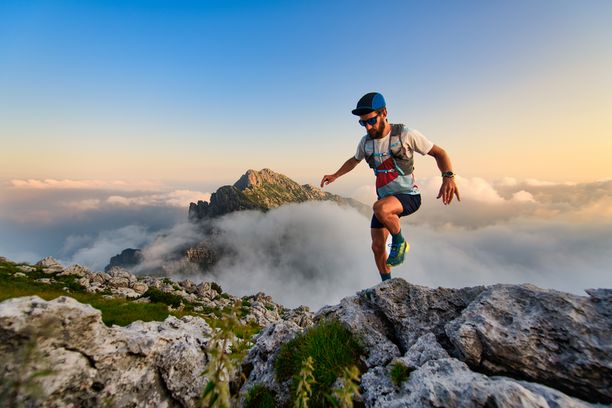
95% of researchers rate our articles as excellent or good
Learn more about the work of our research integrity team to safeguard the quality of each article we publish.
Find out more
REVIEW article
Front. Nutr., 24 March 2025
Sec. Nutrition and Metabolism
Volume 12 - 2025 | https://doi.org/10.3389/fnut.2025.1484827
Numerous animal and clinical studies have demonstrated that the arcuate nucleus of the hypothalamus, a central regulator of appetite, plays a significant role in modulating feeding behavior. However, current research primarily focuses on long-term dietary changes and their effects on the body, with limited investigation into neuroendocrine dynamics during individual meals across diverse populations. In contrast to long-term dietary adjustments, directives for dietary behavior during a specific meal are more actionable, potentially enhancing patient adherence and achieving better outcomes in dietary behavior interventions. This review aimed to explore the neural pathways and endocrine changes activated by gastrointestinal expansion and variations in blood nutrient levels during a single meal, with the goal of informing dietary behavior guidance.
The driving forces of eating can be attributed to two distinct factors: (1) the sensation of hunger caused by gastrointestinal peristalsis and (2) signals from the brain that generate the desire to eat. The hypothalamic arcuate nucleus (ARC), located adjacent to the third ventricle, is widely recognized as a central regulator of appetite. This region features a specialized and more permeable blood–brain barrier compared to other brain regions (1). The increased permeability allows circulating nutrients and hormones—such as glucose, leptin, and insulin (3)—to directly access agouti-related protein (AgRP) and pro-opiomelanocortin (POMC) neurons within the ARC. This direct access makes these neurons highly responsive to nutrient fluctuations, facilitating the regulation of energy homeostasis. Various appetite-regulating hormones including insulin, leptin, and peptide YY (PYY), as well as small-molecule nutrients such as amino acids and glucose, can directly stimulate these neurons and play a crucial role in appetite regulation.
Eating habits have been shown to affect energy metabolism and contribute to the development of metabolic diseases. Specifically, faster eating (4), higher cooking temperatures (5), fewer chews (6), and late eating (7) have been associated with increased food intake and increased risk of obesity, diabetes, and hypertension. A high-fat diet (HFD) can remodel brain neurons (8), alter the dominant species of intestinal flora (9), increase body fat deposition, and contribute to metabolic disorders (10). Conversely, intermittent fasting improves insulin sensitivity, reduces blood triglyceride and cholesterol levels, alleviates chronic inflammation (11), and helps regulate metabolic disorders (12).
To date, few studies have focused on the effects of feeding during a specific meal or conducted a comprehensive analysis of neurological and humoral changes. Compared to existing dietary guidelines, behavior guidance for a single meal is more specific and practical, aiding patients in self-control and implementation. In this review, we explore the factors that influence appetite, focusing on the feeding process during a complete eating cycle. We analyze the immediate neurological and humoral changes observed in animals. Due to limitations in current research, this article predominantly focuses on experimental mice, while findings from mouse studies may offer insights applicable to human research. We also discuss how these changes impact appetite during this process. This review provides insights for dietary behavior guidance.
For a relatively balanced meal in terms of both quantity and quality, gastric emptying in humans typically takes 4–6 h. Feelings of hunger typically emerge around this time, marking the starting point of the feeding process addressed in this review. The orexigenic effect of AgRP depends on the release of neuropeptide Y (NPY) and gamma-aminobutyric acid (GABA) (2, 13). Mouse experiments have demonstrated that mice deficient in NPY or GABA transporters do not rapidly increase food intake when AgRP neurons are activated. This finding indicates that NPY and GABA mediate the rapid, short-term effects of AgRP on feeding behavior (2, 14). In contrast, the orexigenic effect induced by AgRP itself is slower and more prolonged (2). In addition to stimulating appetite, activating AgRP decreases energy expenditure, enhances carbohydrate utilization, and reduces fat breakdown (2, 15). Under conditions of extreme hunger, the AgRP→parabrachial nucleus (PBN) pathway suppresses pain responses, allowing animals to prioritize foraging for food (16). Mouse experiments have demonstrated that activating AgRP/NPY neurons increases their willingness to take greater risks when seeking food (17, 18).
Upon food discovery, visual (19) and olfactory (20) signals transmitted into the brain activate different brain areas; AgRP secretion rapidly decreases, while POMC neurons, which antagonize AgRP, are rapidly activated. Simultaneously, saliva, digestive enzymes, insulin, and other substances are secreted to prepare food intake (21). Vagal receptors in the oropharyngeal area detect changes in taste and tension, activating the vagus nerve to initiate the vagovagal reflex of the stomach and facilitate the smooth entry of food into the stomach. Vagal receptors distributed throughout the gastrointestinal tract sense changes in tension and nutrient levels within the digestive system. These stimuli are transmitted to the brain, inducing satiety and activating secretory cells in the intestine to release appetite-suppressing hormones, such as PYY and serotonin, which stimulate the hypothalamus to create a feeling of fullness (22). Through the coordinated actions of multiple factors, the eating event concludes as food is gradually digested into chyme in the stomach. As nutrients are absorbed by intestinal epithelial cells, blood glucose levels rise, providing the energy required for growth and activity. Over time, these nutrients—primarily glucose—are utilized, leading to decline in blood glucose levels, reduction in insulin secretion, and gradual increase in AgRP/NPY neuron activity, eventually triggering the next sensation of hunger. The trend of changes in the main neurohumoral regulation during eating is illustrated in Figure 1.
Figure 1. Schematic diagram of neurohumoral changes over time. The vertical axis represents the percentage of normalized changes in neural activity and hormone levels (109–114), 100% consider it as complete activation of nerves/peak release of hormones in the body, 0% consider it as complete inhibition of nerves/hormone content in the body measured as 0. The horizontal axis indicates meal time. The timeline assumes a state of hunger at −60 min, food discovery at 20 min, and the initiation of meals at 0 min. Gastric emptying concludes at 360 min, at which point the system returns to a hunger state. It should be noted that this is only a schematic diagram and does not represent, for example, an equal release of GLP-1 and insulin during the eating process.
During the period between detection of food signals and swallowing, the body undergoes a series of anticipatory responses, collectively referred to as cephalic phase responses. These responses include the secretion of saliva, digestive enzymes, and insulin (23); they ensure rapid digestion, efficient food absorption, and effective nutrient metabolism (24). Recently, cephalic phase responses have gained increasing attention. Studies on rodents and humans have shown that, when transmitted to corresponding areas of the cerebral cortex, sensory food stimuli, such as visual (25), taste (26), and olfactory cues (27), have corresponding effects on subsequent eating behavior through different pathways, some of which are associated with the reward system.
The nucleus tractus solitarius (NTS) integrates these signals and relays them to the dorsal motor nucleus of the vagus nerve (DMV), initiating vagal nerve activity and mediating the early secretion of insulin (28, 29). Although this hypothesis remains unconfirmed, it is well established that cephalic phase insulin release positively correlates with the amount of food ingested during a meal. The cephalic phase insulin release also counteracts hepatic glucose production mediated by glucagon, helping to maintain relatively stable blood glucose levels (23).
