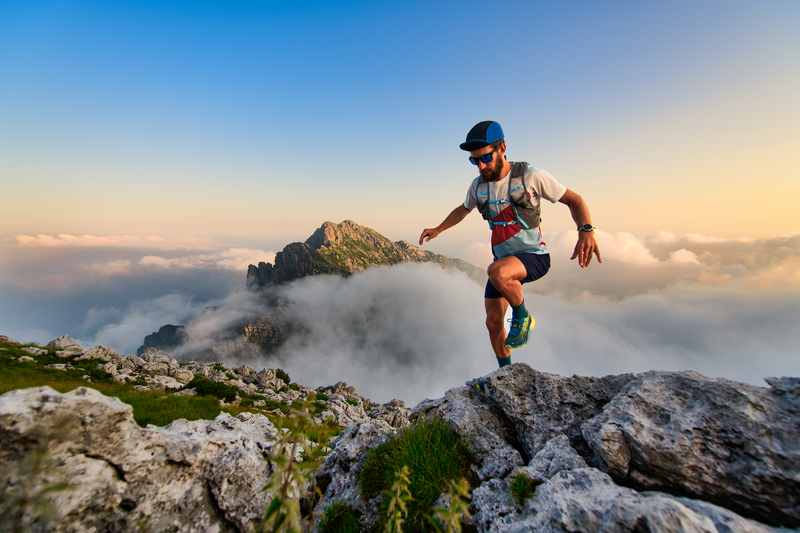
95% of researchers rate our articles as excellent or good
Learn more about the work of our research integrity team to safeguard the quality of each article we publish.
Find out more
PERSPECTIVE article
Front. Nutr. , 17 January 2025
Sec. Nutrition and Metabolism
Volume 12 - 2025 | https://doi.org/10.3389/fnut.2025.1524125
Intermittent fasting has been linked to metabolic health by improving lipid profiles, reducing body weight, and increasing insulin sensitivity. However, several randomized clinical trials have shown that intermittent fasting is not more effective than standard daily caloric restriction for short-term weight loss or cardiometabolic improvements in patients with obesity. Observational studies also suggest cardiovascular benefits from extended rather than reduced eating windows, and indicate that long-term intermittent fasting regimens may increase the risk of cardiovascular disease mortality. In this perspective, we discuss evidence that may support potential adverse effects of intermittent fasting on cardiovascular health through the loss of lean mass, circadian misalignment and poor dietary choices associated with reward-based eating. Given the ongoing revolution in obesity pharmacotherapy, we argue that future research should integrate anti-obesity medications with dietary strategies that confer robust benefits to cardiometabolic health, combine exercise regimens, and consider genetic factors to personalize obesity treatment. Comprehensive approaches combining diet, pharmacotherapy, and lifestyle modifications will become crucial for managing obesity and minimizing long-term cardiovascular risk.
Intermittent fasting (IF) has gained attention as an effective strategy for reducing body weight and improving metabolic health. IF includes various protocols, such as time-restricted eating (TRE), alternate-day fasting (ADF), and the 5:2 diet (1). TRE confines food intake to a specific window each day, typically less than 12 h, and involves fasting for the remaining hours. ADF alternates between days of normal eating and days with significant calorie restriction or complete fasting. The 5:2 diet involves eating ad libitum for 5 days and restricting caloric intake for two nonconsecutive days each week. Several lines of clinical, epidemiological and experimental evidence have suggested that short-term application of IF protocols may improve risk factors associated with cardiovascular disease (2–5). These benefits have been attributed to weight loss, improved lipid profiles, reduced oxidative stress and systolic blood pressure that have been extensively reviewed elsewhere (3, 6, 7).
However, several randomized clinical trials (RCTs) (8–11) and a recent meta-analysis of RCTs (12) have provided robust evidence that neither TRE nor ADF or 5:2 IF is more effective than standard daily caloric restriction in terms of short-term reductions in body weight or improvements in cardiometabolic risk factors in patients with obesity. Likewise, a 3-week ADF regimen in lean adults was reported to be less effective at reducing body fat and improving cardiometabolic health parameters than an equivalent daily energy restriction (13). These observations suggest that many of the short term health-promoting effects of IF are most likely mediated by caloric restriction. Indeed, an 8-week ADF intervention study in overweight patients failed to improve cardiometabolic risk factors in the absence of caloric restriction (14).
In this perspective, we critically evaluate the effects of IF on cardiovascular health, focusing on potential adverse outcomes that may be associated with loss of lean mass, circadian misalignment, and dietary patterns driven by reward-based eating. These links are examined also in the context of the transformative advances in obesity pharmacotherapy, by discussing how pharmacological agents could be combined with dietary interventions for the management of cardiometabolic risk. By synthesizing current evidence, we aim to provide a comprehensive understanding of the role of IF in cardiovascular health and stimulate discussions about optimized personalized dietary strategies for obesity management in the era of obesity pharmacotherapy.
The scarcity of observational studies addressing the effects of long-term application of IF on cardiovascular health has been recognized as a major gap in nutrition research (1). Intriguingly, a recently published observational analysis of data from the National Health and Nutrition Examination Survey (NHANES) revealed that an eating window extending beyond 11 h is associated with lower cardiovascular disease (CVD) mortality in adults with heart failure (15). Along the same lines, an independent preliminary analysis of data from the NHANES presented at the 2024 American Heart Association conference by Zhong et al. (16) suggested that adhering to an 8-h TRE schedule is associated with a 91% increased risk of CVD mortality among a cohort of over 20,000 adults. The mortality risk was found to be particularly pronounced in individuals with preexisting heart conditions (16). Despite potential limitations, such as confounding factors and reliance on self-reported data which may introduce errors, these studies raise concerns about the long-term impact of IF on cardiovascular health.
