- 1Henan University of Traditional Chinese Medicine, Zhengzhou, Henan, China
- 2Department of Occupational and Environmental Health, College of Public Health, Zhengzhou University, Zhengzhou, Henan, China
Pulmonary fibrosis is a fatal interstitial disease characterized by diffuse alveolitis, abnormal fibroblast proliferation, and extracellular matrix (ECM) accumulation, resulting in structural lung destruction and impaired lung function. Numerous studies have demonstrated that vitamins appear to play a crucial role in regulating inflammatory responses, cell differentiation, redox homeostasis, and collagen synthesis. Beyond their conventional nutritional functions, specific vitamins have recently been found to modulate various biological processes involved in pulmonary fibrosis. This study aims to provide a comprehensive overview of the current understanding regarding the impact of vitamins on pulmonary fibrotic disease.
1 Introduction
Pulmonary fibrosis is a chronic pulmonary disease characterized by the progressive accumulation of extracellular matrix (ECM) proteins, resulting in the formation of scar tissue and thickening of connective tissue, and the pathological process is often accompanied by persistent tissue inflammation (1). This refractory pulmonary disorder is characterized by progressive and irreversible destruction of lung structure caused by scar, ultimately leading to lung dysfunction, gas exchange obstruction, and respiratory failure (1). Currently, there are no effective therapeutic interventions available for pulmonary fibrosis apart from lung transplantation.
In recent years, emerging research of healthy diet and nutrition interventions in preventing or alleviating various chronic diseases leading to substantial pharmaceutical investments. Numerous studies have been devoted to demonstrating the intricate relationship between nutrients and diverse chronic diseases. For instance, dietary interventions play a pivotal role in effectively managing metabolic disorders such as diabetes mellitus (2); and dietary modifications hold substantial value in ameliorating renal failure (3); additionally, a well-established association exists between malnutrition and immune system dysregulation (4); furthermore, the association between nutrition and cancer has also garnered considerable interest (5). New evidence suggests that there may be a substantial link between dietary nutrient intake and lung health, as supported by epidemiological studies demonstrating that the consumption of antioxidant-rich foods can mitigate the risk of developing chronic lung disease (6–8). Additionally, the incorporation of fresh fruits and vegetables into one's diet appears to confer protection against lung disease.
Multivitamins, widely used as nutritional supplements, have garnered significant attention in the scientific community due to their potential for preventive or ameliorative effects on various chronic diseases. Many chronic diseases share common pathogenesis that can be modulated by vitamins, such as folic acid regulates DNA methylation (9), vitamin D governs bone mineral density (10), and both vitamin A and vitamin D regulate cell proliferation and differentiation (11, 12). In pulmonary fibrosis, a case-control study in Japan investigated the association between vegetable, fruit, grain, and fiber intake and the risk of idiopathic pulmonary fibrosis (IPF), it was observed that there is a beneficial association between higher fruit intake and IPF, although larger studies with more details information are warranted to confirm it (13). Moreover, numerous studies have found an association between hypovitaminosis and pulmonary fibrosis and attempted to explore the content characteristics of specific micronutrients (including vitamins) and the possibility of alleviation or treatment for pulmonary fibrosis (14, 15).
Despite numerous studies have investigated the roles of different vitamins in various pulmonary diseases over the past decades, there has been a limited coverage or even neglect of their specific protective activities in relation to pulmonary fibrosis within otherwise comprehensive reviews. This review aims to elucidate the pathophysiology of pulmonary fibrosis and provide a summary of the involvement of vitamins in the development and progression of this condition, thereby offering valuable insights into potential vitamin targets for pulmonary fibrosis.
2 Pathogenesis of pulmonary fibrosis
Tissue repair following injury is an inherent biological mechanism that restores tissue integrity through the organized replacement of damaged or deceased cells (16). However, dysregulation of this reparative process leads to excessive proliferation of myofibroblasts and aberrant secretion of ECM, resulting in permanent fibrous scarring at the site of tissue injury. Consequently, pulmonary fibrosis represents an uncontrolled hyper-reparative response to tissue wounds. The pathological process of pulmonary fibrosis can be roughly divided into four phases, as illustrated in Figure 1: the initial sustained injury phase, the inflammatory cell migration phase, the fibroblast migration/proliferation/activation phase and finally, the excessive deposition of ECM and fibrosis phase. The immediate pathological manifestations of pulmonary fibrosis involve injury to alveolar epithelial cells accompanied by secretion of inflammatory factors (17). There is efficient recruitment of inflammatory cells to the site of injury for clearance, concomitant with production of various cytokines and chemokines such as TGF-β, IL-1β, IL-13, TNF-α, etc., which stimulate proliferation and recruitment of lung fibroblasts. Myofibroblasts predominantly arise from three distinct pathways: (1) activation of interstitial fibroblasts; (2) epithelial mesenchymal transition (EMT); and (3) migration of bone marrow fibroblasts. Myofibroblasts secrete ECM, thereby facilitating wound contraction. While an appropriate ECM quantity aids in tissue damage repair, persistent injury leads to dysregulated excessive ECM deposition, resulting in the replacement of normal lung tissue with fibrous scars (18).
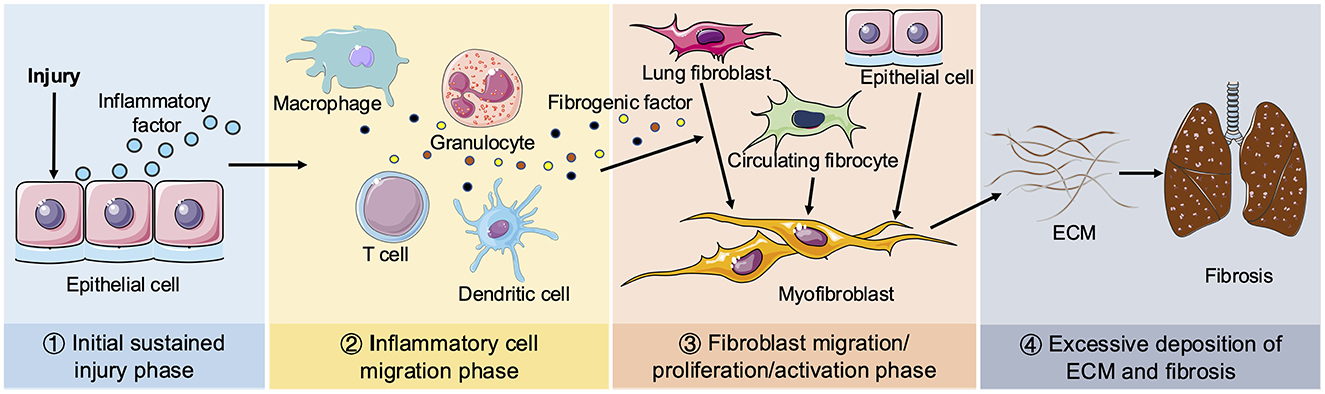
Figure 1. Schematic diagram of pulmonary fibrosis. Four phases of pathological process of pulmonary fibrosis: ① Initial sustained injury phase. ② Inflammatory cell migration phase. ③ Fibroblast migration/proliferation/activation phase. ④ Excessive deposition of ECM and fibrosis phage. When lungs are continuously stimulated by the external environment, epithelial cells release inflammatory mediators. Next, various inflammatory cells (including macrophages, granulocytes, T cells and dendritic cells, etc.) are recruited to the damaged tissue to remove foreign substances and secrete multiple fibrogenic factors, including TGF-β, IL-β1, IL-13, TNF-α. Circulating fibroblasts and interstitial fibroblasts subsequently proliferate and differentiate into myofibroblasts. Epithelial cells can also be transformed into myofibroblasts by EMT. Sustained injury causes the disorder of myofibroblasts secreting ECM to promote the wound repair process, resulting in excessive deposition of ECM and ultimate pulmonary fibrosis.
3 Discovery of vitamins
Vitamins are a group of organic substances that play an essential role as micronutrients in cellular metabolism and the overall maintenance of organismal health. In 1906, Frederick Hopkins first proposed the importance of vitamins through experiments. He demonstrated that rats fed only proteins, fats, carbohydrates, and minerals failed to grow properly, but exhibited improved growth when supplemented with a small amount of milk. This led him to suggest the existence of “accessory factors” in food that were crucial for life processes, in addition to the known major nutrients. These “accessory factors” were later identified as vitamins. In 1912, Casimir Funk coined the term “vitamine” (meaning “vital amine”) for these substances. When scientists subsequently discovered that not all vitamins contained amine structures, the final “e” was dropped, resulting in the modern term “vitamin” (19, 20). Vitamins can be classified into water-soluble types, such as vitamin B and C, and fat-soluble types, including vitamin A, D, E, and K. Each vitamin has distinct optimal food sources, and their deficiencies can cause a variety of diseases (Table 1).
4 Vitamins and pulmonary fibrosis
4.1 Vitamin A
Vitamin A, derived from carotenoid pigments in nature (known as provitamin A), belongs to the class of fat-soluble micronutrients and undergoes two consecutive oxidation reactions to convert into its primary biologically active derivatives, retinal and retinoic acid (RA). Vitamin A serves multiple crucial functions within the human body, encompassing cell proliferation and differentiation, vision, immunity, and embryological development (21–25). Moreover, it has been proved to be intricately associated with numerous respiratory defense mechanisms and assumes a pivotal role in upholding the structural integrity of respiratory mucosa (26, 27). Vitamin A is also necessary for maintaining alveolar structure and tissue regeneration, and trophic vitamin A deficiency (VAD) leads to detrimental histological alterations in lung parenchyma which predispose to severe tissue dysfunction and respiratory diseases, even increasing the incidence and morbidity of patients, suggesting that vitamin A plays an important role in adult lungs (28–30).
The excessive deposition of ECM is widely recognized as the primary characteristic of pulmonary interstitial fibrosis. Pathological changes in lung tissue induced by VAD encompass alterations in both the content and distribution of ECM proteins (31–33). Retinoid signaling directly or indirectly modulates gene promoters, thereby participating in the regulation of ECM protein expression and influencing the expression of ECM receptor proteins on cell membranes (34). Guillermo et al. observed elevated levels of collagen I and collagen IV in vitamin A-deficient rat lungs, accompanied by a nearly twofold increase in alveolar basement membrane thickness and ectopic deposition of collagen I protein, while treatment with retinoic acid reversed these alterations (35). The mechanism underlying the alteration of ECM by VAD remains elusive, but there is some evidence. Dysregulation of transforming growth factor-β1 (TGF-β1)/Smad3 signaling pathway is considered to play a central role and is associated with bleomycin-induced pulmonary fibrosis (36). Additionally, Chiharu et al. reported all-trans retinoic acid (ATRA) inhibits both proliferation and transdifferentiation of lung fibroblasts through downregulating TGF-β expression and suppressing the IL-6/IL-6R system (IL-6 stimulates lung fibroblast proliferation in a dose-dependent manner), thereby preventing radiation- and bleomycin-induced pulmonary fibrosis in mice (37). Inhibition of IL-6 and TGF-β production by ATRA was achieved by blocking the PKC-δ/NF-κB and p38MAPK/NF-κB pathways (38) and shifting the regulatory T/T helper 17 ratio and reducing the secretion of IL-17A (39). Vitamin A also affects the EMT process of lung epithelial cells, the effect of retinoic acid in inhibiting this process has been demonstrated (40, 41). In lungs of VAD rats, there is an increased level of N-cadherin protein accompanying decreased levels of E-cadherin and β-catenin (42). Supplementation with ATRA improves alveolar septal defect at the tissue level and promotes alveolar epithelial recovery (43). All these findings collectively suggest a potential association between vitamin A and EMT process in lung fibrosis.
