF could also promote cancer invasion by secreting matrix remodeling factors (115, 116). A.F et al. and V.S et al. showed that in the process of PNI, CAF at the injured site produced inactivated MMP2, which is then activated by membrane type I matrix metalloproteinases (MT1-MMPs) from cancer cells, then could degrade extracellular collagen of nerve membrane and promote the process of PDAC PNI (94, 117).
T cells, one of the main components of the immune system, originated from the thymus and perform the function of killing infected host cells or cancer cells (118). As mentioned earlier, in PDAC PNI, the vagus nerve played an immunomodulatory role by controlling the infiltration or activation of T cells (119). ACh released from vagus nerve inhibited the expression of CCL5 in PDAC by mediating histone deacetylation. Low levels of CCL5 damage the recruitment of CD8+T cells, leading to immunosuppressive tumor microenvironment. Interferon-γ from CD8+T cells was also inhibited by ACh, which promoted the differentiation of T cell helper 2 cells and decreased T cell helper 1 cells (119). And vagotomy destroyed the PNI process and inhibited tumor growth in vivo (119). However, BW et al. reported that subseptal vagotomy could accelerate the tumor formation of KrasG12D-driven PDAC in mice (120). These two distinct results suggest that cholinergic nervous system plays a dual role in PDAC and participates in the recruitment and activation of T cells.
Cancer cells, with the help of nerve cells, evade the immune system, continue to proliferate, metastasize along nerve fibers and infiltrate them. The damaged nerve fibers are repaired and regenerated through the combined action of Schwann cells, macrophages and CAFs and continue to exert chemotactic effects on cancer cells, forming a vicious cycle (Figure 4D). Once damaged and invaded by cancer cells, axons may trigger the dedifferentiation and activation of Schwann cells through the release of neuromodulators (NRGs) from remaining neurons (121). In PDAC, overexpression of Artemin, a member of the α ligand family, promotes neuronal remodeling and nerve fiber proliferation around tumors through Ret/GFRα3 receptors (122). Damaged Schwann cells play an important role in maintaining neuron survival by producing various neurotrophic factors and cell surface proteins, releasing pro-inflammatory mediators to alter the local signaling environment, participating in axonal maintenance and post-injury repair and contributing to subsequent nerve regeneration (123). After partial peripheral nerve injury, the continuous demyelination of peripheral nerves relieves the inhibition of axon growth and promotes nerve germination. CAFs contribute to axonal growth by secreting Eph-B, which activates the EphB2 receptor on Schwann cells, and inducing the migration of Schwann cells within the nerve bridge through the Eph-B/EphB2 signaling pathway, thereby guiding axons to reach the injured site (124). As members of the membrane-associated or secretory glycoprotein family, signaling proteins are involved in axon guidance and cell migration, with axon guidance being the most important function. Annexin A2 (ANXA2) in nerve cells regulates the secretion of axonal SEMA3D (125), which binds to and activates PLXND1 (126), thereby promoting PNI in PDAC (127). The levels of interleukin-6 (IL-6), which is produced and secreted by CAFs and mast cells, are high in serum or tissue samples from patients with PDAC (128). After binding to corresponding receptors on Schwann cells, IL-6 activates the STAT3 pathway, triggering the growth of Schwann cells and promoting neuronal plasticity (128).
In addition, Mauffrey et al. demonstrated that neural precursor cells expressing the neural stem cell marker Doublecortin (DCX+) migrated from the region of neural origin to the niche of the tumor and differentiated into mature noradrenergic neuronal phenotypes (129). More DCX+cells were observed in high-risk cancer samples compared to low-risk samples. The depletion of DCX+cells also reduced the incidence of tumor lesions, and with the addition of DCX+neural precursor cells, tumor growth accelerated (129). Cancer stem cells could also form new neurons. Lu et al. found that cancer stem cells from gastrointestinal patients could differentiate into sympathetic neurons that produced tyrosine hydroxylase (TH) and parasympathetic neurons that produced vesicular acetylcholine transporters (130). On the contrary, these neurons could communicate with cancer cells in xenografts to promote tumor growth (130). In summary, cancer cells could promote the regeneration of nerve cells to a certain extent.
Surgery is the first-line treatment strategy for early-stage PDAC. PNI is detected in the early postoperative stages in most patients (131). The expanded resection scope includes the entire pancreatic structure, dissection of lymph nodes and removal of all relevant blood vessels (132, 133). Meanwhile, explore new methods for intraoperative use of staining techniques to identify neural structures and alleviate PDAC PNI (133). A combination of gemcitabine and Nab-paclitaxel or mFOLFIRINOX is commonly used to treat advanced PDAC with local or distant metastasis (134). Denervation of the pancreas using an ethanol-induced celiac plexus block represents a useful strategy for prolonging the survival of patients with advanced PDAC. A study showed that patients treated with ethanol-induced splanchnicectomy, a procedure for the surgical resection of splanchnic nerves, survived longer than patients in the control group (median survival, 9.15 vs. 6.75 months) (135). The frequency of PNI ranges from 70.8 to 93.0% in patients with PDAC treated with pancreatectomy and from 43 to 58% in patients treated with neoadjuvant therapy and pancreatectomy (9).
SBRT radiotherapy, a neoadjuvant treatment modality, is widely used for treating locally advanced pancreatic cancer; however, the overall radiation dose was not high, and does not reach the radiotherapy mode of high dose and low frequency (136). In a study, the incidence of PNI in patients with PDAC who received neoadjuvant radiotherapy (58%) was lower than that in patients who did not receive neoadjuvant radiotherapy (80%) (P = 0.002) (137). Preclinical studies have shown that radiotherapy may help limit PNI. In particular, 4Gy radiation can significantly reduce the release of GDNF in DRG, thereby inhibiting the invasive ability of MiaPACA2 cells (138). 8Gy radiation can lead to a decrease in the secretion of GDNF by the sciatic nerve, inhibiting the progression of PNI and protecting the sciatic nerve against cancer cell-induced damage in vivo. In addition, sustained low-dose irradiation with iodine-125 seeds has been shown to inhibit tumor growth and PNI in preclinical models, suggesting that local implantation of iodine-125 seeds in tumors can alleviate PNI-related pain in PDAC (139).
The characteristics of PDAC, a malignant tumor, were chemotherapy resistance and radiation resistance. 80% patients receiving surgical treatment experience recurrence and PNI, and over 50% patients receiving neoadjuvant therapy also experience recurrence and PNI (9, 137). PDAC PNI patients also have strong abdominal and back pain, and poor life quality (140). In this context, it is particularly important to promote research and development of new drugs targeting PDAC PNI.
