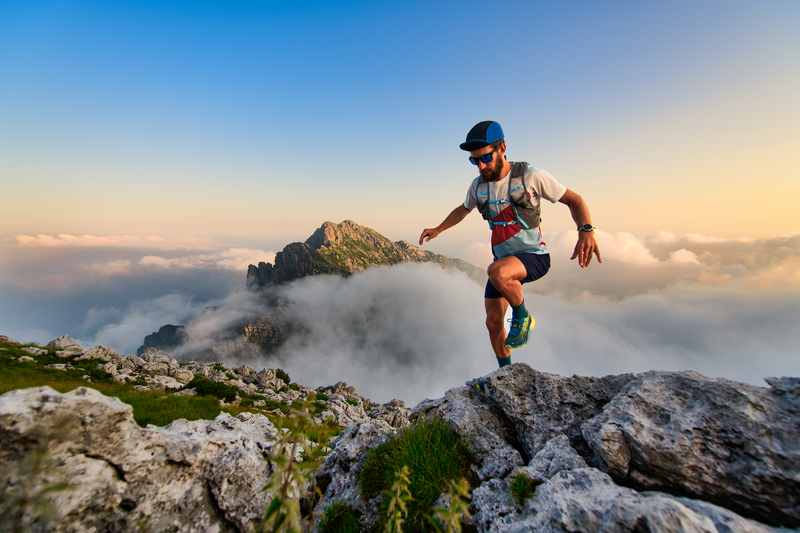
95% of researchers rate our articles as excellent or good
Learn more about the work of our research integrity team to safeguard the quality of each article we publish.
Find out more
REVIEW article
Front. Nutr. , 23 January 2025
Sec. Nutrition and Metabolism
Volume 12 - 2025 | https://doi.org/10.3389/fnut.2025.1508695
This article is part of the Research Topic Ketogenic Metabolic Therapies in Prevention & Treatment of Non-communicable Diseases View all 11 articles
Diet has been proven to have significant impacts on the pathogenesis and treatment of osteoporosis. This review attempts to elucidate the current progress and controversy surrounding the ketogenic diet (KD) and β-hydroxybutyrate (BHB) in osteoporosis and offers a novel perspective on the prevention and treatment of osteoporosis. The ketogenic diet has been broadly used in the treatment of epilepsy, diabetes, obesity, and certain neoplasms by triggering ketone bodies, mainly BHB. However, in most osteoporosis-related clinical and preclinical studies, the ketogenic diet has demonstrated the detrimental effects of inhibiting bone accumulation and damaging bone microarchitecture. In contrast, BHB is thought to ameliorate osteoporosis by promoting osteoblastogenesis and inhibiting osteoclastogenesis. The main purpose of this review is to summarize the current research progress and hope that more basic and clinical experiments will focus on the similarities and differences between ketogenic diet (KD) and BHB in osteoporosis.
Low bone mass and damage to the microarchitecture of bone tissue, which increases bone fragility and fracture risk, are the hallmarks of osteoporosis, a systemic metabolic bone disease (1). An imbalance between bone resorption, mediated by osteoclasts, and bone generation, mediated by osteoblasts, is the fundamental process driving osteoporosis. Loss of gonadal function and aging are also major factors in the development of the disease (2). Bisphosphonates, as first-line medications for osteoporosis, are not always feasible due to their side effects (3), Instead, dietary modifications, like eating more calcium, vitamin D, and protein and following the Mediterranean diet, may have broader applicability (4–7).
The ketogenic diet is a special dietary structure with high fat, low carbohydrates, and moderate protein contents (8). The metabolic pattern shifts from glucose metabolism to fat metabolism during the ketogenic diet. Fatty acids are metabolized by the liver to produce ketone bodies, leading to nutritional ketosis. β-hydroxybutyrate (BHB), as the highest-content ketone body, not only provides energy but also participates in many metabolism processes in the body as a signal molecule, such as binding to G protein-coupled receptors (GPCRs) or histone deacetylase (HDAC) to induce anti-inflammatory and antioxidant functions. Meanwhile, the ketogenic diet has also been reported to be beneficial for various diseases, such as type 2 diabetes mellitus and neurodegenerative diseases (9–11). Recently, attention has been paid to the impact of a ketogenic diet on osteoporosis; however, BHB seems to have different outcomes for osteoporosis. In this review, we summarize the possible mechanisms underlying the actions of ketogenic diet and BHB in osteoporosis, hoping to provide directions for future research.
Woodyatt et al. first identified ketone bodies in the blood of healthy subjects on a starvation diet or a high-fat, low-carbohydrate (HF-LC) diet in 1921. Ketone bodies are intermediate products of fatty acid β-oxidation during starvation or certain pathological states. Acetyl coenzyme A, which is converted from free fatty acids, is transported to hepatocyte mitochondria through carnitine palmitoyl transferase and then converted to acetoacetate through a series of biochemical reactions. β-hydroxybutyrate dehydrogenase 1 (BDH1) reduces acetoacetate to β-hydroxybutyrate (BHB), which enters the blood circulation via the monocarboxylic acid transporter (12–14). Serum levels of BHB are quite low under normal circumstances but can reach 1–2 mM after fasting and even 6–8 mM with prolonged starvation. Meanwhile, BHB levels can spike to 20 mM in diabetic ketoacidosis (12, 15). As a major component of ketone bodies, BHB can be used by metabolically active tissues, such as the heart, brain, and muscles, where it generates cellular energy in mitochondria (13, 16). Recently, in addition to serving as an alternative fuel during starvation, more and more studies have found that BHB ameliorates oxidative stress-inflammatory states by inhibiting HDAC or binding to GPCRs (12, 16). Besides that, research on new histone post-translational modifications (HPTMs) has shown that BHB as a histone modification β-hydroxybutyrylation modified substrate regulates epigenetics (17), which may play an important role in the development of cardiovascular diseases, metabolic diseases, and other diseases (18–21).
Simultaneously, the “ketogenic diet”, which is heavy in fat and low in carbohydrates, was initially suggested by Wilder et al. at the Mayo Clinic to treat patients with refractory epilepsy by simulating a fasting condition. As such, the classic ketogenic diet was born (8, 22). With the introduction of anti-epileptic drugs, the use of the ketogenic diet declined dramatically. However, in recent decades, the ketogenic diet has drawn extensive attention again. Four variants of the ketogenic diet have emerged to provide flexibility to boost adherence: the classic ketogenic diet (cKD), the modified Atkins diet (MAD), the low glycaemic index treatment (LGIT), and the medium-chain triglyceride ketogenic diet (MCTKD) (23). The most conventional type of ketogenic diet is the classic one, which has a 4:1 ratio of fat to protein and carbs (grams to ams). Under this diet, fats provide 90% of the energy, and they are mainly derived from long-chain fatty acids, with carbohydrates and proteins providing the remaining 10%. The MCTKD provides 70%−75% of total daily energy from fat, 15%−18% from carbohydrates, and 10% from protein. Unlike the classical ketogenic diet, the MCTKD consumes more medium-chain triglycerides, which have a higher ketogenic efficiency and are more acceptable. The MAD is a much less restrictive diet with a 1:1–2:1 ratio of fat to protein and carbs (grams to ams). Here, patients can consume 1 g of carbohydrates and protein for every 1–2 g of fat consumed. During the low glycaemic index treatment, 40–60 g of carbohydrates per day is allowed, but the glycaemic index of the carbohydrate source needs to be < 50, while fat and protein intakes are not restricted. It is worth mentioning that very low-energy ketogenic therapy (VLEKT) is a novel nutritional regimen frequently employed in the management of overweight and obese individuals. VLEKT prioritizes complete calorie restrictions, permitting a total energy intake of less than 800 kcal per day, with carbohydrates limited to around 30 g/day, while fats and proteins contribute ~44% and 43%, respectively (24, 25). During the ketogenic diet, fatty acid metabolism produces ketone bodies, and ketone body levels are elevated (23, 26).
