- Department of Biochemistry and Biotechnology, Vasyl Stefanyk Precarpathian National University, Ivano-Frankivsk, Ukraine
Fructose is a valuable and healthy nutrient when consumed at normal levels (≤50 g/day). However, long-term consumption of excessive fructose and elevated endogenous production can have detrimental health impacts. Fructose-initiated nonenzymatic glycation (fructation) is considered as one of the most likely mechanisms leading to the generation of reactive species and the propagation of nonenzymatic processes. In the later stages of glycation, poorly degraded advanced glycation products (AGEs) are irreversibly produced and accumulated in the organism in an age- and disease-dependent manner. Fructose, along with various glycation products—especially AGEs—are present in relatively high concentrations in our daily diet. Both endogenous and exogenous AGEs exhibit a wide range of biological effects, mechanisms of which can be associated with following: (1) AGEs are efficient sources of reactive species in vivo, and therefore can propagate nonenzymatic vicious cycles and amplify glycation; and (2) AGEs contribute to upregulation of the specific receptor for AGEs (RAGE), amplifying RAGE-mediated signaling related to inflammation, metabolic disorders, chronic diseases, and aging. Therefore, downregulation of the AGE-RAGE axis appears to be a promising approach for attenuating disease conditions associated with RAGE-mediated inflammation. Importantly, RAGE is not specific only to AGEs; it can bind multiple ligands, initiating a complex RAGE signaling network that is not fully understood. Maintaining an appropriate balance between various RAGE isoforms with different functions is also crucial. In this context, mild approaches related to lifestyle—such as diet optimization, consuming functional foods, intake of probiotics, and regular moderate physical activity—are valuable due to their beneficial effects and their ability to mildly modulate the fructose-mediated AGE-RAGE axis.
Introduction
Vital processes in living organisms are closely associated with reactive species, which represent a very diverse group from the point of view of both their chemistry and biological impact. These include molecules, atoms, and ions. Some of them belong to a family known as highly reactive free radicals. In aerobic organisms, many reactive species derive from molecular oxygen, some of them are nitrogen- and sulfur-containing, or have other chemical features, for example reactive carbonyl group(s). Although reactive species can be produced endogenously, for instance, as side products of enzymatic metabolism, they are often involved in nonenzymatic reactions (1–4). Unlike enzymatic reactions, which are precisely controlled by respective enzymes, nonenzymatic processes are rather unspecific and occur spontaneously. Generally, nonenzymatic events and reactive species are well-known for playing a detrimental role associated with health risks. On the other hand, being an important part of immune response, regulators of gene expression, and cellular signaling messengers, reactive species at low concentrations can have beneficial effects (5–9).
Historically, most studies in the field of reactive species and nonenzymatic processes in living organisms were focused on free radical oxidation and glycation, related to reactive oxygen species (ROS) and reactive carbonyl species (RCS) in particular. Increased levels of ROS and RCS lead to development of oxidative and carbonyl stresses, respectively. Obviously, both nonenzymatic processes mentioned above are closely linked to each other—oxidation reactions and ROS are found to be involved in certain stages of glycation, at the same time glycation itself is a source of ROS and ROS-mediated reactions (10–12). To reflect the combinational impact of nonenzymatic oxidation and glycation, the term “glycoxidation” has been introduced and is commonly used (13, 14). In addition, another nonenzymatic process such as ROS-induced lipid peroxidation results in a variety of RCS and respective glycation products, demonstrating an interplay between oxidative and carbonyl stresses. Glycoxidation leads to irreversible modifications of biomolecules and damages to cellular constituents with the consequent formation of poorly degraded compounds, collectively named advanced glycation end products (AGEs). Elevated levels of AGEs initiate activation of the specific receptor for AGEs (RAGE), mediating and amplifying inflammatory events related to metabolic disorders, chronic diseases, and aging (4, 15, 16). In this regard, inhibiting the AGE-RAGE axis is considered as a logical target in pathological conditions.
Reducing carbohydrates are major contributors to glycation process under physiological conditions. Among other monosaccharides, fructose has been found to be one of the most potent glycation agents in vivo (17–20). Numerous studies have demonstrated adverse metabolic changes in humans and animals as deleterious side effects of long-term intake of excessive fructose. These effects could be attributed to fructose-mediated glycation, increased levels of RCS and ROS, AGE accumulation, and subsequent activation of RAGE (18, 21–26).
Importantly, there is compelling evidence that short-term application of moderate doses of fructose can have beneficial defensive effects under certain pathophysiological conditions, particularly those associated with oxidative/carbonyl stress (21, 23, 27–30). Therefore, strategies such as diet optimization—limiting added sugars, and fructose in particular—could effectively prevent overactivation of RAGE signaling, and thereby mitigate the detrimental impacts of fructose. Other mild approaches may also offer moderate modulation of the fructose-mediated AGE-RAGE axis.
Fructose-initiated glycation and endogenous/exogenous glycotoxins
The initial step of fructose-initiated glycation (Figure 1) is a covalent interaction between electrophilic carbonyl group of open-chain fructose and nucleophilic functional group of a biomolecule. This interaction produces a diverse group of early glycation products (Schiff bases and Heyns products). Schiff bases are relatively unstable compounds that can be subjected to further isomerization (Heyns rearrangement) and formation of more stable Heyns adducts. Amadori products derived from glucose are also belong to early glycation products. The fructose moiety of the Heyns products can undergo enolization followed by dehydration, oxidation, and/or fragmentation reactions, consequently producing a variety of reactive species (10, 13, 31).