At present, PNI targeted therapy has made certain progress, and several new drugs are undergoing various experiments in order to enter clinical practice. Such as anti-NGF drugs (141), anti-Trk receptor drugs (142), Resiniferatoxin (RTX) (26), oncolytic adenoviruses (143), and Honokiol (HNK) (36). In preclinical models of PDAC, the NGF-TrkA signaling pathway has enormous potential for drug development (25). Specific antibodies or genetic interference could be used to block NGF and alleviate pain, or specific tyrosine kinase receptor blockers could be used to delay tumor progression. The current anti-NGF antibodies, such as muMab911, Tanezumab, MNAC13, PHA-848125, and ARRY-470, all act by blocking the binding of NGF to its receptors. Tanezumab has already been tested in Phase III trials (144). In addition, in environments lacking serine/glycine, Trk-NGF inhibitors (LOXO-101) could reduce nerve innervation and slow down PDAC progression (142). Some data confirm that Resiniferatoxin (RTX) could promote apoptosis of pancreatic cancer cells and reduce pain awareness, which means that RTX may become a new effective drug for PDAC patients to fight against neurogenic pain (26). The model of PNI demonstrated that attenuated herpes simplex virus could detect invading nerves through fluorescence imaging during surgery, making lesion clearance more thorough in vivo (145). T. K et al. reported that oncolytic adenoviruses (OBP-301 and OBP-702) could inhibit the migration and invasion of pancreatic cancer cells (143). OBP-702 acted on ERL signaling, blocking the migration and invasion of PDAC cells. Of course, further research is needed to explore the safety of OBP-301 and OBP-702 in PDAC patients (143). Magnolol (HNK) was a polyphenolic compound extracted from plants of the Magnolia genus. HNK could alleviate PNI. In mechanism, HNK may inhibited PDAC PNI by inhibiting the activation of Smad2/3, leading to the reduction of NGF and BDNF in pancreatic cancer cells (36). These promising studies may provide effective methods for treating PDAC PNI in the future, reducing patient pain and prolonging patient survival.
PNI is a complex phenomenon that involves multidirectional communication among nerve cells, tumor cells and TME. Numerous studies have investigated the pathological processes and mechanisms involved in the development of PNI in PDAC. Targeting these processes or mechanisms represents a promising therapeutic strategy for PDAC. The first half of this review starts with the pain caused by PNI in pancreatic cancer, and combined with the function of nerves, describes the current research progress on nerve pain in pancreatic cancer. By understanding the mechanism of PNI and pain in pancreatic cancer, we may be able to find more therapeutic strategies to delay and block PNI. In the second half of this review, the PNI process of pancreatic cancer is described from six steps: mutual chemotaxis between cancer and nerve cells, extracellular matrix (ECM) remodeling, cancer cell adhesion and invasion, immune evasion of cancer cells and nerve remodeling and regeneration. The whole invasion process is the result of many factors. In this process, identifying specific molecular targets or exploring new ways to inhibit PNI may have potential implications for the treatment of PDAC.
In the past few decades, research on PDAC PNI has been focused on pathology and molecular biology. Due to methodological limitations, there is an urgent need for a new approach to help scholars further understand PNI. With the rapid development of machine learning and artificial intelligence, research on PNI seems to have found new directions. Artificial intelligence (AI) is an exciting new technology that can mimic human cognitive learning processes and automate specific tasks (146). The more data AI algorithms provided, the more reliable they are in executing tasks. According to reports, artificial intelligence has successfully identified PNI in prostate cancer tissue (147). They developed an artificial intelligence (AI) algorithm based on deep neural networks, which measured the PNI of 7406 patients, reducing the workload of pathologists and more clearly demonstrating the relationship between perineural invasion and poor prognosis (148, 149). Fortunately, Borsekofsky et al. developed a highly sensitive artificial intelligence algorithm in the field of PDAC PNI to detect PNI in PDAC surgical specimens. Compared with pathologists analyzing it separately, this algorithm significantly improved time, efficiency, and accuracy (146). From the current perspective, the main function of artificial intelligence is to assist pathologists in early diagnosis of PNI (150). AI in the field of pathology is still very young and will continue to mature in the future.
However, some issues deserve further study: (1) Existing techniques for screening specific molecular mechanisms have not been widely used in studies on PDAC with PNI. Moreover, large-scale molecular data on cancer and nerve cells involved in PNI are lacking. In recent years, single-cell sequencing has improved our understanding of biological systems. Given that the function of many biological systems depends on their spatial organization, novel techniques for analyzing single-cell spatial transcription and metabolism have emerged with the development of high-throughput technologies and the improvement of computational methods. Single-cell spatial transcriptomic techniques can be used to elucidate PNI in pancreatic cancer, understand its role at different spatial points and reveal the underlying mechanisms. With the development and progress of molecular biology and genomics, valuable molecular markers of PNI have been identified, which may provide important clinical evidence for the early prognosis and prompt treatment of patients with PDAC.
(2) Many clinical trials on PDAC lack preclinical modeling in either GEMMs or orthotopic PDX models, which are more representative of human diseases than commercial PDAC cell lines. Although preclinical studies have used commercial PDAC cell line-derived xenograft mouse models with encouraging in vivo results, clinical trials have been unable to proceed. In addition, as research on immunology and PNI is gradually progressing, it is important to use immunocompetent GEMMs (or humanized PDX models) to verify anti-tumor PNI phenotypes and mechanisms. In addition to models of tumor–nerve interactions, organic PDAC cultures derived from patient sources hold great promise in predicting clinical responses to treatment. However, the predictive ability must be validated in prospective clinical trials. Developing novel treatment methods for PDAC is challenging. In addition to the ongoing clinical trials, we have also noticed many clinical conversion failures that attempt to achieve therapeutic benefits by blocking various processes of PNI. Clinical trials should be designed based on strong preclinical data to optimize the chances of success. Future developments and discoveries heavily depend on limited data from experimental and animal models used to simulate PNI. Elucidating the precise mechanisms underlying PNI particularly relies on methodological advances, which is an important focus of future research. In addition, studies investigating the reciprocal interactions between the fields of immunology and metabolism and pipelines that incorporate mathematical modeling as well as artificial intelligence- and machine learning-based methods are required.
YS: Software, Writing – original draft. WJ: Data curation, Validation, Writing – review & editing. XL: Methodology, Project administration, Writing – review & editing. DW: Conceptualization, Methodology, Writing – review & editing, Funding acquisition.
The author(s) declare financial support was received for the research, authorship, and/or publication of this article. This study was supported by grants from the National Natural Science Foundation of China (Grant no. 82071984, 82272066), the Social Development Foundation of Jiangsu Province (Grant no. BE2023759).
The authors declare that the research was conducted in the absence of any commercial or financial relationships that could be construed as a potential conflict of interest.
All claims expressed in this article are solely those of the authors and do not necessarily represent those of their affiliated organizations, or those of the publisher, the editors and the reviewers. Any product that may be evaluated in this article, or claim that may be made by its manufacturer, is not guaranteed or endorsed by the publisher.
