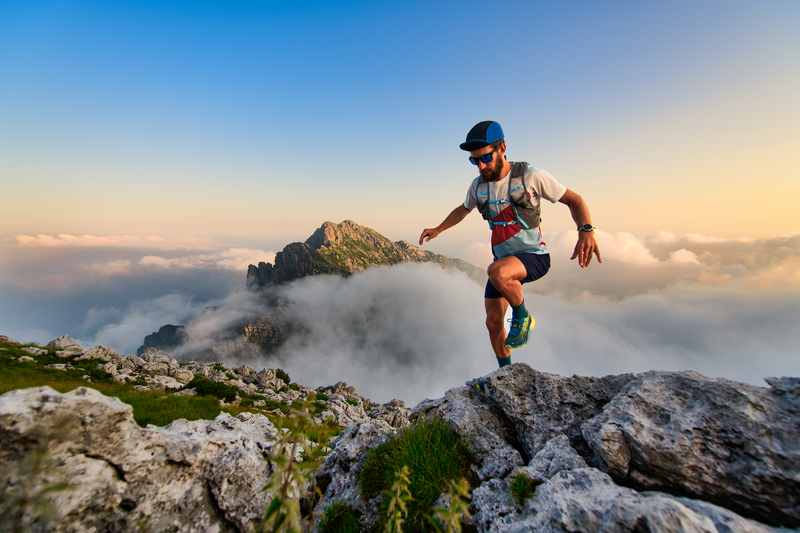
95% of researchers rate our articles as excellent or good
Learn more about the work of our research integrity team to safeguard the quality of each article we publish.
Find out more
MINI REVIEW article
Front. Oncol. , 19 April 2024
Sec. Gastrointestinal Cancers: Colorectal Cancer
Volume 14 - 2024 | https://doi.org/10.3389/fonc.2024.1392565
This article is part of the Research Topic The microbiome in the development of gastrointestinal diseases View all 17 articles
The intestinal tract encompasses one of the largest mucosal surfaces with a well-structured layer of intestinal epithelial cells supported by a network of underlying lamina propria immune cells maintaining barrier integrity. The commensal microflora in this environment is a major contributor to such functional outcomes due to its prominent role in the production of secondary metabolites. Of the several known metabolites of gut microbial origin, such as Short Chain Fatty Acids (SCFAs), amino acid derivatives, etc., secondary bile acids (BAs) are also shown to exhibit pleiotropic effects maintaining gut homeostasis in addition to their canonical role in dietary lipid digestion. However, dysbiosis in the intestine causes an imbalance in microbial diversity, resulting in alterations in the functionally effective concentration of these secondary metabolites, including BAs. This often leads to aberrant activation of the underlying lamina propria immune cells and associated signaling pathways, causing intestinal inflammation. Sustained activation of these signaling pathways drives unregulated cell proliferation and, when coupled with genotoxic stress, promotes tumorigenesis. Here, we aimed to discuss the role of secondary metabolites along with BAs in maintaining immune-gut homeostasis and regulation of inflammation-driven tumorigenesis with emphasis on the classical Wnt/β-Catenin signaling pathway in colon cancer.
Inflammatory Bowel Diseases (IBD) are a collection of chronic inflammatory disorders associated with the gastrointestinal tract consisting of Crohn’s Disease (CD) and Ulcerative Colitis (UC) (1). The presence of IBD also increases the risk of development of colon cancer by 20% at later stages of life (2). Prolonged inflammation, along with other epigenetic factors and a dysregulated immune system, can contribute to the development of Colorectal Cancer (CRC) (2). According to WHO, CRC is the third most prevalent cancer worldwide after breast and lung cancer until 2020 (3). More than 50% of new cases of CRC were reported in Asia, followed by Europe and North America. It is predicted that if the situation persists, then the estimated number of cases will increase from 1.88 million in 2020 to 2.94 million in 2040 globally (4).
Current CRC treatment regimens include chemotherapy, T-cell boosting therapeutics, oncolytic viral treatments, and non-coding RNA therapy. However, these improved treatments did have long-term side effects that can affect quality of life. Up to 85% of survivors treated with Oxaliplatin develop some degree of sensory neuropathy (5). Another survey found that around 20% of patients undergoing chemotherapy experienced grade 3/4 severe toxicities. A smaller percentage (<1%) suffers fatal toxicity, resulting in severe diarrhea, neutropenia, thrombocytopenia, or cardiac symptoms (6). The increased resistance to non-coding RNA therapy over time also poses a major challenge (7). Therefore, despite the developments in biologics, surgery remains one of the major treatment strategies for CRC patients, implying the need for alternative therapies with minimal to no side effects.
The intestinal microbial composition is closely associated with human health and disease. The human gut contains two compartments, the intestinal lumen and lamina propria, separated by the intestinal epithelial barrier. The luminal cavity is colonized by over 1000 species of microbes belonging to the domains Archaea, Bacteria, and Eukarya, which share a commensal relationship with cells of the host. The gut microbiome aids in various biological functions of the host system, such as fermentation of food, vitamin production, secondary metabolite synthesis, and regulation of immune responses (8). It has been reported that certain gut microbiota-derived secondary metabolites influence innate immune cells and non-hematopoietic components of the gut to maintain barrier integrity (9).
Prolonged disease conditions, a change in lifestyle and diet, and imprudent consumption of antibiotics result in gut microbial dysbiosis, subsequently disrupting intestinal homeostasis (9). The modern diet includes calorie-dense and nutritionally deficit options. Additionally, the increased consumption of ultra-processed foods (UPF) is also one of the leading factors contributing to the onset of IBD by reducing gut microbial diversity. Patients suffering from IBD, or gastrointestinal illness, along with medications, are often suggested a strict diet and healthy lifestyle. An appropriate dietary intervention can help in enhancing the effectiveness of the medication. Some dietary strategies have been found effective in improving disease activity and supporting clinical remission; however, some need further prospective evidence (10). For example, in a clinical study conducted in 2015, children suffering from Crohn’s disease were subject to a specific carbohydrate diet for 12-52 weeks. They showed reduced severity of the disease with respect to the Harvey-Bradshaw Index from 3.3 +/- 2.0 to 0.6 +/- 1.2 post-treatment (11).