POMC neurons also influence the liver’s regulation of early-phase blood glucose after a meal. Upon sensing food cues, POMC neurons are rapidly activated and regulate the sympathetic nervous system of the liver in an mTOR-dependent manner, thereby controlling hepatic glycogenolysis (30). Additionally, AgRP neurons are rapidly and uniformly inhibited when detecting food cues, with the level of inhibition positively correlated with palatability and expected energy content of the food (31) (Figure 2). This inhibitory response is mediated by GABAergic neurons expressing leptin receptors in the dorsomedial hypothalamus (DMH) (24), and its duration is proportional to energy expenditure (31).
Figure 2. Neurohumoral pathways mediating feeding cessation during eating. Upon sensing food signals, the body can immediately suppress AgRP neurons and activate POMC neurons, preparing for food intake. Ghrelin, secreted by gastric wall cells during hunger, is currently the only known gastrointestinal hormone with appetite-stimulating effects. It can directly stimulate specific brain nuclei through the bloodstream or exert indirect effects via vagal sensory receptors (orange arrows).After eating, gastrointestinal hormones such as PYY and CCK inhibit appetite by directly stimulating relevant brain nuclei through the humoral circulation (orange arrows).Gastrointestinal hormones released after eating, along with mechanical stimuli from gastrointestinal distension, are sensed by different vagal sensory receptors. These stimuli are transmitted to the corresponding brain nuclei, generating a sense of satiety (satiety) and controlling gastrointestinal motility to create a feeling of fullness (satiation) (black arrows). The parabrachial nucleus (PBN), upon receiving these stimuli, activates the central amygdala (CeA) in the cerebral cortex, mediating the sensation of satiety. Activated POMC, NTS, PVN, and AP neurons can stimulate the sympathetic nervous system, leading to systemic responses such as increased heart rate, elevated blood pressure, enhanced hepatic gluconeogenesis, accelerated fat metabolism, and increased insulin secretion.
Although both types of neurons respond rapidly to food cues, they do not directly mediate the initiation or termination of eating behaviors. The dorsal vagal complex, located in the brainstem, receives input signals from the hypothalamus and integrates them with signals from the gastrointestinal tract via the vagus nerve, playing a key role in mediating eating behavior (32).
Food enters the stomach after chewing and swallowing, mixes with gastric juice, breaks down into chyme and then passes into the intestine for absorption. This process involves complex neurohumoral changes, primarily mediated by the sensory branches of the vagus nerve distributed throughout the stomach and intestinal walls. These changes stimulate vagal receptors in the oropharyngeal region, which transmit feeding signals to the stomach via the vagovagal reflex, initiating gastric accommodation. Gastric accommodation maintains the relative stability of intragastric pressure (IGP), a factor significantly correlated with satiety. A brief decrease in IGP is observed at the onset of eating (33). As food accumulates in the stomach, the muscles of the gastric cardia, fundus, and body gradually become tense, increasing IGP. The tension receptors widely distributed in the gastric walls are stimulated, transmitting signals via the nodose ganglion (NG) to the NTS, which mediates the sensation of fullness (34). The rate of gastric emptying during eating is higher than usual, allowing some incompletely digested chyme to enter the intestine, where it stimulates tension and chemoreceptors in the duodenal wall (32). Additionally, tension receptors in the gastrointestinal wall may be involved in regulating insulin secretion during eating (35).
Several gastrointestinal hormones, including ghrelin, glucagon-like peptide-1 (GLP-1), PYY, serotonin, and cholecystokinin (CCK), are involved in appetite regulation. Ghrelin, secreted by P/D1 cells in the gastric fundus, increases during fasting and rapidly decreases after eating (31), promoting feeding by stimulating AgRP neurons. Enteroendocrine cells in the duodenum detect changes in intestinal tension and nutrient levels, releasing hormones such as serotonin and CCK. These hormones activate vagal afferents or enter the bloodstream, mediating the sensation of satiety (32).
As the amount of food entering the stomach increases, the tension in the gastrointestinal wall also rises, causing a gradual increase in IGP and the onset of satiation. Vagal afferent nerves (VANs) detect this increased tension and transmit the signals to the NTS. Beyond its role in regulating gastrointestinal motility via the dorsal motor nucleus of the vagus (36), NTS Calcr neurons mediate the cessation of feeding by suppressing AgRP activity and activating non-calcitonin gene-related peptide (CGRP) neurons in the PBN (37). Additionally, satiety hormones stimulate neurons such as POMC to create a sense of fullness (38). Aversive signals, discomfort (39), and other negative feedback mechanisms also contribute to the cessation of feeding. These mechanisms are primarily mediated by the area postrema (AP) and PBN (see Section 3.2 for details). The detailed process of cessation of eating behavior is shown in Figure 2.
The vagus nerve, also known as the tenth cranial nerve, originates in the medulla oblongata of the brainstem. It consists of four types of fibers—somatic sensory, somatic motor, visceral sensory, and visceral motor— and extensively innervates the gastrointestinal tract. The vagus nerve detects changes in gastrointestinal tension and hormone levels and transmits these signals to the NTS. Subsequently, impulses are generated to modulate the feeding process.
Different subtypes of VANs are distributed across various organs and are specialized to perceive distinct stimuli. Five subtypes are predominant in the gastrointestinal tract: calcitonin gene-related peptide 1 (Calca), GLP-1 receptor (GLP-1R), G protein-coupled receptor 65 (Gpr65), vasoactive intestinal peptide (Vip), and oxytocin receptor (Oxtr) (24).
The termination of feeding behavior in mice is triggered primarily by gastric distension rather than an increase in internal nutrients (40), indicating that mechanosensory fibers play a dominant role in this process. VANGLP-1R+ fibers are distributed in the gastric wall, and VANOxtr+ fibers are found in the intestinal wall. Both serve as mechanosensory fibers and possess intraganglionic laminar endings (22, 41–43). Acute chemical and optogenetic stimulation of these two fiber types in mice induces the cessation of feeding behavior (24, 41, 42) and suppresses the activity of AgRP neurons. Notably, stimulation of VANGLP-1R+ fibers results in strong but transient inhibition, while stimulation of VANOxtr+ fibers causes prolonged suppression of AgRP neurons and reduces their responsiveness to food cues (42). Additionally, the activation of VANGLP-1R+ fibers enhances glucose uptake by skeletal muscles and improves glucose tolerance (41).
Intraperitoneal administration of CCK and GLP-1, which are released by intestinal endocrine cells (44) into the mouse intestine, reduces food intake. Forced inhibition of VANGLP-1R+ fibers alleviates the feeding reduction mediated by CCK but does not affect appetite suppression induced by GLP-1R agonists, such as liraglutide. Furthermore, CCK receptors have been detected on VANGLP-1R+ fibers (41), suggesting that CCK, rather than GLP-1, is the hormone that directly activates these fibers.
In the gastric mucosa, VANCalca+, and at the tips of intestinal villi, VANGpr65+ and VANVip+ fibers primarily sense changes in gastrointestinal hormones and nutrients, thereby regulating glucose metabolism (22, 41). Activation of VANGpr65+ fibers increases the expression of phosphoenolpyruvate carboxykinase, a key enzyme in gluconeogenesis, enhancing hepatic gluconeogenesis and elevating blood glucose levels (41, 45). However, its effects on appetite regulation have not yet been determined (24).
The cell bodies of the sensory neurons of the vagus nerve are located in two nodose ganglia near the base of the skull, close to the carotid artery (43). These ganglia serve as crucial sites for mediating satiety signals. Their central axons project to the dorsal hindbrain, activating neurons in the NTS. The NTS processes mechanical signals from the gastrointestinal tract and chemical signals, such as CCK, GLP-1, PYY, and serotonin, which are secreted by enteroendocrine cells (43, 46, 47). Using Ca2+ flux measurements, Williams et al. (22) demonstrated that different subtypes of neurons in the NG can sense gastrointestinal tension and hormonal signals independently. NGGLP-1R+ cells can sense tension signals from the stomach and parts of the intestine, transmit these signals to the NTS and AP, and subsequently inhibit the activation of AgRP neurons (42). Neurons sensing hormonal signals express Gpr65, which, similar to NGGLP-1R+, activates the NTS and AP and modulates IGP (42). A study by Han et al. (48) demonstrated that the activation of the right vagus nerve (NG) in mice induces sustained self-stimulation behavior, similar to the behaviors observed when cortical reward centers are activated, and promotes dopamine release in the substantia nigra. In contrast, the activation of the left NG did not elicit similar responses. This suggests that the activation of the gastrointestinal vagus nerve may indirectly regulate feeding behavior by stimulating cortical reward centers (48).