Experimental findings in rodents align with the conclusions of these observational studies. Thus, rats subjected to ADF for a prolonged period of time (i.e., 6 months, equivalent to approximately 14 years in humans) developed reduced left ventricular diastolic compliance, a 3-fold increase in interstitial myocardial fibrosis and diminished cardiac reserve (17). Together, these findings underscore the need to better appreciate the behavioral and biological processes linking IF to cardiovascular health and disease.
We suggest that increased loss of lean mass, circadian misalignment of food consumption and/or compromised food quality may underpin the adverse effects of IF on cardiovascular health in genetically predisposed individuals (Figure 1).
Figure 1. Some people may experience adverse cardiovascular effects following long-term application of intermittent fasting (bottom right) because of higher loss of lean mass, circadian misalignment of food consumption and/or compromised food quality linked to reward eating. These factors, separately or in combination, may particularly impact individuals with preexisting heart conditions or with genetic susceptibility to CVD leading to higher risk of mortality (15, 16).
An RCT in metabolically healthy patients with obesity reported that the limited eating window of IF may lead to increased food consumption on fast days (10). This behavior, termed “reward-based eating,” may degrade the overall diet quality by including more satisfying rather than nutritionally balanced options (18). Indeed, some studies have reported reduced cognitive restraint, reduced fiber intake and greater sugar and meat consumption in individuals on IF regimes than in those on continuous caloric restriction (19–22). We speculate that at least some of the instances of compromised food quality could be attributed to reward eating. Poor food choices may also explain the elevated levels of LDL-cholesterol, a risk factor for CVD, reported in obesity patients undergoing a 12-month ADF but not in patients receiving a continuous hypocaloric diet (10).
Protein consumption tends to also increase among individuals practicing IF (23–25), potentially to increase satiety. While plant-based protein sources are known to benefit cardiovascular health, increased intake of animal protein has been linked to CVD and all-cause mortality (26, 27). Exaggerated consumption of animal-based foods such as red meat, eggs, and dairy is expected to increase choline and carnitine intake, leading to the production of trimethylamine-N-oxide (TMAO) through a metabolic pathway involving the gut microbiota and the liver. Elevated levels of circulating TMAO are associated with a higher incidence of atherosclerotic CVD and major adverse cardiovascular events (28, 29). Although a definitive link between prolonged IF and TMAO has not been established, elevated TMAO levels have been reported in patients with obesity undergoing 5:2 IF for 4 weeks (30).
Loss of lean mass is another factor that may link IF to adverse cardiovascular outcomes. An RCT in patients with overweight or obesity found that a 12-week hypocaloric TRE regimen led to a greater loss of lean mass compared to a conventional hypocaloric diet (9). The reduction in lean mass accounted for approximately 65% of total weight loss (9) which is notably higher than the typical 20–30% range observed with standard hypocaloric diets (31), and primarily involved loss of skeletal muscle mass. Reduced muscle mass has been associated with an increased risk of CVD (32, 33), as well as cardiovascular events and mortality (34). These findings highlight the need for caution when considering IF for individuals at risk of sarcopenia, such as the elderly and cancer patients.
Circadian misalignment in food intake may also impact metabolic and cardiac health (35), suggesting additional putative links between IF and CVD. Several observational and interventional studies have shown that eating breakfast is overall associated with cardiometabolic health (36), whereas skipping breakfast increases the risk of CVD (37, 38) and type 2 diabetes (T2D) (39). The behavior of eating breakfast is highly heritable, with twin studies indicating a 56% heritability rate (40). In a Mendelian randomization analysis of data from 200,000 individuals, genetically predisposed breakfast skipping was causally linked to obesity (41). Circadian misalignment caused by irregular eating patterns, such as skipping breakfast during IF, may exacerbate metabolic dysfunction in genetically predisposed individuals. For example, the polymorphism 3111T/C in circadian-related gene CLOCK has been associated with differences in glucose tolerance and lipid metabolism (42), both of which are critical to cardiovascular risk. These findings highlight the potential risks of skipping breakfast, particularly for those with a genetic predisposition, and underscores the importance of considering genetic factors when assessing the health impacts of IF and related dietary practices.
Similar to breakfast skipping, late-night eating has been linked to arterial stiffness (43) and to a greater risk of CHD in a 16-year cohort study (38). A recent observational analysis from the NutriNet-Santé study, which included over 103,000 adults, found that for each hour breakfast was delayed after 8:00 a.m. or the last meal was consumed after 8:00 p.m., the overall risk of CVD increased, regardless of body weight (44). This study also revealed a potential protective association between a longer night-time fasting duration and cardiovascular health, only when it was coupled with early first and last meals but not with skipping breakfast (44). Different TRE protocols have assigned varying eating time windows (e.g., early 8 am–2 pm or 12 pm–8 pm), and the multitude of differences in study designs and participant selection render them largely incomparable (45). In the real world, unsupervised individuals’ self-selection of the eating time window is the standard practice. Since the health impacts of different durations of exposure to IF diets can be important, future studies need to investigate this parameter extensively.
Chrononutrition is an important modulator of peripheral clocks in the circadian system controlling blood pressure rhythms (46), partly through the hypothalamus–pituitary–adrenal (HPA) axis. Cortisol is the main hormone produced by HPA. A small-scale intervention study indicated that while morning cortisol is reduced in females who skip breakfast as part of their intermittent diet regimen, postprandial cortisol levels later in the day are higher than those in females who do not skip breakfast (47). The long-term impacts of such shifts in diurnal cortisol remain undefined, but elevated cortisol levels are linked to hypertension and glucose intolerance, which are known risk factors for CVD (48). Indeed, cortisol activates the rennin/angiotensin/aldosterone system (RAAS), a complex hormonal network influencing blood pressure, fluid and electrolyte balance, and systemic vascular resistance. The activation of the RAAS, particularly through angiotensin II, can influence myocardial energy metabolism. For individuals with heart failure, especially those with compromised left ventricular function, the vasoconstrictive effect of angiotensin II may be detrimental by increasing blood pressure, impairing cardiac output and exacerbating heart failure symptoms (49). Therefore, the effects of skipping different meals in the framework of TRE (e.g., dinner versus breakfast) require further investigations through controlled studies to elucidate how meal timing impacts HPA axis rhythmicity and cardiovascular risk factors, including fluctuations in blood pressure and glucose levels. Notably, the application of an early (8 a.m. to 5 p.m.) TRE schedule for 1 week lowers fasting glucose, whereas late feeding (12 p.m. to 9 p.m.) has no effect (50).