1. Siegel RL, Giaquinto AN, Jemal A. Cancer statistics, 2024. CA: Cancer J Clin. (2024) 74:12–49. doi: 10.3322/caac.21820
2. Gerstberger S, Jiang Q, Ganesh K. Metastasis. Cell. (2023) 186:1564–79. doi: 10.1016/j.cell.2023.03.003
3. Ting KC, Lee TL, Li WY, Chang CF, Chu PY, Wang YF, et al. Perineural invasion/lymphovascular invasion double positive predicts distant metastasis and poor survival in T3-4 oral squamous cell carcinoma. Sci Rep. (2021) 11:19770. doi: 10.1038/s41598-021-99280-2
4. Chen Z, Fang Y, Jiang W. Important cells and factors from tumor microenvironment participated in perineural invasion. Cancers. (2023) 15(5):1360. doi: 10.3390/cancers15051360
5. Schmitd LB, Scanlon CS, D'Silva NJ. Perineural invasion in head and neck cancer. J Dental Res. (2018) 97:742–50. doi: 10.1177/0022034518756297
6. Niu Y, Förster S, Muders M. The role of perineural invasion in prostate cancer and its prognostic significance. Cancers. (2022) 14(17):4065. doi: 10.3390/cancers14174065
7. Liebig C, Ayala G, Wilks JA, Berger DH, Albo D. Perineural invasion in cancer: A review of the literature. Cancer. (2009) 115:3379–91. doi: 10.1002/cncr.24396
8. Wang H, Huo R, He K, Cheng L, Zhang S, Yu M, et al. Perineural invasion in colorectal cancer: mechanisms of action and clinical relevance. Cell Oncol (Dordrecht). (2024) 47:1–17. doi: 10.1007/s13402-023-00857-y
9. Li J, Kang R, Tang D. Cellular and molecular mechanisms of perineural invasion of pancreatic ductal adenocarcinoma. Cancer Commun (London England). (2021) 41:642–60. doi: 10.1002/cac2.12188
10. Sung H, Ferlay J, Siegel RL, Laversanne M, Soerjomataram I, Jemal A, et al. Global cancer statistics 2020: globocan estimates of incidence and mortality worldwide for 36 cancers in 185 countries. CA Cancer J Clin. (2021) 71:209–49. doi: 10.3322/caac.21660
11. Jobling P, Pundavela J, Oliveira SM, Roselli S, Walker MM, Hondermarck H. Nerve-cancer cell cross-talk: A novel promoter of tumor progression. Cancer Res. (2015) 75:1777–81. doi: 10.1158/0008-5472.CAN-14-3180
12. Crippa S, Pergolini I, Javed AA, Honselmann KC, Weiss MJ, Di Salvo F, et al. Implications of perineural invasion on disease recurrence and survival after pancreatectomy for pancreatic head ductal adenocarcinoma. Ann Surg. (2022) 276:378–85. doi: 10.1097/SLA.0000000000004464
13. Talathi SS, Zimmerman R, Young M. Anatomy, abdomen and pelvis, pancreas. In: Statpearls. StatPearls Publishing Copyright © 2023, StatPearls Publishing LLC, Treasure Island (FL (2023). ineligible companies. Disclosure: Ryan Zimmerman declares no relevant financial relationships with ineligible companies. Disclosure: Michael Young declares no relevant financial relationships with ineligible companies.
14. Zuo HD, Zhang XM, Li CJ, Cai CP, Zhao QH, Xie XG, et al. Ct and Mr imaging patterns for pancreatic carcinoma invading the extrapancreatic neural plexus (Part I): anatomy, imaging of the extrapancreatic nerve. World J Radiol. (2012) 4:36–43. doi: 10.4329/wjr.v4.i2.36
15. Patel BN, Olcott E, Jeffrey RB. Extrapancreatic perineural invasion in pancreatic adenocarcinoma. Abdominal Radiol (New York). (2018) 43:323–31. doi: 10.1007/s00261-017-1343-9
16. Radzimirska M, Kuchinka J, Nowak E, Trybus W, Szczurkowski A. Cholinergic and adrenergic innervation of the pancreas in chinchilla (Chinchilla laniger molina). Folia histochemica cytobiologica. (2020) 58:54–60. doi: 10.5603/FHC.a2020.0005
17. Lindsay TH, Halvorson KG, Peters CM, Ghilardi JR, Kuskowski MA, Wong GY, et al. A quantitative analysis of the sensory and sympathetic innervation of the mouse pancreas. Neuroscience. (2006) 137:1417–26. doi: 10.1016/j.neuroscience.2005.10.055
18. Makhmutova M, Caicedo A. Optical imaging of pancreatic innervation. Front Endocrinol. (2021) 12:663022. doi: 10.3389/fendo.2021.663022
19. Ceyhan GO, Schäfer KH, Kerscher AG, Rauch U, Demir IE, Kadihasanoglu M, et al. Nerve growth factor and artemin are paracrine mediators of pancreatic neuropathy in pancreatic adenocarcinoma. Ann Surg. (2010) 251:923–31. doi: 10.1097/SLA.0b013e3181d974d4
20. Halbrook CJ, Lyssiotis CA, Pasca di Magliano M, Maitra A. Pancreatic cancer: advances and challenges. Cell. (2023) 186:1729–54. doi: 10.1016/j.cell.2023.02.014
21. di Mola FF, di Sebastiano P. Pain and pain generation in pancreatic cancer. Langenbeck's Arch Surg. (2008) 393:919–22. doi: 10.1007/s00423-007-0277-z
22. Lindsay TH, Jonas BM, Sevcik MA, Kubota K, Halvorson KG, Ghilardi JR, et al. Pancreatic cancer pain and its correlation with changes in tumor vasculature, macrophage infiltration, neuronal innervation, body weight and disease progression. Pain. (2005) 119:233–46. doi: 10.1016/j.pain.2005.10.019
23. Zhu Y, Colak T, Shenoy M, Liu L, Pai R, Li C, et al. Nerve growth factor modulates trpv1 expression and function and mediates pain in chronic pancreatitis. Gastroenterology. (2011) 141:370–7. doi: 10.1053/j.gastro.2011.03.046
24. Zhu Z, Friess H, diMola FF, Zimmermann A, Graber HU, Korc M, et al. Nerve growth factor expression correlates with perineural invasion and pain in human pancreatic cancer. J Clin Oncol. (1999) 17:2419–28. doi: 10.1200/JCO.1999.17.8.2419
25. Wang W, Li L, Chen N, Niu C, Li Z, Hu J, et al. Nerves in the tumor microenvironment: origin and effects. Front Cell Dev Biol. (2020) 8:601738. doi: 10.3389/fcell.2020.601738
26. Hartel M, di Mola FF, Selvaggi F, Mascetta G, Wente MN, Felix K, et al. Vanilloids in pancreatic cancer: potential for chemotherapy and pain management. Gut. (2006) 55:519–28. doi: 10.1136/gut.2005.073205
27. Li T, Jiang S, Zhang Y, Luo J, Li M, Ke H, et al. Nanoparticle-mediated trpv1 channel blockade amplifies cancer thermo-immunotherapy via heat shock factor 1 modulation. Nat Commun. (2023) 14:2498. doi: 10.1038/s41467-023-38128-x