Signals from the intestine are transmitted via the NG to the dorsal vagal complex in the brainstem, which includes the NTS, AP, and DMV. In the dorsal vagal complex, these signals are integrated and transmitted to the hypothalamus, PBN, and other regions to regulate appetite while controlling gastrointestinal motility through the DMV (49, 50). The AP, located outside the blood–brain barrier, primarily mediates aversive signals and receives a small portion of stimuli from VANs (34). Hormones involved in satiety, such as CCK and PYY, can activate the AP (41, 51–53) and subsequently the NTS. The NTS plays a critical regulatory role in food intake and energy metabolism by processing mechanical and chemical signals from the gastrointestinal tract. Cheng et al. (37) reported that the activation of the NTS suppresses the expression of AgRP/NPY neurons in the ARC, thereby inhibiting feeding. Additionally, the NTS mediates the cessation of feeding by activating downstream regions, including the PBN, paraventricular nucleus of the hypothalamus, and DMH (37). The PBN, a small cluster of nuclei located at the midbrain-pons junction, acts as a relay center for visceral and gustatory signals as well as somatosensory information, including pain, temperature, and itch. The PBN receives inhibitory inputs from AgRP neurons (54) and excitatory signals from the NTS (55), allowing it to regulate discomfort and energy metabolism. By activating the central nucleus of the amygdala, the PBN suppresses appetite (56). Activation of Calcr-expressing neurons in the NTS (NTSCalcr neurons) transmits signals to the PBN, specifically activating PBN non-CGRP neurons, leading to feeding suppression.
Furthermore, Cheng et al. (37) demonstrated that silencing NTSCalcr neurons in mice resulted in chronic increases in food intake and subsequent weight gain (37). Additionally, the activation of NTS CCK neurons triggers CGRP neurons in the PBN. When NTS CCK neurons detect toxic substances in the blood (e.g., LiCl), the discomfort signals they mediate can outweigh hunger signals driven by AgRP neurons. In such cases, PBN CGRP neurons relay these aversive signals to the central amygdala, resulting in appetite suppression (53).
Short-term acute HFD intake activates the reward system on the edge of the middle brain, and the dopamine energy neurons participating in the reward system can be projected into the regulation of dietary behavior (57). However, impairment of satiety signals induced by long-term HFD is widely recognized as a primary contributor to obesity. HFD not only reduces the sensitivity of VANGLP-1R/CCK neurons (58) but also affects the expression of GLP-1R and CCK in neurons (59). This reduction subsequently decreases the expression of POMC and cocaine- and amphetamine-regulated transcript (60), thereby weakening satiety signals. CCK receptors on VANCCK neurons are coexpressed with leptin receptors (61). Damage to VANCCK can lead to reduced leptin receptor expression, resulting in leptin resistance and increased appetite (62). The gut microbiota mediates CCK gene expression by producing short-chain fatty acids (63), which can directly activate VANs (64). Disruption of the gut microbiota by HFD reduces the abundance of short-chain fatty acid-producing bacteria, such as lactobacilli, and decreases the diversity of short-chain fatty acids. This disruption may lead to increased microglial activity in the NTS, generating neuroinflammation, promoting synaptic remodeling, and impairing satiety signal transmission (58). Rodents with obesity induced by HFD exhibit reduced activation of postprandial brainstem neurons (65), specifically a decrease in NTS neuron activation (66).
In summary, HFD detrimentally affects multiple processes involved in satiety signal generation and transmission mediated by the vagus nerve. These effects are primarily driven by changes in CCK, leptin, and gut microbiota. Further research is needed to determine if acute HFD intake affects dietary behavior through other mechanisms.
Although the sympathetic nervous system is generally believed to be inhibited during feeding behavior, recent studies suggest that sympathetic excitation also plays a role in both the initiation and cessation of feeding behavior. Evidence indicates that sympathetic excitation occurs before the cessation of feeding (67). Neuropeptides within the melanocortin system involved in the regulation of satiety, such as adrenocorticotropic hormone, α-melanocyte-stimulating hormone (MSH), β-MSH, and γ-MSH, exhibit varying excitatory effects on the sympathetic nervous system. Conversely, neurons expressing AgRP/NPY, which project to the paraventricular nucleus (PVN) and DMH, suppress sympathetic activity by releasing NPY (68). Hormones involved in dietary metabolism, such as leptin and insulin, regulate sympathetic nerve activity either directly or indirectly.
As previously mentioned, the gradual increase in gastrointestinal hormones such as insulin, PYY, and CCK, as well as increasing gastrointestinal tension during a meal, is transmitted to the NTS. This activation stimulates POMC neurons in the ARC, generating a sensation of satiety and mediating the cessation of feeding. POMC neurons release various neuropeptides, including adrenocorticotropic hormone, α-MSH, β-MSH, γ-MSH, and β-endorphin, which bind to different melanocortin receptor subtypes (MC1R-MC5R) to produce distinct effects. Among these, the α-MSH neuropeptide binds to MC4R and plays a central role in regulating appetite, energy balance, and sympathetic nervous system activity (69). The activation of MC4R-expressing sympathetic preganglionic neurons in the DMV, NTS, and intermediolateral cell column has been shown to restore sympathetic activity in mice and enhance the thermogenic response mediated by MC4R (70). Mice with MC4R deficiency exhibit significantly reduced sympathetic activity compared to wild-type mice when consuming an HFD. Rahmouni et al. revealed that the MC4R pathway is essential for the modulation of insulin and leptin effects on renal sympathetic nerve activity (71). Hypertension typically associated with obesity through sympathetic activation is not observed in diet-induced obese rats with MC4R deficiency (72). Additionally, studies have shown that MC4R-deficient mice lose the ability to convert white adipose tissue into brown adipose tissue in response to cold exposure, resulting in significantly reduced thermogenic capacity (73). These findings indicate that MC4R enhances sympathetic nervous system activity to increase energy expenditure, thereby maintaining metabolic stability.
In addition, the role of MC4R in regulating blood pressure is significant and should not be overlooked. Intracerebroventricular injection of α-MSH in awake rats increased central venous pressure and heart rate within 1 h, and they returned to baseline levels after 2 h, which may be associated with sympathetic activation (74). However, the effect of α-MSH on blood pressure was completely abolished in animals with MC4R knockout (75). Direct injection of the MC4R agonist melanotan II into the PVN increases renal sympathetic excitability and central venous pressure. However, this effect is not observed in PVN pretreated with MC4R antagonists, such as AgRP (76).
NPY can inhibit KCl-activated POMC neurons in a dose-dependent manner, thereby reducing sympathetic nervous system activity (77). Furthermore, the inhibitory effects of NPY on the sympathetic nervous system are primarily observed in the PVN. The suppression of brown adipose tissue thermogenesis (78) and the promotion of white adipose tissue and liver lipogenesis (79, 80) by NPY may be linked to its suppression of sympathetic nervous system activity. Additionally, the reduction in vascular smooth muscle sympathetic nerve activity induced by NPY facilitates increased blood flow to the gastrointestinal tract and liver, thereby enhancing digestion and absorption (81). NPY receptors are also present in the DMH, where NPY can inhibit sympathetic nerve activity, leading to a reduction in mean arterial pressure and heart rate (37, 82–84). Notably, blocking NPY1 receptors (NPY1Rs) in both the DMH and PVN resulted in differential effects: blocking NPY1Rs in the DMH completely reversed the inhibitory effect of AgRP/NPY neurons on sympathetic nerve activity, whereas blocking NPY1Rs in the PVN did not. Activation of the PVN and DMH elicited comparable effects on the sympathetic nerve activity, mean arterial pressure, and heart rate. The DMH is known to innervate and stimulate the PVN, which likely accounts for the observed similarity in responses (83).