Patients with lung fibrosis exhibit an elevated oxidative burden and heightened levels of reactive oxygen species (ROS) generated by inflammatory cells in lungs (44, 45). ROS have been demonstrated to activate transcription factors, such as AP-1, thereby inducing the synthesis of fibrogenic factor TGF-β, various ECM proteins, as well as type I and type IV collagens (46–48). Early research on the antioxidant properties of vitamin A came from Monaghan & Schmitt, who found that dissolving fresh vitamin A in linoleic acid hindered the absorption of oxygen by linoleic acid, and this effect faded away as vitamin A was oxidized (49). Burton and Ingold proposed that beta-carotene (which is converted into vitamin A in body) and other carotenoids may play an important role in protecting tissue lipids against peroxidation in vivo (50). Both carotenoids with provitamin A activity and non-vitamin A carotenoids exert antioxidant functions in lipid phases by quenching free radicals or O2 (51). Vitamin A and its metabolites have been frequently recognized as notable antioxidants in various tissues, including the lung, liver, and heart (52). Levels of retinol, one of the forms of vitamin A, are elevated in the bronchoalveolar lavage fluid of patients with IPF, and this increase may be part of an adaptive response to oxidative stress (14). Another study proved that VAD can disrupt the balance between ROS production and antioxidant defense mechanisms in lungs (31, 53). The above information suggests a potential association between vitamin A and pulmonary fibrosis through the redox pathway. However, experimental confirmation is required to determine whether vitamin A supplementation can effectively ameliorate pulmonary fibrosis via modulation of the redox pathway. It is worth noting that long-term excessive intake of vitamin A may disrupt the immune balance of the body (24, 54, 55). In the occurrence and development of pulmonary fibrosis, the imbalance of the immune system is an important factor (56). It is speculated from this that excessive intake of vitamin A may alter the functions and activities of immune cells, which indirectly aggravates pulmonary fibrosis.
4.2 Vitamin B
Vitamin B refers to a group of eight water-soluble vitamins that play crucial roles in cellular metabolism. Except for vitamin B3 (niacin), which can be partially synthesized in the human body from tryptophan, the other vitamins in this group cannot be synthesized by the body and must be obtained through dietary sources. All eight components: vitamin B1 (thiamine), vitamin B2 (riboflavin), vitamin B3 (niacin or niacinamide), vitamin B5 (pantothenic acid), vitamin B6 (pyridoxine, pyridoxal, or pyridoxamine, or pyridoxine hydrochloride), vitamin B7 (biotin), vitamin B9 (folic acid) and vitamin B12. Each individual component of vitamin B possesses its own distinct structure and exerts unique physiological functions within the human body.
4.2.1 Niacin
Research on vitamin B and pulmonary fibrosis has mainly focused on niacin at the end of the last century. Niacin serves as a precursor for nicotinamide adenine dinucleotide (NAD, a substrate for poly (ADPribose) polymerase) and nicotinamide adenine dinucleotide phosphate (NADP) and participates in DNA repair. In 1989, Wang et al. reported its potential anti-fibrosis function by attenuating bleomycin-induced thickened alveolar septa, accumulation of inflammatory cells and foci of fibrotic consolidation (57). DNA damage is one of the mechanisms of bleomycin-induced lung injury. One plausible mechanism involves the conversion of niacin into NAD in animals, thereby creating an environment with ample NAD supply that enhances the DNA repair capacity of poly (ADP-ribose) polymerase (a key enzyme regulating cell proliferation and repair in injured lungs) upon stimulation by bleomycin (57, 58). Calcium can activate phospholipase A2 (PLA2) to exacerbate pulmonary inflammatory events. Niacin, on the other hand, may inhibit bleomycin-induced pulmonary fibrosis by suppressing PLA2 activity through the prevention of calcium influx (59). Despite reports of fatty liver disease and growth inhibition in rats with long-term niacin administration, this compound is generally considered non-toxic because it is easily metabolized and excreted (60). In fact, niacin has been clinically utilized at supraphysiological doses as a vasodilator and cholesterol-lowering drug for lipid management (61, 62). Subsequently, numerous studies have analyzed the protective effect of niacin in combination with taurine on pulmonary fibrosis, and the possible mechanisms are as follows: 1) Niacin and taurine treatment suppressed bleomycin-induced transcriptional nuclear factor-NF-κB by preserving IκBα (63) to inhibit the increased levels of inflammatory cell-derived fibrocytokines such as IL-1α, IL-6, TNF-α and TGF-β (64). 2) Niacin and taurine treatment suppressed the expression of procollagen I and procollagen III at a transcription level (65), this may be related to the reduction of lung prolyl hydroxylase activity since the increased activity of this enzyme is positively correlated with and preferentially expressed in the accumulation of collagen in lungs (66). Additionally, niacin and taurine exhibited inhibition of nitric oxide production induced by iNOS in lungs (67).
4.2.2 Folic acid
Folic acid donates a carbon unit during DNA biosynthesis and is important for the regulation of gene expression, transcription, chromatin structure, genomic repair and genomic stability (68). No previous studies have considered the possible relationship between folic acid and pulmonary fibrosis even though DNA damage is involved in this disease. In recent years, studies have revealed an upregulation of folate receptor-β expression in alveolar macrophages of patients with IPF compared to normal alveolar macrophages, which mediates the unidirectional transport of folates into cells (69). Some high-risk factors for IPF disease, such as smoking, can lead to abnormal epithelial barrier and impede folate absorption in patients (40, 70). Furthermore, folate is required for the proliferation of fibroblasts and activated macrophages during pulmonary fibrosis. These facts seem to establish a link between folic acid and pulmonary fibrosis. Although inhibition of fibroblast proliferation is a way to reduce pulmonary fibrosis and it seems necessary to block folic acid absorption, cortisone-based drugs that block folic acid absorption have yielded unsatisfactory outcomes. Conversely, considering that oxidative stress plays a crucial role in the development of pulmonary fibrosis, folic acid as a highly reductive vitamin may have a favorable impact on its prevention or treatment. Our study showed that folic acid supplementation can promote the expression of SLC25A32 and MTHFD2, which are key enzymes in mitochondrial folate metabolism. It can enhance the mitochondrial folate metabolic pathway and reduce the production of mitochondrial ROS, thereby inhibiting the progression of pulmonary fibrosis (71). Another strategy worth considering is the conjugation of folic acid to the drug, enabling cellular entry and exertion of its effect through binding with folate receptors on activated macrophages (72). Of course, further experimental validation is necessary to elucidate the role of folic acid in pulmonary fibrosis.
B-group vitamins are water-soluble, the excessive amount is generally excreted out of the body through urine. This means that they are generally safe even at doses higher than the Recommended Dietary Allowance (RDA). Only three of the B-vitamins have been set an upper limit for daily intake, while the remaining ones are considered safe regardless of the intake dosage (73, 74). The first one is folic acid. Its Recommended Dietary Allowance (RDA) per day is typically between 200 and 400 micrograms, and the upper limit of its intake is generally set at 1,000 micrograms per day. This is simply because an increase in folic acid intake may mask the symptoms of vitamin B12 deficiency, allowing permanent damage related to the latter vitamin to accumulate unknowingly (75). It should also be noted that there is evidence suggesting that the intake of high doses of folic acid, and the resulting increase in the levels of unmetabolized folic acid, may have potential adverse effects on normal folic acid metabolism and immune function. However, up to now, there is no consensus on the blood concentration of folic acid that might cause harm (76). The second one is niacin, and its upper limit of intake is set at 35 milligrams (in the United States/Canada). This is simply because when the dosage exceeds 100 milligrams, it will cause temporary skin flushing. However, after long-term intake of a dosage of 1 gram or more, symptoms such as nausea, vomiting, and diarrhea have been observed, and in extremely rare cases, liver damage may also occur (77). The last B-vitamin with a set upper intake limit is vitamin B6. In the United States, its upper limit is set at 100 mg per day (roughly 75 times the Recommended Dietary Allowance), which is based on case reports showing that reversible sensory neuropathy occurs after long-term use of doses exceeding 1,000 mg (78). Currently, there are relatively few studies on the direct impact of an excess of B-group vitamins on pulmonary fibrosis. Overall, there is no conclusive evidence indicating that an excess of B-group vitamins can directly lead to pulmonary fibrosis or have a definite and severe adverse effect on it.
4.3 Vitamin C
Vitamin C, scientifically referred to as ascorbic acid, is an indispensable micronutrient and a coenzyme that potentiates the activity of diverse enzymes, contributing to the immune response and the development of nervous system in humans (79, 80). Severe deficiency of vitamin C can lead to the occurrence of many serious diseases. Due to the limited ability of organisms to preserve this essential nutrient, continuous intake is necessary to prevent deficiencies. Study has shown that silicosis patients exhibit reduced serum levels of vitamin C compared to individuals not exposed to silica (81). In studies investigating the efficacy of vitamin C treatment for pulmonary fibrosis, Vildan et al. demonstrated that vitamin C did not yield significant improvements in IPF symptoms in a rat model (82). However, other studies showed that administration of vitamins C and E both alleviate fibrotic damage in lung tissue, and with a more pronounced effect observed when these two vitamins are combined in rat models (83). Furthermore, the administration of vitamin C has been shown to protect against collagen I and α-SMA deposition (84). In general, the anti-pulmonary fibrosis effect of vitamin C is primarily manifested in three aspects: anti-oxidation, anti-cell death and anti-inflammation.
4.3.1 Anti-oxidation function
Oxidative stress caused by high concentration of oxygen in the lungs can lead to the dysfunction of alveolar epithelial cells, thereby causing certain damage to the lung tissue. Among small antioxidants, glutathione has garnered significant attention, however, as one of the main cellular antioxidants, vitamin C surpasses glutathione by directly detoxifying ROS. Vitamin C acts as a natural antioxidant by readily donating electrons to safeguard crucial biomolecules (proteins, lipids, carbohydrates, and nucleic acids) against oxidative damage arising from cellular metabolism (85). Furthermore, it reduces the content of ROS and reactive nitrogen species (RNS) by giving electrons to them and preventing the oxidation of other compounds (86). Vitamin C also regenerates other antioxidant molecules such as glutathione (GSH), beta-carotene, urate, alpha-tocopherol and membrane antioxidants glutathione and vitamin E (87–89). Despite being highly hydrophilic in nature, vitamin C effectively inhibits lipid oxidation by reducing tocopherol free radicals and thus maintaining optimal levels of vitamin E, a major fat-soluble antioxidant (90).
While these molecular mechanisms highlight vitamin C's antioxidative potential in pulmonary systems, emerging clinical evidence further explores its therapeutic implications for fibrotic lung diseases. Vitamin C supplementation for a duration of 12 weeks demonstrates significant reduction in serum protein carbonyl levels, which serves as a reliable marker for evaluating the antioxidant status in patients with IPF (91). However, whether vitamin C can definitively alleviate pulmonary fibrosis by lowering serum protein carbonyl levels still lacks concrete evidence. Additionally, it actively participates in collagen biosynthesis, acts as a cofactor for hydroxylases, and regulates gene expression (92, 93). In cystic fibrosis, vitamin C serves as a crucial source of glutathione and aids in maintaining the delicate balance between oxidative stress and antioxidant defense mechanisms within the lungs (94). Moreover, through its modulation of Nrf2/Nox4 redox equilibrium and TGF-β1/Smad3 signaling pathways, vitamin C exerts a protective effect against PQ-induced pulmonary fibrosis (95).
4.3.2 Anti-cell death function
The potential role of vitamin C in cell death has long been proposed (96), and significant advancements have been made across various types of cell death. Herein, we aim to concisely summarize these findings and elucidate the regulatory mechanisms by which vitamin C modulates cell death in pulmonary fibrosis.
4.3.2.1 Anti-apoptosis function
The prevailing perspective suggests that the development of pulmonary fibrosis necessitates repetitive damage to epithelial cells and subsequent apoptosis, with documented instances of alveolar epithelial cell (AEC) apoptosis in lung fibrotic lesions (97). Apoptosis of alveolar type II epithelial cells was also observed in bleomycin- or thoracic irradiation-treated mice (98, 99). Similarly, a study demonstrated that pro-apoptotic markers (p53, p21, Bax, and caspase-3) stained positive in type II AECs from IPF subjects, while anti-apoptotic markers (Bcl-2) exhibited reduced expression compared to control subjects (100). Moreover, the increased apoptosis of type II AECs in IPF patients can directly cause alveolar collapse, thus accelerating the progression of pulmonary interstitial fibrosis (101). In animal models, it has been reported that the activation of apoptosis in type II AEC promotes a fibrotic phenotype, for example, apoptotic cells may release cytokines and growth factors that recruit and activate fibroblasts, prompting them to secrete ECM, whereas inhibiting apoptosis helps to alleviate pulmonary fibrosis (102–104). Although the impact of vitamin C on type II AEC in the pathogenesis of pulmonary fibrosis remains limited, evidence suggests that vitamin C can enhance epithelial barrier function through diverse mechanisms. The administration of vitamin C can significantly alleviate the pro-inflammatory response and the aggregation of polymorphonuclear neutrophils in the lungs of infected mice, and effectively restore the function of the lung epithelial barrier that has been damaged by severe infections (105). Another, in an animal experiment on N-Nitrosodimethylamine (NDMA)-induced pulmonary fibrosis, ascorbic acid was observed to significantly reduce P53, caspase-3, Bax (which inhibits the anti-apoptotic effect of Bcl-2) and Bax/Bcl-2 ratio in lung tissue, while increasing Bcl-2 and mdm2 levels, thereby exerting anti-apoptotic properties (84). Heleen M et al. have also demonstrated the enhancement of vitamin C on the anti-apoptotic ability of Bcl-2 and its inhibitory effect on the expression of Bax protein, thereby impeding mitochondrial release of cytochrome C to the cytoplasm, ultimately reducing caspase-3 activity and suppressing cell apoptosis (106). However, further investigations are warranted to elucidate the mechanism by which vitamin C counteracts apoptosis in alveolar type II epithelial cells as well as its impact on apoptosis in other lung cell types.