28. Jara-Oseguera A, Simon SA, Rosenbaum T. Trpv1: on the road to pain relief. Curr Mol Pharmacol. (2008) 1:255–69. doi: 10.2174/1874467210801030255
29. Renz BW, Takahashi R, Tanaka T, Macchini M, Hayakawa Y, Dantes Z, et al. B2 adrenergic-neurotrophin feedforward loop promotes pancreatic cancer. Cancer Cell. (2018) 33:75–90.e7. doi: 10.1016/j.ccell.2017.11.007
30. Choi YD, Jung JY, Baek M, Khan S, Song PI, Ryu S, et al. Ape1 promotes pancreatic cancer proliferation through gfrα1/src/erk axis-cascade signaling in response to gdnf. Int J Mol Sci. (2020) 21(10):3586. doi: 10.3390/ijms21103586
31. Pingle SC, Matta JA, Ahern GP. Capsaicin receptor: trpv1 a promiscuous trp channel. Handb Exp Pharmacol. (2007) 179):155–71. doi: 10.1007/978-3-540-34891-7_9
32. Malin SA, Molliver DC, Koerber HR, Cornuet P, Frye R, Albers KM, et al. Glial cell line-derived neurotrophic factor family members sensitize nociceptors in vitro and produce thermal hyperalgesia in vivo. J Neurosci. (2006) 26:8588–99. doi: 10.1523/JNEUROSCI.1726-06.2006
33. Liddle RA. The role of transient receptor potential vanilloid 1 (Trpv1) channels in pancreatitis. Biochim Biophys Acta. (2007) 1772:869–78. doi: 10.1016/j.bbadis.2007.02.012
34. Gao L, Bo H, Wang Y, Zhang J, Zhu M. Neurotrophic factor artemin promotes invasiveness and neurotrophic function of pancreatic adenocarcinoma in vivo and in vitro. Pancreas. (2015) 44:134–43. doi: 10.1097/MPA.0000000000000223
35. Khalid S, Khan A, Shal B, Ali H, Kim YS, Khan S. Suppression of trpv1 and P2y nociceptors by honokiol isolated from magnolia officinalis in 3(Rd) degree burn mice by inhibiting inflammatory mediators. Biomedicine pharmacotherapy = Biomedecine pharmacotherapie. (2019) 114:108777. doi: 10.1016/j.biopha.2019.108777
36. Qin T, Li J, Xiao Y, Wang X, Gong M, Wang Q, et al. Honokiol suppresses perineural invasion of pancreatic cancer by inhibiting Smad2/3 signaling. Front Oncol. (2021) 11:728583. doi: 10.3389/fonc.2021.728583
37. Huang C, Li H, Xu Y, Xu C, Sun H, Li Z, et al. Bicc1 drives pancreatic cancer progression by inducing vegf-independent angiogenesis. Signal transduction targeted Ther. (2023) 8:271. doi: 10.1038/s41392-023-01478-5
38. Li TJ, Li H, Zhang WH, Xu SS, Jiang W, Li S, et al. Human splenic ter cells: A relevant prognostic factor acting via the artemin-gfrα3-erk pathway in pancreatic ductal adenocarcinoma. Int J Cancer. (2021) 148:1756–67. doi: 10.1002/ijc.33410
39. Qi J, Sun H, Zhang Y, Wang Z, Xun Z, Li Z, et al. Single-cell and spatial analysis reveal interaction of Fap(+) fibroblasts and Spp1(+) macrophages in colorectal cancer. Nat Commun. (2022) 13:1742. doi: 10.1038/s41467-022-29366-6
40. Alqahtani S. The antiplatelet activity of camel milk in healthy and aluminum chloride-intoxicated rats. Saudi J Biol Sci. (2022) 29:103369. doi: 10.1016/j.sjbs.2022.103369
41. Burkett JB, Doran AC, Gannon M. Harnessing prostaglandin E(2) signaling to ameliorate autoimmunity. Trends Immunol. (2023) 44:162–71. doi: 10.1016/j.it.2023.01.004
42. Ayala GE, Dai H, Powell M, Li R, Ding Y, Wheeler TM, et al. Cancer-related axonogenesis and neurogenesis in prostate cancer. Clin Cancer Res. (2008) 14:7593–603. doi: 10.1158/1078-0432.CCR-08-1164
43. Magnon C. Role of the autonomic nervous system in tumorigenesis and metastasis. Mol Cell Oncol. (2015) 2:e975643. doi: 10.4161/23723556.2014.975643
44. Amit M, Na'ara S, Gil Z. Mechanisms of cancer dissemination along nerves. Nat Rev Cancer. (2016) 16:399–408. doi: 10.1038/nrc.2016.38
45. Chen SH, Zhang BY, Zhou B, Zhu CZ, Sun LQ, Feng YJ. Perineural invasion of cancer: A complex crosstalk between cells and molecules in the perineural niche. Am J Cancer Res. (2019) 9:1–21.
46. Tan X, Sivakumar S, Bednarsch J, Wiltberger G, Kather JN, Niehues J, et al. Nerve fibers in the tumor microenvironment in neurotropic cancer-pancreatic cancer and cholangiocarcinoma. Oncogene. (2021) 40:899–908. doi: 10.1038/s41388-020-01578-4
47. Ma J, Jiang Y, Jiang Y, Sun Y, Zhao X. Expression of nerve growth factor and tyrosine kinase receptor a and correlation with perineural invasion in pancreatic cancer. J Gastroenterol Hepatol. (2008) 23:1852–9. doi: 10.1111/j.1440-1746.2008.05579.x
48. Gil Z, Cavel O, Kelly K, Brader P, Rein A, Gao SP, et al. Paracrine regulation of pancreatic cancer cell invasion by peripheral nerves. J Natl Cancer Institute. (2010) 102:107–18. doi: 10.1093/jnci/djp456
49. Nan L, Qin T, Xiao Y, Qian W, Li J, Wang Z, et al. Pancreatic stellate cells facilitate perineural invasion of pancreatic cancer via Hgf/C-met pathway. Cell Transplant. (2019) 28:1289–98. doi: 10.1177/0963689719851772
50. Qin T, Xiao Y, Qian W, Wang X, Gong M, Wang Q, et al. Hgf/C-met pathway facilitates the perineural invasion of pancreatic cancer by activating the Mtor/Ngf axis. Cell Death Dis. (2022) 13:387. doi: 10.1038/s41419-022-04799-5
51. Marchesi F, Piemonti L, Fedele G, Destro A, Roncalli M, Albarello L, et al. The chemokine receptor Cx3cr1 is involved in the neural tropism and Malignant behavior of pancreatic ductal adenocarcinoma. Cancer Res. (2008) 68:9060–9. doi: 10.1158/0008-5472.CAN-08-1810
52. Shen S, Wang Q, Wang X, Ding J, Chen F, Xiao Y, et al. Nodal enhances perineural invasion in pancreatic cancer by promoting tumor-nerve convergence. J healthcare Eng. (2022) 2022:9658890. doi: 10.1155/2022/9658890
53. Ito Y, Okada Y, Sato M, Sawai H, Funahashi H, Murase T, et al. Expression of glial cell line-derived neurotrophic factor family members and their receptors in pancreatic cancers. Surgery. (2005) 138:788–94. doi: 10.1016/j.surg.2005.07.007
54. Mulligan LM. Gdnf and the ret receptor in cancer: new insights and therapeutic potential. Front Physiol. (2018) 9:1873. doi: 10.3389/fphys.2018.01873
55. Chernichenko N, Omelchenko T, Deborde S, Bakst RL, He S, Chen CH, et al. Cdc42 mediates cancer cell chemotaxis in perineural invasion. Mol Cancer Res MCR. (2020) 18:913–25. doi: 10.1158/1541-7786.MCR-19-0726
