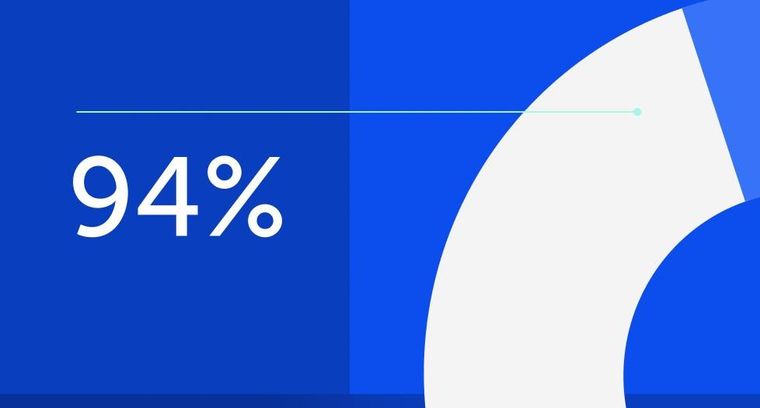
94% of researchers rate our articles as excellent or good
Learn more about the work of our research integrity team to safeguard the quality of each article we publish.
Find out more
REVIEW article
Front. Nutr., 22 January 2025
Sec. Nutrition and Metabolism
Volume 12 - 2025 | https://doi.org/10.3389/fnut.2025.1464269
This article is part of the Research TopicNutrition, Inflammation and Oxidative Stress in Obstetrics and GynecologyView all 12 articles
The consumption of nutritive and non-nutritive sweeteners (NNS) has increased significantly in recent decades. The nutritional status of pregnant women plays a crucial role in determining the likelihood of their offspring developing hypertension in adulthood. While NNSs provide a sweet taste without adding to sugar intake, emerging evidence suggests that maternal consumption of not only nutritive sweeteners (such as fructose) but also NNS may lead to adverse outcomes in offspring, including hypertension. This review provides an overview of the latest research connecting maternal intake of sweeteners to the long-term risk of hypertension in offspring. We examine proposed mechanisms underlying the programming of offspring hypertension by sweeteners, encompassing oxidative stress, dysregulated nutrient sensing signals, abnormal renin-angiotensin system, transcriptome changes, and dysbiotic gut microbiota. Additionally, we outline preventive strategies that can help alleviate offspring hypertension programmed by maternal diets high in sweeteners. Recent advancements in understanding the mechanisms through which maternal consumption of nutritive and non-nutritive sweeteners contributes to offspring hypertension offer promise for addressing this widespread health concern at its developmental roots. Nonetheless, further research is needed to educate the public about the safety of sweetener consumption during pregnancy and lactation.
Hypertension is a widespread health challenge, affecting nearly half of the global adult population (1). Research involving both humans and animals suggests that hypertension may originate early in life (2–4), a concept known as the developmental origins of health and disease (DOHaD) hypothesis (5). An adverse in utero environment can cause morphological and functional changes during fetal development, increasing the likelihood of the offspring developing diseases later in life. Specifically, maternal imbalanced nutrition has been associated with early life insults.
The consumption of nutritive and non-nutritive sweeteners (NNS) has increased in recent decades (6, 7). Nutritive sweeteners add calories and affect blood sugar, whereas NNS are low-calorie or calorie-free. A significant source of added sugars in the diet is sugar-sweetened beverages (SSBs) (6). Emerging evidence suggests a positive association between the intake of SSBs and the global rise in obesity and cardiometabolic disorders (7). Consequently, the World Health Organization advices reducing sugar intake. Of note is that pregnant women consume as much or even more sweeteners than the general population (8, 9). Although the consumption of sweeteners during gestation may negatively impact gestational results and the health of the next generation, debates continue, and the mechanisms remain unclear (9).
The most common nutritive sweeteners are sucrose (i.e., table sugar) and high-fructose corn syrup (HFCS). Artificially sweetened beverages, also known as NNB, are also a significant source of dietary sugar. A comprehensive meta-analysis involving 35 studies revealed a positive correlation between both SSBs and artificially sweetened beverages (ASBs) with hypertension (10). Nevertheless, there is still uncertainty surrounding the impact of nutritive and non-nutritive sweeteners consumption during pregnancy on the blood pressure (BP) of offspring. In this review, we will examine and consolidate existing literature concerning both nutritive and non-nutritive sweeteners. We will explore evidence linking maternal exposure to sweeteners with offspring hypertension and discuss proposed mechanisms. For a comprehensive visual representation, please refer to Figure 1.
Figure 1. Schematic representation of the interrelationships between maternal exposure to sweeteners, developmental programming, and offspring hypertension.
Nutritive sweeteners are substances that provide calories and are commonly used to sweeten foods and beverages. They encompass both natural and processed sugars. Natural sugars include sucrose, fructose, glucose, lactose, maltose, honey, maple syrup, sugar alcohols, molasses, etc. Processed sugars, on the other hand, are sweeteners that have been extracted and refined from their natural sources. Well-known examples of processed sugars include table sugar (sucrose), brown sugar, and HFCS.
Nutritive sweeteners are extensively used not only for their sweetness but also for their ability to improve the preservation and texture of foods. However, excessive consumption of these sweeteners can result in a myriad of health concerns, including diabetes, obesity, and dental cavities. A meta-analysis of human studies demonstrated a positive association between fructose consumption and elevated BP, as well as certain components of metabolic syndrome (11). Additionally, in a cohort study investigating the intake of SSBs and ASBs during gestation, an elevated likelihood of preterm delivery was observed (12). Although fructose consumption may have harmful effects on the developing fetus, no long-term studies have yet evaluated the influence of maternal fructose consumption on the risk of hypertension in offspring (13). The health implications for human offspring resulting from high fructose consumption during gestation remain largely unclear.
Over the past decade, corresponding with trends in the general population is the increase in consumption of NSS during pregnancy (8). For pregnant and lactating women, as well as the general public, seven non-nutritive sweeteners are currently approved for use in foods and consumption: aspartame, acesulfame-K, saccharin, sucralose, neotame, stevia, and advantame (9, 14). Their structures and relative sweetness, as well as their Acceptable Daily Intake (ADI), are summarized in Table 1. Among these, acesulfame-K and aspartame are the most extensively utilized in foods, with childbearing women and their children being the primary consumers (15, 16). Considering that a variety of NNSs are commonly combined in a single food item, accurately estimating the actual intake of NNS in pregnant women poses a challenge due to their widespread presence in numerous foods, beverages, supplements, and medications (15, 16).
While certain NNSs are completely metabolized (e.g., aspartame), the majority (e.g., acesulfame K, saccharin, and sucralose) circulate in the body without being metabolized. Accordingly, transference of NNS to a fetus via the placenta and to an infant through breast milk is plausible. Animal research revealed that acesulfame K, saccharin, and sucralose can be found in the urine of fetus or amniotic fluids, indicating potential transmission via cord blood in utero (17, 18). Though human observational studies have suggested an association between NNS consumption and adulthood metabolic diseases (14), what remains unexplored is the impact of maternal exposure to NNS on the risk of offspring hypertension, as no conclusion has been drawn in this area of research.
Excessive nutritive sweetener consumption is significantly linked to hypertension, primarily due to metabolic effects that promote obesity, insulin resistance, and elevated uric acid levels. NNSs may serve as beneficial substitutes for reducing sugar intake, though their effects on BP can vary.
Both sucrose and HFCS contain fructose, which has been implicated in increasing BP through various mechanisms. Sucrose enzymatically breaks down into one fructose molecule and one glucose molecule, while HFCS contains free fructose and glucose in varying ratios. HFCS-55, a prevalent variety of HFCS utilized in sweetening beverages, contains a composition of 55% fructose, 42% glucose, and 3% oligosaccharides (19). In the Western diet, the main contributors of dietary fructose are sucrose (commonly known as table sugar) and HFCS, despite the natural occurrence of fructose in fruits.
Due to its nearly complete first-pass extraction by the liver, where it is quickly converted to fructose 1-phosphate, fructose metabolism differs significantly from glucose metabolism (20). Unlike glucose, which is transported by various glucose transporters, the transport of fructose occurs via the facilitative glucose transporter 5, utilizing a specific and passive mechanism. The role of glucose transporters in hypertension, however, re-mains largely unknown. Animal studies have shown that the mechanisms by which excess fructose increases BP include increased salt absorption, elevated uric acid levels, chronic stimulation of the sympathetic nervous system, and endothelial dysfunction (21). Utilizing RNA next-generation sequencing (NGS) technology, the transcriptome expression in the offspring was studied within a maternal high-fructose diet model (22, 23). Notably, the onset of hypertension in offspring was related to several differentially expressed genes (DEGs) in the kidneys of neonate offspring, particularly those involved in insulin signaling, fatty acid metabolism, fructose metabolism, gluconeogenesis, and glycolysis (23).