4.3.2.2 Anti-ferroptosis
Ferroptosis, a newly discovered type of programmed cell death, occurs in an iron-dependent manner. As an indispensable trace element in the human body, the imbalance of iron homeostasis may lead to a variety of physiological disorders, including lung fibrotic disease (107). Histological samples from patients with IPF showed accumulation of extracellular iron and hemosiderin within macrophages, and this phenomenon is closely associated with reduced total lung capacity and lung compliance (107–110). In addition, iron overload was found to significantly increase lung inflammation and oxidative stress in mice, which are also crucial mechanisms underlying pulmonary fibrosis (111). In vitro, high iron level have been shown to directly stimulate the proliferation of human lung fibroblasts and enhance the secretion of ECM and pro-inflammatory factors (107). These findings suggest that there may be a close underlying association between iron overload and the development of pulmonary fibrosis. Ferroptosis will be triggered when iron overload surpasses the self-regulatory capacity of cells. Furthermore, ferroptosis can be inhibited by glutathione (a water-soluble antioxidant) or α-tocopherol (a lipid-soluble antioxidant), indicating that ferroptosis is not solely dependent on iron but is also closely associated with oxidative stress (112). Ferrostatin-1 and Liproxstatin-1, specific inhibitors of ferroptosis, work by inhibiting the accumulation of lipid hydroperoxides, which emphasizes the key role of lipid peroxidation in ferroptosis (113). Studies have demonstrated that enhancement of ROS and lipid peroxidation is one of the culprits in the elevation of α-SMA and COL1A1, indicative of myofibroblast formation. The effect of vitamin C on ferroptosis is concentration-dependent. At physiological concentrations, vitamin C behaves as a suppressant of ferroptosis induced by erastin or RSL3 (114). In contrast, at high concentrations, the cytotoxic effects of vitamin C outweigh its protective properties. Specific ferroptosis inhibitors were not effective for tumor cells (HT-1080/MCF-7) treated with ascorbic acid at pharmacologic concentration (114). However, the presence of pyruvate can effectively improve the inhibitory function of high concentration of ascorbic acid on ferroptosis (114). Ferroptosis agonists directly or indirectly affect glutathione peroxidase (GPXs) through various pathways, leading to a reduction in antioxidant capacity, accumulation of ROS, and ultimately oxidative cell death (115, 116). Based on this, it can be inferred that the anti-ferroptosis function of vitamin C at physiological concentrations may stem from its antioxidant property.
4.3.3 Anti-inflammation
The development of lung fibrosis is mostly the result of the development of prior chronic lung inflammation. Alveolar macrophages serve as the first cells to encounter external pathogens and irritants, initiating and subsequently resolving the lung immune response. In response to lung injury, macrophages activated by LPS and IFNγ undergo a transition to the pro-inflammatory M1 phenotype and begin to secrete pro-inflammatory cytokines and chemokines, resulting in enhanced chemotaxis of monocytes and neutrophils (117, 118). Simultaneously, neutrophils also release a plethora of inflammatory mediators. During chronic inflammation, myofibroblasts evade apoptosis and contribute to aberrant wound healing processes characterized by excessive extracellular matrix (ECM) production, ultimately culminating in pulmonary fibrosis. Therefore, reducing lung inflammation seems to be a feasible way to prevent lung tissue fibrosis and has been substantiated through scientific investigations. For example, Cabozantinib ameliorates lipopolysaccharide-induced lung inflammation in mice and inhibited bleomycin-induced early pulmonary fibrosis (119); Thymosin β4 suppresses LPS-induced murine lung fibrosis by alleviating inflammation (120).
The elasticity of the lung tissue in IPF patients is irreversibly reduced, which seriously affects the respiratory function (121). Masha et al. demonstrated although vitamin C, D, and E supplementation had no effect on exhaled parameters, it successfully improved inhaled parameters, especially total lung capacity (TLC) and residual volume (RV), which are the most critical indicators of lung function (122, 123). This implies that vitamins C, D, and E have the potential to enhance maximal oxygen uptake and inspiratory muscle strength in IPF patients. Previous studies have also demonstrated that vitamin C exerts anti-inflammatory effects through modulation of both adaptive and innate immunity (124, 125). Neutrophils are short-lived cells that continuously produced by the bone marrow and delivered to blood, and rapidly migrate into the inflamed tissue during an innate inflammation. Lymphocytes are long-lived cells that infiltrate tissue during an acquired inflammation. Studies have shown that vitamin C treatment reduces leukocyte recruitment in mice with pulmonary fibrosis by paraquat (126), which may indicate a direct relationship between vitamin C and immune cells. Nevertheless, data also showed that neutrophils accumulate a large amount of vitamin C, similarly, lymphocytes also accumulate vitamin C to regulate cell proliferation and the function of B cells and T cells (79), which help protect cells from oxidative stress damage during inflammation. IL-17, which has a variable inflammatory effect that promotes the migration of inflammatory cells to inflammatory tissues, levels of IL-17 were found to decrease in lung homogenates of fibrotic mice treated with vitamin C (126). Therefore, the therapeutic efficacy of vitamin C in treating pulmonary fibrosis appears to be attributed, at least in part, to the attenuation of pro-inflammatory factors secreted by resident or migratory cells.
It is noteworthy that in certain cases, vitamin C may reduce the body's immune response. High doses of vitamin C can inhibit the proliferation of natural killer cells as well as T and B lymphocytes responsible for the secretion of interleukin-2 (IL-2). Additionally, vitamin C can block the activation of T lymphocytes, which are forced into an immature state after stimulation by dendritic cells (127). However, there has been no research on whether excessive intake of vitamin C has an adverse impact on patients with pulmonary fibrosis.
4.4 Vitamin D
Vitamin D is widely distributed throughout the body, not only in the liver but also in various tissues. Numerous hydroxylases have been discovered in these tissues, facilitating the conversion of vitamin D to 1,25-dihydroxyvitamin D. This active form is believed to exert its primary function by binding to the ligand-dependent transcription factor known as the vitamin D receptor (VDR) (128–131). Vitamin D deficiency is a prominent characteristic of physiological aging, with its concentration declining as individuals age (132). Emerging research indicates that vitamin D insufficiency exacerbates cellular aging across various cell types. Supplementation with vitamin D has conventionally been postulated to mitigate the aging process in bone and muscle cells, thereby preserving or even enhancing their overall health (133–135). Vitamin D deficiency is strongly associated with various lung diseases, including asthma and chronic obstructive pulmonary disease (COPD). Low levels of 25-hydroxyvitamin D may contribute to the development or exacerbation of these conditions (136). Furthermore, there is evidence supporting a link between vitamin D deficiency and pulmonary fibrosis. For instance, Zhou et al. found that lung Sirt1 and serum vitamin D levels declined during physiological aging, thus activating the TIME signaling pathway, and promoting senescence-associated pulmonary fibrosis (137). Li et al. discovered that vitamin D deficiency aggravated bleomycin-induced pulmonary fibrosis (138). Notably, in this study, vitamin D appears to influence the development of pulmonary fibrosis through multiple biological pathways: vitamin D deficiency not only exacerbates the lung inflammation caused by bleomycin (similar to the effect observed in the case of vitamin C deficiency), but also increases the likelihood of the occurrence of EMT in the lungs, and these are all crucial steps in the pathogenesis of pulmonary fibrosis. Additionally, it exacerbates pulmonary fibrosis by activating the TGF-β/smad3 pathway (138). Furthermore, several small-scale prospective studies have consistently demonstrated a prevalent insufficiency or deficiency of plasma vitamin D levels in patients with IPF, which is strongly correlated with acute exacerbations of the disease (139, 140). However, it should be noted that there remains a dearth of large-scale randomized controlled trials investigating the potential role of vitamin D supplementation in improving IPF outcomes.
Conversely, vitamin D supplementation has demonstrated the potential to enhance pulmonary function and impede fibrosis progression. Studies have indicated that the inhibitory effect of vitamin D on pulmonary fibrosis manifests across various stages of disease advancement. (1) In the early stage, vitamin D plays an anticoagulant role. Epithelial cells undergo damage and subsequently release inflammatory mediators that activate the anti-fibrinolytic coagulation cascade. Furthermore, studies have revealed that the overactivation of the clotting cascade permeates the entire process of pulmonary fibrosis (141, 142). Coagulation factors are mainly mediated by protease activating receptors (PARs) (141). PARs mediate tissue factor TF, which then works with activating factor VIIa (FVIIa) to form the TF/FVIIa complex (the main promoter of the clotting cascade), and this pathway can be blocked by TFPI, a protease inhibitor (143, 144). Studies have shown that vitamin D may interfere with the above clotting response in two ways: Vitamin D inhibits TF expression through TNF-α (145–147); Vitamin D is potentially involved in the regulation of TFPI expression, as several studies have demonstrated a positive correlation between their levels (146). (2) Excessive inflammatory mediators trigger the infiltration of inflammatory cells, which then release a multitude of cytokines, such as transforming growth factors and fibroblast growth factors. These released factors, in turn, promote both inflammation and fibrosis (148–150). Previous studies have shown that inflammatory mediators play an important role in the occurrence and development of pulmonary fibrosis. Vitamin D has been shown to reduce serum levels of inflammatory cytokines, including IL-3 (151), IL-17 (151, 152), IL-1, IL-6, IL-8, and TNF-α (153), and may directly act on CD4+ T cells to promote the secretion of the anti-inflammatory cytokine IL-10 by T regulatory cells (Tregs), controlling the further expansion of the inflammatory response (151, 152, 154, 155). (3) The activation of the EMT process by TGF-β plays a pivotal role in the pathogenesis of pulmonary fibrosis. TGF-β binds to type I and II serine/threonine receptor kinases on the cell surface, leading to the phosphorylation of SMAD2 and SMAD3. Subsequently, the phosphorylated receptor kinases are released into the cytoplasm where they form a complex with SMAD4 before translocating into the nucleus. Once inside, this activated Smad complex binds to specific Smad binding elements within the genome to execute its regulatory function, such as modulating fibrin expression (156, 157). Studies have shown that vitamin D can inhibit TGF-β-Smad signaling pathway (156, 158–160) through the specific process of 1,25(OH)2D3 binding complex with VDR, which directly interacts with SMAD3 to reduce the binding of SMAD3 to DNA, and ultimately inhibit TGF-β-Smad signal transduction (161, 162). In fact, it has been repeatedly demonstrated that 1,25(OH)2D3 interferes with the pro-fibrotic effects of TGF-β, which could in fact be predicted because calcitriol itself inhibits collagen synthesis in cells (163, 164). Moreover, vitamin D can also block the expression of several matrix metalloproteinases in alveolar macrophages and monocytes and participate in airway remodeling, which helps to reduce the excessive degradation and abnormal remodeling of the extracellular matrix, thus playing a protective role in the airways (165, 166). Additionally, vitamin D can reduce the proliferation of lung fibroblasts through the TGF-β pathway, thus playing a role in inhibiting pulmonary fibrosis (165, 167). Other studies have shown that vitamin D concentrations were positively associated with improvements in lung function, including ΔFEV1(%), ΔFVC(%), and ΔDLCO-SB(%) (166). Additionally, the presence of severe muscle weakness in individuals with IPF is associated with an elevated risk of mortality and disease severity due to cachexia or sarcopenia (168, 169). Previous studies have demonstrated the efficacy of vitamin D supplementation in enhancing muscle strength among patients afflicted with pulmonary disorders (170, 171).