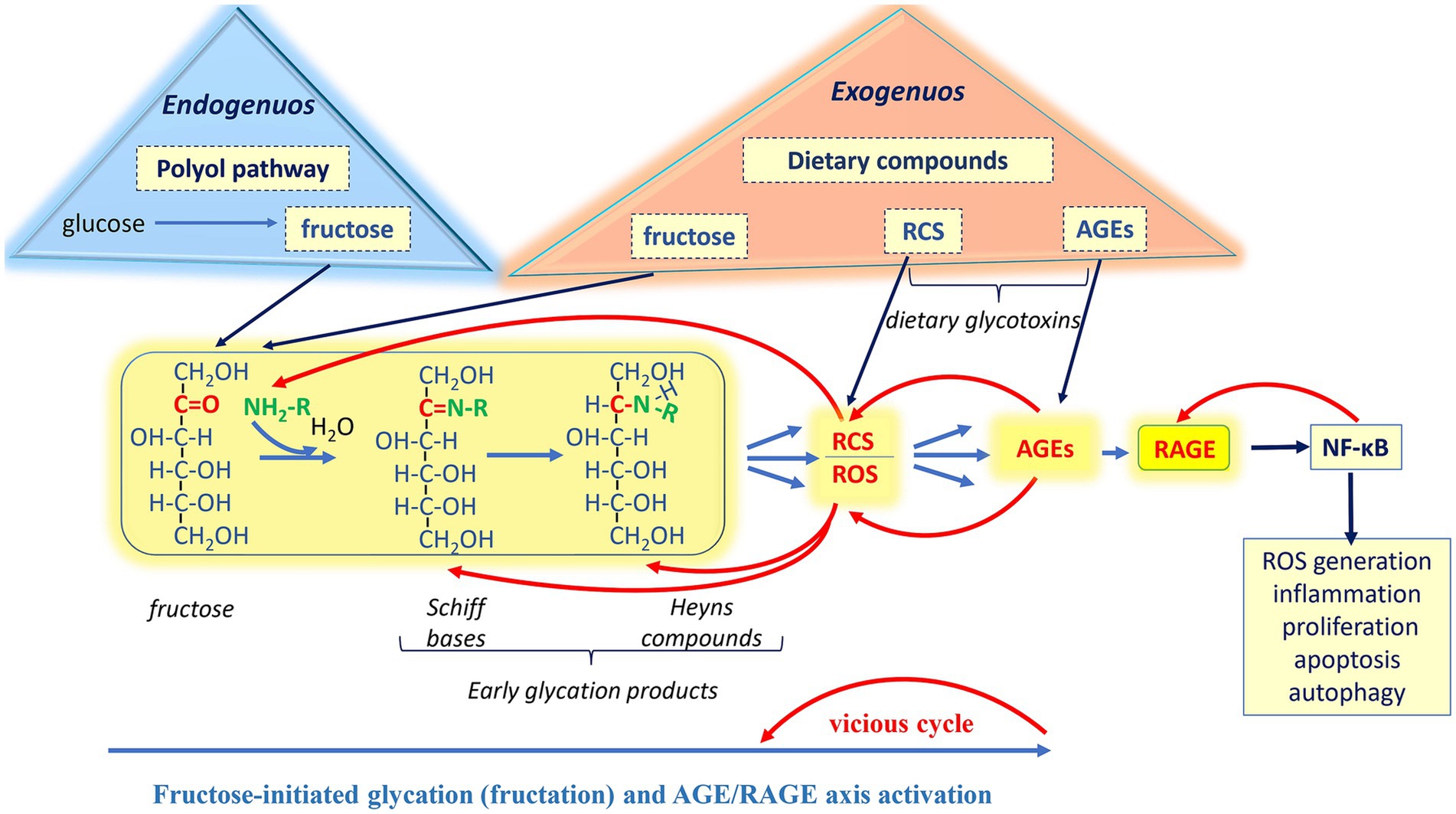
Figure 1. Involvement of exogenously and endogenously derived fructose in the generation of Reactive carbonyl species (RCS), Reactive oxygen species (ROS), and Advanced glycation end products (AGEs), followed by the activation of the Receptor for advanced glycation end products (RAGE). Nonenzymatic reactions: Fructose-initiated glycation. The initial step of the glycation is a covalent interaction between the electrophilic carbonyl group of open-chain fructose and the nucleophilic functional group of a biomolecule. This interaction produces a diverse group of early glycation products (Schiff bases and Heyns products). Schiff bases can undergo further isomerization to form more stable Heyns adducts. The fructose moiety of the Heyns products can undergo enolization, followed by dehydration, oxidation, and/or fragmentation reactions, consequently producing a variety of reactive species. In the advanced stages of glycation, early products and reactive species further undergo complex of irreversible nonenzymatic reactions, ultimately producing a variety of AGEs. Enzymatic reactions: Under hyperglycemia, in the insulin-insensitive tissues, polyol pathway is responsible for converting glucose to fructose, which in turn may initiate glycation. Dietary fructose and glycation products: Most foods and beverages contain added fructose and dietary glycotoxins (RCS and AGEs) in substantive amounts as compared to their endogenous sources. Both exogenously and endogenously derived AGEs bind to the receptor RAGE, which is involved in a variety of biological processes. Stimulation of RAGE leads to activation of the transcription nuclear factor kappa-B (NF-κB), which is associated with an enhancement of prooxidative and proinflammatory responses. In turn, NF-κB increases RAGE expression, which again stimulates NF-κB, forming a vicious cycle. Upregulation of RAGE is associated with various normal physiological processes and pathologies.
Besides glycation, other nonenzymatic reactions contribute to the formation of reactive species. They are autoxidation of glucose, fructose and other monosaccharides (Wolff pathway), autoxidation of Amadori and Heyns compounds (Hodge pathway), and oxidative fragmentation of Schiff bases (Namiki pathway) (2, 11, 14, 32, 33). Some fructose-derived RCS have been identified in vivo (Figure 2), for example, glycolaldehyde and acrolein. Importantly, most biological damages caused by RCS are related to α-dicarbonyls (compounds with two adjacent reactive carbonyl groups), including glyoxal, methylglyoxal, 3-deoxyglucosone, 3-deoxyfructose. This is likely because these compounds are about 20,000-fold more reactive than fructose and other reducing carbohydrates (31, 34, 35).