One of the well-established effects of cortisol is the reduction of brain-derived neurotrophic factor (BDNF), which physiologically functions to inhibit appetite and food intake (51). Compared to continuous caloric restriction, cognitive restraint is reduced in individuals following IF (21) but the effects of intermittent diets on basal BDNF levels remain inconclusive (52). Importantly, larger cortisol-to-BDNF ratios have been associated with cardiometabolic risk and silent ischemia in African Americans (53, 54). BDNF is highly polymorphic, and the most common genetic variation, rs6265 (Val66Met), has been associated with greater severity of coronary artery disease (55). Experimental studies in homozygous Val66Met knock-in mice have confirmed this association and demonstrated that alterations in basal cardiac gene expression profiles are associated with the development of diastolic dysfunction (56). It would be interesting to examine whether carriers of BDNF rs6265 in NHANES who have incorporated TRE into their lifestyle are at higher risk of death from CVD compared with noncarriers (15, 16).
The aforementioned mechanisms underscore the importance of cautious implementation of IF strategies because of their potential links with cardiovascular risk. In a clinical setting, the loss of lean mass associated with some IF protocols, could exacerbate sarcopenia in older adults or individuals with chronic conditions and compromise cardiovascular resilience. Similarly, deregulated chrononutrition caused by irregular eating patterns, including genetically-predisposed breakfast skipping or late-night eating, should be taken into consideration, as it is strongly associated with metabolic dysfunction, elevated blood pressure, and increased cardiovascular risk. Reward-based eating should also be monitored among individuals practicing IF, as it may lead to poor dietary patterns that contribute to atherosclerosis and adverse cardiometabolic outcomes.
To address these concerns, future research should prioritize large-scale, longitudinal studies evaluating the long-term cardiovascular impacts of IF, particularly in vulnerable populations such as the elderly or those with preexisting cardiovascular conditions. Further exploration into the comparative effects of different IF protocols, such as TRE and ADF, on eating behavior, metabolic health, and circadian alignment is essential to delineate the conditions under which IF may pose risks or confer benefits.
From a therapeutic perspective, the controversy surrounding the health outcomes of IF should also be considered in the context of the evolving development of novel anti-obesity and anti-diabetic medications. These drugs include (but are not limited to) the glucagon-like peptide-1 (GLP-1) receptor agonists liraglutide and semaglutide; the dual GLP-1 and GIP (glucose-dependent insulinotropic polypeptide) receptor agonist tirzepatide; and the tri-agonist retatrutide, which activates GLP-1, GIP and glucagon receptors. Their remarkable efficacy in reducing both body weight and obesity-related comorbidities, including CVD and overall mortality (57–60), places IF-based interventions in a new context.
Indeed, pharmacotherapy with GLP-1 receptor or dual GIP/GLP-1 receptor agonists in patients with obesity achieves impressive reductions in body weight in the range of 15–25% compared with TRE or other nutritional interventions, which are typically in the range of 3–4% (1, 61). For example, in a 72-week clinical trial (61), a weekly dose of 15 mg of tirzepatide led to 21% total body weight loss compared with 3% in the placebo group receiving only a hypocaloric diet. Obesity pharmacotherapy reduces food intake and enhances satiety without the need to alter meal timing (62, 63), thus avoiding the potential health risks and reward-based eating behaviors reportedly associated with IF (44, 45). Several studies have also demonstrated that, unlike TRE which decreases lean mass, treatment with tirzepatide, liraglutide or semaglutide reduces body weight with small effects on lean mass (61, 64, 65). Therefore, the available evidence suggests that obesity pharmacotherapy may mitigate some of the behavioral and biological processes observed with IF that could elevate CVD risk.
Indeed, although long-term monitoring of the adverse effects of anti-obesity medications is still needed, data from clinical trials supports favorable efficacy versus safety profiles and demonstrates significant improvements in several cardiometabolic health parameters (57–61). Overweight or obesity patients without T2D but with preexisting CVD experience a marked decrease in the incidence of cardiovascular death, nonfatal myocardial infarction, or nonfatal stroke while on semaglutide treatment (66, 67). Moreover, obesity pharmacotherapy significantly reduces cardiovascular events in patients with type 2 diabetes (T2D) (68, 69).
A mixed strategy incorporating both pharmacotherapy and dietary interventions may offer the most comprehensive and pragmatic approach to obesity and cardiometabolic risk management. Along these lines, the SURMOUNT-3 phase III clinical trial revealed that patients with obesity or overweight who achieved ≥5.0% weight reduction with intensive lifestyle intervention prior to treatment with tirzepatide benefited the most from pharmacotherapy (70). Optimizing long-term dietary interventions will also be required to support and maintain the metabolic and cardiovascular benefits achieved during treatment. Incorporating genetics into this optimization process could provide a more precise and personalized approach, ensuring maximum effectiveness and sustainability of critically required lifestyle changes (71–73).
The prevailing view that IF is cardioprotective largely stems from experimental rodent models and intervention studies (2–4). However, it is important to note that rodents have a significantly greater basal metabolic rate; thus, the reported beneficial metabolic effects of time-restricted eating on blood pressure and cardiac structure in mice and rats (4) may not fully translate to humans. Intermittent fasting studies performed in animals also lack the behavioral aspects of food choice and intake that typify humans, including reward-based eating. Moreover, it is widely recognized that TRE intervention studies in patients with obesity have focused primarily on short-term effects (i.e., durations of less than 12 weeks) and have small sample sizes (1), leaving a gap in our understanding of the safety and effectiveness of long-term intermittent caloric restriction. The observational analyses conducted by Zhong et al. (16) and Billingsley et al. (15) underscore the need for further investigations into the long-term impacts of intermittent diets on cardiovascular health in additional large-scale cohorts.