Incorporating 372 studies, a previous review revealed that there is no conclusive evidence regarding the positive or detrimental effects of NNSs on BP (24). The three primary artificial sweeteners used in soft drinks and diet sodas are aspartame, stevia, and sucralose. An acute injection of aspartame was shown to reduce systolic BP in spontaneously hypertensive rats (25), though its long-term effects remain unclear. Stevia may cause slight reductions in BP, but the magnitude of these effects is minimal (26). Sucralose, which has a molecular structure nearly identical to table sugar, has little impact on BP. In healthy older adults, intra-duodenal administration of sucralose was not related to any BP changes (27). Compared to a high fructose-fed rat model, saccharin was found to elevate fasting blood glucose levels without affecting BP (28). The associations between BP and other alternative sweeteners remain unclear. Overall, adopting a balanced diet with reduced added sugars and mindful use of alternative sweeteners can help improve BP management.
While numerous studies have evaluated the effects of nutritive and non-nutritive sweeteners on BP, there is limited human research on how maternal exposure to these substances impacts fetal development and disease risk in offspring, particularly concerning hypertension. Animal studies have shown that fructose or sucrose alone can alter fetal programming (23). However, most studies have examined these sugars as components of a Western diet that also includes fat and salt.
Studies summarized in Table 2 indicate that maternal consumption of sweeteners during gestation and lactation induces hypertension in adult offspring (29–43). Most studies focused on fructose or sucrose, with none examining non-nutritive sweeteners. The commonly used species in these studies are rats and mice. Excess fructose was administered either in chow (60%) or drinking water (10–40%). Similarly, sucrose was administered either in chow (26%) or in 20% drinking water. Considering that each month of rodent life roughly corresponds to three human years (44), Table 2 presents the age at measurement from 8 to 52 weeks, equating to human ages from adolescence to middle adulthood. Considering the multifactorial nature of hypertension, several studies have investigated the combined impact of maternal fructose intake and other pivotal elements of a Western diet, such as high levels of salt and fat consumption. These studies have demonstrated that these elements together synergistically elevate BP in adult offspring (45–48).
Most studies have focused on fructose. Although various doses and durations of fructose consumption have been used to induce hypertension in offspring, no study has explored the dose-or duration-dependent effects of maternal fructose intake on offspring BP, an area that requires further investigation. While sucrose (which contains both fructose and glucose) and fructose share some metabolic pathways, glucose and fructose are metabolized differently in the body. Glucose is used by most cells for immediate energy, whereas fructose is primarily processed in the liver, where it can be converted into glucose or fat. Given these differences in metabolism, further research is needed to determine whether hypertension in offspring induced by maternal sucrose or fructose intake results from the same mechanisms or if they differ.
Various molecular pathways have been identified as contributing to the development of hypertension in offspring as a result of maternal diets rich in sweeteners (2–4). These pathways include oxidative stress, abnormalities in the renin-angiotensin system (RAS), disturbances in nutrient sensing signals, alterations in the transcriptome, and dysbiosis of gut microbiota. Each of these mechanisms will be examined extensively.
Oxidative stress is crucial in fetal programming due to the fetus’s heightened sensitivity to oxidative damage, attributed to its low antioxidant capacity (49). The generation of reactive oxygen species (ROS) mediated by added sugars contributes to the development of hypertension (50). Research has shown that offspring exposed to a maternal diet high in fructose exhibit hypertension, accompanied by elevated levels of oxidative stress (35, 36). In rat offspring exposed to maternal high-fructose intake, elevated ROS levels were observed, along with increased expression of the pg91phox subunit of NADPH oxidase and decreased levels of superoxide dismutase 2 in the brain (35). Another study revealed that a maternal high-fructose diet caused oxidative stress in tissues and increased the expression of pro-inflammatory cytokines in the spleen of adult offspring (36). Additionally, oxidative stress may reduce the production of nitric oxide (NO) by elevating the synthesis of asymmetric dimethylarginine (ADMA), which acts as an endogenous inhibitor of NO synthase (51). Melatonin, an antioxidant, has been shown to avert hypertension in offspring programmed by a maternal high-fructose diet by reducing plasma ADMA levels and enhancing NO production (29).
It’s important to note that even when consumed within recommended safe limits, aspartame and its metabolites have the potential to disturb the balance between oxidants and antioxidants, leading to oxidative stress-related damage (52). Likewise, several artificial sweeteners like saccharin, acesulfame-K, sucralose, and stevia have been associated with adverse health conditions related to oxidative stress (53–55). Therefore, whether oxidative stress induced by maternal consumption of artificial sweeteners contributes to hypertension in offspring requires further investigation.
Comprising various angiotensin peptides with a range of biological functions, the RAS is intricately associated with hypertension (56, 57). The RAS cascade begins with renin, which is derived from its precursor, prorenin (58). The interaction between circulating renin and prorenin with their (pro) renin receptor (PRR) triggers angiotensin II (ANG II)-independent signaling cascades, leading to local ANG II production. Typically, activation of the classic axis—angiotensin-converting enzyme (ACE)/ANG II/ANG II type 1 receptor (AT1R)—promotes vasoconstriction, oxidative stress, and inflammation, all of which contribute to hypertension (57). Hypertension observed in offspring as a result of maternal high-fructose diet programming correlates with abnormal activation of the classic RAS axis, evidenced by increased levels of PRR, angiotensinogen, ACE, and AT1R in organs that regulate BP, such as the kidneys, blood vessels, and brain (32, 35, 38–40, 42).
One study found that a maternal high-fructose diet could activate the RAS across multiple generations (39). Significant increases in BP were observed in the first-and second-generation offspring when compared to the control group. However, this phenomenon did not manifest in the third and fourth generations. Intriguingly, exhibited by the third-generation offspring were the highest levels of serum ANG II, renin and aldosterone. Furthermore, the fructose diet led to elevated mRNA expression of RAS-related genes in the kidneys from the first to the third generation of rat offspring (40). For instance, maternal high-fructose intake induces offspring hypertension coincided with increased expression of several sodium transporters, including Na/K/2Cl cotransporter (NKCC2), sodium/hydrogen exchanger 3 (NHE3), and Na + -Cl − cotransporter (NCC) (40). However, other studies have reported inconsistent findings regarding the impact of maternal fructose consumption on sodium transporters (45).
Conversely, early blockade of the classic RAS has garnered attention as a reprogramming strategy to avert hypertension caused by various maternal insults (59). Revealed in one study is the potential for preventing hypertension in adults whose mothers consumed a high-fructose diet by administering the renin inhibitor aliskiren to offspring aged 2–4 weeks (32). Despite significant advancements in the availability of different RAS-based interventions, there is still a need for deeper investigation into their reprogramming effects on maternal sugar intake-induced hypertension in offspring. Notably, a metabolite of aspartame has been found to inhibit ACE (60). However, whether maternal consumption of aspartame and other artificial sweeteners has beneficial or harmful effects on offspring BP remains to be clarified.
Throughout pregnancy, mechanisms for sensing nutrients detect specific nutritional components to ensure the proper coordination of fetal development and organ function (61). Maternal dietary nutrients serve as metabolic substrates for various processes through the mediation of nutrient-sensing signals. Disrupted nutrient-sensing signals during gestation can lead to adverse fetal programming, contributing to the development of hypertension originating from developmental issues (62). Several key signals involved in programmed hypertension include AMP-activated protein kinase (AMPK), peroxisome proliferator-activated receptors (PPARs), PPARγ coactivator-1α (PGC-1α), and silent in-formation regulator transcript (SIRT) (63).
AMPK is activated by a decline in cellular energy status and serves as a glucose sensor (64, 65). AMPK and SIRT1 mediate the phosphorylation and deacetylation of PGC-1α, respectively (65). The downstream signaling of PGC-1α impacts PPARγ, which regulates the expression of particular PPAR target genes involved in hypertension with developmental origins (66, 67).
In a rat model where both maternal and post-weaning diets were high in fructose, there was a observed decrease in the renal mRNA expression of PGC-1α, AMPK, and PPARs (68). Conversely, resveratrol, which acts as an AMPK activator, can modulate these nutrient-sensing pathways to activate PPAR target genes, thereby offering protection against hypertension in offspring (69). Additionally, maternal prebiotic therapy, which has been shown to prevent elevated BP in adult offspring of fructose-fed dams, was connected to increased protein abundance of phosphorylated AMPKα2 (33). These findings suggest a potential connection between maternal sugar intake and dysregulated nutrient-sensing signals underlying hypertension with developmental origins.