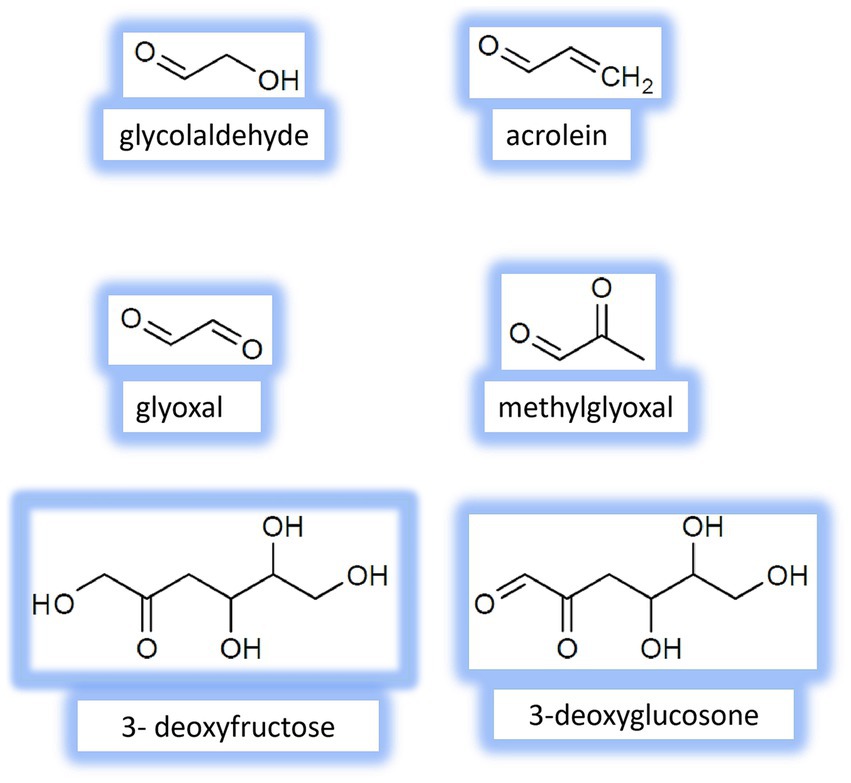
Figure 2. Chemical structure of the main fructose-derived Reactive carbonyl species (RCS) identified in vivo.
In the advanced stages of glycation, early products of the process and reactive species further undergo complex of parallel, sequential, and branched chains of irreversible nonenzymatic reactions, ultimately producing a variety of AGEs. Fructose-derived AGEs have been found in various tissues and peripheral blood. Common AGEs detected in vivo include pentosidine, glucosepane, argpyrimidine, methylglyoxal-lysine dimmer, glyoxal-lysine dimmer, carboxymethylcysteine, carboxymethylguanosine, carboxymethyllysine, glycolaldehyde-pyridine, 3-deoxyglucosone-lysine dimer, imidizalone, pyrraline, etc. (23, 25, 26). The chemical structures of some AGEs are presented in Figure 3. Generally, AGEs are highly heterogeneous group of compounds with different structures, sizes, and molecular weights. They exhibit various physico-chemical properties; for example, some of AGEs, like pentosidine, are fluorescent, while others, such as methylglyoxal- and glyoxal-lysine dimmers, are nonfluorescent. Also, AGEs may demonstrate different cross-linking abilities (14, 36).
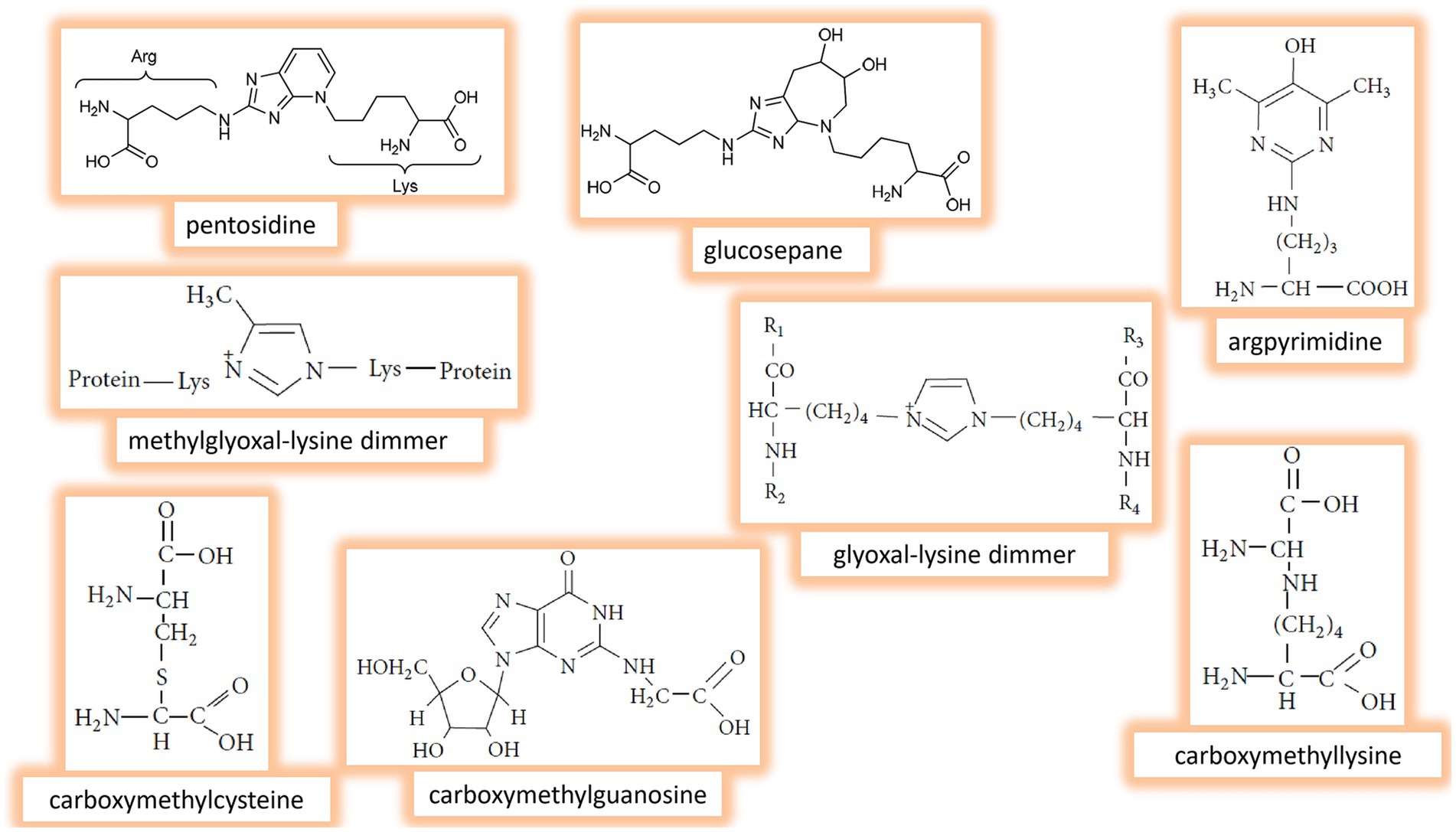
Figure 3. Chemical structure of the main fructose-derived Advanced glycation end products (AGEs) identified in vivo.