A major goal for future research will be to identify dietary interventions that both optimally support patients with obesity during pharmacotherapy and maintain the metabolic and cardiovascular benefits achieved during treatment. Given that the health effects and long-term consequences of IF remain unclear (1, 16), we propose that hypocaloric Mediterranean diets may serve as the preferred starting point. Mediterranean diets have been strongly associated with both body weight control and improved long-term cardiovascular outcomes, including clinically meaningful reductions in coronary heart disease, ischemic stroke, and overall CVD mortality (74, 75). Among several popular diets evaluated in a recent meta-analysis (76), the Mediterranean diet had the most consistent and robust evidence of a beneficial effect on both anthropometric parameters and cardiometabolic risk factors.
In addition to Mediterranean dietary interventions, the integration of exercise and behavioral modifications in new pharmacotherapy protocols needs to be considered for their potential to enhance both the achievement and maintenance of reduced body weight. Increased physical activity in combination with reduced caloric intake enables additional weight loss, better glycemic control, and improved insulin sensitivity and lipid profiles (77, 78). Emerging evidence suggests that adherence to physical activity recommendations while on liraglutide leads to a significant, albeit limited, reduction in body weight (79). Future clinical trials should thus aim to identify the optimal combination of diet, physical activity protocols, and behavioral modification techniques to be combined with new pharmacotherapies, as this combination could have a multiplier beneficial effect on the management of obesity.
In conclusion, we herein underscore the need for caution in applying intermittent fasting as a long-term dietary strategy for cardiovascular health. While short-term benefits of IF, such as weight loss and improvements in lipid profiles, have been demonstrated, these effects appear primarily mediated by caloric restriction rather than unique attributes of intermittent fasting. Importantly, the long-term impacts of IF remain largely unexplored. Emerging observational evidence indicates cardiovascular benefits from extended rather than reduced eating windows and increased mortality risk associated with long-term application of TRE. Such adverse effects may be linked to the loss of lean mass, circadian misalignment and poor dietary choices associated with reward-based eating in genetically-predisposed individuals (Figure 1). Conversely, advances in obesity pharmacotherapy offer highly effective alternatives for managing body weight and cardiometabolic risk, yet their long-term safety profiles also require better evaluation.
As we continue to navigate the intricate balance between diet, pharmacotherapy, and cardiovascular risk, it is essential that future research prioritizes the development of integrative approaches that not only address the immediate challenges of obesity but also mitigate long-term cardiometabolic risks, ensuring that treatment strategies are both effective and sustainable. We propose that such a balanced strategy should incorporate evidence-based dietary patterns with proven cardiometabolic benefits, such as Mediterranean diets. Underpinned by genetic and nutritional counseling, the integration of genetic parameters influencing the response to dietary components, type of exercise, different pharmacotherapies and behavioral traits, such as adherence and reward eating, as well as their interactions, will enable further personalization and greater potential for improved pharmacotherapy outcomes in patients with obesity (73, 80–83).
The original contributions presented in this study are included in this article/supplementary material, further inquiries can be directed to the corresponding author.
AE: Conceptualization, Investigation, Writing – original draft, Writing – review and editing. KG: Data curation, Writing – original draft. KT: Data curation, Writing – original draft. DS: Data curation, Funding acquisition, Writing – original draft.
The author(s) declare that financial support was received for the research, authorship, and/or publication of this article. DS is supported by CUREPLaN, a grant from the Leducq Foundation for Cardiovascular Research (18CVD01).
AE and KG were employed by Genosophy S.A.
The remaining authors declare that the research was conducted in the absence of any commercial or financial relationships that could be construed as a potential conflict of interest.
The author(s) declared that they were an editorial board member of Frontiers, at the time of submission. This had no impact on the peer review process and the final decision.
The authors declare that no Generative AI was used in the creation of this manuscript.
All claims expressed in this article are solely those of the authors and do not necessarily represent those of their affiliated organizations, or those of the publisher, the editors and the reviewers. Any product that may be evaluated in this article, or claim that may be made by its manufacturer, is not guaranteed or endorsed by the publisher.
1. Varady K, Cienfuegos S, Ezpeleta M, Gabel K. Clinical application of intermittent fasting for weight loss: Progress and future directions. Nat Rev Endocrinol. (2022) 18(5):309–21. doi: 10.1038/s41574-022-00638-x
2. Patikorn C, Roubal K, Veettil S, Chandran V, Pham T, Lee Y, et al. Intermittent fasting and obesity-related health outcomes: An umbrella review of meta-analyses of randomized clinical trials. JAMA Netw Open. (2021) 4(12):e2139558. doi: 10.1001/jamanetworkopen.2021.39558
3. Varady K, Cienfuegos S, Ezpeleta M, Gabel K. Cardiometabolic benefits of intermittent fasting. Annu Rev Nutr. (2021) 41:333–61.
4. Arumugam T, Alli-Shaik A, Liehn E, Selvaraji S, Poh L, Rajeev V, et al. Multiomics analyses reveal dynamic bioenergetic pathways and functional remodeling of the heart during intermittent fasting. Elife. (2023) 12:R89214. doi: 10.7554/eLife.89214
5. Park J, Seo Y, Paek Y, Song H, Park K, Noh H. Effect of alternate-day fasting on obesity and cardiometabolic risk: A systematic review and meta-analysis. Metabolism. (2020) 111:154336. doi: 10.1016/j.metabol.2020.154336
6. Marko D, Conn M, Schertzer J. Intermittent fasting influences immunity and metabolism. Trends Endocrinol Metab. (2024) 35(9):821–33.