Preliminary experiments have demonstrated the anticipatory nature of AgRP/NPY neurons, whose activity rapidly declines prior to feeding. The extent of this decline correlates with the anticipated energy density of the food to be consumed (37). The rapid reduction in NPY levels increases inhibition of the sympathetic nervous system, potentially contributing to the increase in heart rate and blood pressure during feeding. Additionally, the increase in gastrointestinal blood flow activates baroreceptors, leading to vasodilation to maintain stable blood flow and facilitate the post-feeding absorption process.
Leptin- and insulin-induced activation of the sympathetic nervous system is closely associated with maintaining homeostasis. Leptin receptors, which are widely distributed throughout the hypothalamus, respond to leptin stimulation and converge signals in the PVN. These signals subsequently activate the rostral ventrolateral medulla, which excites sympathetic preganglionic neurons in the spinal cord (85, 86).
Studies have shown that the effect of leptin on the sympathetic nervous system is sex-dependent. In female rats, the sympathetic nervous system’s response to leptin varies cyclically with fluctuations in estrogen levels (87). Unlike in male rats, leptin-induced excitation of the sympathetic nervous system in female rats does not increase blood pressure (87, 88). Additionally, female rats with higher estrogen levels are reportedly more sensitive to the anorectic effects of leptin and less sensitive to those of insulin (89). The differential effects of leptin between sexes are not attributed to differences in sites or pathways of action but rather to a positive interaction between leptin and estrogen at the cellular level (90, 91).
Unlike leptin, the effect of insulin on sympathetic activation is not sex-dependent. In rats, insulin primarily increases the sympathetic excitability of peripheral vessels, with the most pronounced effect on spinal sympathetic neurons. Additionally, insulin enhances the excitability of human muscle sympathetic nerves and increases the muscle vascular baroreceptor reflex (92). A meta-analysis by Grassi et al. (93), involving 314 individuals with diabetes and healthy controls across 11 studies, confirmed that patients with type 2 diabetes exhibit higher sympathetic excitability than healthy individuals and those with type 1 diabetes. Patients with type 2 diabetes showed significantly elevated muscle sympathetic nerve activity, which was associated with plasma insulin levels, corroborating the excitatory effect of insulin on the sympathetic nervous system.
Postprandial activation of the sympathetic nervous system has been observed, with different dietary components influencing its activation to varying degrees. High carbohydrate intake is associated with greater sympathetic activation (94). However, the statistical significance of these changes remains unclear (95). Postprandial insulin secretion promotes peripheral vasodilation, enhancing nutrient absorption. This is followed by a decrease in venous return, which stimulates the sympathetic nervous system. In some older individuals, postprandial hypotension may occur due to reduced sympathetic activity (96). Reducing dietary carbohydrate intake may help alleviate postprandial hypotension in these cases.
Obesity is believed to increase sympathetic activity. Research suggests that obesity decreases NPY content or mRNA levels in the ARC and PVN (97–99). This reduction enhances sympathetic activation during eating, which may explain why obese individuals tend to eat faster than others. HFD has been shown to reduce NPY1R expression in the DMH in males but not in females (100, 101). Reduced NPY release or receptor levels may contribute to the increased sympathetic activity associated with obesity (81). Sakamoto et al. demonstrated that overnutrition leads to excessive activation of the sympathetic nervous system, resulting in insulin resistance and metabolic disorders, ultimately contributing to obesity.
Reducing catecholamine release from the sympathetic nervous system has been shown to protect the body from the adverse effects of overnutrition-induced insulin resistance, hyperinsulinemia, hyperglucagonemia, adipose tissue dysfunction, and fatty liver disease (102).
Numerous studies have investigated the mechanisms underlying appetite generation and satiation, the effects of diet on appetite, and the role of dietary modifications in controlling appetite. Due to space limitations, certain factors regulating appetite, such as sleep (103–105), sex (106), alcohol (107), and stress (108), were not thoroughly reviewed in this paper. Most of these studies have primarily focused on the nutritional components of the diet and the long-term effects of dietary control, with relatively few examining specific behaviors associated with a single meal. The advent of optogenetics has enabled precise stimulation and observation of neurons, resulting in increased research on the neural pathways that regulate dietary behavior. This review summarizes existing gut-brain neural pathways and provides a comprehensive description of neurohumoral changes throughout the entire eating process, from the onset of hunger, discovery of food, and initiation of eating to the gradual feeling of satiety, cessation of eating, and completion of gastric emptying. It also analyzes changes in appetite during this process, aiming to inspire future research on dietary regulation. It is anticipated that future dietary guidance will place greater emphasis on specific meal processes to enhance patient compliance. Future studies should prioritize investigating how factors such as eating speed and nutritional content influence satiety from a meal-specific perspective. Additionally, personalized assessments of neural and endocrine alterations during the eating process across different individuals are essential to provide more precise dietary recommendations and achieve the goals of behavioral eating counseling.
XS: Writing – original draft, Conceptualization, Investigation. BL: Visualization, Writing – original draft. YY: Resources, Writing – original draft. YR: Writing – review & editing, Resources. RP: Writing – original draft. QL: Funding acquisition, Writing – review & editing, Supervision.
The author(s) declare that financial support was received for the research and/or publication of this article. This work was supported by the Major Basic Research Project of Shandong Natural Science Foundation (grant number ZR2023ZD28), Shandong Provincial Hospital (Sodium Trestatil in Animal Studies on Sarcopenia, grant number 1678778400040), and Research on Organoid Construction Techniques and the Establishment of Microcirculation in Various Organoids with Shandong Yuanchen Biomedical Technology Group Co., Ltd.
The authors declare that the research was conducted in the absence of any commercial or financial relationships that could be construed as a potential conflict of interest.
All claims expressed in this article are solely those of the authors and do not necessarily represent those of their affiliated organizations, or those of the publisher, the editors and the reviewers. Any product that may be evaluated in this article, or claim that may be made by its manufacturer, is not guaranteed or endorsed by the publisher.