Maternal high-fructose intake can induce long-term transcriptome changes (22, 69), which is a key player involved in hypertension of developmental origins (23, 70, 71). Notably, different organs respond uniquely to developmental programming, leading to organ-specific alterations in the transcriptome (69).
Our previous research revealed significant transcriptome changes in the 1-day-old male offspring kidneys exposed to maternal high-fructose diet (22). In total, 2,706 DEGs were identified, comprising 1,214 upregulated and 1,492 downregulated genes. Among them, 20 out of 2,706 high-fructose diet-related DEGs are associated with regulation of BP. Notably, we also identified 14 significantly regulated Kyoto Encyclopedia of Genes and Genomes (KEGG) pathways. Notably, the PPAR signaling pathway emerged as a nutrient-sensing signaling mechanism linking maternal fructose intake to offspring hypertension. Furthermore, our findings implicate Hpgds, Cyp2c23, Ptges, and Ptgds, which are involved in arachidonic acid metabolism, in the development of hypertension in offspring induced by a maternal high-fructose diet.
Arachidonic acid metabolites involved in the pathogenesis of hypertension (72). The metabolism of arachidonic acid can lead to the formation of epoxyeicosatrienoic acids (EETs) through the action of cytochrome P450 (CYP) epoxygenases. Following their formation, EETs undergo conversion into dihydroxyeicosatrienoic acids (DHETs) through the action of soluble epoxide hydrolase (SEH) (73). EETs have vasodilatory effects, where-as DHETs exert vasoconstrictive actions. Maternal high-fructose diet can enhance SEH activity and renal DHET in the kidneys of 3-week-old male rat offspring (30). Conversely, our follow-up study revealed that targeting on arachidonic acid pathway by 15-Deoxy-Δ12,14-prostagandin J2 (15dPGJ2) or SEH inhibitor 12-(3-adamantan-1-yl-ureido)-dodecanoic acid prevented offspring hypertension pro-grammed by maternal high-fructose intake (31).
The gut microbiota forms a symbiotic community of trillions of microbes from more than 1,000 species, playing an active role in modulating the host’s physiological functions (74), including the regulation of BP (75–77). Interactions between the host and microbes are primarily facilitated by microbial-derived metabolites. Among these, dietary-derived bacterial metabolites, such as trimethylamine N-oxide (TMAO), tryptophan derivatives, short-chain fatty acids (SCFAs), and branched-chain amino acids, are notably involved in regulating BP homeostasis (75–77).
Small amounts of dietary fructose are effectively metabolized by the gut, but higher doses can exceed the intestinal capacity, resulting in alterations in gut microbiota composition and dysbiosis (78, 79). The effects of alternative sweeteners on gut microbiota have also been documented (80). Among NNSs, consumption of saccharin (81, 82), sucralose (83), and acesulfame K (84, 85) has been shown to alter gut microbiota composition. Although studies do not consistently identify specific changes in taxa in response to different NNSs (86), a consistent finding is the depletion of Akkermansia muciniphila when exposed to NNSs like saccharin, sucralose, and acesulfame K in both adult and infant mice (87, 88). Additionally, aspartame can be rapidly metabolized and related to SCFA production, especially propionate production (17).
Offspring hypertension induced by maternal fructose intake is correlated with dysbiosis of gut microbiota (89). Supplementing the drinking water of pregnant rats with 10% fructose led to significant alterations in the maternal microbiome, particularly diminishing beneficial microbes like Lactobacillus and Bacteroides (90). Likewise, a maternal diet high in fructose also influenced the microbiome of rat offspring. In adult male offspring, hypertension programmed by maternal consumption of high fructose was associated with a reduced presence of the genus Akkermansia (34). In another study, adult rat progeny born to dams who were fed a diet comprising 60% fructose throughout gestation and lactation periods exhibited an elevation in the Firmicutes to Bacteroidetes ratio (68), a microbial marker of hypertension.
Conversely, treatments such as prebiotics, postbiotics, probiotics, and modulation of microbial metabolites have shown potential in mitigating hypertension induced by maternal fructose diets (85). For instance, perinatal supplementation with Lactobacillus casei prevented hypertension in adult offspring born to dams received excessive intake of fructose (33). Additionally, perinatal supplementation of inulin, a widely recognized prebiotic, conferred protection against maternal high-fructose diet-primed hypertension (33). The beneficial effects of inulin are linked to elevated plasma propionate levels and the reversal of reduced expression of SCFA receptors caused by a high-fructose diet. Postbiotics, encompassing diverse constituents like SCFAs, have also demonstrated potential in reversing hypertension programming (91). Acetate, a SCFA, can regulate BP via its receptors (77). In a rodent model of maternal high-fructose diet, supplementing acetate during the perinatal period showed beneficial effects against offspring hypertension (34). Moreover, adjusting the microbial metabolite TMAO has shown efficacy in shielding against hypertension induced by fructose in offspring. TMAO, derived from trimethylamine (TMA), has been linked to the risk of cardiovascular disease (92, 93). Inhibition of microbe-dependent TMAO and TMA formation, achieved using the choline analog DMB (94), protected adult rat offspring from hypertension programmed by a maternal diet high in fructose (34). Despite the documented issues in offspring’s gut microbiota associated with the maternal consumption of fructose, the programming effects of alternative sweeteners on offspring BP has yet to be fully understood.
Given that sweeteners are only one component of the maternal diet and various maternal nutritional factors can contribute to offspring hypertension, there may be other mechanisms, aside from those mentioned above, that play a role in the pathogenesis of nutritional programming associated with hypertension of developmental origins (95–97). One potential mechanism is epigenetic regulation, a key factor in developmental programming and hypertension (98–100). Changes in gene expression could result from epigenetic mechanisms, including aberrant DNA methylation, histone modification, and microRNAs (miRNAs) (101). One study found that maternal high-fructose diet-induced hypertension in multigenerational offspring is linked to an enrichment of active histone marks, such as H3Ac and H3K4me2, and a decrease in repressive histone marks, such as H3K9me3 and H3K27me3, on the pro(renin) receptor promoter (40). While the exact effects of epigenetic mechanisms related to maternal sweetener intake and their influence on the risk of hypertension in offspring are not fully understood, they warrant further investigation. Additionally, NSSs can interact with taste receptors and the taste-selective G-protein, α-gustducin, which are involved in body weight maintenance and the risk of chronic diseases, including hypertension (102, 103). Therefore, the potential role of taste receptor signaling should be considered, despite the current lack of supporting information.
Primary prevention aims to avert hypertension before it manifests. To this end, it is crucial to avoid excessive consumption of sweeteners during pregnancy and lactation. Additionally, early-life interventions can reprogram the molecular mechanisms underlying sweetener-related hypertension with developmental origins, thereby preventing the onset of hypertension in adulthood (4).
First, several antioxidants have been investigated for their potential to counteract the adverse effects of excessive fructose consumption (104). These include L-arginine (105), L-citrulline (106), L-taurine (107), folic acid (108), melatonin (109), N-acetylcysteine (110), α-lipoic acid (111), polyphenols (112), and resveratrol (113). However, only a limited number of interventions have been investigated in the context of maternal sweetener intake-induced hypertension in offspring. Melatonin, a multifunctional hormone primarily secreted by the pineal gland, possesses strong antioxidant properties (114, 115). In a rat model of a maternal high-fructose diet, perinatal melatonin treatment was shown to decrease ADMA levels and increase NO bioavailability (29). Melinjo seed extract is rich in polyphenols, including resveratrol (116). Numerous studies have demonstrated that dietary polyphenol intake is associated with positive health outcomes, including reduced hypertension (117). One study found that maternal intake of melinjo seed extract during lactation mitigated the increase in BP induced by high-fructose consumption in female adult offspring (37). Another study indicated that maternal resveratrol supplementation reduced renal oxidative stress and prevented programmed hypertension induced by high-fructose intake (68).
Secondly, several interventions targeting the RAS have demonstrated benefits in protecting against hypertension with developmental origins. These interventions include renin inhibitors (32), ACE inhibitors (118), angiotensin receptor blockers (ARBs) (119), and AT1R antisense (120). Of these, only the aliskirin (a renin inhibitor) and losartan (an ARB) have been specifically evaluated for its reprogramming effects against hypertension programmed by maternal high-fructose diets in offspring (32, 35).