In a healthy individual, the intestinal epithelial cell barrier can prevent the transmission of pathogens, proinflammatory substances, and antigens from the lumen to the internal environment (8). However, an imbalance in intestinal microbiota alters the tight intercellular junctions that allow pathogens and toxins (bacterial lipopolysaccharides, LPS) to cross the intestinal barrier, contributing to the activation of Pattern Recognition Receptors (PRRs) on lamina propria immune cells (12, 13). The intracellular signaling cascades triggered by these PRRs, which include Toll-like receptors (TLRs), RIG-I-like receptors (RLRs), NOD-like receptors (NLRs), and C-type lectin receptors (CLRs), upregulates the expression of inflammatory modulators. These modulators orchestrate the elimination of pathogens and affected cells. However, aberrant activation of this system also leads to the overproduction of immuno-oncogenic signals initiating tumorigenesis (13).
Cumulative studies illustrate that NLRs can negatively regulate cell differentiation and proliferation via the Wnt pathway in various cancers, including CRC (14). Similarly, TLR activation negatively regulates mesenchymal stem cell proliferation by disrupting canonical Wnt signaling by interrupting the expression of Wnt2, Wnt3, Wnt3a, and Wnt8 along with Frizzled Receptors (10). Wnt signaling is involved in the modulation of immune responses during inflammation, providing us with a potential drug target for CRC (12, 15). Therefore, through this review, we aim to shed light on the possible non-invasive methods to treat chronic intestinal inflammation and modulate the Wnt pathway using naturally occurring secondary metabolites of host gut-microbial origin.
CRC may result from one or more mechanisms such as chromosomal instability (CIN), CpG island methylator phenotype (CIMP), and microsatellite instability (MSI). The most studied mode of mechanistic progression is chromosomal instability, initiated by adenomatous polyposis coli (APC) mutations. Approximately 80% of CRC cases are a result of APC mutation. This mutation activates Wnt signaling mechanisms, increasing the transcription of several oncogenes (16) An interesting study conducted in 2020 revealed APC is also imperative for controlling Wnt-induced beta-catenin destruction complex recruitment in colonocytes to prevent aberrant cell proliferation and tumorigenesis (17), suggesting the involvement of Wnt signaling in CRC progression.
The Wnt/β-catenin pathway, Wnt/Ca2+ pathway, Wnt planar cell polarization pathway, and intracellular pathway that regulates spindle direction and asymmetric cell division are four major Wnt signaling pathways (18). The Wnt/β-catenin pathway displays a duality while modulating inflammation, possessing anti- and proinflammatory potential (14). In IBD’s pathophysiology, Wnt ligands secreted by activated immune cells bind to the Frizzled (Fzd), a G-protein coupled receptor, producing a proinflammatory tumor microenvironment (14, 19). Once Wnt ligands bind to membrane receptor Fzd and Lipoprotein-receptor related protein 5/6 (LRP5/6), they destabilize the β-Catenin degradation complex (GSK-3-β-APC-AXIN- β-Catenin). Accumulated β-Catenin translocates to the nucleus and triggers the TCF-4/LEF-1 (T cell factor/lymphoid enhancer factor) transcription factors to induce the expression of genes involved in cell cycle function and promote cell growth, differentiation, and metastasis (19).
A higher concentration of the Wnt ligands causes greater activation of the Wnt/β-Catenin signaling pathway, leading to increased cell proliferation. This uncontrolled cell proliferation or hypertrophy is followed by hyperplasia, causing an Epithelial-to-Mesenchymal (20) transition and increased cell motility, metastasis, and other related properties of cancer cells (21). Studies show that Wnt3a is the primary ligand involved in oral carcinogenesis, Wnt5a in breast tissue carcinogenesis, and Wnt3 is responsible for colon cancer proliferation (15, 21–23). Additionally, multiple types of cancer are known to be driven by uncontrolled expression of β-Catenin. β-Catenin expression is directly proportional to the depth of tumor infiltration (20). A swelling body of evidence suggests that β-Catenin inhibition suppresses tumor progression and recurrence.
During the clinical treatment of CRC, Wnt inhibitors are a common mode of therapy (24). The transcription factor SP1 (Specificity protein 1) is a crucial factor expressed in cell proliferation pathways (25). The direct interaction of SP1 with β-Catenin prevents the association of SP1 with degrading factors, thereby contributing to its stabilization (25). Interestingly, a study found suppression of the transcription factor SP1 by siRNAs truncated the growth of colon cancer stem cells (CCSCs) (21). Another transcription factor that promotes the proliferation of Wnt-driven colon cancer cells is SOX9. The regulation of gene expression by the Wnt/β-Catenin pathway results from the formation of a β-Catenin complex with the transcription factor TCF7 (T cell factor). TCF7 and SOX9 interact through nonDNA-contacting residues to produce a synergistic effect that encourages cancer cell proliferation (26). Inhibition of such factors can be a potential means of CRC therapeutics. These studies indicate that uncovering molecular targets within the Wnt/β-Catenin pathway will be capable of down-regulating CRC and related predisposing conditions such as IBD.
Culture-based studies show the dominance of Bacteroidetes and Firmicutes in the healthy gut, while Actinobacteria, Proteobacteria, and Verrucomicrobia are found in minor constituents. Reduction in diversity within the Firmicutes phylum is a major contributor to gut microbial dysbiosis causing IBD (8). Molecular cues of gut microbial origin regulating intestinal cell function are attributed to diversified small molecule metabolites (24). These metabolites are the intermediate or end products of host-gut bacterial metabolic processes. They are known to play a significant role in maintaining intestinal barrier integrity and intestinal immune homeostasis. Gut microbiota is widely involved in the metabolism of carbohydrates to generate SCFAs (27). Other majorly explored metabolites include tryptophan and indole derivatives, followed by primary and secondary BAs (27, 28).