7. de Cabo R, Mattson M. Effects of intermittent fasting on health, aging, and disease. N Engl J Med. (2019) 381(26):2541–51.
8. Liu D, Huang Y, Huang C, Yang S, Wei X, Zhang P, et al. Calorie restriction with or without time-restricted eating in weight loss. N Engl J Med. (2022) 386(16):1495–504.
9. Lowe D, Wu N, Rohdin-Bibby L, Moore A, Kelly N, Liu Y, et al. Effects of time-restricted eating on weight loss and other metabolic parameters in women and men with overweight and obesity: The TREAT randomized clinical trial. JAMA Intern Med. (2020) 180(11):1491–9. doi: 10.1001/jamainternmed.2020.4153
10. Trepanowski J, Kroeger C, Barnosky A, Klempel M, Bhutani S, Hoddy K, et al. Effect of alternate-day fasting on weight loss, weight maintenance, and cardioprotection among metabolically healthy obese adults: A randomized clinical trial. JAMA Intern Med. (2017) 177(7):930–8. doi: 10.1001/jamainternmed.2017.0936
11. Harvie M, Pegington M, Mattson M, Frystyk J, Dillon B, Evans G, et al. The effects of intermittent or continuous energy restriction on weight loss and metabolic disease risk markers: A randomized trial in young overweight women. Int J Obes (Lond). (2011) 35(5):714–27.
12. Schroor M, Joris P, Plat J, Mensink R. Effects of intermittent energy restriction compared with those of continuous energy restriction on body composition and cardiometabolic risk markers - A systematic review and meta-analysis of randomized controlled trials in adults. Adv Nutr. (2024) 15(1):100130. doi: 10.1016/j.advnut.2023.10.003
13. Templeman I, Smith H, Chowdhury E, Chen Y, Carroll H, Johnson-Bonson D, et al. A randomized controlled trial to isolate the effects of fasting and energy restriction on weight loss and metabolic health in lean adults. Sci Transl Med. (2021) 13(598):eabd8034.
14. Hutchison A, Liu B, Wood R, Vincent A, Thompson C, O’Callaghan N, et al. Effects of intermittent versus continuous energy intakes on insulin sensitivity and metabolic risk in women with overweight. Obesity (Silver Spring). (2019) 27(1):50–8.
15. Billingsley H, St-Onge M, Alonso W, Kirkman D, Kim Y, Carbone S. Time of eating and mortality in U.S. adults with heart failure: Analyses of the national health and nutrition examination survey 2003-2018. Nutr Metab Cardiovasc Dis. (2024) 34(2):445–54. doi: 10.1016/j.numecd.2023.10.013
16. Chen M, Zhong VW. Association Between Time-Restricted Eating and All-Cause and Cause-Specific Mortality. (2024). Available online at: https://www.ahajournals.org/doi/10.1161/circ.149.suppl_1.P192
17. Ahmet I, Wan R, Mattson M, Lakatta E, Talan M. Chronic alternate-day fasting results in reduced diastolic compliance and diminished systolic reserve in rats. J Card Fail. (2010) 16(10):843–53. doi: 10.1016/j.cardfail.2010.05.007
18. Sutton C, L’Insalata A, Fazzino T. Reward sensitivity, eating behavior, and obesity-related outcomes: A systematic review. Physiol Behav. (2022) 252:113843. doi: 10.1016/j.physbeh.2022.113843
19. Pannen S, Maldonado S, Nonnenmacher T, Sowah S, Gruner L, Watzinger C, et al. Adherence and dietary composition during intermittent vs. continuous calorie restriction: Follow-up data from a randomized controlled trial in adults with overweight or obesity. Nutrients. (2021) 13(4):1195.
20. Harvie M, Wright C, Pegington M, McMullan D, Mitchell E, Martin B, et al. The effect of intermittent energy and carbohydrate restriction v. daily energy restriction on weight loss and metabolic disease risk markers in overweight women. Br J Nutr. (2013) 110(8):1534–47. doi: 10.1017/S0007114513000792
21. Sundfor T, Tonstad S, Svendsen M. Effects of intermittent versus continuous energy restriction for weight loss on diet quality and eating behavior. A randomized trial. Eur J Clin Nutr. (2019) 73(7):1006–14. doi: 10.1038/s41430-018-0370-0
22. Jospe M, Roy M, Brown R, Haszard J, Meredith-Jones K, Fangupo L, et al. Intermittent fasting, Paleolithic, or Mediterranean diets in the real world: Exploratory secondary analyses of a weight-loss trial that included choice of diet and exercise. Am J Clin Nutr. (2020) 111(3):503–14.
23. Panizza C, Lim U, Yonemori K, Cassel K, Wilkens L, Harvie M, et al. Effects of intermittent energy restriction combined with a mediterranean diet on reducing visceral adiposity: A randomized active comparator pilot study. Nutrients. (2019) 11(6):1386.
24. Pinto A, Bordoli C, Buckner L, Kim C, Kaplan P, Del Arenal I, et al. Intermittent energy restriction is comparable to continuous energy restriction for cardiometabolic health in adults with central obesity: A randomized controlled trial; the Met-IER study. Clin Nutr. (2020) 39(6):1753–63. doi: 10.1016/j.clnu.2019.07.014
25. Kroeger C, Trepanowski J, Klempel M, Barnosky A, Bhutani S, Gabel K, et al. Eating behavior traits of successful weight losers during 12 months of alternate-day fasting: An exploratory analysis of a randomized controlled trial. Nutr Health. (2018) 24(1):5–10. doi: 10.1177/0260106017753487
26. Sun Y, Liu B, Snetselaar L, Wallace R, Shadyab A, Kroenke C, et al. Association of major dietary protein sources with all-cause and cause-specific mortality: Prospective cohort study. J Am Heart Assoc. (2021) 10(5):e015553.