Thirdly, targeting nutrient-sensing pathways such as AMPK and PPAR has shown potential in reprogramming hypertension with developmental origins in several animal models (66, 67). Metformin, known for its ability to lower blood glucose by targeting gluconeogenesis and activating AMPK (121), has been particularly effective. Oral administration of metformin to young offspring exposed to a maternal high-fructose diet prevented programmed hypertension, coinciding with a decrease in AT1R and increases in SIRT1 and PGC-1α (35). Additionally, perinatal use of 15dPGJ2, a PPARγ ligand, has been shown to avert hypertension programmed by maternal high-fructose intake in offspring (31).
Lastly, gut microbiota-targeted therapies show promise in preventing developmental programming induced by maternal fructose consumption (89). Among these interventions are prebiotics, probiotics, and postbiotics. While fecal microbiota transplantation has demonstrated the ability to alleviate metabolic syndrome-related traits in a rat model on a high-fructose diet (122), its potential effects on the offspring of fructose-fed dams remain unexplored While numerous probiotic microbes, prebiotics, and postbiotics have been linked to health benefits (123–125), there is currently a lack of robust evidence supporting their roles in sugars and alternative sweeteners-induced developmental programming.
Only one study has documented that perinatal supplementation with Lactobacillus casei (a probiotic) or inulin (a prebiotic) protects adult male rat progeny against hypertension programmed by a maternal diet high in fructose (33). Acetate, a SCFA acting as post-biotics, also displayed benefits against maternal high fructose diet-primed offspring hypertension (34). Another approach, involving TMA inhibition through DMB treatment throughout gestation and lactation periods, also protects adult offspring from hypertension complicated by a maternal diet high in fructose (34). Understanding the reprogramming effects of functional foods containing prebiotic-like components, such as polyphenols and resveratrol, on developmental hypertension induced by maternal sugar intake will be critical for their potential clinical application.
Although most animal studies have focused on fructose-induced programmed hypertension, several mechanisms have been hypothesized to be responsible for the programming effects of NSSs (126, 127). These include shaping gut microbiota (80), activation of intestinal sweet taste receptors (128), induction of oxidative stress (129), and changes of brain neurotransmitters (130). Whether these mechanisms can also be targeted for reprogramming deserves further investigation.
Most studies on fructose-induced programmed hypertension focus on males, leaving the role of sex differences—proposed in other models of programmed hypertension (4, 131–133)—unclear. The renal transcriptome in hypertension is sex-specific in offspring programmed by a maternal high-fructose diet (32), which may offer a potential protective mechanism for females. However, whether sex differences influence the programming effects of NSSs and whether they are beneficial or harmful to fetal development remains uncertain. A better understanding of sex-dependent mechanisms underlying sweetener-induced offspring hypertension could lead to novel sex-specific prevention strategies. This review offers a comprehensive overview of different interventions in early life that show promise in addressing hypertension related to maternal sugar intake with developmental origins. In spite of significant progress in animal research, the clinical translation of these findings remains a considerable challenge.
Emerging evidence from animal studies highlights the dangers of maternal sweetener consumption and elucidates the molecular mechanisms that program hypertension in adult offspring. Current research has provided insights into how early-life therapies targeting specific mechanisms can reprogram maternal sweetener-induced hypertension in offspring. These reprogramming approaches include antioxidants, RAS-based interventions, AMPK activators, and gut microbiota-targeted therapies. Given that sweeteners can program multiple organs, resulting in various phenotypes in adult offspring, there is a pressing need for more studies focusing on organ-specific reprogramming effects. Although the primary focus of this review is the maternal consumption of sweeteners, the potential paternal nutritional epigenetic effect cannot be entirely excluded (119), despite the lack of supporting information.
Many NNSs pass through the placenta and are detected in amniotic fluid and breast milk, indicating a potential risk for the developmental programming of adult diseases (134). Although NNSs provide sweetness without contributing to sugar intake, animal models suggest that maternal consumption of NNSs may program offspring to develop obesity or metabolic syndrome (134–136), which are known risk factors for hypertension. However, scant data exist regarding the effect of maternal intake of NNS on offspring BP in both human and animal research. As maternal intake of NNSs continues to rise, it becomes imperative to assess their long-term safety and their effects on offspring.
Y-LT: Conceptualization, Funding acquisition, Supervision, Writing – original draft, Writing – review & editing. C-NH: Conceptualization, Funding acquisition, Supervision, Writing – original draft, Writing – review & editing.
The author(s) declare that financial support was received for the research, authorship, and/or publication of this article. This work was supported by Kaohsiung Chang Gung Memorial Hospital, Kaohsiung, Taiwan, under grants nos. CORPG8P0533, CMRPG8M0721, CMRPG8M0722, CMRPG8M0751, CMRPG8M0752, and CORPG8P0563.
The authors declare that the research was conducted in the absence of any commercial or financial relationships that could be construed as a potential conflict of interest.
All claims expressed in this article are solely those of the authors and do not necessarily represent those of their affiliated organizations, or those of the publisher, the editors and the reviewers. Any product that may be evaluated in this article, or claim that may be made by its manufacturer, is not guaranteed or endorsed by the publisher.
1. Ostchega, Y, Fryar, CD, Nwankwo, T, and Nguyen, DT. Hypertension prevalence among adults aged 18 and over: United States, 2017–2018. NCHS data brief, vol. 364. Hyattsville, MD: National Center for Health Statistics (2020).
2. Paauw, ND, van Rijn, BB, Lely, AT, and Joles, JA. Pregnancy as a critical window for blood pressure regulation in mother and child: programming and reprogramming. Acta Physiol. (2017) 219:241–59. doi: 10.1111/apha.12702
3. Luyckx, VA, Bertram, JF, Brenner, BM, Fall, C, Hoy, WE, Ozanne, SE, et al. Effect of fetal and child health on kidney development and long-term risk of hypertension and kidney disease. Lancet. (2013) 382:273–83. doi: 10.1016/S0140-6736(13)60311-6
4. Hsu, CN, and Tain, YL. Animal models for DOHaD research: focus on hypertension of developmental origins. Biomedicines. (2021) 9:623. doi: 10.3390/biomedicines9060623
5. Fleming, TP, Velazquez, MA, and Eckert, JJ. Embryos, DOHaD and David barker. J Dev Orig Health Dis. (2015) 6:377–83. doi: 10.1017/S2040174415001105
6. Bailey, RL, Fulgoni, VL, Cowan, AE, and Gaine, PC. Sources of added sugars in young children, adolescents, and adults with low and high intakes of added sugars. Nutrients. (2018) 10:102. doi: 10.3390/nu10010102
7. Malik, VS, and Hu, FB. The role of sugar-sweetened beverages in the global epidemics of obesity and chronic diseases. Nat Rev Endocrinol. (2022) 18:205–18. doi: 10.1038/s41574-021-00627-6
8. Sylvetsky, AC, Figueroa, J, Rother, KI, Goran, MI, and Welsh, JA. Trends in low-calorie sweetener consumption among pregnant women in the United States. Curr Dev Nutr. (2019) 3:nzz004. doi: 10.1093/cdn/nzz004
9. Palatnik, A, Moosreiner, A, and Olivier-Van Stichelen, S. Consumption of non-nutritive sweeteners during pregnancy. Am J Obstet Gynecol. (2020) 223:211–8. doi: 10.1016/j.ajog.2020.03.034