Drastic imbalances in the composition of these metabolites have been observed in IBD and CRC patients. IBD patient fecal samples have a lower proportion of SCFA-producing bacteria, whereas mucolytic and pathogenic bacteria are found in abundance. Similarly, an increase in the population of sulfate-reducing bacteria, such as Desulfovibrio, is also found in IBD patient’s fecal samples. This increases the production of hydrogen sulfate, induces mucosal inflammation, and causes damage to the intestinal epithelial barrier (10). Moreover, IBD and IBD-associated cancers are known to cause malabsorption and reduction in the conversion of primary BAs to secondary BAs, thereby disrupting BA pool composition. Such changes pose a higher risk of infection as the mucosal integrity gets compromised (29).
Thus, their ability to behave as biomarkers and regulate metabolism and other homeostatic mechanisms makes them potential non-invasive therapeutic targets. (Supplementary Table 1).
Short Chain Fatty Acids (SCFAs) are crucial in maintaining intestinal barrier integrity, gut homeostasis, and colon health (30). These microbiota-derived SCFAs are the primary energy source for intestinal epithelial cells (IEC) in the digestive tract. The imbalance in SCFAs is known to contribute to intestinal inflammation and associated diseases (30). These SCFAs include butyrate, propionate, and acetate.
One of the significant SCFAs, butyrate, is produced by Faecalibacterium prausnitzii, Clostridium leptum, and Eubacterium rectaleand, among others, displays superior inhibitory efficacy against CRC proliferation (30). It is essential for human health as it is the primary energy source for colonocytes (31). Additionally, butyrate regulates CRC by inhibiting HDAC 1 and 3 in colon cancer cells and suppressing intestinal inflammation and ROS production (32). Butyrate activates GPR109A and inhibits Protein Kinase B and NF-κB signaling pathways to reverse intestinal epithelium barrier dysfunction (33). Furthermore, evidence shows that butyrate plays a vital role in controlling intestinal inflammation by stimulating the differentiation of Treg cells (34) and promoting an anti-tumor effect (35). It was also reported that C. butyricum species indirectly upregulates butyrate production, reduces the levels of β-Catenin, and regulates the Wnt pathway (36).
A study reported that butyrate facilitates M2 macrophage polarization. It was shown that ERK1/2 activation or blockade of Wnt secretion suppressed the beneficial effect of butyrate-primed macrophages on goblet cell function. Adoptive transfer of butyrate-induced M2 macrophages in a dextran sulfate sodium (DSS)-induced mice model of colitis showcased a significant improvement in mucosal layer integrity, mucus secretion, and goblet cell regeneration (37). It is also known that Butyrate stimulates bone formation via T Regulatory cell-mediated regulation of WNT10B expression (38).
A study by Beatrice et al. showed that butyrate inhibits CRC proliferation by autophagy-mediated degradation of β-Catenin. Apart from modulating cancer cell proliferation, the treatment with butyrate plays a significant role in autophagy. Interestingly, the study showed that butyrate promoted the binding between LC3 and β-Catenin, causing its sequestration. The ability of butyrate to inhibit the Wnt/β-Catenin pathway represents a new frontier of targeted cancer therapies (39).
Similarly, propionate, produced by Veillonella parvula, Bacteroides eggerthii, and Bacteroides fragilis in the gut, regulates intestinal homeostasis by promoting turnover of the epithelial cells and promoting barrier integrity (40). As a result, stem cells in intestinal crypts differentiate through the Wnt signaling pathway to replenish lost cells. The absence of propionate results in intestinal disbalance, triggering the unregulated proliferation of IECs (30). Valproic acid (VPA) was able to stimulate the differentiation of neuronal stem cells by activating Wnt3a and β-Catenin (18, 41). The fatty acid acetate aids in the acetylation of β-catenin, reducing the Wnt inhibitor SOX-1 and potentially increasing cell proliferation (14). Recently, another study showed β-hydroxybutyrate is capable of suppressing cancer, by inhibiting EMT via the Wnt/β-Catenin pathway (42). (Figure 1A).
Figure 1 Effect of gut microbiota-derived metabolites on Wnt-mediated cancer progression. (A) Short-chain Chain Fatty Acids include Butyrate, Propionate, and Acetate. Excess butyrate treatment can promote proteasomal degradation of Wnt ligand β-Catenin mediated by autophagy marker LC3. When propionate synthesis reduces, cell differentiation and polarization diminish, leading to a lower cell turnover and increased aberrant intestinal cell growth. Acetate-mediated acetylation of β-Catenin facilitates a decrease of SOX-1, allowing unchecked Wnt-mediated cell proliferation. (B) Lower tryptophan metabolism impairs the integrity of tight junctions and regulates Wnt metabolism by targeting Wnt 3 and β-Catenin. H2S nourishes aberrant cell proliferation via the Wnt pathway. (C) Bile Acids bind to the FXR receptor, resulting in the inhibition of the Wnt/β-Catenin pathway. However, few direct co-relations exist between BAs and Wnt signaling ligands and receptors. *Created in BioRender.com).
Numerous amino acid metabolites, including hydrogen sulfide (H2S) and indole metabolites, are produced due to the fermentation of proteins by the gut microbiota (28). Several studies have shown that tryptophan (Trp), mainly produced by E. coli, can downregulate cell proliferation by suppressing the Wnt signaling pathway, implying targeting tryptophan metabolism is a method of CRC treatment (42, 43). A clinical study was conducted on 117 participants comprising 79 CRC patients and 38 age- sex-and body mass index (BMI) matched healthy controls. It was observed that the indole/tryptophan ratio in fecal matter positively correlated to the mRNA expression of tight junction proteins like Zona Occuladins-1 in colon tissue samples collected from the respective participants, suggesting the involvement of Trp metabolites in the tumorigenesis of CRC in humans (43). Several studies have highlighted the role of wnt signaling in shaping immune cell functions. One of the key mechanisms by which Wnt-β-catenin signaling in DCs promotes immune suppression is through the induction of an immunoregulatory enzyme, IDO, thereby causing the degradation of the essential amino acid tryptophan into kynurenines (44). A study found that 1-Methyl-D-tryptophan Reduces Tumor CD133+ cells, Wnt/β-catenin and NF-κβp65 in Murine Pancreatic Adenocarcinoma.1-Methyl-D-tryptophan significantly modulates the regulatory cytokines in the tumor microenvironment, which significantly inhibited tumor growth and tumor immune escaping potency (45).