Growing evidence has demonstrated that patients with different diseases can benefit from the ketogenic diet. The ketogenic diet can assist in managing obesity and type 2 diabetes mellitus by improving fat catabolism and raising insulin sensitivity to lower blood lipids, facilitate weight loss, and improve glycosylated hemoglobin and glycaemia (10, 27). Studies have shown that the ketogenic diet can slow tumor growth, inhibit tumor metastasis, and increase sensitivity to radiotherapy or chemotherapy (28–30). It is also beneficial for the failing heart by driving increased use of ketone bodies, which can be an alternate fuel to address fuel metabolic deficits (31). Moreover, the ketogenic diet partially drives gut microbial shifts, which alleviates colitis (32, 33).
For normal longitudinal bone growth and bone mass build-up, growth hormone (GH) and insulin-like growth factor I (IGF-1) are necessary (34–36). IGF-1 mediates most impacts of GH on skeletal metabolism. Chondrocytes and osteoblasts in bone growth centers are targets of GH and IGF-1 action. Osteoblasts, the cells responsible for bone formation, originate from bone marrow-derived mesenchymal stem cells (BMSCs) (37). IGF-1 binds to the IGF-1 receptor on preosteoblasts and uses the phosphatidylinositol-3 kinase (PI3K)/Protein kinase B (PKB) pathway to promote stabilization of β-catenin, a signal molecule of the wingless-related integration site (Wnt) canonical pathway, which is critical for osteoblastogenesis (34, 38, 39). Stabilized β-catenin moves into the cell nucleus and attaches to members of the T-cell factor/lymphoid-enhancer factor family of nuclear proteins to regulate gene transcription, such as runt-related transcription factor 2 (RUNX2) and osterix, and promote osteoblastogenesis (38). The efficiency and rate of differentiation of preosteoblasts into mature osteoblasts control the rate of bone formation. Additionally, IGF-1 can also decrease osteoblast apoptosis and moderately promote osteoblast proliferation (40, 41).
GH and calorie intake primarily drive the expression of IGF-1, and IGF-binding proteins (IGFBPs) regulate its availability (36, 42). The ketogenic diet induces a catabolic state similar to starvation. This state increases the levels of IGFBPs, leading to suppression of IGF-1 activity (Figure 1) (43). Research also suggests that a HF-LC diet, as opposed to one with a higher overall calorie intake, may modulate the genetic programming of growth. Consumption of such diets leads to decreased concentrations of circulating GH and IGF-1, which ultimately impair osteogenic differentiation (44). Moreover, the increase in BHB levels caused GH axis by the ketogenic diet can create a chronic acidic environment, which may disrupt the axis (45, 46). Previous studies have found that in chronic metabolic acidosis, human serum IGF-1 concentrations are significantly reduced, and the response of IGF-1 to GH is diminished (45). The role of IGF-1 in promoting the proliferation and differentiation of chondrocytes was significantly diminished in mandibular condyles cultured under acidic conditions (46).
Growing evidence has demonstrated that the acid environment caused by the ketogenic diet can impair bone mineralization (Figure 1) (47, 48). Osteoblasts synthesize and secrete bone matrix, such as collagen type 1 and osteocalcin, to form dense, laminated collagen fibers. And then inorganic salts such as calcium phosphate are deposited to mineralize and harden the bone matrix. Blood pH is usually normal on a ketogenic diet, but serum bicarbonate is below normal levels, suggesting an underproduction or increased demand for associated bicarbonate ions (48). Chronic acidosis reduces bone mineral content (BMC) by speeding up the liberation of cations from bone in conjunction with bicarbonate to provide the necessary extra buffering capacity (49). This is possible due to the huge amount of potential proton buffers that bone contains. Moreover, acidosis interferes with the conversion of serum 25-hydroxyvitamin D (25(OH)D) to 1,25 dihydroxyvitamin D3 (1,25(OH)2D3), exacerbating bone damage (50).
The ketogenic diet often causes weight loss (27), however, weight loss always results in bone loss and increased fracture risk (Figure 1) (51–53). According to the theory of mechanical homeostasis, weight loss leads to the mechanical unloading of bones, resulting in a decrease in bone mass (54). Osteocytes are particularly susceptible to biomechanical stress, and they undergo apoptosis without loading. In the absence of loading, osteoblastic activity will be suppressed and osteoclast differentiation will be encouraged (54, 55). Another possible explanation is that there are interactions among endocrine, inflammatory, and bone (56). Yu et al. found that bone resorption marker β-Crosslaps increased and bone formation marker procollagen I N-terminal propeptide (PINP) decreased after 10% body weight was lost (57). Although most studies have shown that weight loss often leads to a decrease in adipose tissue, we do not consider the inhibition of osteogenic activity caused by a decrease in estrogen and other factors caused by adipose tissue reduction.
The ketogenic diet has a high fat content, which causes bone loss through local and systemic pathways (Figure 1) (58). Firstly, the current results of animal studies have shown that a high-fat diet leads to increased bone marrow tissue fat (59, 60). Bone marrow adipose tissue (BMAT) and osteoblasts are from BMSCs. The more bone marrow adipocytes there are, the fewer osteoblasts there are (61, 62). Secondly, there are numerous pieces of evidence showing that high-fat diet intake is associated with an increase in adipokines. Adipokines such as chemerin and resistin promote osteoclast differentiation (63–65), while adiponectin and leptin have a dual effect on bone cell differentiation (66–69). Generally speaking, the positive action of adipokines is not enough to counteract the negative effects, resulting in bone resorption (70, 71). What's more, a continuous high-fat diet leads to up-regulation of inflammatory genes, such as Fam3c, InhBa, Tnfsf11, and Ackr2. Inflammatory cytokines not only participate in inflammatory activities but also in bone cell differentiation (71). Previous studies indicate that inflammatory factors motivate osteoclast overactivity primarily via the receptor activator of nuclear factor-κB ligand (RANKL) signal pathway and inhibit bone marrow-derived mesenchymal stem cell differentiation into osteoblasts (72). A high-fat diet is a source of palmitic acid, which inhibits osteoblastogenesis and function and promotes apoptosis by decreasing the expression and activity of osteogenic markers such as RUNX2, alkaline phosphatase(ALP), osteocalcin, and osteomyelin (73–75). Similarly, octanoic acid derived from a medium-chain triglyceride ketogenic diet (MCTKD) leads to lower ALP and higher levels of the bone resorption marker triphosphatase (TRAP), which can adversely affect bone (76).