10. Jayalath, VH, de Souza, RJ, Ha, V, Mirrahimi, A, Blanco-Mejia, S, Di Buono, M, et al. Sugar-sweetened beverage consumption and incident hypertension: a systematic review and meta-analysis of prospective cohorts. Am J Clin Nutr. (2015) 102:914–21. doi: 10.3945/ajcn.115.107243
11. Kelishadi, R, Mansourian, M, and Heidari-Beni, M. Association of fructose consumption and components of metabolic syndrome in human studies: a systematic review and meta-analysis. Nutrition. (2014) 30:503–10. doi: 10.1016/j.nut.2013.08.014
12. Englund-Ogge, L, Brantsaeter, AL, Haugen, M, Sengpiel, V, Khatibi, A, Myhre, R, et al. Association between intake of artificially sweetened and sugar-sweetened beverages and preterm delivery: a large prospective cohort study. Am J Clin Nutr. (2012) 96:552–9. doi: 10.3945/ajcn.111.031567
13. Jia, G, Hill, MA, and Sowers, JR. Maternal exposure to high fructose and offspring health. Hypertension. (2019) 74:499–501. doi: 10.1161/HYPERTENSIONAHA.119.13017
14. Gardner, C, Wylie-Rosett, J, Gidding, SS, Steffen, LM, Johnson, RK, Reader, D, et al. Nonnutritive sweeteners: current use and health perspectives: a scientific statement from the American Heart Association and the American Diabetes Association. Circulation. (2012) 126:509–19. doi: 10.1161/CIR.0b013e31825c42ee
15. Fitch, C, and Keim, KS. Position of the academy of nutrition and dietetics: use of nutritive and non-nutritive sweeteners. J Acad Nutr Diet. (2012) 112:739–58. doi: 10.1016/j.jand.2012.03.009
16. Sylvetsky, A, Rother, KI, and Brown, R. Artificial sweetener use among children: epidemiology, recommendations, metabolic outcomes, and future directions. Pediatr Clin N Am. (2011) 58:1467–80. doi: 10.1016/j.pcl.2011.09.007
17. Olivier-Van Stichelen, S, Rother, KI, and Hanover, JA. Maternal exposure to non-nutritive sweeteners impacts Progeny's metabolism and microbiome. Front Microbiol. (2019) 10:1360. doi: 10.3389/fmicb.2019.01360
18. Sweatman, TW, and Renwick, AG. Tissue levels of saccharin in the rat during two-generation feeding studies. Toxicol Appl Pharmacol. (1982) 62:465–73. doi: 10.1016/0041-008X(82)90147-8
19. Hanover, LM, and White, JS. Manufacturing, composition, and applications of fructose. Am J Clin Nutr. (1993) 58:724S–32S. doi: 10.1093/ajcn/58.5.724S
20. Tappy, L, and Le, KA. Metabolic effects of fructose and the worldwide increase in obesity. Physiol Rev. (2010) 90:23–46. doi: 10.1152/physrev.00019.2009
21. Klein, AV, and Kiat, H. The mechanisms underlying fructose-induced hypertension: a review. J Hypertens. (2015) 33:912–20. doi: 10.1097/HJH.0000000000000551
22. Tain, YL, Chan, JY, and Hsu, CN. Maternal fructose intake affects transcriptome changes and programmed hypertension in offspring in later life. Nutrients. (2016) 8:757. doi: 10.3390/nu8120757
23. Tain, YL, Hsu, CN, Chan, JY, and Huang, LT. Renal transcriptome analysis of programmed hypertension induced by maternal nutritional insults. Int J Mol Sci. (2015) 16:17826–37. doi: 10.3390/ijms160817826
24. Lohner, S, Toews, I, and Meerpohl, JJ. Health outcomes of non-nutritive sweeteners: analysis of the research landscape. Nutr J. (2017) 16:55. doi: 10.1186/s12937-017-0278-x
25. Kiritsy, PJ, and Maher, TJ. Acute effects of aspartame on systolic blood pressure in spontaneously hypertensive rats. J Neural Transm. (1986) 66:121–8. doi: 10.1007/BF01260907
26. Onakpoya, IJ, and Heneghan, CJ. Effect of the natural sweetener, steviol glycoside, on cardiovascular risk factors: a systematic review and meta-analysis of randomised clinical trials. Eur J Prev Cardiol. (2015) 22:1575–87. doi: 10.1177/2047487314560663
27. Leibowitz, A, Bier, A, Gilboa, M, Peleg, E, Barshack, I, and Grossman, E. Saccharin increases fasting blood glucose but not liver insulin resistance in comparison to a high fructose-fed rat model. Nutrients. (2018) 10:341. doi: 10.3390/nu10030341
28. Pham, HT, Stevens, JE, Rigda, RS, Phillips, LK, Wu, T, Hausken, T, et al. Effects of intraduodenal administration of the artificial sweetener sucralose on blood pressure and superior mesenteric artery blood flow in healthy older subjects. Am J Clin Nutr. (2018) 108:156–62. doi: 10.1093/ajcn/nqy060
29. Tain, YL, Leu, S, Wu, KL, Lee, WC, and Chan, JY. Melatonin prevents maternal fructose intake-induced programmed hypertension in the offspring: roles of nitric oxide and arachidonic acid metabolites. J Pineal Res. (2014) 57:80–9. doi: 10.1111/jpi.12145
30. Tain, YL, Wu, KL, Lee, WC, Leu, S, and Chan, JY. Maternal fructose-intake-induced renal programming in adult male offspring. J Nutr Biochem. (2015) 26:642–50. doi: 10.1016/j.jnutbio.2014.12.017
31. Tain, YL, Lee, WC, Wu, KL, Leu, S, and Chan, JY. Targeting arachidonic acid pathway to prevent programmed hypertension in maternal fructose-fed male adult rat offspring. J Nutr Biochem. (2016) 38:86–92. doi: 10.1016/j.jnutbio.2016.08.006
32. Hsu, CN, Wu, KL, Lee, WC, Leu, S, Chan, JY, and Tain, YL. Aliskiren administration during early postnatal life sex-specifically alleviates hypertension programmed by maternal high fructose consumption. Front Physiol. (2016) 7:299. doi: 10.3389/fphys.2016.00299
33. Hsu, CN, Lin, YJ, Hou, CY, and Tain, YL. Maternal Administration of Probiotic or prebiotic prevents male adult rat offspring against developmental programming of hypertension induced by high fructose consumption in pregnancy and lactation. Nutrients. (2018) 10:1229. doi: 10.3390/nu10091229
34. Hsu, CN, Chang-Chien, GP, Lin, S, Hou, CY, and Tain, YL. Targeting on gut microbial metabolite trimethylamine-N-oxide and short-chain fatty acid to prevent maternal high-fructose-diet-induced developmental programming of hypertension in adult male offspring. Mol Nutr Food Res. (2019) 63:e1900073. doi: 10.1002/mnfr.201900073
35. Chao, YM, Wu, KLH, Tsai, PC, Tain, YL, Leu, S, Lee, WC, et al. Anomalous AMPK-regulated angiotensin AT1R expression and SIRT1-mediated mitochondrial biogenesis AT RVLM in hypertension programming of offspring to maternal high fructose exposure. J Biomed Sci. (2020) 27:68. doi: 10.1186/s12929-020-00660-z
36. Tsai, PC, Chao, YM, and Chan, JYH. Sympathetic activation of splenic T-lymphocytes in hypertension of adult offspring programmed by maternal high fructose exposure. Chin J Physiol. (2020) 63:263–75. doi: 10.4103/CJP.CJP_85_20
37. Uson-Lopez, RA, Kataoka, S, Mukai, Y, Sato, S, and Kurasaki, M. Melinjo (Gnetum gnemon) seed extract consumption during lactation improved vasodilation and attenuated the development of hypertension in female offspring of fructose-fed pregnant rats. Birth Defects Res. (2018) 110:27–34. doi: 10.1002/bdr2.1109
38. Guo, Q, Zou, Y, Chang, Y, Zhong, Y, Cheng, L, Jia, L, et al. Transcriptomic evidence of hypothalamus for maternal fructose exposure induced offspring hypertension through AT1R/TLR4 pathway. J Nutr Biochem. (2023) 119:109373. doi: 10.1016/j.jnutbio.2023.109373
39. Seong, HY, Cho, HM, Kim, M, and Kim, I. Maternal high-fructose intake induces multigenerational activation of the renin-angiotensin-aldosterone system. Hypertension. (2019) 74:518–25. doi: 10.1161/HYPERTENSIONAHA.119.12941
40. Cho, HM, and Kim, I. Maternal high-fructose intake induces hypertension through activating histone codes on the (pro)renin receptor promoter. Biochem Biophys Res Commun. (2020) 527:596–602. doi: 10.1016/j.bbrc.2020.04.081
41. Saad, AF, Dickerson, J, Kechichian, TB, Yin, H, Gamble, P, Salazar, A, et al. High-fructose diet in pregnancy leads to fetal programming of hypertension, insulin resistance, and obesity in adult offspring. Am J Obstet Gynecol. (2016) 215:e1–6. doi: 10.1016/j.ajog.2016.03.038
42. Wu, L, Shi, A, Zhu, D, Bo, L, Zhong, Y, Wang, J, et al. High sucrose intake during gestation increases angiotensin II type 1 receptor-mediated vascular contractility associated with epigenetic alterations in aged offspring rats. Peptides. (2016) 86:133–44. doi: 10.1016/j.peptides.2016.11.002
43. Samuelsson, AM, Matthews, PA, Jansen, E, Taylor, PD, and Poston, L. Sucrose feeding in mouse pregnancy leads to hypertension, and sex-linked obesity and insulin resistance in female offspring. Front Physiol. (2013) 4:14. doi: 10.3389/fphys.2013.00014