Studies indicate another predominant amino acid metabolite, H2S, an energy source for the metabolism of the colonic epithelium (46). H2S is produced by the action of Desulfovibrio, Escherichia, Bilophila, Porhyromonas, Prevotella, Corynebacterium, Veillonella, Helicobacter, and Clostridium on amino acids (27). Cell culture studies in HT 29 cells discovered the cytotoxic and genotoxic effects of H2S produced by sulfate-reducing bacteria. However, there has been conflicting data on the inhibitory and stimulatory effects of H2S on the proliferation and inflammation of CRC cells (47). Upon further analysis in Human Colon Cancer cell line SW480, the study found that the Wnt/β-Catenin pathway regulates Cystathionine-γ-lyase (CSE) on a transcriptional level, upon secretion responsible for increasing H2S liberation. Furthermore, when tumors were xenografted into nude mice models with a CSE/H2S knockdown, their tumor growth was reduced, implying that H2S plays a role in increasing colon cancer (48) (Figure 1B).
Another well-known host-gut microbiota-derived metabolite of interest are secondary BAs with numerous unknown functions other than role in dietary lipid digestion. BAs are the end-product of cholesterol metabolism generated in the liver by a chain of enzymatic reactions organized in two main metabolic pathways, known as “classic” and “alternative” (49). These liver pathways generate mainly two primary BAs, i.e., cholic acid and chenodeoxycholic acid (CA and CDCA). In hepatocytes, these primary BAs are conjugated with glycine (G) or taurine (T), giving rise to the bile salts. Conjugated BAs are secreted in the intestine, becoming the substrate of an array of bacterial enzymes (49). 7α-dehydroxylation of the OH in the C7 position, a reaction mediated by 7α-hydroxylase expressing bacteria such as Clostridium and Eubacterium, gives rise to two secondary BAs, i.e., mono-hydroxylated BAs like LCA from CDCA, and 3α-12α-di-hydroxylated BAs like DCA from CA. Additionally, the C7 β-epimerization of CDCA by Bacteroides, Clostridium, Escherichia, Eubacterium, and others originates the 7β epimer of CDCA, i.e., the 3α,7β-dihydroxy-5β-cholanoic acid, known as ursodeoxycholic acid (UDCA) (47, 48). The large majority of BA species that reach the terminal ileum are reabsorbed by the intestinal epithelial cells (IEC) and transported back to the liver through the portal vein, completing a cycle in the so-called “entero-hepatic circulation” (49).
BAs regulate mucosal homeostasis and inflammation by interacting directly with a family of receptors known as bile acid-activated receptors or bile acid receptors (BAR), which include Takeda G protein-coupled receptor 5 (TGR5) and nuclear receptors that include the Farnesoid X Receptor (FXR) and Vitamin D Receptor (VDR) (50). BA signaling is known to suppress the proinflammatory phenotype of intestinal cells by the reduced release of TNF-α, IL-1β, IL-6, or IL-12. Studies have also reported that BA stimulates the production of anti-inflammatory cytokines, promoting epithelial barrier renewal (28).
A study reported that secondary BAs, such as LCA’s derivatives, regulate the differentiation of Treg cells, contributing to the suppression of inflammation, maintaining immune homeostasis, and hence, predisposing stages of cancers like CRC (51). LCA is reported to activate VDR on CaCo-2 cells and significantly reduce IL-1β -induced IL-8 secretion by blocking NF-κB inflammatory signaling (52). Kubota et al., in their studies, found VDR mediated the attenuation of Dextran Sulfate Sodium (DSS) induced Colitis in mice fed with LCA (53). Oral administration of LCA suppressed histological injury in an early phase of DSS-induced Colitis in Vdr+/- mice, whereas no significant impact was observed on Vdr-/- mice, suggesting the physiological role of the LCA–VDR axis in intestinal homeostasis (53). Additionally, LCA-dependent PXR activation in epithelial cells promotes TGFβ expression and reduces TLR4-dependent proinflammatory cytokines production by diminishing TLR4 mRNA stability (54). TGR5 is one of the receptors activated by multiple BAs, with LCA being its most potent natural agonist (55). A study found that LCA-induced activation of TGR5 reduces adaptive immune response as there is increased recruitment of NK cells. Another study found that LCA stimulated intestinal epithelial growth in an organoid, as indicated by the increased expression of an intestinal stem cell marker. However, this improved barrier regeneration was lost when LCA was administered to a Tgr5-/- organoid, indicating that LCA-associated TGR5 activation is crucial for barrier integrity (55).
Multiple studies have reported the therapeutic role of another secondary BA, UDCA, in Colitis and colitis-associated cancer. UDCA exerts anti-inflammatory and cytoprotective effects in the AOM-DSS-induced colitis mouse model (55). UDCA has also been shown to prevent colon inflammation in rats treated with 2,4,6-trinitrobenzene sulfonic acid (56). Interestingly, deficiency or absence of the TGR5 receptor significantly reduces the modulatory effect of UDCA, both in vitro and in vivo. He et al. and other studies highlight that UDCA treatment can contribute to intestinal homeostasis by enhancing the intestinal mucosal layer, maintaining epithelial cell integrity, modulating the gut microenvironment, and attenuating intestinal inflammation (55). The collective observation suggests that elucidating the relationship between UDCA and the gut microbiome can be a novel therapeutic strategy for inflammation and inflammation-driven cancer.