56. Wang J, Wang H, Cai J, Du S, Xin B, Wei W, et al. Artemin regulates cxcr4 expression to induce migration and invasion in pancreatic cancer cells through activation of Nf-Kb signaling. Exp Cell Res. (2018) 365:12–23. doi: 10.1016/j.yexcr.2018.02.008
57. Xu Q, Wang Z, Chen X, Duan W, Lei J, Zong L, et al. Stromal-derived factor-1α/Cxcl12-Cxcr4 chemotactic pathway promotes perineural invasion in pancreatic cancer. Oncotarget. (2015) 6:4717–32. doi: 10.18632/oncotarget.v6i7
58. Guo K, Ma Q, Li J, Wang Z, Shan T, Li W, et al. Interaction of the sympathetic nerve with pancreatic cancer cells promotes perineural invasion through the activation of stat3 signaling. Mol Cancer Ther. (2013) 12:264–73. doi: 10.1158/1535-7163.MCT-12-0809
59. Hunt DL, Castillo PE. Synaptic plasticity of nmda receptors: mechanisms and functional implications. Curr Opin Neurobiol. (2012) 22:496–508. doi: 10.1016/j.conb.2012.01.007
60. Li L, Hanahan D. Hijacking the neuronal nmdar signaling circuit to promote tumor growth and invasion. Cell. (2013) 153:86–100. doi: 10.1016/j.cell.2013.02.051
61. Li F, He C, Yao H, Zhao Y, Ye X, Zhou S, et al. Glutamate from nerve cells promotes perineural invasion in pancreatic cancer by regulating tumor glycolysis through Hk2 Mrna-M6a modification. Pharmacol Res. (2023) 187:106555. doi: 10.1016/j.phrs.2022.106555
62. Na'ara S, Amit M, Gil Z. L1cam induces perineural invasion of pancreas cancer cells by upregulation of metalloproteinase expression. Oncogene. (2019) 38:596–608. doi: 10.1038/s41388-018-0458-y
63. Roger E, Martel S, Bertrand-Chapel A, Depollier A, Chuvin N, Pommier RM, et al. Schwann cells support oncogenic potential of pancreatic cancer cells through tgfβ Signaling. Cell Death Dis. (2019) 10:886. doi: 10.1038/s41419-019-2116-x
64. Furuhashi S, Sakaguchi T, Murakami T, Fukushima M, Morita Y, Ikegami K, et al. Tenascin C in the tumor-nerve microenvironment enhances perineural invasion and correlates with locoregional recurrence in pancreatic ductal adenocarcinoma. Pancreas. (2020) 49:442–54. doi: 10.1097/MPA.0000000000001506
65. Li X, Wang Z, Ma Q, Xu Q, Liu H, Duan W, et al. Sonic hedgehog paracrine signaling activates stromal cells to promote perineural invasion in pancreatic cancer. Clin Cancer Res. (2014) 20:4326–38. doi: 10.1158/1078-0432.CCR-13-3426
66. Zeng L, Guo Y, Liang J, Chen S, Peng P, Zhang Q, et al. Perineural invasion and tams in pancreatic ductal adenocarcinomas: review of the original pathology reports using immunohistochemical enhancement and relationships with clinicopathological features. J Cancer. (2014) 5:754–60. doi: 10.7150/jca.10238
67. Cavel O, Shomron O, Shabtay A, Vital J, Trejo-Leider L, Weizman N, et al. Endoneurial macrophages induce perineural invasion of pancreatic cancer cells by secretion of gdnf and activation of ret tyrosine kinase receptor. Cancer Res. (2012) 72:5733–43. doi: 10.1158/0008-5472.CAN-12-0764
68. Göhrig A, Detjen KM, Hilfenhaus G, Körner JL, Welzel M, Arsenic R, et al. Axon guidance factor slit2 inhibits neural invasion and metastasis in pancreatic cancer. Cancer Res. (2014) 74:1529–40. doi: 10.1158/0008-5472.CAN-13-1012
69. Saraswathibhatla A, Indana D, Chaudhuri O. Cell-extracellular matrix mechanotransduction in 3d. Nat Rev Mol Cell Biol. (2023) 24:495–516. doi: 10.1038/s41580-023-00583-1
70. Parmo-Cabañas M, Molina-Ortiz I, Matías-Román S, García-Bernal D, Carvajal-Vergara X, Valle I, et al. Role of metalloproteinases mmp-9 and mt1-mmp in cxcl12-promoted myeloma cell invasion across basement membranes. J Pathol. (2006) 208:108–18. doi: 10.1002/path.1876
71. Pantel K, Brakenhoff RH. Dissecting the metastatic cascade. Nat Rev Cancer. (2004) 4:448–56. doi: 10.1038/nrc1370
72. Watanabe R, Maeda T, Zhang H, Berry GJ, Zeisbrich M, Brockett R, et al. Mmp (Matrix metalloprotease)-9-producing monocytes enable T cells to invade the vessel wall and cause vasculitis. Circ Res. (2018) 123:700–15. doi: 10.1161/CIRCRESAHA.118.313206
73. Rowe R, Weiss SJ. Breaching the basement membrane: who, when and how? Trends Cell Biol. (2008) 18(11):560–74. doi: 10.1016/j.tcb.2008.08.007
74. Okada Y, Eibl G, Guha S, Duffy JP, Reber HA, Hines OJ. Nerve growth factor stimulates mmp-2 expression and activity and increases invasion by human pancreatic cancer cells. Clin Exp metastasis. (2004) 21:285–92. doi: 10.1023/B:CLIN.0000046131.24625.54
75. Okada Y, Eibl G, Duffy JP, Reber HA, Hines OJ. Glial cell-derived neurotrophic factor upregulates the expression and activation of matrix metalloproteinase-9 in human pancreatic cancer. Surgery. (2003) 134:293–9. doi: 10.1067/msy.2003.239
76. Tang D, Zhang J, Yuan Z, Gao J, Wang S, Ye N, et al. Pancreatic satellite cells derived galectin-1 increase the progression and less survival of pancreatic ductal adenocarcinoma. PloS One. (2014) 9:e90476. doi: 10.1371/journal.pone.0090476
77. Huang C, Li Y, Guo Y, Zhang Z, Lian G, Chen Y, et al. Mmp1/par1/sp/nk1r paracrine loop modulates early perineural invasion of pancreatic cancer cells. Theranostics. (2018) 8:3074–86. doi: 10.7150/thno.24281
78. Xu X, Lu X, Chen L, Peng K, Ji F. Downregulation of mmp1 functions in preventing perineural invasion of pancreatic cancer through blocking the nt-3/trkc signaling pathway. J Clin Lab Anal. (2022) 36:e24719. doi: 10.1002/jcla.24719
79. Yamada M, Hirabayashi K, Kawanishi A, Hadano A, Takanashi Y, Izumi H, et al. Nectin-1 expression in cancer-associated fibroblasts is a predictor of poor prognosis for pancreatic ductal adenocarcinoma. Surg Today. (2018) 48:510–6. doi: 10.1007/s00595-017-1618-3
80. Swanson BJ, McDermott KM, Singh PK, Eggers JP, Crocker PR, Hollingsworth MA. Muc1 is a counter-receptor for myelin-associated glycoprotein (Siglec-4a) and their interaction contributes to adhesion in pancreatic cancer perineural invasion. Cancer Res. (2007) 67:10222–9. doi: 10.1158/0008-5472.CAN-06-2483