44. Sengupta, P. The laboratory rat: relating its age with human’s. Int J Prev Med. (2013) 4:624–30.
45. Tain, YL, Lee, WC, Leu, S, Wu, K, and Chan, J. High salt exacerbates programmed hypertension in maternal fructose-fed male offspring. Nutr Metab Cardiovasc Dis. (2015) 25:1146–51. doi: 10.1016/j.numecd.2015.08.002
46. Yamada-Obara, N, Yamagishi, SI, Taguchi, K, Kaida, Y, Yokoro, M, Nakayama, Y, et al. Maternal exposure to high-fat and high-fructose diet evokes hypoadiponectinemia and kidney injury in rat offspring. Clin Exp Nephrol. (2016) 20:853–61. doi: 10.1007/s10157-016-1265-9
47. Eren, OC, Ortiz, A, Afsar, B, Covic, A, Kuwabara, M, Lanaspa, MA, et al. Multilayered interplay between fructose and salt in development of hypertension. Hypertension. (2019) 73:265–72. doi: 10.1161/HYPERTENSIONAHA.118.12150
48. Gray, C, Gardiner, SM, Elmes, M, and Gardner, DS. Excess maternal salt or fructose intake programmes sex-specific, stress-and fructose-sensitive hypertension in the offspring. Br J Nutr. (2016) 115:594–604. doi: 10.1017/S0007114515004936
49. Thompson, LP, and Al-Hasan, Y. Impact of oxidative stress in fetal programming. J Pregnancy. (2012) 2012:1–8. doi: 10.1155/2012/582748
50. Prasad, K, and Dhar, I. Oxidative stress as a mechanism of added sugar-induced cardiovascular disease. Int J Angiol. (2014) 23:217–26. doi: 10.1055/s-0034-1387169
51. Sydow, K, and Münzel, T. ADMA and oxidative stress. Atheroscler Suppl. (2003) 4:41–51. doi: 10.1016/S1567-5688(03)00033-3
52. Choudhary, AK, and Pretorius, E. Revisiting the safety of aspartame. Nutr Rev. (2017) 75:718–30. doi: 10.1093/nutrit/nux035
53. Amin, KA, and AlMuzafar, HM. Alterations in lipid profile, oxidative stress and hepatic function in rat fed with saccharin and methyl-salicylates. Int J Clin Exp Med. (2015) 8:6133–44.
54. Colín-García, K, Elizalde-Velázquez, GA, Gómez-Oliván, LM, and García-Medina, S. Influence of sucralose, acesulfame-k, and their mixture on brain's fish: a study of behavior, oxidative damage, and acetylcholinesterase activity in Daniorerio. Chemosphere. (2023) 340:139928. doi: 10.1016/j.chemosphere.2023.139928
55. Minamiyama, Y, Takemura, S, and Ichikawa, H. Food additive-induced oxidative stress in rat male reproductive organs and hippocampus. Arch Biochem Biophys. (2021) 701:108810. doi: 10.1016/j.abb.2021.108810
56. Te Riet, L, van Esch, JH, Roks, AJ, van den Meiracker, AH, and Danser, AH. Hypertension: renin-angiotensin-aldosterone system alterations. Circ Res. (2015) 116:960–75. doi: 10.1161/CIRCRESAHA.116.303587
57. Bogdarina, I, Welham, S, King, PJ, Burns, SP, and Clark, AJ. Epigenetic modification of the renin-angiotensin system in the fetal programming of hypertension. Circ Res. (2007) 100:520–6. doi: 10.1161/01.RES.0000258855.60637.58
58. Stanton, A. Potential of renin inhibition in cardiovascular disease. J Renin-Angiotensin-Aldosterone Syst. (2003) 4:6–10. doi: 10.3317/jraas.2003.008
59. Tain, YL, and Hsu, CN. The renin–angiotensin system and cardiovascular–kidney–metabolic syndrome: focus on early-life programming. Int J Mol Sci. (2024) 25:3298. doi: 10.3390/ijms25063298
60. Grobelny, D, and Galardy, RE. A metabolite of aspartame inhibits angiotensin converting enzyme. Biochem Biophys Res Commun. (1985) 128:960–4. doi: 10.1016/0006-291X(85)90140-8
61. Jansson, T, and Powell, TL. Role of placental nutrient sensing in developmental programming. Clin Obstet Gynecol. (2013) 56:591–601. doi: 10.1097/GRF.0b013e3182993a2e
62. Tain, YL, and Hsu, CN. Interplay between oxidative stress and nutrient sensing signaling in the developmental origins of cardiovascular disease. Int J Mol Sci. (2017) 18:841. doi: 10.3390/ijms18040841
63. Efeyan, A, Comb, WC, and Sabatini, DM. Nutrient-sensing mechanisms and pathways. Nature. (2015) 517:302–10. doi: 10.1038/nature14190
64. Lin, SC, and Hardie, DG. AMPK: sensing glucose as well as cellular energy status. Cell Metab. (2018) 27:299–313. doi: 10.1016/j.cmet.2017.10.009
65. Sugden, MC, Caton, PW, and Holness, MJ. PPAR control: It’s SIRTainly as easy as PGC. J Endocrinol. (2010) 204:93–104. doi: 10.1677/JOE-09-0359
66. Tain, YL, and Hsu, CN. AMP-activated protein kinase as a reprogramming strategy for hypertension and kidney disease of developmental origin. Int J Mol Sci. (2018) 19:1744. doi: 10.3390/ijms19061744
67. Tain, YL, Hsu, CN, and Chan, JYH. PPARs link early life nutritional insults to later programmed hypertension and metabolic syndrome. Int J Mol Sci. (2016) 17:20. doi: 10.3390/ijms17010020
68. Tain, YL, Lee, WC, Wu, KLH, Leu, S, and Chan, JYH. Resveratrol prevents the development of hypertension programmed by maternal plus post-weaning high-fructose consumption through modulation of oxidative stress, nutrient-sensing signals, and gut microbiota. Mol Nutr Food Res. (2018) 62:e1800066. doi: 10.1002/mnfr.201800066
69. Chao, YM, Tain, YL, Leu, S, Wu, KL, Lee, WC, and Chan, JY. Developmental programming of the metabolic syndrome: next-generation sequencing analysis of transcriptome expression in a rat model of maternal high fructose intake. Sheng Li Xue Bao. (2016) 68:557–67.
70. Tain, YL, Huang, LT, Chan, JY, and Lee, CT. Transcriptome analysis in rat kidneys: importance of genes involved in programmed hypertension. Int J Mol Sci. (2015) 16:4744–58. doi: 10.3390/ijms16034744
71. Costa Ade, F, and Franco, OL. Insights into RNA transcriptome profiling of cardiac tissue in obesity and hypertension conditions. J Cell Physiol. (2015) 230:959–68. doi: 10.1002/jcp.24807
72. Zhou, Y, Khan, H, Xiao, J, and Cheang, WS. Effects of arachidonic acid metabolites on cardiovascular health and disease. Int J Mol Sci. (2021) 22:12029. doi: 10.3390/ijms222112029
73. Morisseau, C, and Hammock, BD. Epoxide hydrolases: mechanisms, inhibitor designs, and biological roles. Annu Rev Pharmacol Toxicol. (2005) 45:311–33. doi: 10.1146/annurev.pharmtox.45.120403.095920
74. Lynch, SV, and Pedersen, O. The human intestinal microbiome in health and disease. N Engl J Med. (2016) 375:2369–79. doi: 10.1056/NEJMra1600266
75. Al Khodor, SA, Reichert, B, and Shatat, IF. The microbiome and blood pressure: can microbes regulate our blood pressure? Front Pediatr. (2017) 5:138. doi: 10.3389/fped.2017.00138
76. O’Donnell, JA, Zheng, T, Meric, G, and Marques, FZ. The gut microbiome and hypertension. Nat Rev Nephrol. (2023) 19:153–67. doi: 10.1038/s41581-022-00654-0
77. Pluznick, JL. Microbial short-chain fatty acids and blood pressure regulation. Curr Hypertens Rep. (2017) 19:25. doi: 10.1007/s11906-017-0722-5
78. Lambertz, J, Weiskirchen, S, Landert, S, and Weiskirchen, R. Fructose: a dietary sugar in crosstalk with microbiota contributing to the development and progression of non-alcoholic liver disease. Front Immunol. (2017) 8:1159. doi: 10.3389/fimmu.2017.01159
79. Zhao, S, Jang, C, Liu, J, Uehara, K, Gilbert, M, Izzo, L, et al. Dietary fructose feeds hepatic lipogenesis via microbiota-derived acetate. Nature. (2020) 579:586–91. doi: 10.1038/s41586-020-2101-7
80. Ruiz-Ojeda, FJ, Plaza-Díaz, J, Sáez-Lara, MJ, and Gil, A. Effects of sweeteners on the gut microbiota: a review of experimental studies and clinical trials. Adv Nutr. (2019) 10:S31–48. doi: 10.1093/advances/nmy037