1. Langlet, F, Mullier, A, Bouret, SG, Prevot, V, and Dehouck, B. Tanycyte-like cells form a blood–cerebrospinal fluid barrier in the circumventricular organs of the mouse brain. J Comp Neurol. (2013) 521:3389–405. doi: 10.1002/cne.23355
2. Krashes, MJ, Shah, BP, Koda, S, and Lowell, BB. Rapid versus delayed stimulation of feeding by the endogenously released AgRP neuron mediators GABA, NPY, and AgRP. Cell Metab. (2013) 18:588–95. doi: 10.1016/j.cmet.2013.09.009
3. Bouret, SG, Draper, SJ, and Simerly, RB. Trophic action of leptin on hypothalamic neurons that regulate feeding. Science. (2004) 304:108–10. doi: 10.1126/science.1095004
4. Argyrakopoulou, G, Simati, S, Dimitriadis, G, and Kokkinos, A. How important is eating rate in the physiological response to food intake, control of body weight, and glycemia? Nutrients. (2020) 12:1734. doi: 10.3390/nu12061734
5. Lu, Y, Liu, J, Boey, J, Hao, R, Cheng, G, Hou, W, et al. Associations between eating speed and food temperature and type 2 diabetes mellitus: a cross-sectional study. Front Nutr. (2023) 10:1205780. doi: 10.3389/fnut.2023.1205780
6. Slyper, A. Oral processing, satiation and obesity: overview and hypotheses. Diabetes Metab Syndr Obes. (2021) 14:3399–415. doi: 10.2147/dmso.s314379
7. Dashti, HS, Gómez-Abellán, P, Qian, J, Esteban, A, Morales, E, Scheer, FAJL, et al. Late eating is associated with cardiometabolic risk traits, obesogenic behaviors, and impaired weight loss. Am J Clin Nutr. (2020) 113:154–61. doi: 10.1093/ajcn/nqaa264
8. Mazzone, CM, Liang-Guallpa, J, Li, C, Wolcott, NS, Boone, MH, Southern, M, et al. High-fat food biases hypothalamic and mesolimbic expression of consummatory drives. Nat Neurosci. (2020) 23:1253–66. doi: 10.1038/s41593-020-0684-9
9. Malesza, IJ, Malesza, M, Walkowiak, J, Mussin, N, Walkowiak, D, Aringazina, R, et al. High-fat, Western-style diet, systemic inflammation, and gut microbiota: a narrative review. Cells. (2021) 10:3164. doi: 10.3390/cells10113164
10. Wu, Y, Wu, C, Shi, T, Cai, Q, Wang, T, Xiong, Y, et al. FAP expression in adipose tissue macrophages promotes obesity and metabolic inflammation. Proc Natl Acad Sci USA. (2023) 120:e2303075120. doi: 10.1073/pnas.2303075120
11. Sadeghi, O, Sadeghian, M, Rahmani, S, Maleki, V, Larijani, B, and Esmaillzadeh, A. Whole-grain consumption does not affect obesity measures: an updated systematic review and meta-analysis of randomized clinical trials. Adv Nutr. (2019) 11:280–92. doi: 10.1093/advances/nmz076
12. Kojta, I, Chacińska, M, and Błachnio-Zabielska, A. Obesity, bioactive lipids, and adipose tissue inflammation in insulin resistance. Nutrients. (2020) 12:1305. doi: 10.3390/nu12051305
13. Tong, Q, Ye, CP, Jones, JE, Elmquist, JK, and Lowell, BB. Synaptic release of GABA by AgRP neurons is required for normal regulation of energy balance. Nature. (2008) 11:998–1000. doi: 10.1038/nn.2167
14. Engstrom Ruud, L, Pereira, MMA, de Solis, AJ, Fenselau, H, and Bruning, JC. NPY mediates the rapid feeding and glucose metabolism regulatory functions of AgRP neurons. Nat Commun. (2020) 11:442. doi: 10.1038/s41467-020-14291-3
15. Krashes, MJ, Koda, S, Ye, C, Rogan, SC, Adams, AC, Cusher, DS, et al. Rapid, reversible activation of AgRP neurons drives feeding behavior in mice. J Clin Invest. (2011) 121:1424–8. doi: 10.1172/JCI46229
16. Alhadeff, AL, Su, Z, Hernandez, E, Klima, ML, Phillips, SZ, Holland, RA, et al. A neural circuit for the suppression of pain by a competing need state. Cell. (2018) 173:140–152.e15. doi: 10.1016/j.cell.2018.02.057
17. Jikomes, N, Ramesh, RN, Mandelblat-Cerf, Y, and Andermann, ML. Preemptive stimulation of AgRP neurons in fed mice enables conditioned food seeking under threat. Curr Biol. (2016) 26:2500–7. doi: 10.1016/j.cub.2016.07.019
18. Joly-Amado, A, Denis, RG, Castel, J, Lacombe, A, Cansell, C, Rouch, C, et al. Hypothalamic AgRP-neurons control peripheral substrate utilization and nutrient partitioning. EMBO J. (2012) 31:4276–88. doi: 10.1038/emboj.2012.250
19. Betley, JN, Xu, S, Cao, ZF, Gong, R, Magnus, CJ, Yu, Y, et al. Neurons for hunger and thirst transmit a negative-valence teaching signal. Nature. (2015) 521:180–5. doi: 10.1038/nature14416
20. Chen, YM, Lin, YC, Kuo, TW, and Knight, ZA. Sensory detection of food rapidly modulates arcuate feeding circuits. Cell. (2015) 160:829–41. doi: 10.1016/j.cell.2015.01.033
21. Pullicin, AJ, Glendinning, JI, and Lim, J. Cephalic phase insulin release: a review of its mechanistic basis and variability in humans. Physiol Behav. (2021) 239:113514. doi: 10.1016/j.physbeh.2021.113514
22. Williams, EK, Chang, RB, Strochlic, DE, Umans, BD, Lowell, BB, and Liberles, SD. Sensory neurons that detect stretch and nutrients in the digestive system. Cell. (2016) 166:209–21. doi: 10.1016/j.cell.2016.05.011
23. Langhans, W, Watts, AG, and Spector, AC. The elusive cephalic phase insulin response: triggers, mechanisms, and functions. Physiol Rev. (2023) 103:1423–85. doi: 10.1152/physrev.00025.2022
24. Brüning, JC, and Fenselau, H. Integrative neurocircuits that control metabolism and food intake. Science. (2023) 381:eabl7398. doi: 10.1126/science.abl7398
25. Wilkinson, LL, Ferriday, D, Bosworth, ML, Godinot, N, Martin, N, Rogrs, PJ, et al. Keeping pace with your eating: visual feedback affects eating rate in humans. PLoS One. (2016) 11:e0147603. doi: 10.1371/journal.pone.0147603
26. Li, T, Zhao, M, Raza, A, Guo, J, He, T, Zou, T, et al. The effect of taste and taste perception on satiation/satiety: a review. Food Funct. (2020) 11:2838–47. doi: 10.1039/c9fo02519g
27. Kuang, D, Hanchate, NK, Lee, CY, Heck, A, Ye, X, Erdenebileg, M, et al. Olfactory and neuropeptide inputs to appetite neurons in the arcuate nucleus. bioRxiv. (2023). doi: 10.1101/2023.02.28.530282
28. Chen, J, Cheng, M, Wang, L, Zhang, L, Xu, D, Cao, P, et al. A vagal-NTS neural pathway that stimulates feeding. Curr Biol. (2020) 30:3986–3998.e5. doi: 10.1016/j.cub.2020.07.084
29. Ly, T, Oh, JY, Sivakumar, N, Shehata, S, La Santa, MN, Huang, H, et al. Sequential appetite suppression by oral and visceral feedback to the brainstem. Nature. (2023) 624:130–7. doi: 10.1038/s41586-023-06758-2
30. Brandt, C, Nolte, H, Henschke, S, Engström Ruud, L, Awazawa, M, Morgan, DA, et al. Food perception primes hepatic ER homeostasis via melanocortin-dependent control of mTOR activation. Cell. (2018) 175:1321–1335.e20. doi: 10.1016/j.cell.2018.10.015
31. Alcantara, IC, Tapia, APM, Aponte, Y, and Krashes, MJ. Acts of appetite: neural circuits governing the appetitive, consummatory, and terminating phases of feeding. Nat Metab. (2022) 4:836–47. doi: 10.1038/s42255-022-00611-y
32. Tack, J, Verbeure, W, Mori, H, Schol, J, Van den Houte, K, Huang, IH, et al. The gastrointestinal tract in hunger and satiety signalling. United European Gastroenterol J. (2021) 9:727–34. doi: 10.1002/ueg2.12097
33. Janssen, P, Verschueren, S, Ly, HG, Vos, R, Van Oudenhove, L, and Tack, J. Intragastric pressure during food intake: a physiological and minimally invasive method to assess gastric accommodation. Neurogastroenterol Motil. (2011) 23:316–e154. doi: 10.1111/j.1365-2982.2011.01676.x
34. Cheng, W, Gordian, D, Ludwig, MQ, Pers, TH, Seeley, RJ, and Myers, MG. Hindbrain circuits in the control of eating behaviour and energy balance. Nat Metab. (2022) 4:826–35. doi: 10.1038/s42255-022-00606-9
35. Furukawa, N, Hatano, M, and Nakamura, E. Antro-pancreatic reflexes: long- and short-route reflexes in exocrine and endocrine pancreatic secretion in dogs. Auton Neurosci. (2003) 106:110–8. doi: 10.1016/s1566-0702(03)00075-4
36. Browning, KN, and Travagli, RA. Central nervous system control of gastrointestinal motility and secretion and modulation of gastrointestinal functions. Hoboken: John Wiley & Sons, Inc. (2014) 4, 1339–1368.