The report of the Institute of Medicine (IOM) of the United States in 2011 not only discussed the upper limits (ULs) of the intake of high-dose vitamin D preparations during acute and short-term administration within a limited time period, but also emphasized the long-term administration of vitamin D. Acute toxicity may occur when the dose of vitamin D exceeds 10,000 international units per day, which will cause the serum 25(OH)D concentration exceeding 150 ng/ml (>375 nmol/L). This level is significantly higher than the upper limit of 4,000 international units per day recommended by the IOM. Taking a dose of more than 4,000 international units of vitamin D per day for several years may lead to the serum 25(OH)D concentration being in the range of 50 to 150 ng/ml (125 to 375 nmol/L), which may have potential chronic toxicity (172). Due to the high prevalence of vitamin D deficiency secondary to exocrine pancreatic insufficiency, it is a common practice to supplement vitamin D in the population of patients with cystic fibrosis. Thomas et al. conducted a retrospective analysis of the medical records of all cystic fibrosis patients followed up at the Cliniques universitaires Saint-Luc over the past 10 years. The results showed that in this high-risk population, the number of cases of excessive vitamin D intake and toxicity increased due to dosage errors in the preparation of magistral liposoluble vitamin preparations. The serum vitamin D levels of 5% of the patients indicated excessive vitamin D intake, and another 2 patients developed severe hypercalcemia (173).
4.5 Vitamin E
Vitamin E encompasses a group of antioxidant fat-soluble vitamins, including α-, β-, γ- and δ-tocopherol as well as α-, β-, γ- and δ-tocotrienols, of which α-tocopherol stands out as the most biologically active form of vitamin E (174). The role of vitamin E in pulmonary fibrosis seems to be controversial, however, certain reports have suggested its inhibitory effect on this condition. Similar to vitamin C, vitamin E also has potent antioxidant properties that directly inhibit ROS formation in radiation fibrosis patients (82). Dietary supplementation of vitamin E significantly reduced the extent of TGF-β1, collagen deposition and histological damage following intratracheal amiodarone administration (175), and vitamin E reduced amiodarone-induced cytotoxicity in pulmonary cells, whereas other antioxidant treatments were ineffective (176). Dietary vitamin E supplementation enables rapid vitamin accumulation in lung tissue and mitigates amiodarone-induced elevation of TGF-β and hydroxyproline levels, thereby preventing lung tissue damage. Another recent study demonstrated that vitamin E significantly attenuates bleomycin-induced pulmonary fibrosis by improving mitochondrial structure and function, modulating iron metabolism, reducing inflammation (significantly reducing the transcriptional levels of interleukin-6 (Il-6), interleukin-33 (Il-33), chemokine ligand 5 (Ccl5) and TNF-α, as well as inhibiting the fibrotic effects on epithelial cells and fibroblasts (177). However, Card et al. point out that although dietary supplementation also significantly increased lung mitochondrial vitamin E content, it did not ameliorate the mitochondrial respiratory depression and disruption of mitochondrial membrane potential caused by amiodarone (178).
The role of vitamin E in cystic fibrosis disease remains a subject of intense debate within current research. In theory, vitamin E is involved in protecting the airways from oxidative stress and inflammation. Previous studies have demonstrated a decline in serum α-tocopherol levels during lung deterioration, which subsequently returned to normal following intravenous antibiotic treatment (179–181). Even within the normal range, reduced serum levels of vitamin E are associated with an elevated incidence of lung deterioration in cystic fibrosis (179). It can be inferred that low serum α-tocopherol levels may be attributed to pulmonary inflammation rather than dietary vitamin E deficiency. Therefore, the degree of chronic pulmonary inflammation should be considered when studying the relationship between pulmonary fibrosis and serum α-tocopherol. However, in a single-walled carbon nanotube-induced pulmonary fibrosis animal experiment, the researchers found that dietary vitamin E deficiency increased lung inflammation and oxidative stress. After feeding mice a vitamin E-adequate and a vitamin E-deficient diet, the researchers found that the vitamin E-deficient mice showed a 90-fold decrease of α-tocopherol in lung tissue, and a significant decrease of antioxidants and increased inflammatory response (182). However, Woestenenk et al. found that serum α-tocopherol deficiency was also rare in a large sample of children and adolescents with cystic fibrosis, although the actual vitamin intake was lower than recommended, and the study did not find a protective effect of higher serum α-tocopherol on lung function in patients with cystic fibrosis (183). The study further notes that the recommended amount of vitamin E supplementation for cystic fibrosis patients in both Europe and North America is higher than needed to prevent deficiency, and may even lead to abnormal vitamin E levels (183). Supplementation of vitamin E exceeding 400 mg/day in patients with cystic fibrosis has demonstrated deleterious effects, including an elevated risk of mortality in adults (184) and occurrence of hemorrhagic stroke (185).
4.6 Vitamin K
Acute exacerbations of IPF are characterized by histopathological features of diffuse alveolar damage (DAD) or hemorrhage (DAH), and studies have found that vitamin K deficiency exacerbates DAH symptoms (186–188). In addition, another study showed approximately 29% of individuals under the age of 18 with cystic fibrosis exhibit diminished levels of vitamin K (189). The risk of clinically relevant vitamin K deficiency is heightened by various factors, such as the use of oral anticoagulants or antibiotics, as well as reduced intestinal vitamin K uptake for multiple reasons, such as fat uptake inhibitors (190). Janssen R. proposed that vitamin K deficiency is one of the potential sources of pathogenesis of IPF. This proposition builds on Booth and colleagues' finding that there is a significant increase in vitamin K-dependent matrix Gla protein (MGP) expression in the lungs of IPF patients, indicating potential vitamin K deficiency (191). Vitamin K plays an important role in preventing tissue degeneration caused by the hardening or degradation of elastin and collagen (192). The vitamin K-activated MGP possesses the capability to penetrate the interior of elastin and collagen fibers, thereby playing a pivotal role in safeguarding ECM proteins against mineralization (193). Growth arrest-specific 6 (GAS6) and protein S are also vitamin K-dependent proteins, which are important regulators of tissue repair after injury and may be involved in the pathogenesis of pulmonary fibrosis (194). In addition to anticoagulant effects, protein S also has a protective effect on lung fibers through anti-apoptotic properties (195). Therefore, assessing the vitamin K status of individuals with pulmonary fibrosis and providing tailored dietary recommendations to enhance their vitamin K levels could potentially serve as a valuable intervention strategy, particularly for those who frequently experience infections (requiring antibiotic treatment) while concurrently using anticoagulant medications.
Since the primary deficiency disease associated with vitamin K is bleeding due to impaired blood clotting, it is commonly believed that high intake of vitamin K may increase the risk of thrombosis. However, in fact, excessive intake of vitamin K does not lead to more carboxylation of clotting factors. Even when monitored with the most sensitive technique (endogenous thrombin potential, ETP), an increased tendency to thrombosis has not been found in any of the participants (196). At present, there is a lack of evidence on whether high intake of vitamin K has an adverse effect on patients with pulmonary fibrosis.
5 The potential value, limitations and future research directions of vitamins in the treatment of pulmonary fibrosis
Vitamins exhibit various advantages in the treatment of pulmonary fibrosis. Their targets of action include oxidative stress, inflammatory responses, and fibrosis-related signaling pathways. The possible targets of action for each vitamin are listed in Table 2. With low toxicity, high cost-effectiveness, and the potential to synergize with traditional therapies (such as pirfenidone), vitamins have become promising adjuvant therapeutic agents. However, several limitations impede the clinical translation of vitamins. Currently, the evidence mainly comes from preclinical models or small-scale studies, lacking sufficient data on long-term efficacy, optimal dosages, or inter-individual variations. There are also some ambiguities in the mechanisms of action. For example, folic acid has a dual effect on fibroblast proliferation and the alleviation of oxidative stress. Moreover, some seemingly contradictory effects further complicate the application of vitamins. High doses of vitamin C may act as a pro-oxidant, and excessive vitamin E can increase the risk of bleeding. Biomarkers for predicting treatment responses (such as serum 25-hydroxyvitamin D for detecting vitamin D levels) remain underdeveloped, which restricts the formulation of personalized treatment regimens. Future research must prioritize large-scale randomized trials to verify the clinical efficacy of vitamins and establish standardized treatment protocols. Combination treatment regimens are worthy of in-depth exploration. For instance, the combination of vitamins C and E can enhance the antioxidant effect, or the use of folic acid-conjugated drugs can achieve targeted drug delivery to macrophages, thereby improving the therapeutic effect. In addition, safety assessment is essential. It is necessary to clarify the upper limit of the safe dosage and address the issue of drug interactions. Filling these research gaps will help to fully unleash the potential of vitamins in the treatment of pulmonary fibrosis.
6 Concluding remarks
New roles and uses for known substances are being discovered all the time, as are substances in our food. In the current era of escalating healthcare expenditures, cost-effective interventions hold great appeal. Numerous studies have demonstrated the significant role of vitamins in decelerating the progression of pulmonary fibrosis. Given the widespread psychological acceptance of vitamins as a relatively safe and economical natural compound, their utilization should be encouraged in regions with positive clinical data.
Author contributions
YQ: Writing – original draft, Writing – review & editing. YZ: Writing – review & editing.
Funding
The author(s) declare that no financial support was received for the research and/or publication of this article.
Conflict of interest
The authors declare that the research was conducted in the absence of any commercial or financial relationships that could be construed as a potential conflict of interest.
Generative AI statement
The author(s) declare that no Gen AI was used in the creation of this manuscript.
Publisher's note
All claims expressed in this article are solely those of the authors and do not necessarily represent those of their affiliated organizations, or those of the publisher, the editors and the reviewers. Any product that may be evaluated in this article, or claim that may be made by its manufacturer, is not guaranteed or endorsed by the publisher.