81. Anderson, RL, and Kirkland, JJ. The effect of sodium saccharin in the diet on caecal microflora. Food Cosmet Toxicol. (1980) 18:353–5. doi: 10.1016/0015-6264(80)90188-1
82. Naim, M, Zechman, JM, Brand, JG, Kare, MR, and Sandovsky, V. Effects of sodium saccharin on the activity of trypsin, chymotrypsin, and amylase and upon bacteria in small intestinal contents of rats. Proc Soc Exp Biol Med. (1985) 178:392–401. doi: 10.3181/00379727-178-42022
83. Bian, X, Chi, L, Gao, B, Tu, P, Ru, H, and Lu, K. Gut microbiome response to sucralose and its potential role in inducing liver inflammation in mice. Front Physiol. (2017) 8:487. doi: 10.3389/fphys.2017.00487
84. Shahriar, S, Ahsan, T, Khan, A, Akhteruzzaman, S, Shehreen, S, and Sajib, AA. Aspartame, acesulfame K and sucralose-influence on the metabolism of Escherichia coli. Metab Open. (2020) 8:100072. doi: 10.1016/j.metop.2020.100072
85. Bian, X, Chi, L, Gao, B, Tu, P, Ru, H, and Lu, K. The artificial sweetener acesulfame potassium affects the gut microbiome and body weight gain in CD-1 mice. PLoS One. (2017) 12:e0178426. doi: 10.1371/journal.pone.0178426
86. Richardson, IL, and Frese, SA. Non-nutritive sweeteners and their impacts on the gut microbiome and host physiology. Front Nutr. (2022) 9:988144. doi: 10.3389/fnut.2022.988144
87. Palmnäs, MS, Cowan, TE, Bomhof, MR, Su, J, Reimer, RA, Vogel, HJ, et al. Low-dose aspartame consumption differentially affects gut microbiota-host metabolic interactions in the diet-induced obese rat. PLoS One. (2014) 9:e109841. doi: 10.1371/journal.pone.0109841
88. Bornemann, V, Werness, SC, Buslinger, L, and Schiffman, SS. Intestinal metabolism and bioaccumulation of sucralose in adipose tissue in the rat. J Toxicol Environ Health A. (2018) 81:913–23. doi: 10.1080/15287394.2018.1502560
89. Hsu, CN, Yu, HR, Chan, JYH, Wu, KLH, Lee, WC, and Tain, YL. The impact of gut microbiome on maternal fructose intake-induced developmental programming of adult disease. Nutrients. (2022) 14:1031. doi: 10.3390/nu14051031
90. Astbury, S, Song, A, Zhou, M, Nielsen, B, Hoedl, A, Willing, BP, et al. High fructose intake during pregnancy in rats influences the maternal microbiome and gut development in the offspring. Front Genet. (2018) 9:203. doi: 10.3389/fgene.2018.00203
91. Wegh, CAM, Geerlings, SY, Knol, J, Roeselers, G, and Belzer, C. Postbiotics and their potential applications in early life nutrition and beyond. Int J Mol Sci. (2019) 20:4673. doi: 10.3390/ijms20194673
92. Schiattarella, GG, Sannino, A, Toscano, E, Giugliano, G, Gargiulo, G, Franzone, A, et al. Gut microbe-generated metabolite trimethylamine-N-oxide as cardiovascular risk biomarker: a systematic review and doseresponse meta-analysis. Eur Heart J. (2017) 38:2948–56. doi: 10.1093/eurheartj/ehx342
93. Guasti, L, Galliazzo, S, Molaro, M, Visconti, E, Pennella, B, Gaudio, GV, et al. TMAO as a biomarker of cardiovascular events: a systematic review and meta-analysis. Intern Emerg Med. (2021) 16:201–7. doi: 10.1007/s11739-020-02470-5
94. Wang, Z, Roberts, AB, Buffa, JA, Levison, BS, Zhu, W, Org, E, et al. Non-lethal inhibition of gut microbial trimethylamine production for the treatment of atherosclerosis. Cell. (2015) 163:1585–95. doi: 10.1016/j.cell.2015.11.055
95. Langley-Evans, SC. Nutritional programming of disease: unravelling the mechanism. J Anat. (2009) 215:36–51. doi: 10.1111/j.1469-7580.2008.00977.x
96. Hsu, CN, and Tain, YL. The double-edged sword effects of maternal nutrition in the developmental programming of hypertension. Nutrients. (1917) 10:10. doi: 10.3390/nu10121917
97. Armitage, JA, Khan, IY, Taylor, PD, Nathanielsz, PW, and Poston, L. Developmental programming of the metabolic syndrome by maternal nutritional imbalance: how strong is the evidence from experimental models in mammals? J Physiol. (2004) 561:355–77. doi: 10.1113/jphysiol.2004.072009
98. Tain, YL, and Hsu, CN. Interplay between maternal nutrition and epigenetic programming on offspring hypertension. J Nutr Biochem. (2024) 127:109604. doi: 10.1016/j.jnutbio.2024.109604
99. Linnér, A, and Almgren, M. Epigenetic programming-the important first 1000 days. Acta Paediatr. (2020) 109:443–52. doi: 10.1111/apa.15050
100. Karabaeva, RZ, Vochshenkova, TA, Mussin, NM, Albayev, RK, Kaliyev, AA, and Tamadon, A. Epigenetics of hypertension as a risk factor for the development of coronary artery disease in type 2 diabetes mellitus. Front Endocrinol (Lausanne). (2024) 15:1365738. doi: 10.3389/fendo.2024.1365738
101. Zhang, L, Lu, Q, and Chang, C. Epigenetics in health and disease. Adv Exp Med Biol. (2020) 1253:3–55. doi: 10.1007/978-981-15-3449-2_1
102. Udagawa, H, Hiramoto, M, Kawaguchi, M, Uebanso, T, Ohara-Imaizumi, M, Nammo, T, et al. Characterization of the taste receptor-related G-protein, α-gustducin, in pancreatic β-cells. J Diabetes Investig. (2020) 11:814–22. doi: 10.1111/jdi.13214
103. Loper, HB, La Sala, M, Dotson, C, and Steinle, N. Taste perception, associated hormonal modulation, and nutrient intake. Nutr Rev. (2015) 73:83–91. doi: 10.1093/nutrit/nuu009
104. Sloboda, DM, Li, M, Patel, R, Clayton, ZE, Yap, C, and Vickers, MH. Early life exposure to fructose and offspring phenotype: implications for long term metabolic homeostasis. J Obes. (2014) 2014:203474. doi: 10.1155/2014/203474
105. Tay, A, Ozçelikay, AT, and Altan, VM. Effects of L-arginine on blood pressure and metabolic changes in fructose-hypertensive rats. Am J Hypertens. (2002) 15:72–7. doi: 10.1016/S0895-7061(01)02231-2
106. Jegatheesan, P, Beutheu, S, Ventura, G, Sarfati, G, Nubret, E, Kapel, N, et al. Effect of specific amino acids on hepatic lipid metabolism in fructose-induced non-alcoholic fatty liver disease. Clin Nutr. (2016) 35:175–82. doi: 10.1016/j.clnu.2015.01.021
107. Li, M, Reynolds, CM, Sloboda, DM, Gray, C, and Vickers, MH. Maternal taurine supplementation attenuates maternal fructose-induced metabolic and inflammatory dysregulation and partially reverses adverse metabolic programming in offspring. J Nutr Biochem. (2015) 26:267–76. doi: 10.1016/j.jnutbio.2014.10.015
108. Tojal, A, Neves, C, Veiga, H, Ferreira, S, Rodrigues, I, Martel, F, et al. Perigestational high folic acid: impact on offspring's peripheral metabolic response. Food Funct. (2019) 10:7216–26. doi: 10.1039/C9FO01807G
109. Song, D, Hutchings, S, and Pang, CC. Chronic N-acetylcysteine prevents fructose-induced insulin resistance and hypertension in rats. Eur J Pharmacol. (2005) 508:205–10. doi: 10.1016/j.ejphar.2004.12.018
110. Pasaoglu, OT, Bircan, FS, Topal, T, and Turkozkan, N. Positive effects of melatonin on renal nitric oxide-asymmetric Dimethylarginine metabolism in fructose-fed rats. Metab Syndr Relat Disord. (2021) 19:120–6. doi: 10.1089/met.2020.0084
111. Li, W, Yang, H, Zhao, Q, Wang, X, Zhang, J, and Zhao, X. Polyphenol-rich loquat fruit extract prevents fructose-induced nonalcoholic fatty liver disease by modulating Glycometabolism, Lipometabolism, oxidative stress, inflammation, intestinal barrier, and gut microbiota in mice. J Agric Food Chem. (2019) 67:7726–37. doi: 10.1021/acs.jafc.9b02523