BHB causes an increase in calcium inward flow, which sets off a signal cascade that promotes cell growth (Figure 2) (77). Calcium ions activate calmodulin (CaM), which regulates the nuclear factor of activated T-cells (NFAT) and calmodulin dependent kinase II (CaMKII) pathways and promotes osteoblast differentiation (78). The likely mechanism is that BHB boosts the output of adenosine 5′-triphosphatase produced by mitochondria, which causes the cell membrane to depolarize, voltage-gated calcium channels to open, and potassium channels to close (77). Calcium signaling mediates important cell cycle events, such as the re-entry of quiescent cells into G1 and the start and conclusion of the M phase (79). In addition, more energy from BHB metabolism speeds up the G1 phase of macromolecular synthesis. Considering that fatty acids make up much of the cell membrane, another logical hypothesis is that BHB supplies carbon atoms for the synthesis of fatty acids. In a manner akin to how glucose stimulates the production of insulin, a number of trophic factors are released that activate pathways that speed up the advancement of G1 (77). Apart from this, previous studies have shown that BHB promotes osteoblastogenesis in vivo and in vitro, thereby ameliorating osteoporosis (80). Thus, polyhydroxyalkanoates (PHAs) containing BHB are often used for bone repair (81). Nevertheless, it seems that the conclusion is debatable. Saito et al. concluded that acetoacetate and BHB, respectively, increase and decrease osteoblast function (82), although the exact method by which ketone bodies alter osteoblast activity remains unknown.
The only cells that can resorb bone are called osteoclasts, which are derived from the large, multinucleated haematopoietic cells of the monocyte/macrophage lineage. Macrophagecolony stimulating factor (M-CSF) binds to its receptor in osteoclast precursors to control cell proliferation and survival throughout differentiation. RANKL, a member of the tumor necrosis factor (TNF) superfamily, triggers many signaling cascades through its binding to receptor activator of nuclear factor-κB (RANK), thus starting the process of osteoclast precursor development and subsequent fusing into adult osteoclasts (83). The nuclear factor of activated T-cells (NFATC1), which is the primary switch that regulates the differentiation of osteoclast precursor cells, is transcriptionally activated under microgravity, leading to osteoporosis. Phosphorylated NFATC1, the inactive form of NFATC1 in the cytoplasm, dephosphorylates under the induction of RNAKL. Dephosphorylated NFATC1 enters the nucleus, attaches itself to the promoter regions of target genes, stimulates the expression of key genes for bone resorption, and activates osteoclast pre-differentiation (84). BHB or β-hydroxybutyrate methyl ester (BHBME) hinders osteoclast differentiation by reducing the active form of NFATC1 and preventing its nuclear translocation (Figure 2) (85).
On the other hand, accumulating evidence indicates that osteoclasts are activated by high levels of reactive oxygen species (ROS) and inflammatory cytokines, including TNF-α and interleukin-β (IL-1β) (86, 87). Simultaneously, previous studies have shown that BHB has a protective role in the nervous system, reproductive system, and diabetes retinopathy by inhibiting the activation of the nod-like receptor family pyrin domain containing 3 (NLRP3) inflammasome and decreasing levels of IL-1β and reactive oxygen species (ROS) production (88, 89). Recently, it has also been found that BHB inhibits inflammation and osteoclast differentiation caused by alloy particles by inhibiting apoptosis-associated speck-like protein containing a CARD (ASC) oligomerisation, assembly, and spot formation and reducing IL-1β secretion, thereby alleviating osteolysis. Notably, the inhibitory function of BHB on inflammation caused by alloy particles does not depend on the G protein-coupled receptor 109A (GPR109A) or HDAC in macrophages, but the inhibition of osteoclast differentiation and function of BHB is achieved by inhibiting HDAC (90).
The adverse effects of the ketogenic diet on bone health have been reported by diverse investigation groups. This section summarizes studies on the findings of the ketogenic diet on bone health and possible influencing factors. Table 1 covers research on the effects of the ketogenic diet on bone health.
Several studies are related to bone abnormalities after a ketogenic diet in children and adolescents. The alterations associated with a ketogenic diet contributing to the failure of bone mineral build-up include abnormalities in altered vitamin D levels, acid loading, decreased weight, increased levels of blood BHB, and indirect or direct disruption of the GH axis (43, 91–98). In these studies, it has been mentioned that a ketogenic diet inevitably leads to growth retardation in children, but there are also studies indicating that after stopping the ketogenic diet for a period of time, children will experience significant catch-up growth (93, 94). There is poor clinical research on the potential impacts of the ketogenic diet on adult bone health, and there is not much data on the long-term risk of osteoporosis and a ketogenic diet. However, due to the close correlation between the development of osteoporosis and the accumulation of peak bone mass in adolescence, there are reasons to believe that a ketogenic diet is harmful to the development of osteoporosis.
In addition, there are several animal model studies that support evidence of bone impairment induced by a ketogenic diet (Table 1). The ketogenic diet disrupted cortical bone mass and bone microstructure in a similar way to ovariectomy (OVX) in mice, mediated by promoting osteoclast differentiation in the research of Wu et al., while Xu et al. thought that a ketogenic diet suppressed osteoblast activity by reducing weight and increasing body fat percentage, leading to reduced bone formation (99, 100). But it has been reported that metformin alleviates the cancellous bone loss caused by the ketogenic diet (101). Rats on a high-fat and low-carbohydrate diet demonstrated visceral fat accumulation, and adipocytes in bone marrow were significantly increased. The expansion of bone marrow adipose tissue (BMAT) inhibits osteoblastogenesis, exacerbating bone loss (44).
Nevertheless, some studies suggest that a ketogenic diet has no unfavorable impacts on bone health. No adverse effects on bone mass or longitudinal growth were noted in a study using MAD. Stable pH, proper serum ketones levels, and more calorie and protein consumption than a classical ketogenic diet may explain the lack of detrimental effects on bone development (102). In a separate trial utilizing MCTKD to treat children with glycogen storage disorder, it revealed enhancements in the patients' BMC and skeletal muscle mass. This may be due to MCTKD's ability to improve hyperlactatemia, which can facilitate osteoclastogenesis and impede osteoblastogenesis. Another reason may be that MCTKD can enhance lipolysis and ameliorate fat inflammation (103). A thorough evaluation found no significant bone density changes in adults receiving a ketogenic diet. Only female participants who dropped 10% of their weight had bone mechanical unloading and increased bone turnover due to energy restrictions and quick weight loss, but osteoporosis risk did not increase (91).
It is generally believed that BHB reduces bone loss and improves osteoporosis by promoting osteoblastogenesis and inhibiting osteoclast formation. Table 2 covers research on BHB's effects on bone health.
The research conducted both in vivo and in vitro supports the potential benefits of BHB in enhancing osteoblastogenesis and anti-osteoporosis in rats undergoing bilateral OVX (80). However, the specific mechanism by which BHB promotes osteoblastogenesis needs further exploration. Furthermore, some studies show that poly(3-hydroxybutyrate) composed of BHB induces ectopic bone formation after implantation into the back muscles of cats, minipigs, and rats (81, 104). Another possible reason is that BHB activates signal transduction pathways for osteoblast proliferation by triggering rapid calcium ion flow into cells (77).