References
1. Martinez FJ, Collard HR, Pardo A, Raghu G, Richeldi L, Selman M, et al. Idiopathic pulmonary fibrosis. Nat Rev Dis Primers. (2017) 3:17074. doi: 10.1038/nrdp.2017.74
2. Xu X, Zhang F, Ren J, Zhang H, Jing C, Wei M, et al. Dietary intervention improves metabolic levels in patients with type 2 diabetes through the gut microbiota: a systematic review and meta-analysis. Front Nutr. (2023) 10:1243095. doi: 10.3389/fnut.2023.1243095
3. Rhee CM, Wang AY, Biruete A, Kistler B, Kovesdy CP, Zarantonello D, et al. Nutritional and dietary management of chronic kidney disease under conservative and preservative kidney care without dialysis. J Ren Nutr. (2023) 33:S56–66. doi: 10.1053/j.jrn.2023.06.010
4. Munteanu C, Schwartz B. The relationship between nutrition and the immune system. Front Nutr. (2022) 9:1082500. doi: 10.3389/fnut.2022.1082500
5. Hamaker ME, Oosterlaan F, van Huis LH, Thielen N, Vondeling A, van den Bos F. Nutritional status and interventions for patients with cancer-a systematic review. J Geriatr Oncol. (2021) 12:6–21. doi: 10.1016/j.jgo.2020.06.020
6. Gilliland FD. Children's lung function and antioxidant vitamin, fruit, juice, and vegetable intake. Am J Epidemiol. (2003) 158:576–84. doi: 10.1093/aje/kwg181
7. Miedema I, Feskens EJM, Heederik D, Kromhout D. Dietary determinants of long-term incidence of chronic nonspecific lung diseases. Am J Epidemiol. (1993) 138:37–45. doi: 10.1093/oxfordjournals.aje.a116775
8. Kelly Y, Sacker A, Marmot M. Nutrition and respiratory health in adults: findings from the Health Survey for Scotland. Eur Respir J. (2003) 21:664–71. doi: 10.1183/09031936.03.00055702
9. Ly A, Hoyt L, Crowell J, Kim Y-I. Folate and DNA methylation. Antioxid Redox Signal. (2012) 17:302–26. doi: 10.1089/ars.2012.4554
10. Reid IR, Bolland MJ, Grey A. Effects of vitamin D supplements on bone mineral density: a systematic review and meta-analysis. Lancet. (2014) 383:146–55. doi: 10.1016/S0140-6736(13)61647-5
11. Polcz ME, Barbul A. The role of vitamin A in wound healing. Nutr Clin Pract. (2019) 34:695–700. doi: 10.1002/ncp.10376
12. Mora JR, Iwata M, von Andrian UH. Vitamin effects on the immune system: vitamins A and D take centre stage. Nat Rev Immunol. (2008) 8:685–98. doi: 10.1038/nri2378
13. Miyake Y, Sasaki S, Yokoyama T, Chida K, Azuma A, Suda T, et al. Vegetable, fruit, and cereal intake and risk of idiopathic pulmonary fibrosis in Japan. Ann Nutr Metab. (2004) 48:390–7. doi: 10.1159/000082465
14. Markart P, Luboeinski T, Korfei M, Schmidt R, Wygrecka M, Mahavadi P, et al. Alveolar oxidative stress is associated with elevated levels of nonenzymatic low-molecular-weight antioxidants in patients with different forms of chronic fibrosing interstitial lung diseases. Antioxid Redox Signal. (2009) 11:227–40. doi: 10.1089/ars.2008.2105
15. Sriram N, Kalayarasan S, Sudhandiran G. Enhancement of antioxidant defense system by epigallocatechin-3-gallate during bleomycin induced experimental pulmonary fibrosis. Biol Pharm Bull. (2008) 31:1306–11. doi: 10.1248/bpb.31.1306
16. Wynn TA. Common and unique mechanisms regulate fibrosis in various fibroproliferative diseases. J Clin Invest. (2007) 117:524–9. doi: 10.1172/JCI31487
17. Zhang X-L, Xing R-G, Chen L, Liu C-R, Miao Z-G. PI3K/Akt signaling is involved in the pathogenesis of bleomycin-induced pulmonary fibrosis via regulation of epithelial-mesenchymal transition. Mol Med Rep. (2016) 14:5699–706. doi: 10.3892/mmr.2016.5960
18. Gharaee-Kermani M, Gyetko MR, Hu B, Phan SH. New insights into the pathogenesis and treatment of idiopathic pulmonary fibrosis: a potential role for stem cells in the lung parenchyma and implications for therapy. Pharm Res. (2007) 24:819–41. doi: 10.1007/s11095-006-9216-x
19. Semba RD. The discovery of the vitamins. Int J Vitam Nutr Res. (2012) 82:310–5. doi: 10.1024/0300-9831/a000124
21. De Luca LM. Retinoids and their receptors in differentiation, embryogenesis, and neoplasia. FASEB J. (1991) 5:2924–33. doi: 10.1096/fasebj.5.14.1661245
22. Livrea MA, Tesoriere L. Antioxidant activity of vitamin A within lipid environments. Subcell Biochem. (1998) 30:113–43. doi: 10.1007/978-1-4899-1789-8_5
23. Clagett-Dame M, Knutson D. Vitamin A in reproduction and development. Nutrients. (2011) 3:385–428. doi: 10.3390/nu3040385
24. Ross AC. Vitamin A and retinoic acid in T cell-related immunity. Am J Clin Nutr. (2012) 96:1166S−72S. doi: 10.3945/ajcn.112.034637
25. Sommer A, Vyas KS. A global clinical view on vitamin A and carotenoids. Am J Clin Nutr. (2012) 96:1204S−6S. doi: 10.3945/ajcn.112.034868
26. Wolbach SB, Howe PR. Tissue changes following deprivation of fat-soluble A vitamin. J Exp Med. (1925) 42:753–77. doi: 10.1084/jem.42.6.753
27. Pinnock CB, Douglas RM, Badcock NR. Vitamin A status in children who are prone to respiratory tract infections. Aust Paediatr J. (1986) 22:95–9. doi: 10.1111/j.1440-1754.1986.tb00197.x
28. Biesalski HK, Nohr D. Importance of vitamin-A for lung function and development. Mol Aspects Med. (2003) 24:431–40. doi: 10.1016/S0098-2997(03)00039-6
29. Karim T, Muhit M, Khandaker G. Interventions to prevent respiratory diseases - nutrition and the developing world. Paediatr Respir Rev. (2017) 22:31–7. doi: 10.1016/j.prrv.2016.09.003
30. Sommer A, Katz J, Tarwotjo I. Increased risk of respiratory disease and diarrhea in children with preexisting mild vitamin A deficiency. Am J Clin Nutr. (1984) 40:1090–5. doi: 10.1093/ajcn/40.5.1090
31. Esteban-Pretel G, Marín MP, Renau-Piqueras J, Barber T, Timoneda J. Vitamin A deficiency alters rat lung alveolar basement membrane Reversibility by retinoic acid. J Nutr Biochem. (2010) 21:227–36. doi: 10.1016/j.jnutbio.2008.12.007
32. Schwartz E, Zelig R, Parker A, Johnson S. Vitamin A supplementation for the prevention of bronchopulmonary dysplasia in preterm infants: an update. Nutr Clin Pract. (2017) 32:346–53. doi: 10.1177/0884533616673613
33. McGowan SE, Takle EJ, Holmes AJ. Vitamin A deficiency alters the pulmonary parenchymal elastic modulus and elastic fiber concentration in rats. Respir Res. (2005) 6:77. doi: 10.1186/1465-9921-6-77
34. Barber T, Esteban-Pretel G, Marín MP, Timoneda J. Vitamin a deficiency and alterations in the extracellular matrix. Nutrients. (2014) 6:4984–5017. doi: 10.3390/nu6114984
35. Esteban-Pretel G, Marín MP, Renau-Piqueras J, Sado Y, Barber T, Timoneda J. Vitamin A deficiency disturbs collagen IV and laminin composition and decreases matrix metalloproteinase concentrations in rat lung Partial reversibility by retinoic acid. J Nutr Biochem. (2013) 24:137–45. doi: 10.1016/j.jnutbio.2012.03.010
36. Song X, Liu W, Xie S, Wang M, Cao G, Mao C, et al. All-transretinoic acid ameliorates bleomycin-induced lung fibrosis by downregulating the TGF-β1/Smad3 signaling pathway in rats. Lab Invest. (2013) 93:1219–31. doi: 10.1038/labinvest.2013.108
37. Tabata C, Kubo H, Tabata R, Wada M, Sakuma K, Ichikawa M, et al. All-trans retinoic acid modulates radiation-induced proliferation of lung fibroblasts via IL-6/IL-6R system. Am J Physiol Lung Cell Mol Physiol. (2006) 290:L597–606. doi: 10.1152/ajplung.00282.2005
38. Leem AY, Shin MH, Douglas IS, Song JH, Chung KS, Kim EY, et al. All-trans retinoic acid attenuates bleomycin-induced pulmonary fibrosis via downregulating EphA2-EphrinA1 signaling. Biochem Biophys Res Commun. (2017) 491:721–6. doi: 10.1016/j.bbrc.2017.07.122
39. Dong Z, Tai W, Yang Y, Zhang T, Li Y, Chai Y, et al. The role of all-trans retinoic acid in bleomycin-induced pulmonary fibrosis in mice. Exp Lung Res. (2012) 38:82–9. doi: 10.3109/01902148.2011.646052
40. Woo Y-J, Jang KL. All-trans retinoic acid activates E-cadherin expression via promoter hypomethylation in the human colon carcinoma HCT116 cells. Biochem Biophys Res Commun. (2012) 425:944–9. doi: 10.1016/j.bbrc.2012.08.038
41. Gong L, Jiang L, Qin Y, Jiang X, Song K, Yu X. Protective effect of retinoic acid receptor α on hypoxia-induced epithelial to mesenchymal transition of renal tubular epithelial cells associated with TGF-β/MMP-9 pathway. Cell Biol Int. (2018) 42:1050–9. doi: 10.1002/cbin.10982
42. Timoneda J, Rodríguez-Fernández L, Zaragozá R, Marín MP, Cabezuelo MT, Torres L, et al. Vitamin A deficiency and the lung. Nutrients. (2018) 10:1132. doi: 10.3390/nu10091132
43. Uniyal S, Tyagi AK, Muyal JP. All trans retinoic acid (ATRA) progresses alveolar epithelium regeneration by involving diverse signalling pathways in emphysematous rat. Biomed Pharmacother. (2020) 131:110725. doi: 10.1016/j.biopha.2020.110725
44. Cantin AM, North SL, Fells GA, Hubbard RC, Crystal RG. Oxidant-mediated epithelial cell injury in idiopathic pulmonary fibrosis. J Clin Invest. (1987) 79:1665–73. doi: 10.1172/JCI113005
45. Psathakis K, Mermigkis D, Papatheodorou G, Loukides S, Panagou P, Polychronopoulos V, et al. Exhaled markers of oxidative stress in idiopathic pulmonary fibrosis. Eur J Clin Invest. (2006) 36:362–7. doi: 10.1111/j.1365-2362.2006.01636.x
46. Iglesias-De La Cruz MC, Ruiz-Torres P, Alcamí J, Díez-Marqués L, Ortega-Velázquez R, Chen S, et al. Hydrogen peroxide increases extracellular matrix mRNA through TGF-beta in human mesangial cells. Kidney Int. (2001) 59:87–95. doi: 10.1046/j.1523-1755.2001.00469.x
47. Douthwaite JA, Johnson TS, Haylor JL, Watson P, El Nahas AM. Effects of transforming growth factor-beta1 on renal extracellular matrix components and their regulating proteins. J Am Soc Nephrol. (1999) 10:2109–19. doi: 10.1681/ASN.V10102109
48. Fine A, Goldstein RH. The effect of transforming growth factor-beta on cell proliferation and collagen formation by lung fibroblasts. J Biol Chem. (1987) 262:3897–902. doi: 10.1016/S0021-9258(18)61441-3
49. Monaghan BR, Schmitt FO. The effects of carotene and of vitamin A on the oxidation of linoleic acid. J Biol Chem. (1932) 96:387–95. doi: 10.1016/S0021-9258(18)76278-9
50. Burton GW, Ingold KU. Beta-carotene: an unusual type of lipid antioxidant. Science. (1984) 224:569–73. doi: 10.1126/science.6710156
51. Sies H, Stahl W, Sundquist AR. Antioxidant functions of vitamins. Vitamins E and C, beta-carotene, and other carotenoids. Ann N Y Acad Sci. (1992) 669:7–20. doi: 10.1111/j.1749-6632.1992.tb17085.x
52. Palace VP, Khaper N, Qin Q, Singal PK. Antioxidant potentials of vitamin A and carotenoids and their relevance to heart disease. Free Radic Biol Med. (1999) 26:746–61. doi: 10.1016/S0891-5849(98)00266-4
53. Barber T, Borrás E, Torres L, Garcia C, Cabezuelo F, Lloret A, et al. Vitamin A deficiency causes oxidative damage to liver mitochondria in rats. Free Radic Biol Med. (2000) 29:1–7. doi: 10.1016/S0891-5849(00)00283-5
54. Chen G, Weiskirchen S, Weiskirchen R. Vitamin A: too good to be bad? Front Pharmacol. (2023) 14:1186336. doi: 10.3389/fphar.2023.1186336
55. Mawson AR, Croft AM. Multiple vaccinations and the enigma of vaccine injury. Vaccines. (2020) 8:676. doi: 10.3390/vaccines8040676
56. Yuan Y. Imbalance of dendritic cell function in pulmonary fibrosis. Cytokine. (2024) 181:156687. doi: 10.1016/j.cyto.2024.156687
57. Wang QJ, Giri SN, Hyde DM, Nakashima JM, Javadi I. Niacin attenuates bleomycin-induced lung fibrosis in the hamster. J Biochem Toxicol. (1990) 5:13–22. doi: 10.1002/jbt.2570050104
58. Hussain MZ, Giri SN, Bhatnagar RS. Poly(ADP-ribose) synthetase activity during bleomycin-induced lung fibrosis in hamsters. Exp Mol Pathol. (1985) 43:162–76. doi: 10.1016/0014-4800(85)90037-1
59. O'Neill CA, Giri SN. Effects of niacin on bleomycin-induced increases in myeloperoxidase, prolyl hydroxylase, and superoxide dismutase activities and collagen accumulation in the lungs of hamsters. J Biochem Toxicol. (1992) 7:229–39. doi: 10.1002/jbt.2570070406
60. Rikans LL, Arata D, Cederquist DC. Fatty livers produced in albino rats by excess niacin in high fat dietsi: alterations in enzyme and coenzyme systems induced by supplementing 40 percent fat diets with 0.1 percent of niacin. J Nutr. (1964) 82:83–7. doi: 10.1093/jn/82.1.83
61. Jacobson EL, Kim H, Kim M, Jacobson MK. Niacin: vitamin and antidyslipidemic drug. Subcell Biochem. (2012) 56:37–47. doi: 10.1007/978-94-007-2199-9_3
62. Guyton JR. Niacin in cardiovascular prevention: mechanisms, efficacy, and safety. Curr Opin Lipidol. (2007) 18:415–20. doi: 10.1097/MOL.0b013e3282364add
63. Giri SN. The combined treatment with taurine and niacin blocks the bleomycin-induced activation of nuclear factor-kappaB and lung fibrosis. Adv Exp Med Biol. (2003) 526:381–94. doi: 10.1007/978-1-4615-0077-3_47
64. Gurujeyalakshmi G, Wang Y, Giri SN. Taurine and niacin block lung injury and fibrosis by down-regulating bleomycin-induced activation of transcription nuclear factor-kappaB in mice. J Pharmacol Exp Ther. (2000) 293:82–90. doi: 10.1016/S0022-3565(24)39206-7
65. Gurujeyalakshmi G, Iyer SN, Hollinger MA, Giri SN. Procollagen gene expression is down-regulated by taurine and niacin at the transcriptional level in the bleomycin hamster model of lung fibrosis. J Pharmacol Exp Ther. (1996) 277:1152–7. doi: 10.1016/S0022-3565(25)12903-0
66. Wang QJ, Giri SN, Hyde DM, Li C. Amelioration of bleomycin-induced pulmonary fibrosis in hamsters by combined treatment with taurine and niacin. Biochem Pharmacol. (1991) 42:1115–22. doi: 10.1016/0006-2952(91)90296-H
67. Gurujeyalakshmi G, Wang Y, Giri SN. Suppression of bleomycin-induced nitric oxide production in mice by taurine and niacin. Nitric Oxide. (2000) 4:399–411. doi: 10.1006/niox.2000.0297
68. Stanger O, Semmelrock HJ, Wonisch W, Bös U, Pabst E, Wascher TC. Effects of folate treatment and homocysteine lowering on resistance vessel reactivity in atherosclerotic subjects. J Pharmacol Exp Ther. (2002) 303:158–62. doi: 10.1124/jpet.102.036715
69. Nagai T, Tanaka M, Hasui K, Shirahama H, Kitajima S, Yonezawa S, et al. Effect of an immunotoxin to folate receptor beta on bleomycin-induced experimental pulmonary fibrosis. Clin Exp Immunol. (2010) 161:348–56. doi: 10.1111/j.1365-2249.2010.04182.x
70. du Bois RM. Strategies for treating idiopathic pulmonary fibrosis. Nat Rev Drug Discov. (2010) 9:129–40. doi: 10.1038/nrd2958
71. Qu Y, Zhai R, Wang D, Wang Z, Hou G, Wu C, et al. Mitochondrial folate pathway regulates myofibroblast differentiation and silica-induced pulmonary fibrosis. J Transl Med. (2023) 21:365. doi: 10.1186/s12967-023-04241-0
72. Carron PM, Crowley A, O'Shea D, McCann M, Howe O, Hunt M, et al. Targeting the folate receptor: improving efficacy in inorganic medicinal chemistry. Curr Med Chem. (2018) 25:2675–708. doi: 10.2174/0929867325666180209143715
73. Rucker RB, Zempleni J, Suttie JW, McCormick DB. “Vitamin B12.” In: Handbook of Vitamins. 46. Boca Raton, FL: CRC Press (2012).