37. Cheng, W, Gonzalez, I, Pan, W, Tsang, AH, Adams, J, Ndoka, E, et al. Calcitonin receptor neurons in the mouse nucleus tractus solitarius control energy balance via the non-aversive suppression of feeding. Cell Metab. (2020) 31:301–312.e5. doi: 10.1016/j.cmet.2019.12.012
38. Tesfa, A, Msc, G, Dutt Soni, N, Hussain, S, Lamoria, M, Mossie, A, et al. Hormones, peptides and neurotransmitters, effects on appetite regulation and their relationship to obesity: systematic review. J Chem Health Risks. (2024) 14:317–29. doi: 10.52783/jchr.v14.i01.2254
39. Padilla, SL, Qiu, J, Soden, ME, Sanz, E, Nestor, CC, Barker, FD, et al. Agouti-related peptide neural circuits mediate adaptive behaviors in the starved state. Nat Neurosci. (2016) 19:734–41. doi: 10.1038/nn.4274
40. Phillips, RJ, and Powley, TL. Gastric volume rather than nutrient content inhibits food intake. Am J Phys. (1996) 271:R766–9. doi: 10.1152/ajpregu.1996.271.3.R766
41. Borgmann, D, Ciglieri, E, Biglari, N, Brandt, C, Cremer, AL, Backes, H, et al. Gut-brain communication by distinct sensory neurons differently controls feeding and glucose metabolism. Cell Metab. (2021) 33:1466–1482.e7. doi: 10.1016/j.cmet.2021.05.002
42. Bai, L, Mesgarzadeh, S, Ramesh, KS, Huey, EL, Liu, Y, Gray, LA, et al. Genetic identification of vagal sensory neurons that control feeding. Cell. (2019) 179:1129–1143.e23. doi: 10.1016/j.cell.2019.10.031
43. Brookes, SJ, Spencer, NJ, Costa, M, and Zagorodnyuk, VP. Extrinsic primary afferent signalling in the gut. Nat Rev Gastroenterol Hepatol. (2013) 10:286–96. doi: 10.1038/nrgastro.2013.29
44. Chambers, AP, Sandoval, DA, and Seeley, RJ. Integration of satiety signals by the central nervous system. Curr Biol. (2013) 23:R379–88. doi: 10.1016/j.cub.2013.03.020
45. Pocai, A, Obici, S, Schwartz, GJ, and Rossetti, L. A brain-liver circuit regulates glucose homeostasis. Cell Metab. (2005) 1:53–61. doi: 10.1016/j.cmet.2004.11.001
46. Dockray, GJ. Enteroendocrine cell signalling via the vagus nerve. Curr Opin Pharmacol. (2013) 13:954–8. doi: 10.1016/j.coph.2013.09.007
47. Gribble, FM, and Reimann, F. Enteroendocrine cells: chemosensors in the intestinal epithelium. Annu Rev Physiol. (2016) 78:277–99. doi: 10.1146/annurev-physiol-021115-105439
48. Han, W, Tellez, LA, Perkins, MH, Perez, IO, Qu, T, Ferreira, J, et al. A neural circuit for gut-induced reward. Cell. (2018) 175:665–678.e23. doi: 10.1016/j.cell.2018.08.049
49. Ring, LE, and Zeltser, LM. Disruption of hypothalamic leptin signaling in mice leads to early-onset obesity, but physiological adaptations in mature animals stabilize adiposity levels. J Clin Invest. (2010) 120:2931–41. doi: 10.1172/JCI41985
50. Kim, M, Heo, G, and Kim, SY. Neural signalling of gut mechanosensation in ingestive and digestive processes. Nat Rev Neurosci. (2022) 23:135–56. doi: 10.1038/s41583-021-00544-7
51. Kanoski, SE, Rupprecht, LE, Fortin, SM, De Jonghe, BC, and Hayes, MR. The role of nausea in food intake and body weight suppression by peripheral GLP-1 receptor agonists, exendin-4 and liraglutide. Neuropharmacology. (2012) 62:1916–27. doi: 10.1016/j.neuropharm.2011.12.022
52. Roman, CW, Sloat, SR, and Palmiter, RD. A tale of two circuits: CCKNTS neuron stimulation controls appetite and induces opposing motivational states by projections to distinct brain regions. Neuroscience. (2017) 358:316–24. doi: 10.1016/j.neuroscience.2017.06.049
53. Roman, CW, Derkach, VA, and Palmiter, RD. Genetically and functionally defined NTS to PBN brain circuits mediating anorexia. Nat Commun. (2016) 7:11905. doi: 10.1038/ncomms11905
54. Wu, Q, Boyle, MP, and Palmiter, RD. Loss of GABAergic signaling by AgRP neurons to the parabrachial nucleus leads to starvation. Cell. (2009) 137:1225–34. doi: 10.1016/j.cell.2009.04.022
55. Wu, Q, Clark, MS, and Palmiter, RD. Deciphering a neuronal circuit that mediates appetite. Nature. (2012) 483:594–7. doi: 10.1038/nature10899
56. Carter, ME, Soden, ME, Zweifel, LS, and Palmiter, RD. Genetic identification of a neural circuit that suppresses appetite. Nature. (2014) 503:111–4. doi: 10.1038/nature12596
57. Spring Valdivia, A, Patrone, A, Reynaldo, M, and Perello, M. Acute high fat diet consumption activates the mesolimbic circuit and requires orexin signaling in a mouse model. PLoS One. (2014) 9:e87478. doi: 10.1371/journal.pone.0087478
58. Hamamah, S, Amin, A, Al-Kassir, AL, Chuang, J, and Covasa, M. Dietary fat modulation of gut microbiota and impact on regulatory pathways controlling food intake. Nutrients. (2023) 15:3365. doi: 10.3390/nu15153365
59. Duca, FA, Zhong, L, and Covasa, M. Reduced CCK signaling in obese-prone rats fed a high fat diet. Horm Behav. (2013) 64:812–7. doi: 10.1016/j.yhbeh.2013.09.004
60. Lee, SJ, Krieger, JP, Vergara, M, Quinn, D, McDougle, M, de Araujo, A, et al. Blunted vagal cocaine- and amphetamine-regulated transcript promotes hyperphagia and weight gain. Cell Rep. (2020) 30:2028–2039.e4. doi: 10.1016/j.celrep.2020.01.045
61. Burdyga, G, Spiller, D, Morris, R, Lal, S, Thompson, DG, Saeed, S, et al. Expression of the leptin receptor in rat and human nodose ganglion neurons. Neuroscience. (2002) 109:339–47. doi: 10.1016/s0306-4522(01)00474-2
62. Duca, FA, Waise, TMZ, Peppler, WT, and Lam, TKT. The metabolic impact of small intestinal nutrient sensing. Nat Commun. (2021) 12:903. doi: 10.1038/s41467-021-21235-y
63. Alhabeeb, H, AlFaiz, A, Kutbi, E, AlShahrani, D, Alsuhail, A, AlRajhi, S, et al. Gut hormones in health and obesity: the upcoming role of short chain fatty acids. Nutrients. (2021) 13:481. doi: 10.3390/nu13020481
64. Lal, S, Kirkup, AJ, Brunsden, AM, Thompson, DG, and Grundy, D. Vagal afferent responses to fatty acids of different chain length in the rat. Am J Physiol Gastroint Liver Physiol. (2001) 281:G907–15. doi: 10.1152/ajpgi.2001.281.4.G907
65. Covasa, M, Grahn, J, and Ritter, RC. Reduced hindbrain and enteric neuronal response to intestinal oleate in rats maintained on high-fat diet. Auton Neurosci. (2000) 84:8–18. doi: 10.1016/s1566-0702(00)00176-4
66. Kim, JS, Kirkland, RA, Lee, SH, Cawthon, CR, Rzepka, KW, Minaya, DM, et al. Gut microbiota composition modulates inflammation and structure of the vagal afferent pathway. Physiol Behav. (2020) 225:113082. doi: 10.1016/j.physbeh.2020.113082
67. Messina, G, De Luca, V, Viggiano, A, Ascione, A, Iannaccone, T, Chieffi, S, et al. Autonomic nervous system in the control of energy balance and body weight: personal contributions. Neurol Res Int. (2013) 2013:1–5. doi: 10.1155/2013/639280