Besides that, Cao et al. found that BHB suppresses osteoclast differentiation and improves osteoporosis under microgravity conditions by inhibiting NFATC1 transcription (85). Wu et al. administered 1,3-butanediol (a BHB precursor) to mice undergoing bone osteolytic surgery. It was found that the bone density of mice increased and the activity of osteoclasts was inhibited. The specific mechanism is that BHB ameliorates osteolysis by inactivating inflammasomes and inhibiting the development and function of osteoclasts (90).
However, as mentioned earlier, acetoacetate and BHB, respectively, up-regulate and down-regulate mineralization and ALP activity in osteoblasts (82). It may partly explain the bone degeneration in diabetics. The ratio of BHB to acetoacetate rises as diabetes worsens (105), and it is possible that BHB has a stronger inhibitory effect than the promotional effect of acetoacetate on bone formation. This suggests that ketone bodies may also be the cause of diabetic osteoporosis (82).
The effects of the ketogenic diet on osteoporosis remain controversial. Most studies suggest that ketogenic diets may impair bone health and increase the risk of osteoporosis by suppressing the GH-IGF-1 axis, acid load, weight loss, and lipotoxicity (Figure 1). However, other clinical studies have shown that some types of ketogenic diets do not damage bone mass. This may be because MAD does not severely restrict protein and calorie intake. In addition, medium-chain triglycerides is thought to improve bone mineral accumulation by inhibiting osteoclastogenesis and promoting osteoblastogenesis. However, the sample size of these studies was small and the follow-up period was limited. BHB improves osteoporosis by inhibiting osteoclastogenesis and promoting osteoblastogenesis through inhibition of the RANKL signaling pathway and promotion of calcium in-flow, respectively (Figure 2). However, it has also been shown that BHB may inhibit ALP activity and thus inhibit mineralization. This may be one of the causative factors of diabetic osteoporosis.
Most of the clinical studies of the effects of ketogenic diets on bone health have targeted populations such as children with refractory epilepsy, glycogen storage disorders, and other disorders and obese populations (Table 1). The majority of clinical studies have shown adverse effects of ketogenic diets on children's bones. Childhood and adolescence are critical stages for bone mass accumulation. Impaired bone mass accumulation or inadequate nutritional intake during this period may cause a decrease in peak bone mass in adulthood, which may increase the risk of osteoporosis and fractures (106). Therefore, during the application of the ketogenic diet in children, regular follow-ups should be performed to monitor growth, including annual growth rate, height, weight, bone density, growth hormone, vitamin D, and blood calcium levels. Adequate vitamin D, calcium, and protein should also be taken during treatment (107, 108). Adequate sunlight hours and physical activity are also important for bone mass accumulation. When using the ketogenic diet for weight loss in obese and overweight people, it should be ensured that the rate of weight loss is within the normal range so as not to cause an increase in bone turnover due to rapid weight loss.
The effects of ketogenic diets and BHB on osteoporosis are the result of a multifactorial overlap. Conflicting results and the lack of precise molecular and biochemical mechanisms of action provide ample opportunities for future research. Emerging avenues of research, including BHB-induced β-hydroxybutyrylation, may play a role in modulating osteoporosis. Current clinical studies have focused on adolescent subjects, and there is a lack of research in the elderly population, where the prevalence of osteoporosis is significantly higher. More targeted, longer-term, and broader clinical studies, including precursor substances that induce ketone bodies, or modified ketogenic diet formulation interventions for different populations, may help to analyze the relationship between ketone bodies and osteoporosis.
CL: Investigation, Writing – original draft. ZD: Investigation, Writing – original draft. WHe: Visualization, Writing – original draft. YH: Visualization, Writing – original draft. PY: Visualization, Writing – original draft. MH: Visualization, Writing – original draft. JL: Visualization, Writing – original draft. YX: Conceptualization, Funding acquisition, Writing – review & editing. WHu: Conceptualization, Funding acquisition, Writing – review & editing.
The author(s) declare financial support was received for the research, authorship, and/or publication of this article. This work was supported by grants from the National Natural Science Foundation of China (Nos. 82170834 and U22A20286), the Office of Science Technology and Talent Work of Luzhou (No. 2021LZXNYD-G01), and the Health Commission of Sichuan Province Medical Science and Technology.
The authors declare that the research was conducted in the absence of any commercial or financial relationships that could be construed as a potential conflict of interest.
The author(s) declare that no Gen AI was used in the creation of this manuscript.
All claims expressed in this article are solely those of the authors and do not necessarily represent those of their affiliated organizations, or those of the publisher, the editors and the reviewers. Any product that may be evaluated in this article, or claim that may be made by its manufacturer, is not guaranteed or endorsed by the publisher.
1. Armas LA, Recker RR. Pathophysiology of osteoporosis: new mechanistic insights. Endocrinol Metab Clin North Am. (2012) 41:475–86. doi: 10.1016/j.ecl.2012.04.006
2. Barron RL, Oster G, Grauer A, Crittenden DB, Weycker D. Determinants of imminent fracture risk in postmenopausal women with osteoporosis. Osteoporos Int. (2020) 31:2103–11. doi: 10.1007/s00198-020-05294-3
3. Black DM, Delmas PD, Eastell R, Reid IR, Boonen S, Cauley JA, et al. Once-yearly zoledronic acid for treatment of postmenopausal osteoporosis. N Engl J Med. (2007) 356:1809–22. doi: 10.1056/NEJMoa067312