27. Chen Z, Glisic M, Song M, Aliahmad H, Zhang X, Moumdjian A, et al. Dietary protein intake and all-cause and cause-specific mortality: Results from the Rotterdam Study and a meta-analysis of prospective cohort studies. Eur J Epidemiol. (2020) 35(5):411–29. doi: 10.1007/s10654-020-00607-6
28. Lee Y, Nemet I, Wang Z, Lai H, de Oliveira Otto M, Lemaitre R, et al. Longitudinal plasma measures of Trimethylamine N-Oxide and risk of atherosclerotic cardiovascular disease events in community-based older adults. J Am Heart Assoc. (2021) 10(17):e020646. doi: 10.1161/JAHA.120.020646
29. Tang W, Wang Z, Levison B, Koeth R, Britt E, Fu X, et al. Intestinal microbial metabolism of phosphatidylcholine and cardiovascular risk. N Engl J Med. (2013) 368(17):1575–84.
30. Mohr A, Jasbi P, Bowes D, Dirks B, Whisner C, Arciero K, et al. Exploratory analysis of one versus two-day intermittent fasting protocols on the gut microbiome and plasma metabolome in adults with overweight/obesity. Front Nutr. (2022) 9:1036080. doi: 10.3389/fnut.2022.1036080
31. Cava E, Yeat N, Mittendorfer B. Preserving healthy muscle during weight loss. Adv Nutr. (2017) 8(3):511–9.
32. Kim S, Lee G, Choi S, Oh Y, Son J, Park M, et al. Changes in predicted lean body mass, appendicular skeletal muscle mass, and body fat mass and cardiovascular disease. J Cachexia Sarcopenia Muscle. (2022) 13(2):1113–23.
33. Tyrovolas S, Panagiotakos D, Georgousopoulou E, Chrysohoou C, Tousoulis D, Haro J, et al. Skeletal muscle mass in relation to 10 year cardiovascular disease incidence among middle aged and older adults: The ATTICA study. J Epidemiol Community Health. (2020) 74(1):26–31. doi: 10.1136/jech-2019-212268
34. Oterdoom L, Gansevoort R, Schouten J, de Jong P, Gans R, Bakker S. Urinary creatinine excretion, an indirect measure of muscle mass, is an independent predictor of cardiovascular disease and mortality in the general population. Atherosclerosis. (2009) 207(2):534–40. doi: 10.1016/j.atherosclerosis.2009.05.010
35. Scheer F, Hilton M, Mantzoros C, Shea S. Adverse metabolic and cardiovascular consequences of circadian misalignment. Proc Natl Acad Sci U S A. (2009) 106(11):4453–8.
36. St-Onge M, Ard J, Baskin M, Chiuve S, Johnson H, Kris-Etherton P, et al. Meal timing and frequency: Implications for cardiovascular disease prevention: A scientific statement from the American heart association. Circulation. (2017) 135(9):e96–121. doi: 10.1161/CIR.0000000000000476
37. Chen H, Zhang B, Ge Y, Shi H, Song S, Xue W, et al. Association between skipping breakfast and risk of cardiovascular disease and all cause mortality: A meta-analysis. Clin Nutr. (2020) 39(10):2982–8.
38. Cahill L, Chiuve S, Mekary R, Jensen M, Flint A, Hu F, et al. Prospective study of breakfast eating and incident coronary heart disease in a cohort of male US health professionals. Circulation. (2013) 128(4):337–43. doi: 10.1161/CIRCULATIONAHA.113.001474
39. Ballon A, Neuenschwander M, Schlesinger S. Breakfast skipping is associated with increased risk of type 2 diabetes among adults: A systematic review and meta-analysis of prospective cohort studies. J Nutr. (2019) 149(1):106–13. doi: 10.1093/jn/nxy194
40. Lopez-Minguez J, Dashti H, Madrid-Valero J, Madrid J, Saxena R, Scheer F, et al. Heritability of the timing of food intake. Clin Nutr. (2019) 38(2):767–73.
41. Dashti H, Merino J, Lane J, Song Y, Smith C, Tanaka T, et al. Genome-wide association study of breakfast skipping links clock regulation with food timing. Am J Clin Nutr. (2019) 110(2):473–84. doi: 10.1093/ajcn/nqz076
42. Garaulet M, Lee Y, Shen J, Parnell L, Arnett D, Tsai M, et al. CLOCK genetic variation and metabolic syndrome risk: Modulation by monounsaturated fatty acids. Am J Clin Nutr. (2009) 90(6):1466–75. doi: 10.3945/ajcn.2009.27536
43. Zhang X, Wu Y, Na M, Lichtenstein A, Xing A, Chen S, et al. Habitual night eating was positively associated with progress of arterial stiffness in Chinese adults. J Am Heart Assoc. (2020) 9(19):e016455. doi: 10.1161/JAHA.120.016455
44. Palomar-Cros A, Andreeva V, Fezeu L, Julia C, Bellicha A, Kesse-Guyot E, et al. Dietary circadian rhythms and cardiovascular disease risk in the prospective NutriNet-Sante cohort. Nat Commun. (2023) 14(1):7899. doi: 10.1038/s41467-023-43444-3
45. Simon S, Blankenship J, Manoogian E, Panda S, Mashek D, Chow L. The impact of a self-selected time restricted eating intervention on eating patterns, sleep, and late-night eating in individuals with obesity. Front Nutr. (2022) 9:1007824. doi: 10.3389/fnut.2022.1007824
46. Zhang D, Colson J, Jin C, Becker B, Rhoads M, Pati P, et al. Timing of food intake drives the circadian rhythm of blood pressure. Function (Oxf). (2021) 2(1):zqaa034. doi: 10.1093/function/zqaa034
47. Witbracht M, Keim N, Forester S, Widaman A, Laugero K. Female breakfast skippers display a disrupted cortisol rhythm and elevated blood pressure. Physiol Behav. (2015) 140:215–21. doi: 10.1016/j.physbeh.2014.12.044
48. Ortiz R, Kluwe B, Lazarus S, Teruel M, Joseph J. Cortisol and cardiometabolic disease: A target for advancing health equity. Trends Endocrinol Metab. (2022) 33(11):786–97. doi: 10.1016/j.tem.2022.08.002
49. Jia G, Aroor A, Hill M, Sowers J. Role of renin-angiotensin-aldosterone system activation in promoting cardiovascular fibrosis and stiffness. Hypertension. (2018) 72(3):537–48.