DCA has been known to play a significant role in the induction of CRC development. In vivo experiments with APCmin/+ mice suggested that DCA contributes to CRC tumorigenesis by activating EGFR to promote a hyperproliferative effect on colorectal mucosa in DCA-fed mice (57). Ji-Yao et al. reported that oral administration of DCA to germ-free mice increased colonic Rspo3 mRNA levels, which function as ligands for LGR4 and LGR5 and potentiate the activation of the Wnt pathway. In primary myofibroblasts, DCA increases Rspo3 mRNA via TGR5 and mediates high-fat diet-induced intestinal epithelial proliferation (58). However, the impact of therapeutic BAs like UDCA and LCA and its derivatives on wnt regulation are largely unexplored. (Figure 1C).
Recent evidence indicates that the Wnt/β-catenin pathway regulates bile homeostasis, including bile synthesis, modification, and transport. Cholesterol synthesis occurs predominantly in periportal hepatocytes (59). CYP7A1 and CYP27, crucial rate-limiting enzymes of BA synthesis, are localized in the perivenous zone of the liver lobule and coincident with β-catenin activation. The close relationship between the two processes was seen in β-catenin KO mice subjected to a methionine and choline-deficient diet, identified by macro vesicular steatosis and fibrosis. Liver-specific β-catenin deletion resulted in increased steatosis, higher hepatic cholesterol accumulation, and jaundice, likely due to defects in cholesterol to bile conversion mechanism and the bile export system. Additionally, conditional β-catenin KO had higher hepatic total BA levels on methionine and choline-deficient and control diets, indicative of basal abnormalities in bile metabolism without β-catenin (60).
Chromatin immunoprecipitation (ChIP) assays showed that CYP27 is a transcriptional target of β-catenin. Similarly, β-catenin KO and LRP5/6 KO models had significantly suppressed expression of CYP7A1, suggesting the involvement of β-catenin in BA metabolism. Interestingly, further studies have found that the β-catenin interacts with FXR, a nuclear receptor that regulates the expression of CYP7A1 and BA efflux transporters. FXR deficiency increases epithelial permeability to luminal bacteria, thereby promoting Wnt/β-catenin signaling, and increasing intestinal inflammation (61).
The crosstalk between Wnt/β-catenin ligands and members of the nuclear receptor (NR) family has been considered a clinically and developmentally important research area of cancer biology. Mao J. et al., in their study, demonstrated that FXR knockdown promotes β-catenin/TCF4 complex formation and, subsequently, its binding ability to the corresponding promoter. Their data indicates a novel mechanism through which FXR expression is mediated during tumor progression, involving the Wnt pathway. Additionally, hepatic bile acid synthesis is downregulated by the activation of the FXR-FGF15/19 signaling pathway (62). Thus, FXR represents a novel Wnt signaling pathway modulator and a potential Wnt signaling cascade molecular target that may be exploited to achieve anti-tumor effects (63).
The role of Wnt signaling in tumorigenesis is predominantly studied in colorectal cancer, where several studies suggest targeting Wnt/β catenin to regulate tumor progression. However, a therapeutic treatment targeting the canonical Wnt pathway achieving efficacy and safety remains a major challenge. Considering the role of FXR in Wnt regulation and the ability of some BAs to activate FXR, understanding the downstream mechanism opens doors to promising hypotheses exploring the impact of BAs via the BAR in regulating pathogenic Wnt signaling and immune modulation in the intestinal inflammation and associated cancers. As new studies describing such processes and our understanding of signaling mechanisms deepen, we must screen for direct interactions between BAs and Wnt pathways with the goal of maintaining intestinal homeostasis. Overall, the development of novel combinatorial therapeutics of natural origin capable of reducing the risk of side effects and improving the treatment outcome in CRC and predisposing IBD is an essential stride.
SSK: Writing – review & editing, Writing – original draft, Conceptualization. AF: Writing – review & editing, Writing – original draft, Conceptualization. PS: Writing – review & editing. TJ: Writing – review & editing, Supervision, Funding acquisition, Conceptualization.
The author(s) declare that financial support was received for the research, authorship, and/or publication of this article. This work is supported by OPERA Award (BITS-Pilani, Hyderabad) and SERB-ECRA-001583.
We would like to thank all the authors for their contributions toward better understanding of the gut microbiota, secondary metabolites, and their implications in IBD as well as colon cancer, and apologize to those whose work has not been cited due to space constraints.
The authors declare that the research was conducted in the absence of any commercial or financial relationships that could be construed as a potential conflict of interest.
All claims expressed in this article are solely those of the authors and do not necessarily represent those of their affiliated organizations, or those of the publisher, the editors and the reviewers. Any product that may be evaluated in this article, or claim that may be made by its manufacturer, is not guaranteed or endorsed by the publisher.