Formation of glycation products is a relatively slow process. In contrast to rapid generation of products in free-radical chain oxidation, AGE formation takes weeks, months, or even years. This could be explained by differences in average reactivities of RCS and ROS and their half-life times. While half-lives of many RCS range from minutes to hours, the most reactive ROS have half-life periods from 10−9 to 10−6 s (37–39). The slow and irreversible modification of biomolecules via glycation correlates with aging. A causative role of glycation in the aging process and age-associated pathologies has been suggested (40). As confirmation, AGE-modified albumin has been reported to be specifically recognized and degraded by macrophages in vitro. Therefore, AGEs have been supposed to serve as specific signals for the recognition and degradation of senescent macromolecules in vivo. On the other hand, incomplete removal of glycated proteins by macrophages was connected with aging (41). In fact, the free-radical theory of aging by Harman (42) and the glycation hypothesis of aging by Monnier and Cerami (40) are currently the most popular and widely accepted basis to explain molecular mechanisms of aging.
During glycation, the appearance of reactive species is an unavoidable step toward the formation of AGEs. In addition to this “main direction” (Figure 1, blue arrows), reactive carbonyls can return to the starting point and initiate new rounds of glycation, since RCS have at least one carbonyl group to interact with new biomolecules. It should be noted that most biomolecules are nucleophiles, as many of them, including proteins, amino lipids, nucleic acids, possess amino, sulfhydryl, guanidine or other nucleophilic functional groups. Thus, many different biomolecules are involved in the irreversible formation of AGEs. The latters, in turn generate both RCS and ROS (33), leading to new stages of glycoxidation, creating more and more vicious nonenzymatic chemical cycles (Figure 1, red arrows).
In addition to nonenzymatic processes, normal enzymatic metabolism can also produce reactive species, including RCS. Under physiological conditions, up to 0.4% of glycolytic intermediates can be converted to methylglyoxal, a side product of glycolysis (43). Under other circumstances, reactive carbonyls are generated enzymatically as necessary products. In activated neutrophils, for example, some amino acids are found to be oxidized to different RCS, that demonstrates particular importance of reactive carbonyls in the immune response (44–46). Also, enzymatically produced RCS are involved in regulating gene expression and signaling networks (5, 7, 39). It is important to note that reactive species play a beneficial role only at their low steady-state concentrations, which are tightly controlled by appropriate enzymes in the organism.
Nonetheless, some enzymatic pathways can intensify RCS production to relatively high levels, when reactive carbonyls demonstrate detrimental effects. For example, the polyol pathway, which is responsible for converting glucose to fructose, increases under hyperglycemia in the insulin-insensitive tissues. As mentioned, fructose itself may initiate glycation and be converted to RCS. In both humans and animal models, increased fructose and enhanced flux of polyols have been suggested as metabolic basis for different pathologies (47, 48).
Importantly, high fructose diet, as a source of exogenous fructose, is clearly related to deleterious side effects, including metabolic syndrome, kidney and heart diseases, insulin resistance and type 2 diabetes, nonalcoholic fatty liver disease, arthritis, dementia and neurodegenerative disorders. Glycation is believed to contribute significantly to the fructose-mediated pathogenesis. As confirmation, chronic exposure to high exogenous fructose has been observed to stimulate RCS/ROS formation and AGE accumulation in various in vitro and in vivo experimental models (17, 22, 32, 49–51). Moreover, excessive fructose and glycation products can alter gut microbiota composition, potentially leading to inflammatory events. Overconsumption of fructose (above 50 g per day) is one of the major factors contributing to chronic inflammation and related diseases (20, 52).
Conversely, epidemiological, clinical, and experimental studies suggest that fructose intake of less than 50 g per day is a moderate dose that may have no detrimental influence (53–56). Moreover, clinical investigations evidenced that small doses of fructose (≤10 g/meal) significantly decreased the postprandial glycemic response to high glycemic index foods and demonstrated beneficial effect on the levels of HbA1c and triglycerides, as well as improved blood pressure and body mass index (53, 57–60). In addition, the Dietary Guidelines for Americans recommend limiting calories from added sugars to less than 10% of total daily calories (61). This recommendation corresponds well to the mentioned above moderate doses of fructose.
According to Marriott and colleagues (62), the highest mean percentage of added fructose intake is associated to the consumption of nonalcoholic beverages (54.3%), grain products (20.3%), and sweets (12.1%). Also, the highest mean percentage of total fructose consumption is linked to nonalcoholic beverages (46.0%), grain products (17.3%), fruits and fruit products (13.4%), sweets (10.3%), milk and milk products (7.1%), and vegetables and vegetable products (2.7%).
Most foods contain reactive carbonyls and AGEs, in fact, in substantive amounts as compared to their endogenous sources, making them a major exogenous source of glycation products, known as dietary glycotoxins (61–67). For example, the amount of ingested Heyns products (calculated as fructoselysine) per day ranges between 500 and 1,200 mg, while the quantity of dietary AGEs (mainly pyrraline and carboxymethyllysine) ranges from 25 to 75 mg (68). High-fructose corn syrup, a widely used sweetener in many foods and beverages, is the major source of such RCS as 3-deoxyglucosone, glyoxal, and methylglyoxal (69–71). Consequently, the Western diet, which is rich in processed and sweetened foods, is strongly associated with a high intake of dietary glycotoxins.