112. Babacanoglu, C, Yildirim, N, Sadi, G, Pektas, MB, and Akar, F. Resveratrol prevents high-fructose corn syrup-induced vascular insulin resistance and dysfunction in rats. Food Chem Toxicol. (2013) 60:160–7. doi: 10.1016/j.fct.2013.07.026
113. Rabaglino, MB, Moreira-Espinoza, MJ, Lagares, C, Garay, MI, Quiroga, P, Pasqualini, ME, et al. Maternal intake of alpha-lipoic acid prevents development of symptoms associated with a fructose-rich diet in the male offspring in Wistar rats. J Dev Orig Health Dis. (2021) 12:758–67. doi: 10.1017/S2040174420001178
114. Reiter, RJ, Mayo, JC, Tan, DX, Sainz, RM, Alatorre-Jimenez, M, and Qin, L. Melatonin as an antioxidant: under promises but over delivers. J Pineal Res. (2016) 61:253–78. doi: 10.1111/jpi.12360
115. Hardeland, R, Tan, DX, and Reiter, RJ. Kynuramines, metabolites of melatonin and other indoles: the resurrection of an almost forgotten class of biogenic amines. J Pineal Res. (2009) 47:109–26. doi: 10.1111/j.1600-079X.2009.00701.x
116. Ariyanto, EF, Danil, AS, Rohmawaty, E, Sujatmiko, B, and Berbudi, A. Effect of resveratrol in Melinjo seed (Gnetum gnemon L.) extract on type 2 diabetes mellitus patients and its possible mechanism: a review. Curr Diabetes Rev. (2023) 19:e280222201512. doi: 10.2174/1573399818666220228160908
117. Godos, J, Vitale, M, Micek, A, Ray, S, Martini, D, Del Rio, D, et al. Dietary polyphenol intake, blood pressure, and hypertension: a systematic review and Meta-analysis of observational studies. Antioxidants (Basel). (2019) 8:152. doi: 10.3390/antiox8060152
118. Harrap, SB, Nicolaci, JA, and Doyle, AE. Persistent effects on blood pressure and renal haemodynamics following chronic angiotensin converting enzyme inhibition with perindopril. Clin Exp Pharmacol Physiol. (1986) 13:753–65. doi: 10.1111/j.1440-1681.1986.tb02379.x
119. Klimas, J, Olvedy, M, Ochodnicka-Mackovicova, K, Kruzliak, P, Cacanyiova, S, Kristek, F, et al. Perinatally administered losartan augments renal ACE2 expression but not cardiac or renal mas receptor in spontaneously hypertensive rats. J Cell Mol Med. (2015) 19:1965–74. doi: 10.1111/jcmm.12573
120. Iyer, SN, Lu, D, Katovich, MJ, and Raizada, MK. Chronic control of high blood pressure in the spontaneously hypertensive rat by delivery of angiotensin type 1 receptor antisense. Proc Natl Acad Sci USA. (1996) 93:9960–5. doi: 10.1073/pnas.93.18.9960
121. Agius, L, Ford, BE, and Chachra, SS. The metformin mechanism on gluconeogenesis and AMPK activation: the metabolite perspective. Int J Mol Sci. (2020) 21:3240. doi: 10.3390/ijms21093240
122. Di Luccia, B, Crescenzo, R, Mazzoli, A, Cigliano, L, Venditti, P, Walser, J-C, et al. Rescue of Fructose-Induced Metabolic Syndrome by antibiotics or Faecal transplantation in a rat model of obesity. PLoS One. (2015) 10:e0134893. doi: 10.1371/journal.pone.0134893
123. Sanders, ME, Merenstein, DJ, Reid, G, Gibson, GR, and Rastall, RA. Probiotics and prebiotics in intestinal health and disease: from biology to the clinic. Nat Rev Gastroenterol Hepatol. (2019) 16:605–16. doi: 10.1038/s41575-019-0173-3
124. Pandey, KR, Naik, SR, and Vakil, BV. Probiotics, prebiotics and synbiotics—a review. J Food Sci Technol. (2015) 52:7577–87. doi: 10.1007/s13197-015-1921-1
125. Peluzio, MDCG, Martinez, JA, and Milagro, FI. Postbiotics: metabolites and mechanisms involved in microbiota-host interactions. Trends Food Sci Technol. (2021) 108:11–26. doi: 10.1016/j.tifs.2020.12.004
126. Araújo, JR, Martel, F, and Keating, E. Exposure to non-nutritive sweeteners during pregnancy and lactation: impact in programming of metabolic diseases in the progeny later in life. Reprod Toxicol. (2014) 49:196–201. doi: 10.1016/j.reprotox.2014.09.007
127. Kearns, ML, and Reynolds, CM. The impact of non-nutritive sweeteners on fertility, maternal and child health outcomes: a review of human and animal studies. Proc Nutr Soc. (2024) 4:1–13. doi: 10.1017/S0029665124000168
128. Pepino, MY, and Bourne, C. Non-nutritive sweeteners, energy balance, and glucose homeostasis. Curr Opin Clin Nutr Metab Care. (2011) 14:391–5. doi: 10.1097/MCO.0b013e3283468e7e
129. Collison, KS, Makhoul, NJ, Zaidi, MZ, Al-Rabiah, R, Inglis, A, Andres, BL, et al. Interactive effects of neonatal exposure to monosodium glutamate and aspartame on glucose homeostasis. Nutr Metab (Lond). (2012) 9:58. doi: 10.1186/1743-7075-9-58
130. Abdel-Salam, OM, Salem, NA, and Hussein, JS. Effect of aspartame on oxidative stress and monoamine neurotransmitter levels in lipopolysaccharide treated mice. Neurotox Res. (2012) 21:245–55. doi: 10.1007/s12640-011-9264-9
131. Ojeda, NB, Intapad, S, and Alexander, BT. Sex differences in the developmental programming of hypertension. Acta Physiol. (2014) 210:307–16. doi: 10.1111/apha.12206
132. Tain, YL, Wu, MS, and Lin, YJ. Sex differences in renal transcriptome and programmed hypertension in offspring exposed to prenatal dexamethasone. Steroids. (2016) 115:40–6. doi: 10.1016/j.steroids.2016.08.006
133. Aiken, CE, and Ozanne, SE. Sex differences in developmental programming models. Reproduction. (2013) 145:R1–R13. doi: 10.1530/REP-11-0489
134. Bridge-Comer, PE, Vickers, MH, Morton-Jones, J, Spada, A, Rong, J, and Reynolds, CM. Impact of maternal intake of artificial sweetener, Acesulfame-K, on metabolic and reproductive health outcomes in male and female mouse offspring. Front Nutr. (2021) 8:745203. doi: 10.3389/fnut.2021.745203
135. Nettleton, JE, Cho, NA, Klancic, T, Nicolucci, AC, Shearer, J, Borgland, SL, et al. Maternal low-dose aspartame and stevia consumption with an obesogenic diet alters metabolism, gut microbiota and mesolimbic reward system in rat dams and their offspring. Gut. (2020) 69:1807–17. doi: 10.1136/gutjnl-2018-317505
Keywords: developmental origins of health and disease, pregnancy, sweetener, hypertension, fructose, sugar, sugar-sweetened beverage
Citation: Tain Y-L and Hsu C-N (2025) Does maternal consumption of nutritive and non-nutritive sweeteners result in offspring hypertension? Front. Nutr. 12:1464269. doi: 10.3389/fnut.2025.1464269
Received: 13 July 2024; Accepted: 10 January 2025;
Published: 22 January 2025.
Edited by:
Dorota Formanowicz, Poznan University of Medical Sciences, PolandReviewed by:
Myron R. Szewczuk, Queen’s University, CanadaCopyright © 2025 Tain and Hsu. This is an open-access article distributed under the terms of the Creative Commons Attribution License (CC BY). The use, distribution or reproduction in other forums is permitted, provided the original author(s) and the copyright owner(s) are credited and that the original publication in this journal is cited, in accordance with accepted academic practice. No use, distribution or reproduction is permitted which does not comply with these terms.
*Correspondence: Chien-Ning Hsu, Y2hpZW5fbmluZ19oc3VAaG90bWFpbC5jb20=
Disclaimer: All claims expressed in this article are solely those of the authors and do not necessarily represent those of their affiliated organizations, or those of the publisher, the editors and the reviewers. Any product that may be evaluated in this article or claim that may be made by its manufacturer is not guaranteed or endorsed by the publisher.
Research integrity at Frontiers
Learn more about the work of our research integrity team to safeguard the quality of each article we publish.