The Supplementary Material for this article can be found online at: https://www.frontiersin.org/articles/10.3389/fonc.2024.1392565/full#supplementary-material
1. Seyedian SS, Nokhostin F, Malamir MD. A review of the diagnosis, prevention, and treatment methods of inflammatory bowel disease. J Med Life. (2019) 12:113–22. doi: 10.25122/jml-2018-0075
2. Hnatyszyn A, Hryhorowicz S, Kaczmarek-Ryś M, Lis E, Słomski R, Scott RJ, et al. Colorectal carcinoma in the course of inflammatory bowel diseases. Hereditary cancer in clinical practice. 17. (2019) 18. doi: 10.1186/s13053-019-0118-4
3. de Martel C, Georges D, Bray F, Jacques, Clifford GM. Global burden of cancer attributable to infections in 2018: a worldwide incidence analysis. Lancet Glob Health. (2020) 8:e180–90. doi: 10.1016/S2214-109X(19)30488-7
4. Morgan E, Arnold M, Gini A, Lorenzoni V, Cabasag CJ, Laversanne M, et al. Global burden of colorectal cancer in 2020 and 2040: incidence and mortality estimates from GLOBOCAN. GUT (2023) 72(2):338–44. doi: 10.1136/gutjnl-2022-327736
5. Kumar A, Gautam V, Sandhu A, Rawat K, Sharma A, Saha L. Current and emerging therapeutic approaches for colorectal cancer: A comprehensive review. World J gastrointestinal Surg. (2023) 15:495–519. doi: 10.4240/wjgs.v15.i4.495
6. Nguyen SM, Pham AT, Nguyen LM, Cai H, Tran TV, Shu XO, et al. Chemotherapy-induced toxicities and their associations with clinical and non-clinical factors among breast cancer patients in Vietnam. Curr Oncol (Toronto Ont.). (2022) 29:8269–84. doi: 10.3390/curroncol29110653
7. Winkle M, El-Daly SM, Fabbri M, Calin GA. Noncoding RNA therapeutics - challenges and potential solutions. Nat Rev Drug Discovery. (2021) 20:629–51. doi: 10.1038/s41573-021-00219-z
8. Álvarez J, Fernández Real JM, Guarner F, Gueimonde M, Rodríguez JM, Saenz de Pipaon M, et al. Gut microbes and health. Microbiota intestinal y salud. Gastroenterologia y hepatologia. (2021) 44:519–35. doi: 10.1016/j.gastrohep.2021.01.009
9. Li C, Liang Y, Qiao Y. Messengers from the gut: gut microbiota-derived metabolites on host regulation. Front Microbiol. (2022) 13:863407. doi: 10.3389/fmicb.2022.863407
10. Yan J, Wang L, Gu Y, Hou H, Liu T, Ding Y, et al. Dietary patterns and gut microbiota changes in inflammatory bowel disease: current insights and future challenges. Nutrients. (2022) 14:4003. doi: 10.3390/nu14194003
11. Aziz I, Branchi F, Pearson K, Priest J, Sanders DS. A study evaluating the bidirectional relationship between inflammatory bowel disease and self-reported non-celiac gluten sensitivity. Inflammatory bowel Dis. (2015) 21:847–53. doi: 10.1097/MIB.0000000000000335
12. Zhang Q, Yu J, Chen Q, Yan H, Du H, Luo W. Regulation of pathophysiological and tissue regenerative functions of MSCs mediated via the WNT signaling pathway (Review). Mol Med Rep. (2021) 24:648. doi: 10.3892/mmr.2021.12287
13. Franchi L, Warner N, Viani K, Nuñez G. Function of Nod-like receptors in microbial recognition and host defense. Immunol Rev. (2009) 227:106–28. doi: 10.1111/j.1600-065X.2008.00734.x
14. Jridi I, Canté-Barrett K, Pike-Overzet K, Staal FJT. Inflammation and wnt signaling: target for immunomodulatory therapy? Front Cell Dev Biol. (2021) 8:615131. doi: 10.3389/fcell.2020.615131
15. Nie X, Xia F, Liu Y, Zhou Y, Ye W, Hean P, et al. Downregulation of wnt3 suppresses colorectal cancer development through inhibiting cell proliferation and migration. Front Pharmacol. (2019) 10:1110. doi: 10.3389/fphar.2019.01110
16. Tariq K, Ghias K. Colorectal cancer carcinogenesis: a review of mechanisms. Cancer Biol Med. (2016) 13:120–35. doi: 10.28092/j.issn.2095-3941.2015.0103
17. Parker TW, Neufeld KL. APC controls Wnt-induced β-catenin destruction complex recruitment in human colonocytes. Sci Rep. (2020) 10:2957. doi: 10.1038/s41598-020-59899-z
18. Wang C, Chen Q, Xu H. Wnt/β-catenin signal transduction pathway in prostate cancer and associated drug resistance. Discover Oncol. (2021) 12:40. doi: 10.1007/s12672-021-00433-6
19. Arensman MD, Kovochich AN, Kulikauskas RM, Lay AR, Yang PT, Li X, et al. WNT7B mediates autocrine Wnt/β-catenin signaling and anchorage-independent growth in pancreatic adenocarcinoma. Oncogene. (2014) 33:899–908. doi: 10.1038/onc.2013.23
20. Gao ZH, Lu C, Wang MX, Han Y, Guo LJ. Differential β-catenin expression levels are associated with morphological features and prognosis of colorectal cancer. Oncol Lett. (2014) 8:2069–76. doi: 10.3892/ol.2014.2433
21. Mir R, Sharma A, Pradhan SJ, Galande S. Regulation of transcription factor SP1 by the β-catenin destruction complex modulates wnt response. Mol Cell Biol. (2018) 38:e00188–18. doi: 10.1128/MCB.00188-18
22. Reyes M, Peña-Oyarzun D, Maturana A, Torres VA. Nuclear localization of β-catenin and expression of target genes are associated with increased Wnt secretion in oral dysplasia. Oral Oncol. (2019) 94:58–67. doi: 10.1016/j.oraloncology.2019.05.010
23. Roarty K, Baxley SE, Crowley MR, Frost AR, Serra R. Loss of TGF-beta or Wnt5a results in an increase in Wnt/beta-catenin activity and redirects mammary tumour phenotype. Breast Cancer research: BCR. (2009) 11:R19. doi: 10.1186/bcr2244
24. Novellasdemunt L, Antas P, Li VS. Targeting Wnt signaling in colorectal cancer. A Review in the Theme: Cell Signaling: Proteins, Pathways and Mechanisms. Am J Physiol Cell Physiol. (2015) 309:C511–21. doi: 10.1152/ajpcell.00117.2015