74. Zempleni J, Suttie JW, Gregory III JF, Stover PJ. “Pantothenic acid.” In: Handbook of Vitamins. 26. Boca Raton, FL: CRC Press (2013). doi: 10.1201/b15413
75. Institute Institute of Medicine (US) Standing Committee on the Scientific Evaluation of Dietary Reference Intakes and its Panel on Folate Other B Vitamins and Choline. Dietary Reference Intakes for Thiamin, Riboflavin, Niacin, Vitamin B6, Folate, Vitamin B12, Pantothenic Acid, Biotin, and Choline. National Academies Press (US), Washington (DC), (1998).
76. Smith AD, Kim Y-I, Refsum H. Is folic acid good for everyone? Am J Clin Nutr. (2008) 87:517–33. doi: 10.1093/ajcn/87.3.517
77. Rucker RB, Zempleni J, Suttie JW, McCormick DB. “Bioorganic mechanisms important to coenzyme functions.” In: Handbook of Vitamins. 16. CRC Press.
78. Stover JZ, Suttie JW, Gregory III JF, Patrick J. “Vitamin b6.” In: Handbook of Vitamins. 605. CRC Press (2013).
79. Carr AC, Maggini S. Vitamin C and immune function. Nutrients. (2017) 9:1211. doi: 10.3390/nu9111211
80. Granger M, Eck P. Dietary vitamin C in human health. Adv Food Nutr Res. (2018) 83:281–310. doi: 10.1016/bs.afnr.2017.11.006
81. Nardi J, Nascimento S, Göethel G, Gauer B, Sauer E, Fão N, et al. Inflammatory and oxidative stress parameters as potential early biomarkers for silicosis. Clin Chim Acta. (2018) 484:305–13. doi: 10.1016/j.cca.2018.05.045
82. Kaya V, Yazkan R, Yildirim M, Doguç DK, Süren D, Bozkurt KK, et al. The relation of radiation-induced pulmonary fibrosis with stress and the efficiency of antioxidant treatment: an experimental study. Med Sci Monit. (2014) 20:290–6. doi: 10.12659/MSM.890334
83. Hemmati AA, Nazari Z, Ranjbari N, Torfi A. Comparison of the preventive effect of vitamin C and E on hexavalent chromium induced pulmonary fibrosis in rat. Inflammopharmacology. (2008) 16:195–7. doi: 10.1007/s10787-008-7004-4
84. Somade OT, Adeyi OE, Ajayi BO, Asunde OO, Iloh PD, Adesanya AA, et al. Syringic and ascorbic acids prevent NDMA-induced pulmonary fibrogenesis, inflammation, apoptosis, and oxidative stress through the regulation of PI3K-Akt/PKB-mTOR-PTEN signaling pathway. Metabol Open. (2022) 14:100179. doi: 10.1016/j.metop.2022.100179
85. Carr A, Frei B. Does vitamin C act as a pro-oxidant under physiological conditions? FASEB J. (1999) 13:1007–24. doi: 10.1096/fasebj.13.9.1007
86. Amatore C, Arbault S, Ferreira DCM, Tapsoba I. Verchier Y. Vitamin C stimulates or attenuates reactive oxygen and nitrogen species (ROS, RNS) production depending on cell state: Quantitative amperometric measurements of oxidative bursts at PLB-985 and RAW 2647 cells at the single cell level. J Electroanal Chem. (2008) 615:34–44. doi: 10.1016/j.jelechem.2007.11.037
87. Molina N, Morandi AC, Bolin AP, Otton R. Comparative effect of fucoxanthin and vitamin C on oxidative and functional parameters of human lymphocytes. Int Immunopharmacol. (2014) 22:41–50. doi: 10.1016/j.intimp.2014.06.026
88. Halliwell B. Vitamin C: antioxidant or pro-oxidant in vivo? Free Radic Res. (1996) 25:439–54. doi: 10.3109/10715769609149066
89. Buettner GR. The pecking order of free radicals and antioxidants: lipid peroxidation, alpha-tocopherol, and ascorbate. Arch Biochem Biophys. (1993) 300:535–43. doi: 10.1006/abbi.1993.1074
90. Packer JE, Slater TF, Willson RL. Direct observation of a free radical interaction between vitamin E and vitamin C. Nature. (1979) 278:737–8. doi: 10.1038/278737a0
91. Frijhoff J, Winyard PG, Zarkovic N, Davies SS, Stocker R, Cheng D, et al. Clinical relevance of biomarkers of oxidative stress. Antioxid Redox Signal. (2015) 23:1144–70. doi: 10.1089/ars.2015.6317
92. Villagran M, Ferreira J, Martorell M, Mardones L. The role of vitamin C in cancer prevention and therapy: a literature review. Antioxidants. (2021) 10:1894. doi: 10.3390/antiox10121894
93. Munday K. Vitamin C and bone markers: investigations in a Gambian population. Proc Nutr Soc. (2003) 62:429–36. doi: 10.1079/PNS2003247
94. Ciofu O, Smith S, Lykkesfeldt J. Antioxidant supplementation for lung disease in cystic fibrosis. Cochrane Database Syst Rev. (2019) 10:CD007020. doi: 10.1002/14651858.CD007020.pub4
95. Liu B, Cao B, Zhang D, Xiao N, Chen H, Li GQ, et al. Salvianolic acid B protects against paraquat-induced pulmonary injury by mediating Nrf2/Nox4 redox balance and TGF-β1/Smad3 signaling Toxicol Appl Pharmacol. (2016) 309:111–20. doi: 10.1016/j.taap.2016.08.004
96. Cameron E, Pauling L, Leibovitz B. Ascorbic acid and cancer: a review. Cancer Res. (1979) 39:663–81.
97. Disayabutr S, Kim EK, Cha SI, Green G, Naikawadi RP, Jones KD, et al. miR-34 miRNAs regulate cellular senescence in type II alveolar epithelial cells of patients with idiopathic pulmonary fibrosis. PLoS ONE. (2016) 11:e0158367. doi: 10.1371/journal.pone.0158367
98. Hecker L, Logsdon NJ, Kurundkar D, Kurundkar A, Bernard K, Hock T, et al. Reversal of persistent fibrosis in aging by targeting Nox4-Nrf2 redox imbalance. Sci Transl Med. (2014) 6:231ra47. doi: 10.1126/scitranslmed.3008182
99. Citrin DE, Shankavaram U, Horton JA, Shield III W, Zhao S, Asano H, et al. Role of type II pneumocyte senescence in radiation-induced lung fibrosis. J Natl Cancer Inst. (2013) 105:1474–84. doi: 10.1093/jnci/djt212
100. Plataki M, Koutsopoulos AV, Darivianaki K, Delides G, Siafakas NM, Bouros D. Expression of apoptotic and antiapoptotic markers in epithelial cells in idiopathic pulmonary fibrosis. Chest. (2005) 127:266–74. doi: 10.1378/chest.127.1.266
101. Fine A, Janssen-Heininger Y, Soultanakis RP, Swisher SG, Uhal BD. Apoptosis in lung pathophysiology. Am J Physiol Lung Cell Mol Physiol. (2000) 279:L423–427. doi: 10.1152/ajplung.2000.279.3.L423
102. Kuwano K, Kunitake R, Maeyama T, Hagimoto N, Kawasaki M, Matsuba T, et al. Attenuation of bleomycin-induced pneumopathy in mice by a caspase inhibitor. Am J Physiol Lung Cell Mol Physiol. (2001) 280:L316–325. doi: 10.1152/ajplung.2001.280.2.L316
103. Lee CG, Cho SJ, Kang MJ, Chapoval SP, Lee PJ, Noble PW, et al. Early growth response gene 1-mediated apoptosis is essential for transforming growth factor beta1-induced pulmonary fibrosis. J Exp Med. (2004) 200:377–89. doi: 10.1084/jem.20040104
104. Kang H-R, Cho SJ, Lee CG, Homer RJ, Elias JA. Transforming growth factor (TGF)-beta1 stimulates pulmonary fibrosis and inflammation via a Bax-dependent, bid-activated pathway that involves matrix metalloproteinase-12. J Biol Chem. (2007) 282:7723–32. doi: 10.1074/jbc.M610764200
105. Fisher BJ, Kraskauskas D, Martin EJ, Farkas D, Wegelin JA, Brophy D, et al. Mechanisms of attenuation of abdominal sepsis induced acute lung injury by ascorbic acid. Am J Physiol Lung Cell Mol Physiol. (2012) 303:L20–32. doi: 10.1152/ajplung.00300.2011
106. Oudemans-van Straaten HM, Spoelstra-de Man AM, de Waard MC. Vitamin C revisited. Crit Care. (2014) 18:460. doi: 10.1186/s13054-014-0460-x
107. Ali MK, Kim RY, Brown AC, Donovan C, Vanka KS, Mayall JR, et al. Critical role for iron accumulation in the pathogenesis of fibrotic lung disease. J Pathol. (2020) 251:49–62. doi: 10.1002/path.5401
108. Ali MK, Kim RY, Karim R, Mayall JR, Martin KL, Shahandeh A, et al. Role of iron in the pathogenesis of respiratory disease. Int J Biochem Cell Biol. (2017) 88:181–95. doi: 10.1016/j.biocel.2017.05.003
109. Bargagli E, Refini RM, d'Alessandro M, Bergantini L, Cameli P, Vantaggiato L, et al. Metabolic dysregulation in idiopathic pulmonary fibrosis. Int J Mol Sci. (2020) 21:5663. doi: 10.3390/ijms21165663
110. Neves J, Leitz D, Kraut S, Brandenberger C, Agrawal R, Weissmann N, et al. Disruption of the hepcidin/ferroportin regulatory system causes pulmonary iron overload and restrictive lung disease. EBioMedicine. (2017) 20:230–9. doi: 10.1016/j.ebiom.2017.04.036
111. Toblli JE, Cao G, Giani JF, Dominici FP, Angerosa M. Markers of oxidative/nitrosative stress and inflammation in lung tissue of rats exposed to different intravenous iron compounds. Drug Des Devel Ther. (2017) 11:2251–63. doi: 10.2147/DDDT.S132612
112. Yang WS, Stockwell BR. Synthetic lethal screening identifies compounds activating iron-dependent, nonapoptotic cell death in oncogenic-RAS-harboring cancer cells. Chem Biol. (2008) 15:234–45. doi: 10.1016/j.chembiol.2008.02.010