4. Levis S, Lagari VS. The role of diet in osteoporosis prevention and management. Curr Osteoporos Rep. (2012) 10:296–302. doi: 10.1007/s11914-012-0119-y
5. Munoz-Garach A, Garcia-Fontana B, Munoz-Torres M. Nutrients and dietary patterns related to osteoporosis. Nutrients. (2020) 12:1986. doi: 10.3390/nu12071986
6. Liu C, Kuang X, Li K, Guo X, Deng Q, Li D. Effects of combined calcium and vitamin D supplementation on osteoporosis in postmenopausal women: a systematic review and meta-analysis of randomized controlled trials. Food Funct. (2020) 11:10817–27. doi: 10.1039/D0FO00787K
7. Palomeras-Vilches A, Vinals-Mayolas E, Bou-Mias C, Jorda-Castro M, Aguero-Martinez M, Busquets-Barcelo M, et al. Adherence to the Mediterranean diet and bone fracture risk in middle-aged women: a case control study. Nutrients. (2019) 11:2508. doi: 10.3390/nu11102508
8. Wheless JW. History of the ketogenic diet. Epilepsia. (2008) 49:3–5. doi: 10.1111/j.1528-1167.2008.01821.x
9. Ivan CR, Messina A, Cibelli G, Messina G, Polito R, Losavio F, et al. Italian ketogenic Mediterranean diet in overweight and obese patients with prediabetes or type 2 diabetes. Nutrients. (2022) 14:4361. doi: 10.3390/nu14204361
10. Yuan X, Wang J, Yang S, Gao M, Cao L, Li X, et al. Effect of the ketogenic diet on glycemic control, insulin resistance, and lipid metabolism in patients with T2DM: a systematic review and meta-analysis. Nutr Diabetes. (2020) 10:38. doi: 10.1038/s41387-020-00142-z
11. Jiang Z, Yin X, Wang M, Chen T, Wang Y, Gao Z, et al. Effects of ketogenic diet on neuroinflammation in neurodegenerative diseases. Aging Dis. (2022) 13:1146–65. doi: 10.14336/AD.2021.1217
12. Newman JC, Verdin E. Beta-hydroxybutyrate: a signaling metabolite. Annu Rev Nutr. (2017) 37:51–76. doi: 10.1146/annurev-nutr-071816-064916
13. Gershuni VM, Yan SL, Medici V. Nutritional ketosis for weight management and reversal of metabolic syndrome. Curr. Nutr. Rep. (2018) 7:97–106. doi: 10.1007/s13668-018-0235-0
14. Li Z, Lü J, Yu L, Situ W, Xue L, Wang H, et al. Applications and perspectives of ketone body D-beta-hydroxybutyrate in the medical fields. Sheng Wu Gong Cheng Xue Bao. (2022) 38:976–89. doi: 10.13345/j.cjb.210343
15. Dabek A, Wojtala M, Pirola L, Balcerczyk A. Modulation of cellular biochemistry, epigenetics and metabolomics by ketone bodies. Implications of the ketogenic diet in the physiology of the organism and pathological states. Nutrients. (2020) 12:788. doi: 10.3390/nu12030788
16. He Y, Cheng X, Zhou T, Li D, Peng J, Xu Y, et al. beta-Hydroxybutyrate as an epigenetic modifier: underlying mechanisms and implications. Heliyon. (2023) 9:e21098. doi: 10.1016/j.heliyon.2023.e21098
17. Xie Z, Zhang D, Chung D, Tang Z, Huang H, Dai L, et al. Metabolic regulation of gene expression by histone lysine beta-hydroxybutyrylation. Mol Cell. (2016) 62:194–206. doi: 10.1016/j.molcel.2016.03.036
18. Zhou T, Cheng X, He Y, Xie Y, Xu F, Xu Y, et al. Function and mechanism of histone beta-hydroxybutyrylation in health and disease. Front Immunol. (2022) 13:981285. doi: 10.3389/fimmu.2022.981285
19. Yang X, Li X, Yu N, Yan R, Sun Y, Tang C, et al. Proteomics and beta-hydroxybutyrylation modification characterization in the hearts of naturally senescent mice. Mol Cell Proteomics. (2023) 22:100659. doi: 10.1016/j.mcpro.2023.100659
20. Wang X, Tian GG, Cheng W, Yu X, Li X, Wu J. Metformin promotes female germline stem cell proliferation by upregulating Gata-binding protein 2 with histone β-hydroxybutyrylation. Stem Cell Res Ther. (2023) 14:144. doi: 10.1186/s13287-023-03360-1
21. Nishitani S, Fukuhara A, Shin J, Okuno Y, Otsuki M, Shimomura I. Metabolomic and microarray analyses of adipose tissue of dapagliflozin-treated mice, and effects of 3-hydroxybutyrate on induction of adiponectin in adipocytes. Sci Rep. (2018) 8:8805. doi: 10.1038/s41598-018-27181-y
22. Barry D, Ellul S, Watters L, Lee D, Haluska R, White R. The ketogenic diet in disease and development. Int J Dev Neurosci. (2018) 68:53–8. doi: 10.1016/j.ijdevneu.2018.04.005
23. Kossoff EH, Zupec-Kania BA, Auvin S, Ballaban-Gil KR, Christina Bergqvist AG, Blackford R, et al. Optimal clinical management of children receiving dietary therapies for epilepsy: updated recommendations of the International Ketogenic Diet Study Group. Epilepsia Open. (2018) 3:175–92. doi: 10.1002/epi4.12225
24. Muscogiuri G, Verde L, Frias-Toral E, Reytor-Gonzalez C, Annunziata G, Progano M, et al. Weight loss, changes in body composition and inflammatory status after a very low-energy ketogenic therapy (VLEKT): does gender matter? J Transl Med. (2024) 22:949. doi: 10.1186/s12967-024-05733-3
25. Barrea L, Caprio M, Grassi D, Cicero AFG, Bagnato C, Paolini B, et al. A new nomenclature for the very low-calorie ketogenic diet (VLCKD): very low-energy ketogenic therapy (VLEKT) ketodiets and nutraceuticals expert panels: “KetoNut”, Italian Society of Nutraceuticals (SINut) and the Italian Association of Dietetics and Clinical Nutrition (ADI). Curr Nutr Rep. (2024) 13:552–6. doi: 10.1007/s13668-024-00560-w
26. Wells J, Swaminathan A, Paseka J, Hanson C. Efficacy and safety of a ketogenic diet in children and adolescents with refractory epilepsy-a review. Nutrients. (2020) 12:1809. doi: 10.3390/nu12061809
27. Bruci A, Tuccinardi D, Tozzi R, Balena A, Santucci S, Frontani R, et al. Very low-calorie ketogenic diet: a safe and effective tool for weight loss in patients with obesity and mild kidney failure. Nutrients. (2020) 12:1809. doi: 10.3390/nu12020333
28. Yang L, TeSlaa T, Ng S, Nofal M, Wang L, Lan T, et al. Ketogenic diet and chemotherapy combine to disrupt pancreatic cancer metabolism and growth. Med. (2022) 3:119–136. doi: 10.1016/j.medj.2021.12.008
29. Hagihara K, Kajimoto K, Osaga S, Nagai N, Shimosegawa E, Nakata H, et al. Promising effect of a new ketogenic diet regimen in patients with advanced cancer. Nutrients. (2020) 12:1473. doi: 10.3390/nu12051473
30. Egashira R, Matsunaga M, Miyake A, Hotta S, Nagai N, Yamaguchi C, et al. Long-term effects of a ketogenic diet for cancer. Nutrients. (2023) 15:2334. doi: 10.3390/nu15102334