25. Bajpai R, Nagaraju GP. Specificity protein 1: Its role in colorectal cancer progression and metastasis. Crit Rev oncology/hematol. (2017) 113:1–7. doi: 10.1016/j.critrevonc.2017.02.024
26. Ramakrishnan AB, Burby PE, Adiga K, Cadigan KM. SOX9 and TCF transcription factors associate to mediate Wnt/β-catenin target gene activation in colorectal cancer. J Biol Chem. (2023) 299:102735. doi: 10.1016/j.jbc.2022.102735
27. Rowland I, Gibson G, Heinken A, Scott K, Swann J, Thiele I, et al. Gut microbiota functions: metabolism of nutrients and other food components. Eur J Nutr. (2018) 57:1–24. doi: 10.1007/s00394-017-1445-8
28. Wammers M, Schupp AK, Bode JG, Ehlting C, Wolf S, Deenen R, et al. Reprogramming of pro-inflammatory human macrophages to an anti-inflammatory phenotype by bile acids. Sci Rep. (2018) 8:255. doi: 10.1038/s41598-017-18305-x
29. He Q, Wu J, Ke J, Zhang Q, Zeng W, Luo Z, et al. & Lan, P. (2023). Therapeutic role of ursodeoxycholic acid in colitis-associated cancer via gut microbiota modulation. Mol Ther. (2023) 31:585–98. doi: 10.1016/j.ymthe.2022.10.014
30. Mirzaei R, Afaghi A, Babakhani S, Sohrabi MR, Hosseini-Fard SR, Babolhavaeji K, et al. Role of microbiota-derived short-chain fatty acids in cancer development and prevention. Biomedicine pharmacother = Biomedecine pharmacotherapie. (2021) 139:111619. doi: 10.1016/j.biopha.2021.111619
31. Chen G, Ran X, Li B, Li Y, He D, Huang B, et al. Sodium butyrate inhibits inflammation and maintains epithelium barrier integrity in a TNBS-induced inflammatory bowel disease mice model. EBioMedicine. (2018) 30:317–25. doi: 10.1016/j.ebiom.2018.03.030
32. Klepinina L, Klepinin A, Truu L, Chekulayev V, Vija H, Kuus K, et al. Colon cancer cell differentiation by sodium butyrate modulates metabolic plasticity of Caco-2 cells via alteration of phosphotransfer network. PloS One. (2021) 16:e0245348. doi: 10.1371/journal.pone.0245348
33. Chen J, Zhao KN, Vitetta L. Effects of intestinal microbial-Elaborated butyrate on oncogenic signaling pathways. Nutrients. (2019) 11:1026. doi: 10.3390/nu11051026
34. Furusawa Y, Obata Y, Fukuda S, Endo TA, Nakato G, Takahashi D, et al. Commensal microbe-derived butyrate induces the differentiation of colonic regulatory T cells. Nature. (2013) 504:446–50. doi: 10.1038/nature12721
35. Luu M, Weigand K, Wedi F, Breidenbend C, Leister H, Pautz S, et al. Regulation of the effector function of CD8+ T cells by gut microbiota-derived metabolite butyrate. Sci Rep. (2018) 8:14430. doi: 10.1038/s41598-018-32860-x
36. Chen D, Jin D, Huang S, Wu J, Xu M, Liu T, et al. Clostridium butyricum, a butyrate-producing probiotic, inhibits intestinal tumor development through modulating Wnt signaling and gut microbiota. Cancer Lett. (2020) 469:456–67. doi: 10.1016/j.canlet.2019.11.019
37. Liang L, Liu L, Zhou W, Yang C, Mai G, Li H, et al. Gut microbiota-derived butyrate regulates gut mucus barrier repair by activating the macrophage/WNT/ERK signaling pathway. Clin Sci (London England: 1979). (2022) 136:291–307. doi: 10.1042/CS20210778
38. Tyagi AM, Yu M, Darby TM, Vaccaro C, Li JY, Owens JA, et al. The microbial metabolite butyrate stimulates bone formation via T regulatory cell-mediated regulation of WNT10B expression. Immunity. (2018) 49:1116–1131.e7. doi: 10.1016/j.immuni.2018.10.013
39. Garavaglia B, Vallino L, Ferraresi A, Esposito A, Salwa A, Vidoni C, et al. Butyrate inhibits colorectal cancer cell proliferation through autophagy degradation of β-catenin regardless of APC and β-catenin mutational status. Biomedicines. (2022) 10:1131. doi: 10.3390/biomedicines10051131
40. Mirzaei R, Dehkhodaie E, Bouzari B, Rahimi M, Gholestani A, Hosseini-Fard SR, et al. Dual role of microbiota-derived short-chain fatty acids on host and pathogen. Biomedicine Pharmacother. (2022) 145:112352. doi: 10.1016/j.biopha.2021.112352
41. Zeng Q, Long Z, Feng M, Zhao Y, Luo S, Wang K, et al. Valproic acid stimulates hippocampal neurogenesis via activating the wnt/β-catenin signaling pathway in the APP/PS1/nestin-GFP triple transgenic mouse model of alzheimer’s disease. Front Aging Neurosci. (2019) 11:62. doi: 10.3389/fnagi.2019.00062
42. Dmitrieva-Posocco O, Wong AC, Lundgren P, Golos AM, Descamps HC, Dohnalová L, et al. β-Hydroxybutyrate suppresses colorectal cancer. Nature. (2022) 605:160–5. doi: 10.1038/s41586-022-04649-6
43. Sun XZ, Zhao DY, Zhou YC, Wang QQ, Qin G, Yao SK. Alteration of fecal tryptophan metabolism correlates with shifted microbiota and may be involved in pathogenesis of colorectal cancer. World J Gastroenterol. (2020) 26:7173–90. doi: 10.3748/wjg.v26.i45.7173
44. Suryawanshi A, Hussein MS, Prasad PD, Manicassamy S. Wnt signaling cascade in dendritic cells and regulation of anti-tumor immunity. Front Immunol. (2020) 11:122. doi: 10.3389/fimmu.2020.00122
45. Alahdal M, Xing Y, Tang T, Liang J. 1-Methyl-D-tryptophan Reduces Tumor CD133+ cells, Wnt/β-catenin and NF-κβp65 while Enhances Lymphocytes NF-κβ2, STAT3, and STAT4 Pathways in Murine Pancreatic Adenocarcinoma. Sci Rep. (2018) 8:9869. doi: 10.1038/s41598-018-28238-8
46. Beaumont M, Andriamihaja M, Lan A, Khodorova N, Audebert M, Blouin JM, et al. Detrimental effects for colonocytes of an increased exposure to luminal hydrogen sulfide: The adaptive response. Free Radical Biol Med. (2016) 93:155–64. doi: 10.1016/j.freeradbiomed.2016.01.028