81. Ceyhan GO, Demir IE, Altintas B, Rauch U, Thiel G, Müller MW, et al. Neural invasion in pancreatic cancer: A mutual tropism between neurons and cancer cells. Biochem Biophys Res Commun. (2008) 374:442–7. doi: 10.1016/j.bbrc.2008.07.035
82. Abiatari I, DeOliveira T, Kerkadze V, Schwager C, Esposito I, Giese NA, et al. Consensus transcriptome signature of perineural invasion in pancreatic carcinoma. Mol Cancer Ther. (2009) 8:1494–504. doi: 10.1158/1535-7163.MCT-08-0755
83. Schwann cells promote cancer cell invasion. Cancer Discovery. (2016) 6:473. doi: 10.1158/2159-8290.CD-RW2016-059
84. Kameda K, Shimada H, Ishikawa T, Takimoto A, Momiyama N, Hasegawa S, et al. Expression of highly polysialylated neural cell adhesion molecule in pancreatic cancer neural invasive lesion. Cancer Lett. (1999) 137:201–7. doi: 10.1016/S0304-3835(98)00359-0
85. Hong H, Choi HK, Yoon TY. Untangling the complexity of membrane protein folding. Curr Opin Struct Biol. (2022) 72:237–47. doi: 10.1016/j.sbi.2021.11.013
86. Nigri J, Gironella M, Bressy C, Vila-Navarro E, Roques J, Lac S, et al. Pap/reg3a favors perineural invasion in pancreatic adenocarcinoma and serves as a prognostic marker. Cell Mol Life Sci CMLS. (2017) 74:4231–43. doi: 10.1007/s00018-017-2579-9
87. Kadomatsu K, Muramatsu T. Midkine and pleiotrophin in neural development and cancer. Cancer Lett. (2004) 204:127–43. doi: 10.1016/S0304-3835(03)00450-6
88. Yao J, Hu XF, Feng XS, Gao SG. Pleiotrophin promotes perineural invasion in pancreatic cancer. World J Gastroenterol. (2013) 19:6555–8. doi: 10.3748/wjg.v19.i39.6555
89. Yao J, Zhang LL, Huang XM, Li WY, Gao SG. Pleiotrophin and N-syndecan promote perineural invasion and tumor progression in an orthotopic mouse model of pancreatic cancer. World J Gastroenterol. (2017) 23:3907–14. doi: 10.3748/wjg.v23.i21.3907
90. Xu M, Wang Y, Xia R, Wei Y, Wei X. Role of the ccl2-ccr2 signalling axis in cancer: mechanisms and therapeutic targeting. Cell proliferation. (2021) 54:e13115. doi: 10.1111/cpr.13115
91. Ferdoushi A, Li X, Griffin N, Faulkner S, Jamaluddin MFB, Gao F, et al. Schwann cell stimulation of pancreatic cancer cells: A proteomic analysis. Front Oncol. (2020) 10:1601. doi: 10.3389/fonc.2020.01601
92. Deborde S, Gusain L, Powers A, Marcadis A, Yu Y, Chen CH, et al. Reprogrammed schwann cells organize into dynamic tracks that promote pancreatic cancer invasion. Cancer Discovery. (2022) 12:2454–73. doi: 10.1158/2159-8290.CD-21-1690
93. Kim J, Park H, Kim H, Kim Y, Oh HJ, Chung S. Microfluidic one-directional interstitial flow generation from cancer to cancer associated fibroblast. Acta biomaterialia. (2022) 144:258–65. doi: 10.1016/j.actbio.2022.03.044
94. Tassone P, Caruso C, White M, Tavares Dos Santos H, Galloway T, Dooley L, et al. The role of matrixmetalloproteinase-2 expression by fibroblasts in perineural invasion by oral cavity squamous cell carcinoma. Oral Oncol. (2022) 132:106002. doi: 10.1016/j.oraloncology.2022.106002
95. Wang ZH, Peng WB, Zhang P, Yang XP, Zhou Q. Lactate in the tumour microenvironment: from immune modulation to therapy. EBioMedicine. (2021) 73:103627. doi: 10.1016/j.ebiom.2021.103627
96. Pamies D, Sartori C, Schvartz D, González-Ruiz V, Pellerin L, Nunes C, et al. Neuroinflammatory response to tnfα and il1β Cytokines is accompanied by an increase in glycolysis in human astrocytes in vitro. Int J Mol Sci. (2021) 22(8):4065. doi: 10.3390/ijms22084065
97. Pundavela J, Roselli S, Faulkner S, Attia J, Scott RJ, Thorne RF, et al. Nerve fibers infiltrate the tumor microenvironment and are associated with nerve growth factor production and lymph node invasion in breast cancer. Mol Oncol. (2015) 9:1626–35. doi: 10.1016/j.molonc.2015.05.001
98. Hayakawa Y, Sakitani K, Konishi M, Asfaha S, Niikura R, Tomita H, et al. Nerve growth factor promotes gastric tumorigenesis through aberrant cholinergic signaling. Cancer Cell. (2017) 31:21–34. doi: 10.1016/j.ccell.2016.11.005
99. Xia Y, Wei Y, Li ZY, Cai XY, Zhang LL, Dong XR, et al. Catecholamines contribute to the neovascularization of lung cancer via tumor-associated macrophages. Brain behavior Immun. (2019) 81:111–21. doi: 10.1016/j.bbi.2019.06.004
100. Qiao G, Bucsek MJ, Winder NM, Chen M, Giridharan T, Olejniczak SH, et al. B-adrenergic signaling blocks murine cd8(+) T-cell metabolic reprogramming during activation: A mechanism for immunosuppression by adrenergic stress. Cancer immunology immunotherapy CII. (2019) 68:11–22. doi: 10.1007/s00262-018-2243-8
101. Qiao G, Chen M, Mohammadpour H, MacDonald CR, Bucsek MJ, Hylander BL, et al. Chronic adrenergic stress contributes to metabolic dysfunction and an exhausted phenotype in T cells in the tumor microenvironment. Cancer Immunol Res. (2021) 9:651–64. doi: 10.1158/2326-6066.CIR-20-0445
102. Kim-Fuchs C, Le CP, Pimentel MA, Shackleford D, Ferrari D, Angst E, et al. Chronic stress accelerates pancreatic cancer growth and invasion: A critical role for beta-adrenergic signaling in the pancreatic microenvironment. Brain behavior Immun. (2014) 40:40–7. doi: 10.1016/j.bbi.2014.02.019
103. Qian BZ, Pollard JW. Macrophage diversity enhances tumor progression and metastasis. Cell. (2010) 141:39–51. doi: 10.1016/j.cell.2010.03.014
104. Bakst RL, Xiong H, Chen CH, Deborde S, Lyubchik A, Zhou Y, et al. Inflammatory monocytes promote perineural invasion via ccl2-mediated recruitment and cathepsin B expression. Cancer Res. (2017) 77:6400–14. doi: 10.1158/0008-5472.CAN-17-1612
105. Sanford DE, Belt BA, Panni RZ, Mayer A, Deshpande AD, Carpenter D, et al. Inflammatory monocyte mobilization decreases patient survival in pancreatic cancer: A role for targeting the Ccl2/Ccr2 axis. Clin Cancer Res. (2013) 19:3404–15. doi: 10.1158/1078-0432.CCR-13-0525