68. Smith, AI, and Funder, JW. Proopiomelanocortin processing in the pituitary, central nervous system, and peripheral tissues. Endocr Rev. (1988) 9:159179:159–79. doi: 10.1210/edrv-9-1-159
69. Copperi, F, Kim, JD, and Diano, S. Role of the melanocortin system in the central regulation of cardiovascular functions. Front Physiol. (2021) 12:725709. doi: 10.3389/fphys.2021.725709
70. da Silva, AA, Do Carmo, JM, Wang, Z, and Hall, JE. Melanocortin-4 receptors and sympathetic nervous system activation in hypertension. Curr Hypertens Rep. (2019) 21:46. doi: 10.1007/s11906-019-0951-x
71. Rahmouni, K, Haynes, WG, Morgan, DA, and Mark, AL. Role of melanocortin-4 receptors in mediating renal sympathoactivation to leptin and insulin. J Neurosci. (2003) 23:5998–6004. doi: 10.1523/JNEUROSCI.23-14-05998.2003
72. Samuelsson, AMS, Mullier, A, Maicas, N, Oosterhuis, NR, Bae, SE, Novoselova, TV, et al. Central role for melanocortin-4 receptors in offspring hypertension arising from maternal obesity. Proc Natl Acad Sci USA. (2016) 113:12298–303. doi: 10.1073/pnas.1607464113
73. Berglund, ED, Liu, TM, Kong, XX, Sohn, JW, Vong, L, Deng, Z, et al. Melanocortin 4 receptors in autonomic neurons regulate thermogenesis and glycemia. Nat Neurosci. (2014) 17:911–3. doi: 10.1038/nn.3737
74. Hill, C, and Dunbar, JC. The effects of acute and chronic alpha melanocyte stimulating hormone (αMSH) on cardiovascular dynamics in conscious rats. Peptides. (2002) 23:1625–30. doi: 10.1016/s0196-9781(02)00103-1
75. Ni, XP, Pearce, D, Butler, AA, Cone, RD, and Humphreys, MH. Genetic disruption of gamma-melanocyte-stimulating hormone signaling leads to salt-sensitive hypertension in the mouse. J Clin Invest. (2003) 111:1251–8. doi: 10.1172/JCI16993
76. Li, P, Cui, BP, Zhang, LL, Sun, HJ, Liu, TY, and Zhu, GQ. Melanocortin 3/4 receptors in paraventricular nucleus modulate sympathetic outflow and blood pressure. Exp Physiol. (2012) 98:435–43. doi: 10.1113/expphysiol.2012.067256
77. Blasquez, C, Jégou, S, Tranchand Bunel, D, Fournier, A, and Vaudry, H. Neuropeptide Y inhibits alpha-MSH release from rat hypothalamic slices through a pertussis toxin-sensitive G protein. Brain Res. (1992) 596:163–8. doi: 10.1016/0006-8993(92)91544-o
78. Nakamura, Y, Yanagawa, Y, Morrison, SF, and Nakamura, K. Medullary reticular neurons mediate neuropeptide Y-induced metabolic inhibition and mastication. Cell Metab. (2017) 25:322–34. doi: 10.1016/j.cmet.2016.12.002
79. Bruinstroop, E, Fliers, E, and Kalsbeek, A. Hypothalamic control of hepatic lipid metabolism via the autonomic nervous system. Best Pract Res Clin Endocrinol Metab. (2014) 28:673–84. doi: 10.1016/j.beem.2014.05.001
80. Joly-Amado, A, Cansell, C, Denis, RG, Delbes, AS, Castel, J, Martinez, S, et al. The hypothalamic arcuate nucleus and the control of peripheral substrates. Best Pract Res Clin Endocrinol Metab. (2014) 28:725–37. doi: 10.1016/j.beem.2014.03.003
81. Shi, Z, Madden, CJ, and Brooks, VL. Arcuate neuropeptide Y inhibits sympathetic nerve activity via multiple neuropathways. J Clin Invest. (2017) 127:2868–80. doi: 10.1172/jci92008
82. Shi, Z, Li, B, and Brooks, VL. Role of the paraventricular nucleus of the hypothalamus in the sympathoexcitatory effects of leptin. Hypertension. (2015) 66:1034–41. doi: 10.1161/hypertensionaha.115.06017
83. Cassaglia, PA, Shi, Z, Li, B, Reis, WL, Clute-Reinig, NM, Stern, JE, et al. Neuropeptide Y acts in the paraventricular nucleus to suppress sympathetic nerve activity and its baroreflex regulation. J Physiol. (2014) 592:1655–75. doi: 10.1113/jphysiol.2013.268763
84. Wehrens, SMT, Christou, S, Isherwood, C, Middleton, B, Gibbs, MA, Archer, SN, et al. Meal timing regulates the human circadian system. Curr Biol. (2017) 27:1768–1775.e3. doi: 10.1016/j.cub.2017.04.059
85. Shi, Z, Pelletier, NE, Wong, J, Li, B, Sdrulla, AD, Madden, CJ, et al. Leptin increases sympathetic nerve activity via induction of its own receptor in the paraventricular nucleus. eLife. (2020) 9:e55357. doi: 10.7554/eLife.55357
86. Obradovic, M, Sudar-Milovanovic, E, Soskic, S, Essack, M, Arya, S, Stewart, AJ, et al. Leptin and obesity: role and clinical implication. Front Endocrinol. (2021) 12:585887. doi: 10.3389/fendo.2021.585887
87. Shi, Z, and Brooks, VL. Leptin differentially increases sympathetic nerve activity and its baroreflex regulation in female rats: role of oestrogen. J Physiol. (2014) 593:1633–47. doi: 10.1113/jphysiol.2014.284638
88. Cassaglia, PA, Hermes, SM, Aicher, SA, and Brooks, VL. Insulin acts in the arcuate nucleus to increase lumbar sympathetic nerve activity and baroreflex function in rats. J Physiol. (2011) 589:1643–62. doi: 10.1113/jphysiol.2011.205575
89. Clegg, DJ, Brown, LM, Woods, SC, and Benoit, SC. Gonadal hormones determine sensitivity to central leptin and insulin. Diabetes. (2006) 55:978–87. doi: 10.2337/diabetes.55.04.06.db05-1339
90. Kelly, MJ, and Qiu, J. Estrogen signaling in hypothalamic circuits controling reproduction. Brain Res. (2010) 1364:44–52. doi: 10.1016/j.brainres.2010.08.082
91. Shi, Z, Wong, J, and Brooks, VL. Obesity: sex and sympathetics. Biol Sex Differ. (2020) 11:10. doi: 10.1186/s13293-020-00286-8
92. Young, CN, Deo, SH, Chaudhary, K, Thyfault, JP, and Fadel, PJ. Insulin enhances the gain of arterial baroreflex control of muscle sympathetic nerve activity in humans. J Physiol. (2010) 588:3593–603. doi: 10.1113/jphysiol.2010.191866
93. Grassi, G, Biffi, A, Dell'Oro, R, Quarti Trevano, F, Seravalle, G, Corrao, G, et al. Sympathetic neural abnormalities in type 1 and type 2 diabetes: a systematic review and meta-analysis. J Hypertens. (2020) 38:1436–42. doi: 10.1097/hjh.0000000000002431
94. Waaler, BA, and Eriksen, M. Postprandial cardiovascular responses in man after ingestion of carbohydrate, protein or fat. Acta Physiol Scand. (1992) 146:321–7. doi: 10.1111/j.1748-1716.1992.tb09426.x
95. Dagenais, GR, Oriol, A, and McGregor, M. Hemodynamic effects of carbohydrate and protein meals in man: rest and exercise. J Appl Physiol. (1966) 21:1157–62. doi: 10.1152/jappl.1966.21.4.1157
96. Gentilcore, D, Jones, KL, O'Donovan, DG, and Horowitz, M. Postprandial hypotension - novel insights into pathophysiology and therapeutic implications. Curr Vasc Pharmacol. (2006) 4:161–71. doi: 10.2174/157016106776359826