50. Hutchison A, Regmi P, Manoogian E, Fleischer J, Wittert G, Panda S, et al. Time-restricted feeding improves glucose tolerance in men at risk for type 2 diabetes: A randomized crossover trial. Obesity (Silver Spring). (2019) 27(5):724–32. doi: 10.1002/oby.22449
51. Xu B, Xie X. Neurotrophic factor control of satiety and body weight. Nat Rev Neurosci. (2016) 17(5):282–92.
52. Alkurd R, Mahrous L, Zeb F, Khan M, Alhaj H, Khraiwesh H, et al. Effect of calorie restriction and intermittent fasting regimens on brain-derived neurotrophic factor levels and cognitive function in humans: A systematic review. Medicina (Kaunas). (2024) 60(1):191. doi: 10.3390/medicina60010191
53. Smith A, Malan L, Uys A, Malan N, Harvey B, Ziemssen T. Attenuated brain-derived neurotrophic factor and hypertrophic remodelling: The SABPA study. J Hum Hypertens. (2015) 29(1):33–9. doi: 10.1038/jhh.2014.39
54. Schutte C, Malan L, Scheepers J, Oosthuizen W, Cockeran M, Malan N. Cortisol:brain-derived neurotrophic factor ratio associated with silent ischaemia in a black male cohort: The SA BPA study. Cardiovasc J Afr. (2016) 27(6):387–91.
55. Jiang R, Babyak M, Brummett B, Hauser E, Shah S, Becker R, et al. Brain-derived neurotrophic factor rs6265 (Val66Met) polymorphism is associated with disease severity and incidence of cardiovascular events in a patient cohort. Am Heart J. (2017) 190:40–5. doi: 10.1016/j.ahj.2017.05.002
56. Negron M, Kristensen J, Nguyen V, Gansereit L, Raucci F, Chariker J, et al. Sex-based differences in cardiac gene expression and function in BDNF Val66Met mice. Int J Mol Sci. (2021) 22(13):7002. doi: 10.3390/ijms22137002
57. Chakhtoura M, Haber R, Ghezzawi M, Rhayem C, Tcheroyan R, Mantzoros C. Pharmacotherapy of obesity: An update on the available medications and drugs under investigation. EClinicalMedicine. (2023) 58:101882.
58. Ryan D, Lingvay I, Colhoun H, Deanfield J, Emerson S, Kahn S, et al. Semaglutide effects on cardiovascular outcomes in people with overweight or obesity (SELECT) rationale and design. Am Heart J. (2020) 229:61–9.
59. Rosenstock J, Frias J, Jastreboff A, Du Y, Lou J, Gurbuz S, et al. Retatrutide, a GIP, GLP-1 and glucagon receptor agonist, for people with type 2 diabetes: A randomised, double-blind, placebo and active-controlled, parallel-group, phase 2 trial conducted in the USA. Lancet. (2023) 402(10401):529–44. doi: 10.1016/S0140-6736(23)01053-X
60. Jastreboff A, Kaplan L, Frias J, Wu Q, Du Y, Gurbuz S, et al. Triple-hormone-receptor agonist retatrutide for obesity - A phase 2 trial. N Engl J Med. (2023) 389(6):514–26.
61. Jastreboff A, Aronne L, Ahmad N, Wharton S, Connery L, Alves B, et al. Tirzepatide once weekly for the treatment of obesity. N Engl J Med. (2022) 387(3):205–16.
62. Rubino D, Greenway F, Khalid U, O’Neil P, Rosenstock J, Sorrig R, et al. Effect of weekly subcutaneous semaglutide vs daily liraglutide on body weight in adults with overweight or obesity without diabetes: The STEP 8 randomized clinical trial. JAMA. (2022) 327(2):138–50.
63. Farr O, Sofopoulos M, Tsoukas M, Dincer F, Thakkar B, Sahin-Efe A, et al. GLP-1 receptors exist in the parietal cortex, hypothalamus and medulla of human brains and the GLP-1 analogue liraglutide alters brain activity related to highly desirable food cues in individuals with diabetes: A crossover, randomised, placebo-controlled trial. Diabetologia. (2016) 59(5):954–65. doi: 10.1007/s00125-016-3874-y
64. Uchiyama S, Sada Y, Mihara S, Sasaki Y, Sone M, Tanaka Y. Oral semaglutide induces loss of body fat mass without affecting muscle mass in patients with type 2 diabetes. J Clin Med Res. (2023) 15(7):377–83. doi: 10.14740/jocmr4987
65. Xiang J, Ding X, Zhang W, Zhang J, Zhang Y, Li Z, et al. Clinical effectiveness of semaglutide on weight loss, body composition, and muscle strength in Chinese adults. Eur Rev Med Pharmacol Sci. (2023) 27(20):9908–15. doi: 10.26355/eurrev_202310_34169
66. Lincoff A, Brown-Frandsen K, Colhoun H, Deanfield J, Emerson S, Esbjerg S, et al. Semaglutide and cardiovascular outcomes in obesity without diabetes. N Engl J Med. (2023) 389(24):2221–32.
67. Sanoudou D, Mantzoros C, Hill M. Sodium-glucose cotransporter-2 inhibitors: A treatment option for recurrent vasovagal syndrome? Metabolism. (2022) 137:155309. doi: 10.1016/j.metabol.2022.155309
68. Sarafidis P, Ferro C, Morales E, Ortiz A, Malyszko J, Hojs R, et al. SGLT-2 inhibitors and GLP-1 receptor agonists for nephroprotection and cardioprotection in patients with diabetes mellitus and chronic kidney disease. A consensus statement by the EURECA-m and the DIABESITY working groups of the ERA-EDTA. Nephrol Dial Transplant. (2019) 34(2):208–30.