47. Libiad M, Vitvitsky V, Bostelaar T, Bak DW, Lee HJ, Sakamoto N, et al. Hydrogen sulfide perturbs mitochondrial bioenergetics and triggers metabolic reprogramming in colon cells. J Biol Chem. (2019) 294:12077–90. doi: 10.1074/jbc.RA119.009442
48. Fan K, Li N, Qi J, Yin P, Zhao C, Wang L, et al. Wnt/β-catenin signaling induces the transcription of cystathionine-γ-lyase, a stimulator of tumor in colon cancer. Cell signalling. (2014) 26:2801–8. doi: 10.1016/j.cellsig.2014.08.023
49. Jia W, Wei M, Rajani C, Zheng X. Targeting the alternative bile acid synthetic pathway for metabolic diseases. Protein Cell. (2021) 12:411–25. doi: 10.1007/s13238-020-00804-9
50. Wan YY, Sheng L. Regulation of bile acid receptor activity☆. Liver Res. (2018) 2:180–5. doi: 10.1016/j.livres.2018.09.008
51. Hang S, Paik D, Yao L, Kim E, Trinath J, Lu J, et al. Bile acid metabolites control TH17 and Treg cell differentiation. Nature. (2019) 576:143–8. doi: 10.1038/s41586-019-1785-z
52. Sun J, Mustafi R, Cerda S, Chumsangsri A, Xia YR, Li YC, et al. Lithocholic acid down-regulation of NF-kappaB activity through vitamin D receptor in colonic cancer cells. J Steroid Biochem Mol Biol. (2008) 111:37–40. doi: 10.1016/j.jsbmb.2008.01.003
53. Kubota H, Ishizawa M, Kodama M, Nagase Y, Kato S, Makishima M, et al. Vitamin D receptor mediates attenuating effect of lithocholic acid on dextran sulfate sodium induced colitis in mice. Int J Mol Sci. (2023) 24:3517. doi: 10.3390/ijms24043517
54. Staudinger JL, Goodwin B, Jones SA, Hawkins-Brown D, MacKenzie KI, LaTour A, et al. The nuclear receptor PXR is a lithocholic acid sensor that protects against liver toxicity. Proc Natl Acad Sci United States America. (2001) 98:3369–74. doi: 10.1073/pnas.051551698
55. Pols TWH, Nomura M, Harach T, Lo Sasso G, Oosterveer MH, Thomas C, et al. TGR5 activation inhibits atherosclerosis by reducing macrophage inflammation and lipid loading. Cell Metab. (2011) 14:747–57. doi: 10.1016/j.cmet.2011.11.006
56. Ward JBJ, Lajczak NK, Kelly OB, O’Dwyer AM, Giddam AK, Ní Gabhann J, et al. Ursodeoxycholic acid and lithocholic acid exert anti-inflammatory actions in the colon. Am J Physiol Gastrointestinal liver Physiol. (2017) 312:G550–8. doi: 10.1152/ajpgi.00256.2016
57. Dong W, Liu L, Dou Y, Xu M, Liu T, Wang S, et al. Deoxycholic acid activates epidermal growth factor receptor and promotes intestinal carcinogenesis by ADAM17-dependent ligand release. J Cell Mol Med. (2018) 22:4263–73. doi: 10.1111/jcmm.13709
58. Li JY, Gillilland M, Lee AA, Wu X, Zhou SY, Owyang C. Secondary bile acids mediate high-fat diet-induced upregulation of R-spondin 3 and intestinal epithelial proliferation. JCI Insight. (2022) 7:e148309. doi: 10.1172/jci.insight.148309
59. Fiorucci S, Distrutti E. Chenodeoxycholic acid: an update on its therapeutic applications. Handb Exp Pharmacol. (2019) 256:265–82. doi: 10.1007/164_2019_226
60. Behari J, Yeh TH, Krauland L, Otruba W, Cieply B, Hauth B, et al. Liver-specific beta-catenin knockout mice exhibit defective bile acid and cholesterol homeostasis and increased susceptibility to diet-induced steatohepatitis. Am J Pathol. (2010) 176:744–53. doi: 10.2353/ajpath.2010.090667
61. Goel C, Monga SP, Nejak-Bowen K. Role and regulation of wnt/β-catenin in hepatic perivenous zonation and physiological homeostasis. Am J Pathol. (2022) 192:4–17. doi: 10.1016/j.ajpath.2021.09.007
62. Zheng L, Wen XL, Duan SL. Role of metabolites derived from gut microbiota in inflammatory bowel disease. World J Clin cases. (2022) 10:2660–77. doi: 10.12998/wjcc.v10.i9.2660
Keywords: gut microbiota, secondary metabolites, bile acids, inflammation, Wnt/β-catenin signaling, CRC
Citation: Kumar SS, Fathima A, Srihari P and Jamma T (2024) Host-gut microbiota derived secondary metabolite mediated regulation of Wnt/β-catenin pathway: a potential therapeutic axis in IBD and CRC. Front. Oncol. 14:1392565. doi: 10.3389/fonc.2024.1392565
Received: 27 February 2024; Accepted: 08 April 2024;
Published: 19 April 2024.
Edited by:
Ralf Weiskirchen, RWTH Aachen University, GermanyReviewed by:
Santhakumar Manicassamy, Augusta University, United StatesCopyright © 2024 Kumar, Fathima, Srihari and Jamma. This is an open-access article distributed under the terms of the Creative Commons Attribution License (CC BY). The use, distribution or reproduction in other forums is permitted, provided the original author(s) and the copyright owner(s) are credited and that the original publication in this journal is cited, in accordance with accepted academic practice. No use, distribution or reproduction is permitted which does not comply with these terms.
*Correspondence: Trinath Jamma, dHJpbmF0aEBoeWRlcmFiYWQuYml0cy1waWxhbmkuYWMuaW4=; a2FydW55YS5mcmllbmRAZ21haWwuY29t
†These authors have contributed equally to this work
Disclaimer: All claims expressed in this article are solely those of the authors and do not necessarily represent those of their affiliated organizations, or those of the publisher, the editors and the reviewers. Any product that may be evaluated in this article or claim that may be made by its manufacturer is not guaranteed or endorsed by the publisher.
Research integrity at Frontiers
Learn more about the work of our research integrity team to safeguard the quality of each article we publish.