Several comprehensive works demonstrate the content of AGEs in commonly consumed foods, providing a solid foundation for creating dietary AGE database (67, 72–74). Unfortunately, there are no standardized conventional methods for measuring dietary AGEs and other glycotoxins, which limits inter-laboratory comparisons of the data obtained. Nevertheless, the AGE levels in common foods are examined and some studies report how AGE content varies by food category and cooking method. It has been shown that total amounts of AGEs were high in processed nuts, bakery products, and certain types of meats and cereals (>150 mg/kg), while AGE content is rather low in dairy products, vegetables, and fruits (< 40 mg/kg) (73).
In fact, AGEs are unavoidable components of our daily meals, as they are present in natural, uncooked foods. However, processed foods—especially those high in fat, sugar, and protein (Western diet)—tend to be particularly rich in AGEs. Any food processing increases AGE content in regime-dependent manner during cooking. It has been well demonstrated that frying, broiling, grilling, and roasting substantially accelerated dietary AGE formation, whereas foods cooked by steaming, stewing, poaching, or boiling had relatively low amounts of AGEs. For example, grilled, boiled, and raw carrots had approximately 220, 25, and 10 AGE kU/100 g, respectively. At the same time, fried, poached, and raw chicken contained about 7,000, 1,000, and 800 AGE kU/100 g (67).
Consumption of AGE-rich diet, in which the amounts of AGEs were 3-fold higher than in a regular diet, has been shown to elevate blood concentration of AGEs by about 1.5-fold (75). It has been also observed that up to 30% of dietary AGEs were absorbed in human organism, two-thirds of the absorbed AGEs remained in the body and one-third was excreted during the next 3 days (75–77). Interestingly, the comparison of AGE concentrations in plasma of vegetarian and omnivorous individuals presented significantly higher (by 25%) AGE levels in vegetarians. In comparison with the omnivores, vegans, lacto-ovo-vegetarians, and semi-vegetarians demonstrated the levels of some AGEs higher by 15%, 32%, and 22%, respectively (18, 78).
As noted, some biological effects of AGEs result from their involvement in generating RCS/ROS (Figure 1). An increase in the levels of reactive species leads, in turn, to chronic carbonyl/oxidative stress development and modification of biomolecules with consequent loss of their functions. Also, ingested AGEs elicit toxicological effects by disrupting gut microbiome and immune homeostasis (79, 80). Both exogenously and endogenously derived AGEs are responsible for modulating certain signaling pathways. In particular, they bind to RAGE (Figure 1) and activate the RAGE-downstream pathway, which is involved in a variety of biological processes from proliferation and inflammation to apoptosis and autophagy (15, 20, 81). Upregulation of RAGE is known mostly for playing a detrimental role associated with various pathologies.
Multifaceted RAGE
The Receptor for AGEs (RAGE) is a member of the immunoglobulin superfamily of cell-surface molecules. Originally purified from bovine lung endothelial cells (82, 83), RAGE is now known to be an ubiquitous receptor present in a variety of both immune and nonimmune cells (leucocytes, monocytes, macrophages, pericytes, and endothelial cells, etc.). RAGE is found in various organs and tissues such as the heart, liver, lungs, kidneys, skeletal muscles, and, the cardiovascular and nervous systems (84). Besides AGEs, RAGE recognizes a number of other ligands: (1) damage-associated molecular pattern molecules (DAMPs)-amyloid-β peptides (Aβ), β-sheet fibrils, high mobility group box 1 (HMGB1), and S100/calgranulinprotein; (2) pathogen-associated molecular pattern (PAMP) lipopolysaccharide (LPS); and (3) DAMP/PAMP DNA and RNA species (15, 85, 86). The above demonstrates that RAGE is the multiligand receptor, which is able to bind various compounds with different structures, sizes, functions, stimulating thereby multiple downstream signaling cascades.
What molecular mechanisms enable RAGE to recognize such a wide range of ligands and contribute to its diverse bioeffects? Structural studies reveal that full-length human RAGE has three domains (Figure 4): an extracellular part responsible for ligand binding, a hydrophobic transmembrane spanning region, and a highly charged cytoplasmic tail, which is essential for RAGE ligand-mediated signal transduction (83, 87).