31. Mohammadifard N, Haghighatdoost F, Rahimlou M, Rodrigues APS, Gaskarei MK, Okhovat P, et al. The effect of ketogenic diet on shared risk factors of cardiovascular disease and cancer. Nutrients. (2022) 14:3499. doi: 10.3390/nu14173499
32. Kong C, Yan X, Liu Y, Huang L, Zhu Y, He J, et al. Ketogenic diet alleviates colitis by reduction of colonic group 3 innate lymphoid cells through altering gut microbiome. Signal Transduct Target Ther. (2021) 6:154. doi: 10.1038/s41392-021-00549-9
33. Gubatan J, Kulkarni CV, Talamantes SM, Temby M, Fardeen T, Sinha SR. Dietary exposures and interventions in inflammatory bowel disease: current evidence and emerging concepts. Nutrients. (2023) 15:579. doi: 10.3390/nu15030579
34. Giustina A, Mazziotti G, Canalis E. Growth hormone, insulin-like growth factors, and the skeleton. Endocr Rev. (2008) 29:535–559. doi: 10.1210/er.2007-0036
35. Tritos NA. Focus on growth hormone deficiency and bone in adults. Best Pract Res Clin Endocrinol Metab. (2017) 31:49–57. doi: 10.1016/j.beem.2017.02.002
36. Yakar S, Werner H, Rosen CJ. Insulin-like growth factors: actions on the skeleton. J Mol Endocrinol. (2018) 61:T115–37. doi: 10.1530/JME-17-0298
37. Huang W, Yang S, Shao J, Li YP. Signaling and transcriptional regulation in osteoblast commitment and differentiation. Front Biosci. (2007) 12:3068–92. doi: 10.2741/2296
38. Vlashi R, Zhang X, Wu M, Chen G. Wnt signaling: essential roles in osteoblast differentiation, bone metabolism and therapeutic implications for bone and skeletal disorders. Genes Dis. (2023) 10:1291–317. doi: 10.1016/j.gendis.2022.07.011
39. Yuan Y, Duan R, Wu B, Huang W, Zhang X, Qu M, et al. Gene expression profiles and bioinformatics analysis of insulin-like growth factor-1 promotion of osteogenic differentiation. Mol Genet Genomic Med. (2019) 7:e00921. doi: 10.1002/mgg3.921
40. Ogino Y, Ayukawa Y, Kukita T, Koyano K. The contribution of platelet-derived growth factor, transforming growth factor-beta1, and insulin-like growth factor-I in platelet-rich plasma to the proliferation of osteoblast-like cells. Oral Surg Oral Med Oral Pathol Oral Radiol Endod. (2006) 101:724–9. doi: 10.1016/j.tripleo.2005.08.016
41. Zhang W, Shen X, Wan C, Zhao Q, Zhang L, Zhou Q, et al. Effects of insulin and insulin-like growth factor 1 on osteoblast proliferation and differentiation: differential signalling via Akt and ERK. Cell Biochem Funct. (2012) 30:297–302. doi: 10.1002/cbf.2801
42. LeRoith D, Holly JMP, Forbes BE. Insulin-like growth factors: Ligands, binding proteins, and receptors. Mol Metab. (2021) 52:101245. doi: 10.1016/j.molmet.2021.101245
43. Spulber G, Spulber S, Hagenas L, Amark P, Dahlin M. Growth dependence on insulin-like growth factor-1 during the ketogenic diet. Epilepsia. (2009) 50:297–303. doi: 10.1111/j.1528-1167.2008.01769.x
44. Bielohuby M, Matsuura M, Herbach N, Kienzle E, Slawik M, Hoeflich A, et al. Short-term exposure to low-carbohydrate, high-fat diets induces low bone mineral density and reduces bone formation in rats. J Bone Miner Res. (2010) 25:275–84. doi: 10.1359/jbmr.090813
45. Brungger M, Hulter HN, Krapf R. Effect of chronic metabolic acidosis on the growth hormone/IGF-1 endocrine axis: new cause of growth hormone insensitivity in humans. Kidney Int. (1997) 51:216–21. doi: 10.1038/ki.1997.26
46. Green J, Maor G. Effect of metabolic acidosis on the growth hormone/IGF-I endocrine axis in skeletal growth centers. Kidney Int. (2000) 57:2258–67. doi: 10.1046/j.1523-1755.2000.00086.x
47. Gasser JA, Hulter HN, Imboden P, Krapf R. Effect of chronic metabolic acidosis on bone density and bone architecture in vivo in rats. Am J Physiol Renal Physiol. (2014) 306:F517–24. doi: 10.1152/ajprenal.00494.2013
48. Bergqvist AG, Schall JI, Stallings VA, Zemel BS. Progressive bone mineral content loss in children with intractable epilepsy treated with the ketogenic diet. Am J Clin Nutr. (2008) 88:1678–84. doi: 10.3945/ajcn.2008.26099
49. Bushinsky DA, Smith SB, Gavrilov KL, Gavrilov LF, Li J, Levi-Setti R. Chronic acidosis-induced alteration in bone bicarbonate and phosphate. Am J Physiol Renal Physiol. (2003) 285:F532–9. doi: 10.1152/ajprenal.00128.2003
50. Lee SW, Russell J, Avioli LV. 25-hydroxycholecalciferol to 1,25-dihydroxycholecalciferol: conversion impaired by systemic metabolic acidosis. Science. (1977) 195:994–6. doi: 10.1126/science.841324
51. Asomaning K, Bertone-Johnson ER, Nasca PC, Hooven F, Pekow PS. The association between body mass index and osteoporosis in patients referred for a bone mineral density examination. J Womens Health (Larchmt). (2006) 15:1028–34. doi: 10.1089/jwh.2006.15.1028
52. Nguyen TV, Sambrook PN, Eisman JA. Bone loss, physical activity, and weight change in elderly women: the Dubbo Osteoporosis Epidemiology Study. J Bone Miner Res. (1998) 13:1458–67. doi: 10.1359/jbmr.1998.13.9.1458
53. Ravn P, Cizza G, Bjarnason NH, Thompson D, Daley M, Wasnich RD, et al. Low body mass index is an important risk factor for low bone mass and increased bone loss in early postmenopausal women. Early Postmenopausal Intervention Cohort (EPIC) study group. J Bone Miner Res. (1999) 14:1622–7. doi: 10.1359/jbmr.1999.14.9.1622
54. Iwaniec UT, Turner RT. Influence of body weight on bone mass, architecture and turnover. J Endocrinol. (2016) 230:R115–30. doi: 10.1530/JOE-16-0089
55. Turner RT, Iwaniec UT. Moderate weight gain does not influence bone metabolism in skeletally mature female rats. Bone. (2010) 47:631–5. doi: 10.1016/j.bone.2010.06.010
56. Labouesse MA, Gertz ER, Piccolo BD, Souza EC, Schuster GU, Witbracht MG, et al. Associations among endocrine, inflammatory, and bone markers, body composition and weight loss induced bone loss. Bone. (2014) 64:138–46. doi: 10.1016/j.bone.2014.03.047
57. Yu D, Chen W, Zhang J, Wei L, Qin J, Lei M, et al. Effects of weight loss on bone turnover, inflammatory cytokines, and adipokines in Chinese overweight and obese adults. J Endocrinol Invest. (2022) 45:1757–67. doi: 10.1007/s40618-022-01815-5
58. Qiao J, Wu Y, Ren Y. The impact of a high fat diet on bones: potential mechanisms. Food Funct. (2021) 12:963–75. doi: 10.1039/D0FO02664F