106. Wu T, Dai Y. Tumor microenvironment and therapeutic response. Cancer Lett. (2017) 387:61–8. doi: 10.1016/j.canlet.2016.01.043
107. Vitale I, Manic G, Coussens LM, Kroemer G, Galluzzi L. Macrophages and metabolism in the tumor microenvironment. Cell Metab. (2019) 30:36–50. doi: 10.1016/j.cmet.2019.06.001
108. Hui L, Chen Y. Tumor microenvironment: sanctuary of the devil. Cancer Lett. (2015) 368:7–13. doi: 10.1016/j.canlet.2015.07.039
109. Hagemann T, Lawrence T, McNeish I, Charles KA, Kulbe H, Thompson RG, et al. "Re-educating" Tumor-associated macrophages by targeting Nf-Kappab. J Exp Med. (2008) 205:1261–8. doi: 10.1084/jem.20080108
110. Kalluri R, Zeisberg M. Fibroblasts in cancer. Nat Rev Cancer. (2006) 6:392–401. doi: 10.1038/nrc1877
111. Liu H, Ma Q, Xu Q, Lei J, Li X, Wang Z, et al. Therapeutic potential of perineural invasion, hypoxia and desmoplasia in pancreatic cancer. Curr Pharm design. (2012) 18:2395–403. doi: 10.2174/13816128112092395
112. Shin N, Son GM, Shin DH, Kwon MS, Park BS, Kim HS, et al. Cancer-associated fibroblasts and desmoplastic reactions related to cancer invasiveness in patients with colorectal cancer. Ann coloproctology. (2019) 35:36–46. doi: 10.3393/ac.2018.09.10
113. Paszek MJ, Zahir N, Johnson KR, Lakins JN, Rozenberg GI, Gefen A, et al. Tensional homeostasis and the Malignant phenotype. Cancer Cell. (2005) 8:241–54. doi: 10.1016/j.ccr.2005.08.010
114. Demir IE, Tieftrunk E, Schorn S, Saricaoglu ÖC, Pfitzinger PL, Teller S, et al. Activated schwann cells in pancreatic cancer are linked to analgesia via suppression of spinal astroglia and microglia. Gut. (2016) 65:1001–14. doi: 10.1136/gutjnl-2015-309784
116. Labernadie A, Kato T, Brugués A, Serra-Picamal X, Derzsi S, Arwert E, et al. A mechanically active heterotypic E-cadherin/N-cadherin adhesion enables fibroblasts to drive cancer cell invasion. Nat Cell Biol. (2017) 19:224–37. doi: 10.1038/ncb3478
117. Fullár A, Kovalszky I, Bitsche M, Romani A, Schartinger VH, Sprinzl GM, et al. Tumor cell and carcinoma-associated fibroblast interaction regulates matrix metalloproteinases and their inhibitors in oral squamous cell carcinoma. Exp Cell Res. (2012) 318:1517–27. doi: 10.1016/j.yexcr.2012.03.023
118. Thome JJ, Bickham KL, Ohmura Y, Kubota M, Matsuoka N, Gordon C, et al. Early-life compartmentalization of human T cell differentiation and regulatory function in mucosal and lymphoid tissues. Nat Med. (2016) 22:72–7. doi: 10.1038/nm.4008
119. Yang MW, Tao LY, Jiang YS, Yang JY, Huo YM, Liu DJ, et al. Perineural invasion reprograms the immune microenvironment through cholinergic signaling in pancreatic ductal adenocarcinoma. Cancer Res. (2020) 80:1991–2003. doi: 10.1158/0008-5472.CAN-19-2689
120. Renz BW, Tanaka T, Sunagawa M, Takahashi R, Jiang Z, Macchini M, et al. Cholinergic signaling via muscarinic receptors directly and indirectly suppresses pancreatic tumorigenesis and cancer stemness. Cancer Discovery. (2018) 8:1458–73. doi: 10.1158/2159-8290.CD-18-0046
121. Deborde S, Wong RJ. How schwann cells facilitate cancer progression in nerves. Cell Mol Life Sci CMLS. (2017) 74:4405–20. doi: 10.1007/s00018-017-2578-x
122. Honma Y, Araki T, Gianino S, Bruce A, Heuckeroth R, Johnson E, et al. Artemin is a vascular-derived neurotropic factor for developing sympathetic neurons. Neuron. (2002) 35:267–82. doi: 10.1016/S0896-6273(02)00774-2
123. Corfas G, Velardez MO, Ko CP, Ratner N, Peles E. Mechanisms and roles of axon-schwann cell interactions. J Neurosci. (2004) 24:9250–60. doi: 10.1523/JNEUROSCI.3649-04.2004
124. Parrinello S, Napoli I, Ribeiro S, Wingfield Digby P, Fedorova M, Parkinson DB, et al. Ephb signaling directs peripheral nerve regeneration through sox2-dependent schwann cell sorting. Cell. (2010) 143:145–55. doi: 10.1016/j.cell.2010.08.039
125. Foley K, Rucki AA, Xiao Q, Zhou D, Leubner A, Mo G, et al. Semaphorin 3d autocrine signaling mediates the metastatic role of annexin A2 in pancreatic cancer. Sci Signaling. (2015) 8:ra77. doi: 10.1126/scisignal.aaa5823
126. Higgins DMO, Caliva M, Schroeder M, Carlson B, Upadhyayula PS, Milligan BD, et al. Semaphorin 3a mediated brain tumor stem cell proliferation and invasion in egfrviii mutant gliomas. BMC Cancer. (2020) 20:1213. doi: 10.1186/s12885-020-07694-4
127. Lu D, Shang G, He X, Bai XC, Zhang X. Architecture of the sema3a/plexina4/neuropilin tripartite complex. Nat Commun. (2021) 12:3172. doi: 10.1038/s41467-021-23541-x
128. Bressy C, Lac S, Nigri J, Leca J, Roques J, Lavaut MN, et al. Lif drives neural remodeling in pancreatic cancer and offers a new candidate biomarker. Cancer Res. (2018) 78:909–21. doi: 10.1158/0008-5472.CAN-15-2790
129. Mauffrey P, Tchitchek N, Barroca V, Bemelmans AP, Firlej V, Allory Y, et al. Progenitors from the central nervous system drive neurogenesis in cancer. Nature. (2019) 569:672–8. doi: 10.1038/s41586-019-1219-y
130. Lu R, Fan C, Shangguan W, Liu Y, Li Y, Shang Y, et al. Neurons generated from carcinoma stem cells support cancer progression. Signal transduction targeted Ther. (2017) 2:16036. doi: 10.1038/sigtrans.2016.36
131. Felsenstein M, Lindhammer F, Feist M, Hillebrandt KH, Timmermann L, Benzing C, et al. Perineural invasion in pancreatic ductal adenocarcinoma (Pdac): A saboteur of curative intended therapies? J Clin Med. (2022) 11(9):2367. doi: 10.3390/jcm11092367
132. Selvaggi F, Mascetta G, Daskalaki D, dal Molin M, Salvia R, Butturini G, et al. Outcome of superior mesenteric-portal vein resection during pancreatectomy for borderline ductal adenocarcinoma: results of a prospective comparative study. Langenbeck's Arch Surg. (2014) 399:659–65. doi: 10.1007/s00423-014-1194-6