69. Liu H, Sridhar V, Boulet J, Dharia A, Khan A, Lawler P, et al. Cardiorenal protection with SGLT2 inhibitors in patients with diabetes mellitus: From biomarkers to clinical outcomes in heart failure and diabetic kidney disease. Metabolism. (2022) 126:154918. doi: 10.1016/j.metabol.2021.154918
70. Wadden T, Chao A, Machineni S, Kushner R, Ard J, Srivastava G, et al. Tirzepatide after intensive lifestyle intervention in adults with overweight or obesity: The SURMOUNT-3 phase 3 trial. Nat Med. (2023) 29(11):2909–18.
71. Gkouskou K, Lazou E, Skoufas E, Eliopoulos AG. Genetically guided mediterranean diet for the personalized nutritional management of type 2 diabetes mellitus. Nutrients. (2021) 13(2):355.
72. Gkouskou K, Grammatikopoulou M, Vlastos I, Sanoudou D, Eliopoulos AG. Genotype-guided dietary supplementation in precision nutrition. Nutr Rev. (2021) 79(11):1225–35. doi: 10.1093/nutrit/nuaa132
73. Gkouskou K, Grammatikopoulou M, Lazou E, Vasilogiannakopoulou T, Sanoudou D, Eliopoulos AG. A genomics perspective of personalized prevention and management of obesity. Hum Genomics. (2024) 18(1):4.
74. Martinez-Gonzalez M, Gea A, Ruiz-Canela M. The mediterranean diet and cardiovascular health. Circ Res. (2019) 124(5):779–98.
75. Salas-Salvado J, Diaz-Lopez A, Ruiz-Canela M, Basora J, Fito M, Corella D, et al. Effect of a lifestyle intervention program with energy-restricted mediterranean diet and exercise on weight loss and cardiovascular risk factors: One-year results of the PREDIMED-plus trial. Diabetes Care. (2019) 42(5):777–88. doi: 10.2337/dc18-0836
76. Dinu M, Pagliai G, Angelino D, Rosi A, Dall’Asta M, Bresciani L, et al. Effects of popular diets on anthropometric and cardiometabolic parameters: An umbrella review of meta-analyses of randomized controlled trials. Adv Nutr. (2020) 11(4):815–33. doi: 10.1093/advances/nmaa006
77. Goodpaster B, Delany J, Otto A, Kuller L, Vockley J, South-Paul J, et al. Effects of diet and physical activity interventions on weight loss and cardiometabolic risk factors in severely obese adults: A randomized trial. JAMA. (2010) 304(16):1795–802.
78. Chomiuk T, Niezgoda N, Mamcarz A, Sliz D. Physical activity in metabolic syndrome. Front Physiol. (2024) 15:1365761. doi: 10.3389/fphys.2024.1365761
79. Tronieri J, Fabricatore A, Wadden T, Auerbach P, Endahl L, Sugimoto D, et al. Effects of dietary self-monitoring, physical activity, Liraglutide 3.0 mg, and placebo on weight loss in the SCALE IBT trial. Obes Facts. (2020) 13(6):572–83. doi: 10.1159/000511130
80. Stouras I, Papaioannou T, Tsioufis K, Eliopoulos A, Sanoudou D. The challenge and importance of integrating drug-nutrient-genome interactions in personalized cardiovascular healthcare. J Pers Med. (2022) 12(4):513. doi: 10.3390/jpm12040513
81. Gkouskou K, Vlastos I, Karkalousos P, Chaniotis D, Sanoudou D, Eliopoulos AG. The “virtual digital twins” concept in precision nutrition. Adv Nutr. (2020) 11(6):1405–13. doi: 10.1093/advances/nmaa089
82. Kyriakidou A, Kyriazou A, Koufakis T, Vasilopoulos Y, Avramidis I, Baltagiannis S, et al. Association between variants in TCF7L2, CTRB1/2, and GLP-1R genes and response to therapy with glucagon-like peptide-1 receptor agonists. Postgrad Med. (2024) 136(2):218–25. doi: 10.1080/00325481.2024.2328513
Keywords: intermittent fasting, precision medicine, anti-obesity pharmacotherapy, cardiovascular disease, genetics, GLP-1, GIP
Citation: Eliopoulos AG, Gkouskou KK, Tsioufis K and Sanoudou D (2025) A perspective on intermittent fasting and cardiovascular risk in the era of obesity pharmacotherapy. Front. Nutr. 12:1524125. doi: 10.3389/fnut.2025.1524125
Received: 07 November 2024; Accepted: 02 January 2025;
Published: 17 January 2025.
Edited by:
Xiaoyong Yu, Shaanxi Provincial Hospital of Traditional Chinese Medicine, ChinaReviewed by:
Fahd Beddar, Universidad de Valladolid, SpainCopyright © 2025 Eliopoulos, Gkouskou, Tsioufis and Sanoudou. This is an open-access article distributed under the terms of the Creative Commons Attribution License (CC BY). The use, distribution or reproduction in other forums is permitted, provided the original author(s) and the copyright owner(s) are credited and that the original publication in this journal is cited, in accordance with accepted academic practice. No use, distribution or reproduction is permitted which does not comply with these terms.
*Correspondence: Aristides G. Eliopoulos, ZWxpb3BhZ0BtZWQudW9hLmdy
Disclaimer: All claims expressed in this article are solely those of the authors and do not necessarily represent those of their affiliated organizations, or those of the publisher, the editors and the reviewers. Any product that may be evaluated in this article or claim that may be made by its manufacturer is not guaranteed or endorsed by the publisher.
Research integrity at Frontiers
Learn more about the work of our research integrity team to safeguard the quality of each article we publish.