59. Ali D, Figeac F, Caci A, Ditzel N, Schmal C, Kerckhofs G, et al. High-fat diet-induced obesity augments the deleterious effects of estrogen deficiency on bone: Evidence from ovariectomized mice. Aging Cell. (2022) 21:e13726. doi: 10.1111/acel.13726
60. McGrath C, Little-Letsinger SE, Pagnotti GM, Sen B, Xie Z, Uzer G, et al. Diet-stimulated marrow adiposity fails to worsen early, age-related bone loss. Obesity Facts. (2024) 17:145–57. doi: 10.1159/000536159
61. Ali D, Tencerova M, Figeac F, Kassem M, Jafari A. The pathophysiology of osteoporosis in obesity and type 2 diabetes in aging women and men: the mechanisms and roles of increased bone marrow adiposity. Front Endocrinol (Lausanne). (2022) 13:981487. doi: 10.3389/fendo.2022.981487
62. Sebo ZL, Rendina-Ruedy E, Ables GP, Lindskog DM, Rodeheffer MS, Fazeli PK, et al. Bone marrow adiposity: basic and clinical implications. Endocr Rev. (2019) 40:1187–206. doi: 10.1210/er.2018-00138
63. Thommesen L, Stunes AK, Monjo M, Grosvik K, Tamburstuen MV, Kjobli E, et al. Expression and regulation of resistin in osteoblasts and osteoclasts indicate a role in bone metabolism. J Cell Biochem. (2006) 99:824–34. doi: 10.1002/jcb.20915
64. Jiang XY, Wang Q, Zhang Y, Chen Y, Wu LF. Association of high serum chemerin with bone mineral density loss and osteoporotic fracture in elderly Chinese women. Int J Womens Health. (2022) 14:107–18. doi: 10.2147/IJWH.S337985
65. Ramos-Junior ES, Leite GA, Carmo-Silva CC, Taira TM, Neves KB, Colon DF, et al. Adipokine chemerin bridges metabolic dyslipidemia and alveolar bone loss in mice. J Bone Miner Res. (2017) 32:974–84. doi: 10.1002/jbmr.3072
66. Conroy R, Girotra M, Shane E, McMahon DJ, Pavlovich KH, Leibel RL, et al. Leptin administration does not prevent the bone mineral metabolism changes induced by weight loss. Metabolism. (2011) 60:1222–6. doi: 10.1016/j.metabol.2011.02.010
67. Holloway WR, Collier FM, Aitken CJ, Myers DE, Hodge JM, Malakellis M, et al. Leptin inhibits osteoclast generation. J Bone Miner Res. (2002) 17:200–9. doi: 10.1359/jbmr.2002.17.2.200
68. Cornish J, Callon KE, Bava U, Lin C, Naot D, Hill BL, et al. Leptin directly regulates bone cell function in vitro and reduces bone fragility in vivo. J Endocrinol. (2002) 175:405–15. doi: 10.1677/joe.0.1750405
69. Williams GA, Wang Y, Callon KE, Watson M, Lin JM, Lam JB, et al. In vitro and in vivo effects of adiponectin on bone. Endocrinology. (2009) 150:3603–10. doi: 10.1210/en.2008-1639
70. Shapses SA, Sukumar D. Bone metabolism in obesity and weight loss. Annu Rev Nutr. (2012) 32:287–309. doi: 10.1146/annurev.nutr.012809.104655
71. Montalvany-Antonucci CC, Zicker MC, Ferreira AVM, Macari S, Ramos-Junior ES, Gomez RS, et al. High-fat diet disrupts bone remodeling by inducing local and systemic alterations. J Nutr Biochem. (2018) 59:93–103. doi: 10.1016/j.jnutbio.2018.06.006
72. Zhao L, Huang J, Zhang H, Wang Y, Matesic LE, Takahata M, et al. Tumor necrosis factor inhibits mesenchymal stem cell differentiation into osteoblasts via the ubiquitin E3 ligase Wwp1. Stem Cells. (2011) 29:1601–10. doi: 10.1002/stem.703
73. Yeh LC, Ford JJ, Lee JC, Adamo ML. Palmitate attenuates osteoblast differentiation of fetal rat calvarial cells. Biochem Biophys Res Commun. (2014) 450:777–81. doi: 10.1016/j.bbrc.2014.06.063
74. Annevelink CE, Sapp PA, Petersen KS, Shearer GC, Kris-Etherton PM. Diet-derived and diet-related endogenously produced palmitic acid: effects on metabolic regulation and cardiovascular disease risk. J Clin Lipidol. (2023) 17:577–86. doi: 10.1016/j.jacl.2023.07.005
75. Glenske K, Schape K, Wieck A, Failing K, Werner J, Rohnke M, et al. Effect of long term palmitate treatment on osteogenic differentiation of human mesenchymal stromal cells—Impact of albumin. Bone Rep. (2020) 13:100707. doi: 10.1016/j.bonr.2020.100707
76. Jain S, Rai R, Singh D, Vohora D. Octanoic acid a major component of widely consumed medium-chain triglyceride ketogenic diet is detrimental to bone. Sci Rep. (2021) 11:7003. doi: 10.1038/s41598-021-86468-9
77. Cheng S, Wu Q, Yang F, Xu M, Leski M, Chen GQ. Influence of DL-beta-hydroxybutyric acid on cell proliferation and calcium influx. Biomacromolecules. (2005) 6:593–7. doi: 10.1021/bm049465y
78. Zayzafoon M. Calcium/calmodulin signaling controls osteoblast growth and differentiation. J Cell Biochem. (2006) 97:56–70. doi: 10.1002/jcb.20675
79. Berridge MJ. Calcium signalling and cell proliferation. Bioessays. (1995) 17:491–500. doi: 10.1002/bies.950170605
80. Zhao Y, Zou B, Shi Z, Wu Q, Chen GQ. The effect of 3-hydroxybutyrate on the in vitro differentiation of murine osteoblast MC3T3-E1 and in vivo bone formation in ovariectomized rats. Biomaterials. (2007) 28:3063–3073. doi: 10.1016/j.biomaterials.2007.03.003
81. Daris B, Knez Z. Poly(3-hydroxybutyrate): Promising biomaterial for bone tissue engineering. Acta Pharm. (2020) 70:1–15. doi: 10.2478/acph-2020-0007
82. Saito A, Yoshimura K, Miyamoto Y, Kaneko K, Chikazu D, Yamamoto M, et al. Enhanced and suppressed mineralization by acetoacetate and beta-hydroxybutyrate in osteoblast cultures. Biochem Biophys Res Commun. (2016) 473:537–44. doi: 10.1016/j.bbrc.2016.03.109
83. Boyle WJ, Simonet WS, Lacey DL. Osteoclast differentiation and activation. Nature. (2003) 423:337–42. doi: 10.1038/nature01658
84. Kim JH, Kim N. Regulation of NFATc1 in osteoclast differentiation. J Bone Metab. (2014) 21:233–241. doi: 10.11005/jbm.2014.21.4.233
85. Cao Q, Zhang J, Liu H, Wu Q, Chen J, Chen GQ. The mechanism of anti-osteoporosis effects of 3-hydroxybutyrate and derivatives under simulated microgravity. Biomaterials. (2014) 35:8273–83. doi: 10.1016/j.biomaterials.2014.06.020
86. Zha L, He L, Liang Y, Qin H, Yu B, Chang L, et al. TNF-alpha contributes to postmenopausal osteoporosis by synergistically promoting RANKL-induced osteoclast formation. Biomed Pharmacother. (2018) 102:369–74. doi: 10.1016/j.biopha.2018.03.080
87. Agidigbi TS, Kim C. Reactive oxygen species in osteoclast differentiation and possible pharmaceutical targets of ROS-mediated osteoclast diseases. Int J Mol Sci. (2019) 20:3576. doi: 10.3390/ijms20143576
88. Wang Z, Li T, Du M, Zhang L, Xu L, Song H, et al. Beta-hydroxybutyrate improves cognitive impairment caused by chronic cerebral hypoperfusion via amelioration of neuroinflammation and blood-brain barrier damage. Brain Res Bull. (2023) 193:117–30. doi: 10.1016/j.brainresbull.2022.12.011