113. Zilka O, Shah R, Li B, Friedmann Angeli JP, Griesser M, Conrad M, et al. On the mechanism of cytoprotection by ferrostatin-1 and liproxstatin-1 and the role of lipid peroxidation in ferroptotic cell death. ACS Cent Sci. (2017) 3:232–43. doi: 10.1021/acscentsci.7b00028
114. Lorincz T, Holczer M, Kapuy O, Szarka A. The interrelationship of pharmacologic ascorbate induced cell death and ferroptosis. Pathol Oncol Res. (2019) 25:669–79. doi: 10.1007/s12253-018-0539-9
115. Zhu K, Zhu X, Liu S, Yu J, Wu S, Hei M. Glycyrrhizin attenuates hypoxic-ischemic brain damage by inhibiting ferroptosis and neuroinflammation in neonatal rats via the HMGB1/GPX4 pathway. Oxid Med Cell Longev. (2022) 2022:8438528. doi: 10.1155/2022/8438528
116. Wang X, Wang Y, Huang D, Shi S, Pei C, Wu Y, et al. Astragaloside IV regulates the ferroptosis signaling pathway via the Nrf2/SLC7A11/GPX4 axis to inhibit PM25-mediated lung injury in mice. Int Immunopharmacol. (2022) 112:109186. doi: 10.1016/j.intimp.2022.109186
117. Mills CD. M1 and M2 macrophages: oracles of health and disease. Crit Rev Immunol. (2012) 32:463–88. doi: 10.1615/CritRevImmunol.v32.i6.10
118. Meyer NJ, Gattinoni L, Calfee CS. Acute respiratory distress syndrome. Lancet. (2021) 398:622–37. doi: 10.1016/S0140-6736(21)00439-6
119. Li X, Ma L, Wei Y, Gu J, Liang J, Li S, et al. Cabozantinib ameliorates lipopolysaccharide-induced lung inflammation and bleomycin–induced early pulmonary fibrosis in mice. Int Immunopharmacol. (2021) 101:108327. doi: 10.1016/j.intimp.2021.108327
120. Tian Z, Yao N, Wang F, Ruan L. Thymosin β4 suppresses LPS-induced murine lung fibrosis by attenuating oxidative injury and alleviating inflammation. Inflammation. (2022) 45:59–73. doi: 10.1007/s10753-021-01528-6
121. Kinoshita Y, Ishii H, Kushima H, Johkoh T, Yabuuchi H, Fujita M, et al. Remodeling of the pulmonary artery in idiopathic pleuroparenchymal fibroelastosis. Sci Rep. (2020) 10:306. doi: 10.1038/s41598-019-57248-3
122. Marinelli JP, Levin DL, Vassallo R, Carter RE, Hubmayr RD, Ehman RL, et al. Quantitative assessment of lung stiffness in patients with interstitial lung disease using MR elastography. J Magn Reson Imaging. (2017) 46:365–74. doi: 10.1002/jmri.25579
123. Yavari M, Mousavi SAJ, Janani L, Feizy Z, Vafa M. Effects of supplementation of vitamins D, C and E on idiopathic pulmonary fibrosis (IPF): a clinical trial. Clin Nutr ESPEN. (2022) 49:295–300. doi: 10.1016/j.clnesp.2022.03.035
124. Ginde AA, Mansbach JM, Camargo CA. Association between serum 25-hydroxyvitamin D level and upper respiratory tract infection in the third national health and nutrition examination survey. Arch Intern Med. (2009) 169:384–90. doi: 10.1001/archinternmed.2008.560
126. Rodrigues da. Silva M, Schapochnik A, Peres Leal M, Esteves J, Bichels Hebeda C, Sandri S, et al. Beneficial effects of ascorbic acid to treat lung fibrosis induced by paraquat. PLoS ONE. (2018) 13:e0205535. doi: 10.1371/journal.pone.0205535
127. Tan PH, Sagoo P, Chan C, Yates JB, Campbell J, Beutelspacher SC, et al. Inhibition of NF-kappa B and oxidative pathways in human dendritic cells by antioxidative vitamins generates regulatory T cells. J Immunol. (2005) 174:7633–44. doi: 10.4049/jimmunol.174.12.7633
128. Hosseinpour F, Wikvall K. Porcine microsomal vitamin D(3) 25-hydroxylase (CYP2D25). Catalytic properties, tissue distribution, and comparison with human CYP2D6. J Biol Chem. (2000) 275:34650–5. doi: 10.1074/jbc.M004185200
129. Zhu J, DeLuca HF. Vitamin D 25-hydroxylase - four decades of searching, are we there yet? Arch Biochem Biophys. (2012) 523:30–6. doi: 10.1016/j.abb.2012.01.013
130. Flanagan JN, Young MV, Persons KS, Wang L, Mathieu JS, Whitlatch LW, et al. Vitamin D metabolism in human prostate cells: implications for prostate cancer chemoprevention by vitamin D. Anticancer Res. (2006) 26:2567–72.
131. Haussler MR, Wikvall K. Porcine microsomal vitamin D3 25-hydroxylase (CYP2D25). Catalytic properties, tissue distribution, and comparison with human CYP2D6. J Bone Miner Res. (1998) 13:325–49.
132. Berridge MJ. Vitamin D, reactive oxygen species and calcium signalling in ageing and disease. Philos Trans R Soc Lond B Biol Sci. (2016) 371:20150434. doi: 10.1098/rstb.2015.0434
133. Shao D, Yao C, Kim MH, Fry J, Cohen RA, Costello CE, et al. Improved mass spectrometry-based activity assay reveals oxidative and metabolic stress as sirtuin-1 regulators. Redox Biol. (2019) 22:101150. doi: 10.1016/j.redox.2019.101150
134. Chen H, Hu X, Yang R, Wu G, Tan Q, Goltzman D, et al. SIRT1/FOXO3a axis plays an important role in the prevention of mandibular bone loss induced by 1, 25(OH)2D deficiency. Int J Biol Sci. (2020) 16:2712–26. doi: 10.7150/ijbs.48169
135. Domingues-Faria C, Chanet A, Salles J, Berry A, Giraudet C, Patrac V, et al. Vitamin D deficiency down-regulates Notch pathway contributing to skeletal muscle atrophy in old wistar rats. Nutr Metab. (2014) 11:47. doi: 10.1186/1743-7075-11-47
136. Jolliffe DA, Stefanidis C, Wang Z, Kermani NZ, Dimitrov V, White JH, et al. Vitamin D metabolism is dysregulated in asthma and chronic obstructive pulmonary disease. Am J Respir Crit Care Med. (2020) 202:371–82. doi: 10.1164/rccm.201909-1867OC
137. Zhou J, Chen H, Wang Q, Chen S, Wang R, Wang Z, et al. Sirt1 overexpression improves senescence-associated pulmonary fibrosis induced by vitamin D deficiency through downregulating IL-11 transcription. Aging Cell. (2022) 21:e13680. doi: 10.1111/acel.13680
138. Li S-R, Tan ZX, Chen YH, Hu B, Zhang C, Wang H, et al. Vitamin D deficiency exacerbates bleomycin-induced pulmonary fibrosis partially through aggravating TGF-β/Smad2/3-mediated epithelial-mesenchymal transition. Respir Res. (2019) 20:266. doi: 10.1186/s12931-019-1232-6
139. Yang L, Zhai Z, Zhang J. The role of serum 1,25-dihydroxy vitamin D3 and PCT in idiopathic pulmonary fibrosis. Int J Gen Med. (2022) 15:8081–92. doi: 10.2147/IJGM.S386984
140. Faverio P, Fumagalli A, Conti S, Madotto F, Bini F, Harari S, et al. Nutritional assessment in idiopathic pulmonary fibrosis: a prospective multicentre study. ERJ Open Res. (2022) 8:00443–2021. doi: 10.1183/23120541.00443-2021
141. Park YS, Park CM, Lee HJ, Goo JM, Chung DH, Lee SM, et al. Clinical implication of protease-activated receptor-2 in idiopathic pulmonary fibrosis. Respir Med. (2013) 107:256–62. doi: 10.1016/j.rmed.2012.10.011
142. Imokawa S, Sato A, Hayakawa H, Kotani M, Urano T, Takada A. Tissue factor expression and fibrin deposition in the lungs of patients with idiopathic pulmonary fibrosis and systemic sclerosis. Am J Respir Crit Care Med. (1997) 156:631–6. doi: 10.1164/ajrccm.156.2.9608094
143. Schaffner F, Ruf W. Tissue factor and protease-activated receptor signaling in cancer. Semin Thromb Hemost. (2008) 34:147–53. doi: 10.1055/s-2008-1079254
144. Subramaniam S, Kanse SM, Kothari H, Reinhardt C, Fletcher C. Post-transcriptional, post-translational and pharmacological regulation of tissue factor pathway inhibitor. Blood Coagul Fibrinolysis. (2018) 29:668–82. doi: 10.1097/MBC.0000000000000775
145. Martinez-Moreno JM, Herencia C, Oca AM, Muñoz-Castañeda JR, Rodríguez-Ortiz ME, Díaz-Tocados JM, et al. Vitamin D modulates tissue factor and protease-activated receptor 2 expression in vascular smooth muscle cells. FASEB J. (2016) 30:1367–76. doi: 10.1096/fj.15-272872
146. Kasthuri RS, Glover SL, Boles J, Mackman N. Tissue factor and tissue factor pathway inhibitor as key regulators of global hemostasis: measurement of their levels in coagulation assays. Semin Thromb Hemost. (2010) 36:764–71. doi: 10.1055/s-0030-1265293
147. Ohsawa M, Koyama T, Yamamoto K, Hirosawa S, Kamei S, Kamiyama R. 1alpha,25-dihydroxyvitamin D(3) and its potent synthetic analogs downregulate tissue factor and upregulate thrombomodulin expression in monocytic cells, counteracting the effects of tumor necrosis factor and oxidized LDL. Circulation. (2000) 102:2867–72. doi: 10.1161/01.CIR.102.23.2867
148. Liu Y-M, Nepali K, Liou J-P. Idiopathic pulmonary fibrosis: current status, recent progress, and emerging targets. J Med Chem. (2017) 60:527–53. doi: 10.1021/acs.jmedchem.6b00935
149. Noble PW. Idiopathic pulmonary fibrosis. New insights into classification and pathogenesis usher in a new era therapeutic approaches. Am J Respir Cell Mol Biol. (2003) 29:S27–31.
150. Selman M, Pardo A. The epithelial/fibroblastic pathway in the pathogenesis of idiopathic pulmonary fibrosis. Am J Respir Cell Mol Biol. (2003) 29:S93–97.