133. Wang X, Zhang H, Wang T, Lau WY, Wang X, Sun J, et al. The concept and controversy of retroperitoneal nerve dissection in pancreatic head carcinoma (Review). Int J Oncol. (2015) 47:2017–27. doi: 10.3892/ijo.2015.3190
134. Nevala-Plagemann C, Hidalgo M, Garrido-Laguna I. From state-of-the-art treatments to novel therapies for advanced-stage pancreatic cancer. Nat Rev Clin Oncol. (2020) 17:108–23. doi: 10.1038/s41571-019-0281-6
135. Saloman JL, Albers KM, Li D, Hartman DJ, Crawford HC, Muha EA, et al. Ablation of sensory neurons in a genetic model of pancreatic ductal adenocarcinoma slows initiation and progression of cancer. Proc Natl Acad Sci United States America. (2016) 113:3078–83. doi: 10.1073/pnas.1512603113
136. Shen ZT, Zhou H, Li AM, Ji XQ, Jiang CC, Yuan X, et al. Clinical outcomes and prognostic factors of stereotactic body radiation therapy combined with gemcitabine plus capecitabine for locally advanced unresectable pancreatic cancer. J Cancer Res Clin Oncol. (2020) 146:417–28. doi: 10.1007/s00432-019-03066-z
137. Chatterjee D, Katz MH, Rashid A, Wang H, Iuga AC, Varadhachary GR, et al. Perineural and intraneural invasion in posttherapy pancreaticoduodenectomy specimens predicts poor prognosis in patients with pancreatic ductal adenocarcinoma. Am J Surg Pathol. (2012) 36:409–17. doi: 10.1097/PAS.0b013e31824104c5
138. Bakst RL, Lee N, He S, Chernichenko N, Chen CH, Linkov G, et al. Radiation impairs perineural invasion by modulating the nerve microenvironment. PloS One. (2012) 7:e39925. doi: 10.1371/journal.pone.0039925
139. Lu Z, Dong TH, Si PR, Shen W, Bi YL, Min M, et al. Continuous low-dose-rate irradiation of iodine-125 seeds inhibiting perineural invasion in pancreatic cancer. Chin Med J. (2016) 129:2460–8. doi: 10.4103/0366-6999.191777
140. Coveler AL, Mizrahi J, Eastman B, Apisarnthanarax SJ, Dalal S, McNearney T, et al. Pancreas cancer-associated pain management. oncologist. (2021) 26:e971–e82. doi: 10.1002/onco.13796
141. Brasca MG, Amboldi N, Ballinari D, Cameron A, Casale E, Cervi G, et al. Identification of N,1,4,4-tetramethyl-8-{[4-(4-methylpiperazin-1-yl)Phenyl]Amino}-4,5-dihydro-1h-pyrazolo[4,3-H]Quinazoline-3-carboxamide (Pha-848125), a potent, orally available cyclin dependent kinase inhibitor. J medicinal Chem. (2009) 52:5152–63. doi: 10.1021/jm9006559
142. Banh RS, Biancur DE, Yamamoto K, Sohn ASW, Walters B, Kuljanin M, et al. Neurons release serine to support mrna translation in pancreatic cancer. Cell. (2020) 183:1202–18.e25. doi: 10.1016/j.cell.2020.10.016
143. Koujima T, Tazawa H, Ieda T, Araki H, Fushimi T, Shoji R, et al. Oncolytic virus-mediated targeting of the erk signaling pathway inhibits invasive propensity in human pancreatic cancer. Mol Ther oncolytics. (2020) 17:107–17. doi: 10.1016/j.omto.2020.03.016
144. Silverman DA, Martinez VK, Dougherty PM, Myers JN, Calin GA, Amit M. Cancer-associated neurogenesis and nerve-cancer cross-talk. Cancer Res. (2021) 81:1431–40. doi: 10.1158/0008-5472.CAN-20-2793
145. Demir IE, Ceyhan GO, Liebl F, D'Haese JG, Maak M, Friess H. Neural invasion in pancreatic cancer: the past, present and future. Cancers. (2010) 2:1513–27. doi: 10.3390/cancers2031513
146. Borsekofsky S, Tsuriel S, Hagege RR, Hershkovitz D. Perineural invasion detection in pancreatic ductal adenocarcinoma using artificial intelligence. Sci Rep. (2023) 13:13628. doi: 10.1038/s41598-023-40833-y
147. Kartasalo K, Ström P, Ruusuvuori P, Samaratunga H, Delahunt B, Tsuzuki T, et al. Detection of perineural invasion in prostate needle biopsies with deep neural networks. Virchows Archiv an Int J Pathol. (2022) 481:73–82. doi: 10.1007/s00428-022-03326-3
148. Egevad L, Ström P, Kartasalo K, Olsson H, Samaratunga H, Delahunt B, et al. The utility of artificial intelligence in the assessment of prostate pathology. Histopathology. (2020) 76:790–2. doi: 10.1111/his.14060
149. Pantanowitz L, Quiroga-Garza GM, Bien L, Heled R, Laifenfeld D, Linhart C, et al. An artificial intelligence algorithm for prostate cancer diagnosis in whole slide images of core needle biopsies: A blinded clinical validation and deployment study. Lancet Digital Health. (2020) 2:e407–e16. doi: 10.1016/S2589-7500(20)30159-X
Keywords: pancreatic ductal adenocarcinoma, perineural invasion, tumor microenvironment, neural reprogramming, pain
Citation: Sun Y, Jiang W, Liao X and Wang D (2024) Hallmarks of perineural invasion in pancreatic ductal adenocarcinoma: new biological dimensions. Front. Oncol. 14:1421067. doi: 10.3389/fonc.2024.1421067
Received: 21 April 2024; Accepted: 01 July 2024;
Published: 25 July 2024.
Edited by:
Prasad Ravindra Dandawate, Abeda Inamdar Senior College, IndiaReviewed by:
Hindole Ghosh, University of Kansas Medical Center, United StatesCopyright © 2024 Sun, Jiang, Liao and Wang. This is an open-access article distributed under the terms of the Creative Commons Attribution License (CC BY). The use, distribution or reproduction in other forums is permitted, provided the original author(s) and the copyright owner(s) are credited and that the original publication in this journal is cited, in accordance with accepted academic practice. No use, distribution or reproduction is permitted which does not comply with these terms.
*Correspondence: Xiang Liao, bGlhb3hpYW5nMDI1QDEyNi5jb20=; Dongqing Wang, d2FuZ2RvbmdxaW5nNzFAMTYzLmNvbQ==
†These authors have contributed equally to this work and share first authorship
Disclaimer: All claims expressed in this article are solely those of the authors and do not necessarily represent those of their affiliated organizations, or those of the publisher, the editors and the reviewers. Any product that may be evaluated in this article or claim that may be made by its manufacturer is not guaranteed or endorsed by the publisher.
Research integrity at Frontiers
Learn more about the work of our research integrity team to safeguard the quality of each article we publish.