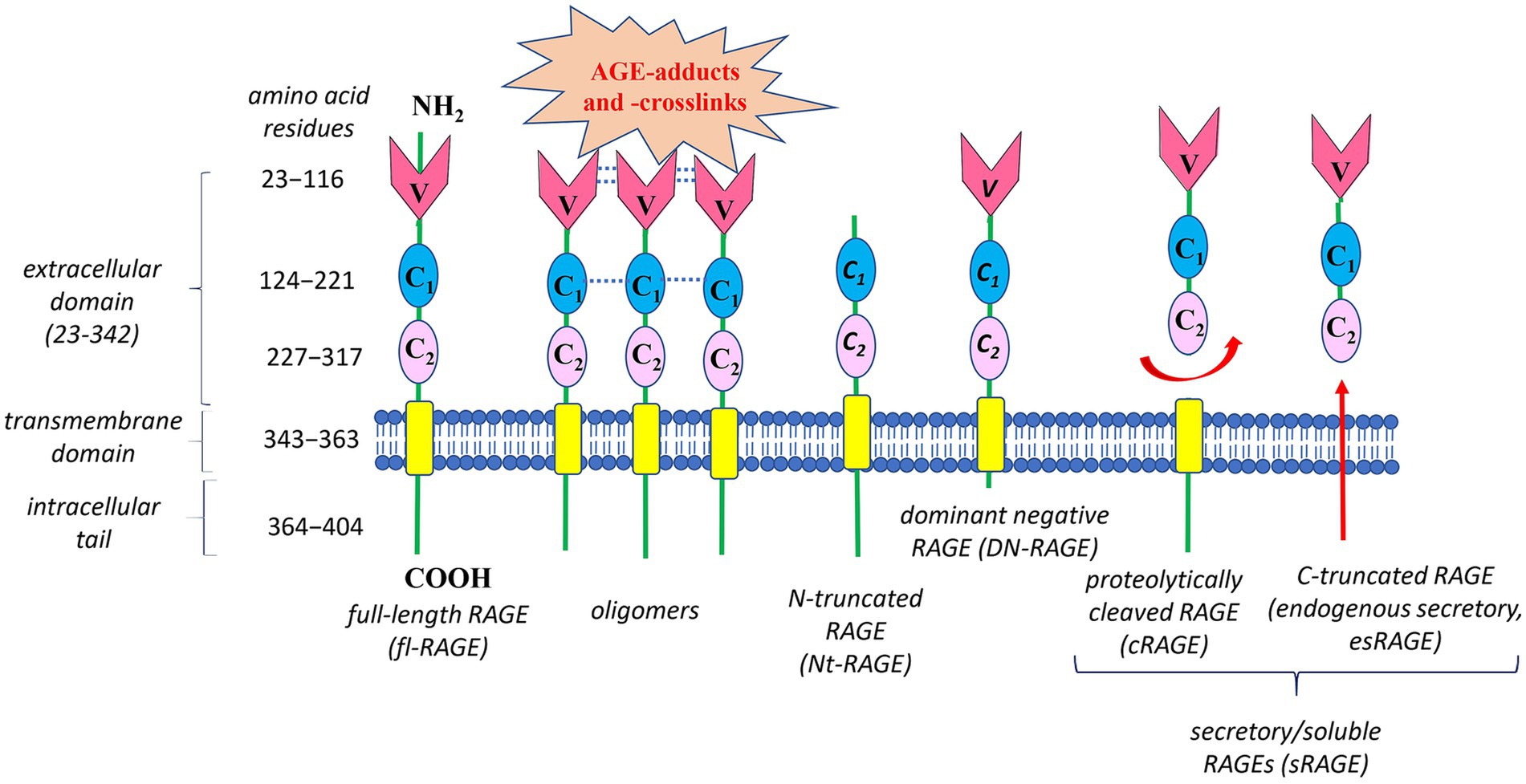
Figure 4. Structure of the human Receptor for advanced glycation end products (RAGE) and its main isoforms. Human RAGE has three domains: (1) an extracellular part responsible for ligand binding, consisting of three immunoglobulin-like parts – a variable N-terminal V-type domain followed by two constant C1- and C2-type domains; (2) a hydrophobic transmembrane region responsible for anchoring on the membrane; and (3) a cytoplasmic tail required for RAGE ligand-mediated signal transduction. Oligomerization of RAGE and its ligands is an important mechanism for controlling AGE-RAGE interplay. Oligomeric ligands can initiate self-association of the V-V/C1-C1 regions in RAGE, forming stable VC1-ligand complex with increased affinity to RAGE ligands, in particular AGE-adducts and AGE-crosslink. The best-studied RAGE isoforms are full-length RAGE (fl-RAGE), N-truncated RAGE (Nt-RAGE), dominant negative RAGE (DN-RAGE), and two soluble/secretory forms (sRAGE)—proteolytically cleaved RAGE (cRAGE) and C-truncated RAGE (endogenous secretory RAGE, esRAGE). Deletion of the V-type domain transforms fl-RAGE into the Nt-RAGE variant, which is a membrane-bound protein like fl-RAGE, but lacking the ability to bind AGEs. The DN-RAGE form has the extracellular (V- and C-type regions) and transmembrane domains like fl-RAGE, but lacks the intracellular cytoplasmic domain required for initiating downstream signaling. Circulating sRAGE has no the intracellular signaling tail and transmembrane domain. sRAGE is a heterogeneous group of proteins resulting from either proteolytic cleavage of fl-RAGE from the cell surface (cRAGE) or alternative splicing of fl-RAGE mRNA (esRAGE).
The extracellular fragment of RAGE consists of three immunoglobulin-like parts: a variable N-terminal V-type domain, followed by two constant C1- and C2-type domains. The V-type domain is essential for ligand binding. Interestingly, both in vitro and in vivo studies report that V-type and the adjacent C1-type domains are able to form structural and functional VC1 tandem unit with multiple binding sites to recognize numerous different RAGE ligands (87–89). These ligands interact with positively charged VC1 complex in a heterogeneous manner and, at first sight, they have no significant structural similarity. Nevertheless, a diverse range of RAGE ligands have at least one common feature – their acidic nature (negative charge) (90), though hydrophobicity-dependent mechanisms for RAGE-ligands interaction are also important (91).
In addition to the mentioned above, oligomerization of either RAGE or its ligands is one more key mechanism, controlling AGE-RAGE interplay (90). For example, oligomeric ligands can initiate self-association of the V-V/C1-C1regions in RAGE (Figure 4), forming relatively stable VC1-ligand complex with increased affinity to a diverse spectrum of ligands, including AGE-adducts and AGE-crosslinks. Also, such clustering shifts the equilibrium between monomer/oligomeric states of RAGE and respective ligands. This creates a positive feed-back loop and amplifies the RAGE ligand-mediated signal transduction. Under normal physiological conditions, RAGE expression is rather low. In contrast, during chronic inflammation RAGE is upregulated due to elevation of its ligand levels and the amplifying mechanisms of RAGE activation (92, 93). Generally, increased levels of AGEs, along with oligomerization and activation of RAGE, may explain why the RAGE signaling pathway is highly activated in various inflammation-related pathologies.
Obviously, the oligomerization is not the only mechanism underlying AGE-RAGE axis operation. Due to alternative splicing and metalloprotease-mediated cleavage, several variants of human RAGE appear, which regulate downstream pathways (84, 93). Figure 4 demonstrates the structures of the best-characterized RAGE isoforms: full-length RAGE (fl-RAGE), N-truncated RAGE (Nt-RAGE), dominant negative RAGE (DN-RAGE), and two soluble/secretory forms (sRAGE)—proteolytically cleaved RAGE (cRAGE) and C-truncated RAGE (endogenous secretory, esRAGE).
Deletion of the V-type domain transforms fl-RAGE into the Nt-RAGE variant, which is membrane-bound protein like fl-RAGE, but lacking the ability to bind AGEs. The Nt-RAGE protein is suggested to be involved in the control of angiogenesis by regulating AGE-independent migration of endothelial cells (94). Overexpression of Nt-RAGE may contribute to pathological events independently on the V-type domain.