151. Brown SD, Calvert HH, Fitzpatrick AM. Vitamin D and asthma. Dermatoendocrinol. (2012) 4:137–45. doi: 10.4161/derm.20434
152. Paul G, Brehm JM, Alcorn JF, Holguín F, Aujla SJ, Celedón JC. Vitamin D and asthma. Am J Respir Crit Care Med. (2012) 185:124–32. doi: 10.1164/rccm.201108-1502CI
153. Stubbs JR, Idiculla A, Slusser J, Menard R, Quarles LD. Cholecalciferol supplementation alters calcitriol-responsive monocyte proteins and decreases inflammatory cytokines in ESRD. J Am Soc Nephrol. (2010) 21:353–61. doi: 10.1681/ASN.2009040451
154. Finklea JD, Grossmann RE, Tangpricha V. Vitamin D and chronic lung disease: a review of molecular mechanisms and clinical studies. Adv Nutr. (2011) 2:244–53. doi: 10.3945/an.111.000398
155. Herr C, Greulich T, Koczulla RA, Meyer S, Zakharkina T, Branscheidt M, et al. The role of vitamin D in pulmonary disease: COPD, asthma, infection, and cancer. Respir Res. (2011) 12:31. doi: 10.1186/1465-9921-12-31
156. Bonventre JV. Antifibrotic vitamin D analogs. J Clin Invest. (2013) 123:4570–3. doi: 10.1172/JCI72748
157. Huang LS, Natarajan V. Sphingolipids in pulmonary fibrosis. Adv Biol Regul. (2015) 57:55–63. doi: 10.1016/j.jbior.2014.09.008
158. Fischer KD, Agrawal DK. Vitamin D regulating TGF-β induced epithelial-mesenchymal transition. Respir Res. (2014) 15:146. doi: 10.1186/s12931-014-0146-6
159. Mehdipoor M, Damirchi A, Razavi Tousi SMT, Babaei P. Concurrent vitamin D supplementation and exercise training improve cardiac fibrosis via TGF-β/Smad signaling in myocardial infarction model of rats. J Physiol Biochem. (2021) 77:75–84. doi: 10.1007/s13105-020-00778-6
160. Wang L, Yuan T, Du G, Zhao Q, Ma L, Zhu J. The impact of 1, 25–dihydroxyvitamin D3 on the expression of connective tissue growth factor and transforming growth factor–β1 in the myocardium of rats with diabetes. Diabetes Res Clin Pract. (2014) 104:226–33. doi: 10.1016/j.diabres.2014.01.031
161. Ito I, Waku T, Aoki M, Abe R, Nagai Y, Watanabe T, et al. A nonclassical vitamin D receptor pathway suppresses renal fibrosis. J Clin Invest. (2013) 123:4579–94. doi: 10.1172/JCI67804
162. Jiang F, Yang Y, Xue L, Li B, Zhang Z. 1α,25-dihydroxyvitamin D3 attenuates TGF-β-induced pro-fibrotic effects in human lung epithelial cells through inhibition of epithelial-mesenchymal transition. Nutrients. (2017) 9:980. doi: 10.3390/nu9090980
163. Potter JJ, Liu X, Koteish A, Mezey E. 1,25-dihydroxyvitamin D3 and its nuclear receptor repress human α1 (I) collagen expression and type I collagen formation. Liver Int. (2013) 33:677–86. doi: 10.1111/liv.12122
164. Lichtler A, Stover ML, Angilly J, Kream B, Rowe DW. Isolation and characterization of the rat alpha 1(I) collagen promoter. Regulation by 1,25-dihydroxyvitamin D. J Biol Chem. (1989) 264:3072–7. doi: 10.1016/S0021-9258(18)94032-9
165. Sundar IK, Hwang J-W, Wu S, Sun J, Rahman I. Deletion of vitamin D receptor leads to premature emphysema/COPD by increased matrix metalloproteinases and lymphoid aggregates formation. Biochem Biophys Res Commun. (2011) 406:127–33. doi: 10.1016/j.bbrc.2011.02.011
166. Gao Y, Zhao Q, Qiu X, Zhuang Y, Yu M, Dai J, et al. Vitamin D levels are prognostic factors for connective tissue disease associated interstitial lung disease (CTD-ILD). Aging. (2020) 12:4371–8. doi: 10.18632/aging.102890
167. Ramirez AM, Wongtrakool C, Welch T, Steinmeyer A, Zügel U, Roman J. Vitamin D inhibition of pro-fibrotic effects of transforming growth factor beta1 in lung fibroblasts and epithelial cells. J Steroid Biochem Mol Biol. (2010) 118:142–50. doi: 10.1016/j.jsbmb.2009.11.004
168. Jeon YK, Shin MJ, Kim MH, Mok JH, Kim SS, Kim BH, et al. Low pulmonary function is related with a high risk of sarcopenia in community-dwelling older adults: the Korea National Health and Nutrition Examination Survey (KNHANES) 2008–2011. Osteoporos Int. (2015) 26:2423–9. doi: 10.1007/s00198-015-3152-8
169. Moon SW, Choi JS, Lee SH, Jung KS, Jung JY, Kang YA, et al. Thoracic skeletal muscle quantification: low muscle mass is related with worse prognosis in idiopathic pulmonary fibrosis patients. Respir Res. (2019) 20:35. doi: 10.1186/s12931-019-1001-6
170. Carson EL, Pourshahidi LK, Madigan SM, Baldrick FR, Kelly MG, Laird E, et al. Vitamin D status is associated with muscle strength and quality of life in patients with COPD: a seasonal prospective observation study. Int J Chron Obstruct Pulmon Dis. (2018) 13:2613–22. doi: 10.2147/COPD.S166919
171. Tzilas V, Bouros E, Barbayianni I, Karampitsakos T, Kourtidou S, Ntassiou M, et al. Vitamin D prevents experimental lung fibrosis and predicts survival in patients with idiopathic pulmonary fibrosis. Pulm Pharmacol Ther. (2019) 55:17–24. doi: 10.1016/j.pupt.2019.01.003
172. Institute of Medicine (US) Committee to Review Dietary Reference Intakes for Vitamin D and Calcium. Dietary Reference Intakes for Calcium and Vitamin D. National Academies Press (US), Washington (DC), (2011).
173. Planté-Bordeneuve T, Berardis S, Bastin P, Gruson D, Henri L, Gohy S. Vitamin D intoxication in patients with cystic fibrosis: report of a single-center cohort. Sci Rep. (2021) 11:7719. doi: 10.1038/s41598-021-87099-w
174. Abdala-Valencia H, Berdnikovs S, Cook-Mills JM. Vitamin E isoforms as modulators of lung inflammation. Nutrients. (2013) 5:4347–63. doi: 10.3390/nu5114347
175. Card JW, Leeder RG, Racz WJ, Brien JF, Bray TM, Massey TE. Effects of dietary vitamin E supplementation on pulmonary morphology and collagen deposition in amiodarone- and vehicle-treated hamsters. Toxicology. (1999) 133:75–84. doi: 10.1016/S0300-483X(99)00009-8
176. Futamura Y. Toxicity of amiodarone on mouse pulmonary endothelial cells cultured with or without alveolar macrophages. J Toxicol Sci. (1996) 21:253–67. doi: 10.2131/jts.21.4_253
177. Chang J, Wang J, Luo B, Li W, Xiong Z, Du C, et al. Vitamin E stabilizes iron and mitochondrial metabolism in pulmonary fibrosis. Front Pharmacol. (2023) 14:1240829. doi: 10.3389/fphar.2023.1240829
178. Card JW, Racz WJ, Brien JF, Massey TE. Attenuation of amiodarone-induced pulmonary fibrosis by vitamin E is associated with suppression of transforming growth factor-beta1 gene expression but not prevention of mitochondrial dysfunction. J Pharmacol Exp Ther. (2003) 304:277–83. doi: 10.1124/jpet.102.043208
179. Hakim F, Kerem E, Rivlin J, Bentur L, Stankiewicz H, Bdolach-Abram T, et al. Vitamins A and E and pulmonary exacerbations in patients with cystic fibrosis. J Pediatr Gastroenterol Nutr. (2007) 45:347–53. doi: 10.1097/MPG.0b013e31804069e5
180. Lagrange-Puget M, Durieu I, Ecochard R, Abbas-Chorfa F, Drai J, Steghens JP, et al. Longitudinal study of oxidative status in 312 cystic fibrosis patients in stable state and during bronchial exacerbation. Pediatr Pulmonol. (2004) 38:43–9. doi: 10.1002/ppul.20041
181. Range SP, Dunster C, Knox AJ, Kelly FJ. Treatment of pulmonary exacerbations of cystic fibrosis leads to improved antioxidant status. Eur Respir J. (1999) 13:560–4. doi: 10.1183/09031936.99.13356099
182. Shvedova AA, Kisin ER, Murray AR, Gorelik O, Arepalli S, Castranova V, et al. Vitamin E deficiency enhances pulmonary inflammatory response and oxidative stress induced by single-walled carbon nanotubes in C57BL/6 mice. Toxicol Appl Pharmacol. (2007) 221:339–48. doi: 10.1016/j.taap.2007.03.018
183. Woestenenk JW, Broos N, Stellato RK, Arets HG, Van Der Ent CK, Houwen RH. Vitamin E intake, α-tocopherol levels and pulmonary function in children and adolescents with cystic fibrosis. Br J Nutr. (2015) 113:1096–101. doi: 10.1017/S0007114515000215
184. Miller ER, Pastor-Barriuso R, Dalal D, Riemersma RA, Appel LJ, Guallar E. Meta-analysis: high-dosage vitamin E supplementation may increase all-cause mortality. Ann Intern Med. (2005) 142:37–46. doi: 10.7326/0003-4819-142-1-200501040-00110
185. Sesso HD, Buring JE, Christen WG, Kurth T, Belanger C, MacFadyen J, et al. Vitamins E and C in the prevention of cardiovascular disease in men: the Physicians' Health Study II randomized controlled trial. JAMA. (2008) 300:2123–33. doi: 10.1001/jama.2008.600
186. Spagnolo P, Wuyts W. Acute exacerbations of interstitial lung disease: lessons from idiopathic pulmonary fibrosis. Curr Opin Pulm Med. (2017) 23:411–7. doi: 10.1097/MCP.0000000000000405
187. Oda K, Ishimoto H, Yamada S, Kushima H, Ishii H, Imanaga T, et al. Autopsy analyses in acute exacerbation of idiopathic pulmonary fibrosis. Respir Res. (2014) 15:109. doi: 10.1186/s12931-014-0109-y
188. Drent M, Wessels S, Jacobs JA, Thijssen H. Association of diffuse alveolar haemorrhage with acquired vitamin K deficiency. Respiration. (2000) 67:697. doi: 10.1159/000056305
189. Rana M, Wong-See D, Katz T, Gaskin K, Whitehead B, Jaffe A, et al. Fat-soluble vitamin deficiency in children and adolescents with cystic fibrosis. J Clin Pathol. (2014) 67:605–8. doi: 10.1136/jclinpath-2013-201787
190. Drent M, Wijnen P, Bast A. Pharmacogenetic variants and vitamin K deficiency: a risk factor or trigger for fibrosing interstitial pneumonias? Curr Opin Pulm Med. (2018) 24:287–95. doi: 10.1097/MCP.0000000000000467
191. Janssen R. Can bisphosphonates prevent vitamin K antagonist toxicity in patients with idiopathic pulmonary fibrosis? Am J Respir Crit Care Med. (2017) 195:269–70. doi: 10.1164/rccm.201605-1074LE
192. Piscaer I, Wouters EF, Vermeer C, Janssens W, Franssen FM, Janssen R. Vitamin K deficiency: the linking pin between COPD and cardiovascular diseases? Respir Res. (2017) 18:189. doi: 10.1186/s12931-017-0673-z
193. Schurgers LJ, Uitto J, Reutelingsperger CP. Vitamin K-dependent carboxylation of matrix Gla-protein: a crucial switch to control ectopic mineralization. Trends Mol Med. (2013) 19:217–26. doi: 10.1016/j.molmed.2012.12.008
194. Lemke G. Biology of the TAM receptors. Cold Spring Harb Perspect Biol. (2013) 5:a009076. doi: 10.1101/cshperspect.a009076
195. Urawa M, Kobayashi T, D'Alessandro-Gabazza CN, Fujimoto H, Toda M, Roeen Z, et al. Protein S is protective in pulmonary fibrosis. J Thromb Haemost. (2016) 14:1588–99. doi: 10.1111/jth.13362
Keywords: pulmonary fibrosis, vitamin, dietary nutrition, EMT, vitamin deficiency
Citation: Qu Y and Zhao Y (2025) Nutritional insights into pulmonary fibrosis: a comprehensive review on the impact of vitamins. Front. Nutr. 12:1525408. doi: 10.3389/fnut.2025.1525408
Received: 11 November 2024; Accepted: 25 March 2025;
Published: 11 April 2025.
Edited by:
Xiaopeng Li, Michigan State University, United StatesReviewed by:
Hee Joon Yoo, Duke University, United StatesRicardo Adrian Nugraha, Faculty of Medicine Universitas Airlangga - Dr. Soetomo General Hospital, Indonesia
Pengxiu Cao, Hebei Normal University, China
Copyright © 2025 Qu and Zhao. This is an open-access article distributed under the terms of the Creative Commons Attribution License (CC BY). The use, distribution or reproduction in other forums is permitted, provided the original author(s) and the copyright owner(s) are credited and that the original publication in this journal is cited, in accordance with accepted academic practice. No use, distribution or reproduction is permitted which does not comply with these terms.
*Correspondence: Yaqian Qu, cXV5YXFpYW5AaGFjdGNtLmVkdS5jbg==