89. Trotta MC, Maisto R, Guida F, Boccella S, Luongo L, Balta C, et al. The activation of retinal HCA2 receptors by systemic beta-hydroxybutyrate inhibits diabetic retinal damage through reduction of endoplasmic reticulum stress and the NLRP3 inflammasome. PLoS ONE. (2019) 14:e0211005. doi: 10.1371/journal.pone.0211005
90. Wu Y, Teng Y, Zhang C, Pan Y, Zhang Q, Zhu X, et al. The ketone body beta-hydroxybutyrate alleviates CoCrMo alloy particles induced osteolysis by regulating NLRP3 inflammasome and osteoclast differentiation. J Nanobiotechnol. (2022) 20:120. doi: 10.1186/s12951-022-01320-0
91. Garofalo V, Barbagallo F, Cannarella R, Calogero AE, La Vignera S, Condorelli RA. Effects of the ketogenic diet on bone health: a systematic review. Front Endocrinol (Lausanne). (2023) 14:1042744. doi: 10.3389/fendo.2023.1042744
92. Hahn TJ, Halstead LR, DeVivo DC. Disordered mineral metabolism produced by ketogenic diet therapy. Calcif Tissue Int. (1979) 28:17–22. doi: 10.1007/BF02441213
93. Kim JT, Kang HC, Song JE, Lee MJ, Lee YJ, Lee EJ, et al. Catch-up growth after long-term implementation and weaning from ketogenic diet in pediatric epileptic patients. Clin Nutr. (2013) 32:98–103. doi: 10.1016/j.clnu.2012.05.019
94. Armeno M, Verini A, Del Pino M, Araujo MB, Mestre G, Reyes G, et al. A prospective study on changes in nutritional status and growth following two years of ketogenic diet (KD) therapy in children with refractory epilepsy. Nutrients. (2019) 11:1596. doi: 10.3390/nu11071596
95. Ferraris C, Guglielmetti M, Pasca L, De Giorgis V, Ferraro OE, Brambilla I, et al. Impact of the ketogenic diet on linear growth in children: a single-center retrospective analysis of 34 cases. Nutrients. (2019) 11:1442. doi: 10.3390/nu11071442
96. Neal EG, Chaffe HM, Edwards N, Lawson MS, Schwartz RH, Cross JH. Growth of children on classical and medium-chain triglyceride ketogenic diets. Pediatrics. (2008) 122:e334–40. doi: 10.1542/peds.2007-2410
97. Groleau V, Schall JI, Stallings VA, Bergqvist CA. Long-term impact of the ketogenic diet on growth and resting energy expenditure in children with intractable epilepsy. Dev Med Child Neurol. (2014) 56:898–904. doi: 10.1111/dmcn.12462
98. Simm PJ, Bicknell-Royle J, Lawrie J, Nation J, Draffin K, Stewart KG, et al. The effect of the ketogenic diet on the developing skeleton. Epilepsy Res. (2017) 136:62–6. doi: 10.1016/j.eplepsyres.2017.07.014
99. Wu X, Huang Z, Wang X, Fu Z, Liu J, Huang Z, et al. Ketogenic diet compromises both cancellous and cortical bone mass in mice. Calcif Tissue Int. (2017) 101:412–21. doi: 10.1007/s00223-017-0292-1
100. Xu X, Ding J, Wu X, Huang Z, Kong G, Liu Q, et al. Bone microstructure and metabolism changes under the combined intervention of ketogenic diet with intermittent fasting: an in vivo study of rats. Exp Anim. (2019) 68:371–80. doi: 10.1538/expanim.18-0084
101. Liu Q, Xu X, Yang Z, Liu Y, Wu X, Huang Z, et al. Metformin alleviates the bone loss induced by ketogenic diet: an In Vivo study in mice. Calcif Tissue Int. (2019) 104:59–69. doi: 10.1007/s00223-018-0468-3
102. Svedlund A, Hallbook T, Magnusson P, Dahlgren J, Swolin-Eide D. Prospective study of growth and bone mass in Swedish children treated with the modified Atkins diet. Eur J Paediatr Neurol. (2019) 23:629–38. doi: 10.1016/j.ejpn.2019.04.001
103. Subih HS, Qudah RA, Janakat S, Rimawi H, Elsahoryi NA, Alyahya L. Medium-chain triglyceride oil and dietary intervention improved body composition and metabolic parameters in children with glycogen storage disease type 1 in Jordan: a clinical trial. Foods. (2024) 13:1091. doi: 10.3390/foods13071091
104. Gredes T, Gedrange T, Hinuber C, Gelinsky M, Kunert-Keil C. Histological and molecular-biological analyses of poly(3-hydroxybutyrate) (PHB) patches for enhancement of bone regeneration. Ann Anat. (2015) 199:36–42. doi: 10.1016/j.aanat.2014.04.003
105. Stephens JM, Sulway MJ, Watkins PJ. Relationship of blood acetoacetate and 3-hydroxybutyrate in diabetes. Diabetes. (1971) 20:485–9. doi: 10.2337/diab.20.7.485
106. Caroli A, Poli A, Ricotta D, Banfi G, Cocchi D. Invited review: dairy intake and bone health: a viewpoint from the state of the art. J Dairy Sci. (2011) 94:5249–62. doi: 10.3168/jds.2011-4578
107. Rizzoli R. Dairy products, yogurts, and bone health. Am J Clin Nutr. (2014) 99:1256S–62S. doi: 10.3945/ajcn.113.073056
Keywords: ketogenic diet, β-hydroxybutyrate, osteoporosis, osteoblast, osteoclast
Citation: Luo C, Dai Z, He W, He Y, Yang P, Huang M, Li J, Xu Y and Huang W (2025) Ketogenic diet and β-hydroxybutyrate in osteoporosis: current progress and controversy. Front. Nutr. 12:1508695. doi: 10.3389/fnut.2025.1508695
Received: 09 October 2024; Accepted: 06 January 2025;
Published: 23 January 2025.
Edited by:
Peter J. Voshol, Independent Researcher, Culemborg, NetherlandsReviewed by:
Matteo Della Porta, University of Milan, ItalyCopyright © 2025 Luo, Dai, He, He, Yang, Huang, Li, Xu and Huang. This is an open-access article distributed under the terms of the Creative Commons Attribution License (CC BY). The use, distribution or reproduction in other forums is permitted, provided the original author(s) and the copyright owner(s) are credited and that the original publication in this journal is cited, in accordance with accepted academic practice. No use, distribution or reproduction is permitted which does not comply with these terms.
*Correspondence: Yong Xu, eHl3eWxsQHN3bXUuZWR1LmNu; Wei Huang, aHVhbmd3ZWkxMjEyNTIwQDE2My5jb20=
†These authors have contributed equally to this work
Disclaimer: All claims expressed in this article are solely those of the authors and do not necessarily represent those of their affiliated organizations, or those of the publisher, the editors and the reviewers. Any product that may be evaluated in this article or claim that may be made by its manufacturer is not guaranteed or endorsed by the publisher.
Research integrity at Frontiers
Learn more about the work of our research integrity team to safeguard the quality of each article we publish.