The DN-RAGE form has the extracellular (V- and C-type regions) and transmembrane domains like fl-RAGE, but lacks the intracellular cytoplasmic domain required for initiating downstream signaling. Consequently, DN-RAGE binds AGE ligands, forming complexes that are not signaling-competent. Moreover, DN-RAGE can suppress RAGE-induced signaling by clustering with fl-RAGE and formation of hetero-complexes able to bind extracellular ligands without transducing signals into the cell. It is highly possible, that under certain conditions, DN-RAGE formation serves as an important mechanism to attenuate of fl-RAGE-induced signaling. For example, DN-RAGE has been found to inhibit tumor cell proliferation and invasion in vitro and tumorigenesis in vivo (95). Under normal physiological conditions, the level of DN-RAGE expression is similar to that of fl-RAGE (96).
Similar to DN-RAGE, sRAGE has no intracellular signaling tail, but also does not have the transmembrane domain, thus it is circulating protein. sRAGE is a heterogeneous group of proteins, resulting from either alternative splicing of fl-RAGE mRNA (esRAGE) or proteolytic cleavage of fl-RAGE from the cell surface (cRAGE) (84, 93). A unique 16-amino-acid segment in the C2 domain distinguishes esRAGE from cRAGE. The serum level of cRAGE is 5-fold higher than that of esRAGE in healthy individuals (97). Both sRAGE forms are believed to be competitive inhibitors of RAGE. By possessing the same AGE-binding ability as fl-RAGE, sRAGE acts as a “decoy” receptor, preventing circulating AGEs from interacting with RAGE (98), and thus providing cytoprotective effects against the AGE-RAGE complex negative impact. In addition, similar to DN-RAGE, esRAGE can interact with fl-RAGE, leading to the formation of hetero-complexes, which can bind extracellular ligands, but without transducing a signal into the cell.
Overall, understanding how various fructose-derived AGEs interact with multifaceted RAGE opens numerous ways to influence both the normal physiological and pathological events in the organism. Growing evidence supports a dual role of fructose itself, fructose-derived glycation products, and RAGE (21, 23, 93, 99–101). However, the AGE-RAGE axis is primarily known for its adverse effects on cellular metabolism and function through inflammation and nonenzymatic processes. For instance, the AGE-RAGE signaling pathway is believed to induce the expression of proinflammatory modulators (IL-6, TNF-α, IFN-γ) via activation of the transcription nuclear factor kappa-B (NF-κB) (15). Also, NF-κB increases RAGE expression, which in turn again stimulates NF-κB (88), creating a vicious cycle and generating more and more reactive species in new rounds (Figure 1). Additionally, RAGE expression induces NADPH oxidase-dependent generation of superoxide anion-radical and hydrogen peroxide (15), which contribute to the production of new AGEs as additional RAGE ligands. Overexpression of RAGE has been observed in various pathologies across a wide range of cells, including hepatocytes, cardiomyocytes, neuronal cells, vascular endothelial cells, and renal cells. Upregulation of RAGE was also found in such immune cells as neutrophils, monocytes/macrophages, lymphocytes, dendritic cells, where its activation resulted in the induction of expression of NF-κB-regulated genes encoding proinflammatory cytokines, chemokines, and adhesion molecules (15, 102–104).
Approaches for mild modulation of the AGE-RAGE axis
At least the aforementioned points suggest that attenuation of the AGE-RAGE signaling could be a beneficial approach to preventing fructose-mediated disturbances. While fructose is a valuable, healthy, and safe nutrient at moderate intake levels (≤50 g/day or < 10% of total calories per day) (53–56), long-term consumption of excessive fructose and elevated endogenous its production have been shown to have detrimental impacts (19–23, 47, 48). There several potential targets within the pathway from fructose to active RAGE that could be considered for modulating the fructose-initiated AGE-RAGE axis (Figure 5, red arrows 1–7). These targets to some extension may serve as “stepping stones” in the following strategies: (1) prevention of RCS/AGE formation, (2) reduction of RCS/AGE levels, and (3) suppression of RAGE-mediated signaling.
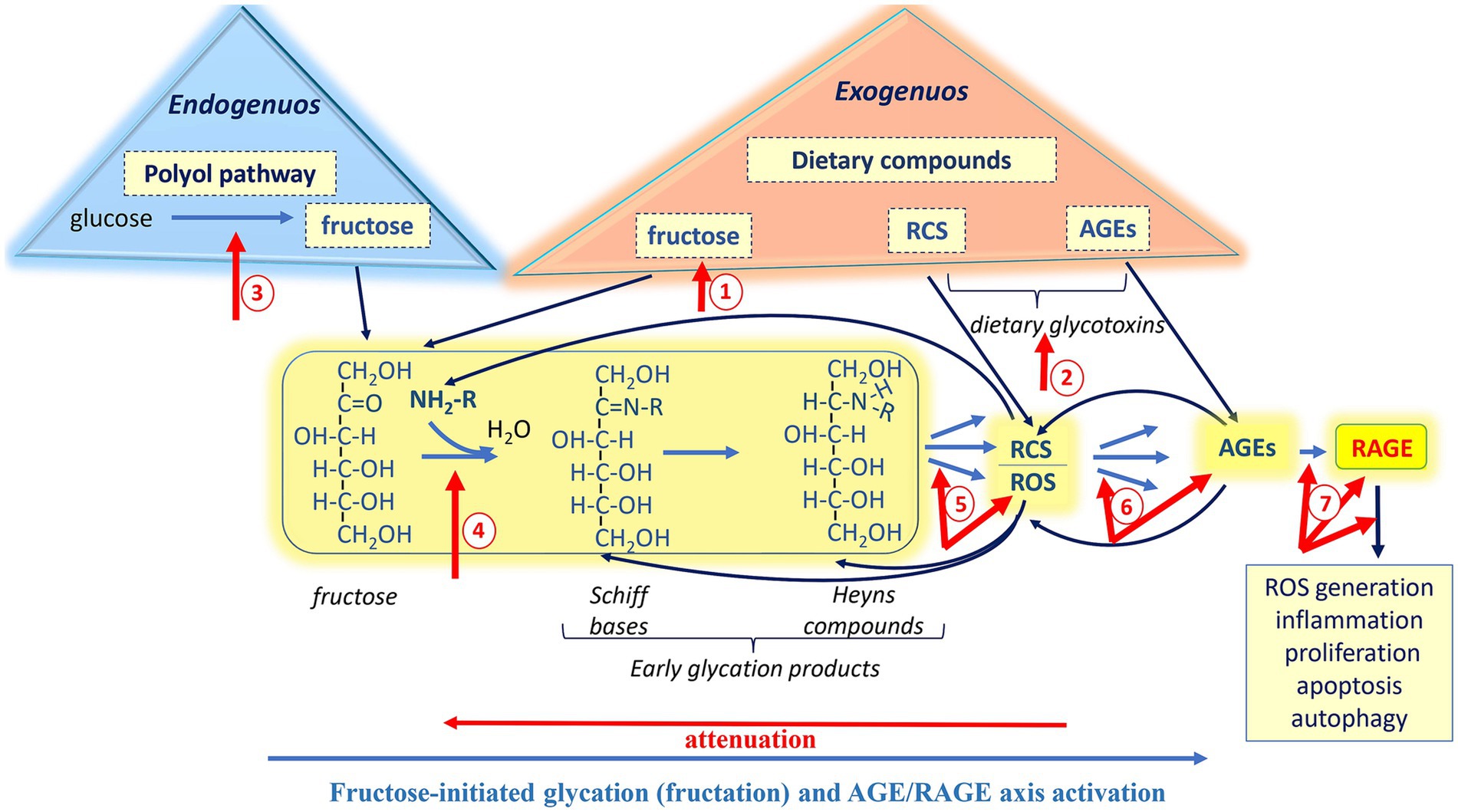
Figure 5. Potential targets іn the pathway from fructose to RAGE for mild modulation of the AGE-RAGE axis. The strategies toward the modulation of fructose-initiated AGE-RAGE axis include: (1) prevention of RCS/AGE formation (red arrows 1–4); (2) reduction of RCS/AGE levels (red arrows 4–6); and (3) suppression of RAGE-mediated signaling (red arrows 7). Mild approaches such as diet optimization, utilization of functional foods, proper intake of probiotics, and regular moderate physical training may provide moderate control of the fructose-mediated AGE-RAGE axis and help manage the adverse effects associated with its overactivation.