97. Beck, B. Neuropeptide Y in normal eating and in genetic and dietary-induced obesity. Philos Trans R Soc Lond Ser B Biol Sci. (2006) 361:1159–85. doi: 10.1098/rstb.2006.1855
98. Kohsaka, A, Laposky, AD, Ramsey, KM, Estrada, C, Joshu, C, Kobayashi, Y, et al. High-fat diet disrupts behavioral and molecular circadian rhythms in mice. Cell Metab. (2007) 6:414–21. doi: 10.1016/j.cmet.2007.09.006
99. Lee, AK, Mojtahed-Jaberi, M, Kyriakou, T, Astarloa, EA, Arno, M, Marshall, NJ, et al. Effect of high-fat feeding on expression of genes controlling availability of dopamine in mouse hypothalamus. Nutrition. (2010) 26:411–22. doi: 10.1016/j.nut.2009.05.007
100. Zammaretti, F, Panzica, G, and Eva, C. Sex-dependent regulation of hypothalamic neuropeptide Y-Y1receptor gene expression in moderate/high fat, high-energy diet-fed mice. J Physiol. (2007) 583:445–54. doi: 10.1113/jphysiol.2007.133470
101. Mele, P, Zammaretti, F, Longo, A, Panzica, G, Oberto, A, and Eva, C. Sex-dependent regulation of hypothalamic neuropeptide Y-Y1 receptor gene expression in leptin treated obese (Ob/Ob) or lean mice. Brain Res. (2016) 1649:102–9. doi: 10.1016/j.brainres.2016.07.022
102. Sakamoto, K, Butera, MA, Zhou, C, Maurizi, G, Chen, B, Ling, L, et al. Overnutrition causes insulin resistance and metabolic disorder through increased sympathetic nervous system activity. Cell Metab. (2025) 37:121–137.e6. doi: 10.1016/j.cmet.2024.09.012
103. Pereira, R, Costa, A, Warkentin, S, Vilela, S, and Oliveira, A. Sleep duration is associated with appetitive traits in school-age years – results from the generation XXI birth cohort. Appetite. (2024) 199:107384. doi: 10.1016/j.appet.2024.107384
104. Meyhöfer, S, Chamorro, R, Hallschmid, M, Spyra, D, Klinsmann, N, Schultes, B, et al. Late, but not early, night sleep loss compromises neuroendocrine appetite regulation and the desire for food. Nutrients. (2023) 15:2035. doi: 10.3390/nu15092035
105. Templeman, I, Smith, HA, Walhin, JP, Middleton, B, Gonzalez, JT, Karagounis, LG, et al. Unacylated ghrelin, leptin, and appetite display diurnal rhythmicity in lean adults. J Appl Physiol. (2021) 130:1534–43. doi: 10.1152/japplphysiol.00920.2020
106. Gallegos, JV, Boege, HL, Zuraikat, FM, and St-Onge, MP. Does sex influence the effects of experimental sleep curtailment and circadian misalignment on regulation of appetite? Curr Opin Endocr Metab Res. (2021) 17:20–5. doi: 10.1016/j.coemr.2020.10.006
107. Podgórski, R, Galiniak, S, Mazur, A, and Domin, A. The association of the hypothalamic-pituitary-adrenal axis with appetite regulation in children with fetal alcohol spectrum disorders (FASDs). Nutrients. (2023) 15:1366. doi: 10.3390/nu15061366
108. Carnell, S, Benson, L, Papantoni, A, Chen, L, Huo, Y, Wang, Z, et al. Obesity and acute stress modulate appetite and neural responses in food word reactivity task. PLoS One. (2022) 17:e0271915. doi: 10.1371/journal.pone.0271915
109. Toft-Nielsen, MB, Damholt, MB, Madsbad, S, Hilsted, LM, Hughes, TE, Michelsen, BK, et al. Determinants of the impaired secretion of glucagon-like peptide-1 in type 2 diabetic patients. J Clin Endocrinol Metab. (2001) 86:3717–23. doi: 10.1210/jcem.86.8.7750
110. Aponte, Y, Atasoy, D, and Sternson, SM. AGRP neurons are sufficient to orchestrate feeding behavior rapidly and without training. Hoboken: John Wiley & Sons, Inc. (2011) 14, 351–355.
111. De Solis, AJ, Del Río-Martín, A, Radermacher, J, Chen, W, Steuernagel, L, Bauder, CA, et al. Reciprocal activity of AgRP and POMC neurons governs coordinated control of feeding and metabolism. Hoboken: John Wiley & Sons, Inc. (2024) 6, 473–493.
112. Adam, TC, and Westerterp-Plantenga, MS. Nutrient-stimulated GLP-1 release in normal-weight men and women. Hoboken: John Wiley & Sons, Inc. (2005).
113. Enç, FY, Imeryüz, N, Akin, L, Turoğlu, T, Dede, F, Haklar, G, et al. Inhibition of gastric emptying by acarbose is correlated with GLP-1 response and accompanied by CCK release. Hoboken: John Wiley & Sons, Inc. (2001).
114. Geliebter, A, Yahav, EK, Gluck, ME, and Hashim, SA. Gastric capacity, test meal intake, and appetitive hormones in binge eating disorder. Hoboken: John Wiley & Sons, Inc. (2004).
AgRP – Agouti-related protein
AP – Area postrema
ARC – Arcuate nucleus
CCK – Cholecystokinin
CGRP – Calcitonin gene-related peptide
DMH – Dorsomedial hypothalamus
DMV – Dorsal motor nucleus of the vagus
GABA – Gamma-aminobutyric acid
GLP-1 – Glucagon-like peptide-1
GLP-1R – Glucagon-like peptide-1 receptor
Gpr65 – G protein-coupled receptor 65
HFD – High-fat diet
IGP – Intragastric pressure
MC1R-MC5R – Melanocortin receptor 1–5
MSH – Melanocyte-stimulating hormone
NG – Nodose ganglion
NPY – Neuropeptide Y
NTS – Nucleus of the solitary tract
Oxtr – Oxytocin receptor
PBN – Parabrachial nucleus
POMC – Pro-opiomelanocortin
PVN – Paraventricular nucleus
PYY – Peptide YY
VANs – Vagal afferent nerves
Vip – Vasoactive intestinal peptide
Keywords: appetite control, neural pathways, blood nutrient level, dietary behavior, vagal afferent nerves
Citation: Sun X, Liu B, Yuan Y, Rong Y, Pang R and Li Q (2025) Neural and hormonal mechanisms of appetite regulation during eating. Front. Nutr. 12:1484827. doi: 10.3389/fnut.2025.1484827
Received: 22 August 2024; Accepted: 07 March 2025;
Published: 24 March 2025.
Edited by:
Eric Gumpricht, Independent Researcher, Gilbert, AZ, United StatesReviewed by:
Chloé Berland, Université Paris Cité, FranceCopyright © 2025 Sun, Liu, Yuan, Rong, Pang and Li. This is an open-access article distributed under the terms of the Creative Commons Attribution License (CC BY). The use, distribution or reproduction in other forums is permitted, provided the original author(s) and the copyright owner(s) are credited and that the original publication in this journal is cited, in accordance with accepted academic practice. No use, distribution or reproduction is permitted which does not comply with these terms.
*Correspondence: Qiu Li, bGlxaXUxMEAxNjMuY29t
Disclaimer: All claims expressed in this article are solely those of the authors and do not necessarily represent those of their affiliated organizations, or those of the publisher, the editors and the reviewers. Any product that may be evaluated in this article or claim that may be made by its manufacturer is not guaranteed or endorsed by the publisher.
Research integrity at Frontiers
Learn more about the work of our research integrity team to safeguard the quality of each article we publish.