Most importantly, the human organism itself possesses a highly developed and tightly regulated antiglycoxidation system that operates at various levels to protect against the adverse effects of AGE-RAGE axis overactivation. Also, it should be kept in mind that strategies to counteract the detrimental impacts of RAGE signaling should preserve beneficial effects of RAGE. In this regard, it makes sense to apply mild approaches to modulate the axis.
Strategy #1 (prevention of RCS/AGE formation)
It is well documented that limiting added sugars, and fructose in particular (Figure 5, red arrow 1), as well as dietary glycotoxins (RCS and AGEs; Figure 5, red arrow 2) has a beneficial impact on health (72, 105–109). For example, fructose- and glycotoxin-restricted diet increases insulin sensitivity, improves renal failure, and reduces cardiovascular risk. Obviously, fructose, RCS, and AGEs are inevitable constituents of our daily diet, therefore completely avoiding them is practically impossible. Nevertheless, reducing the intake of fructose and glycotoxins seems to be an effective strategy for mitigating the adverse health effects associated with the AGE-RAGE axis.
To decrease carbohydrate consumption and/or lower elevated blood glucose levels, some researchers propose substituting carbohydrates with ketone bodies. The latters are substantial energy substrates and can effectively replace carbohydrate requirements under certain circumstances (110). In fact, the level of ketone bodies increases with the consumption of a high fat/low carbohydrate diet, commonly known as a ketogenic diet. However, at high amounts, fats undergo lipid peroxidation, producing both RCS and ROS. Moreover, at high concentrations, ketones can be damaging due to their reactive carbonyl groups. Therefore, ketogenic diet must be well-balanced and optimized. Under chronic hyperglycemia, excessive glucose enters the polyol pathway, where it can be converted to fructose with the consequent detrimental effects. Thus, it is believed that inhibition of aldose reductase, the key enzyme of the polyol pathway (Figure 5, red arrow 3), may be beneficial (111–113), especially regarding the strategy discussed. Natural inhibitors of aldose reductase have been tested in many studies. Generally, such phytochemicals as alkaloids, phenolic compounds, coumarins seem to be promising (114, 115).
Naturally occurring compounds and their synthetic analogs exhibit good inhibitory activity against glycation and RCS/AGE formation (Figure 5, red arrow 4). Many of them are ubiquitous nutrients, some of which belong to plant or fungal secondary metabolites: polyphenols (e.g., ferulic, gallic and caffeic acids, curcumin, resveratrol), vitamins (e.g., thiamin, pyridoxamine), isothiocyanates (e.g., sulforaphane), alkaloids (e.g., berberine), and statins (35, 116–123). Among compounds with antiglycation activity are peptides such as glutathione and carnosine (32, 124–128). Interestingly, besides antiglycation activity, some compounds are powerful antioxidants and demonstrate inhibitory effects on aldose reductase (129), thereby affecting multiple points in the pathway from fructose to active RAGE.
Additionally, some evidence suggests that optimizing diet, proper intake of probiotics, and moderate physical exercise can be efficient tools to maintain gut microbiome, which has been shown to reduce dietary AGE absorption, modulate AGE levels and sRAGE expression (66, 130–132), and thus have a beneficial impact on human health.
Strategy #2 (reduction of RCS/AGE levels)
Under normal conditions, the multilevel and complex antiglycation system is effective enough at both preventing the formation (Figure 5, red arrows 4, 5 and 6) and reducing the amount of reactive carbonyls and AGEs (Figure 5, red arrows 5 and 6). The following defensive enzymes found to play a key role in the organism protection against glycoxidation: aldo- and keto-reductases, aldehyde and alcohol dehydrogenases, carbonyl reductases, amadoriases (deglycases), cytochromes P450 family, glutathione reductase, glucose-6-phosphate dehydrogenase, glutathione-S-transferases and glutathione-dependent glyoxalase system (2, 14, 133, 134). In fact, many of these enzymes are associated with important antiglycation agents such as reduced glutathione and reduced equivalents NADPH or NADH. Thus, the defensive system includes both high- and low-molecular-weight components. It is worth noting that many of the antiglycation enzymes, along with their low-molecular-weight “assistants,” also belong to the antioxidant system. This again highlights the interplay between glycation and nonenzymatic oxidation.
Common factors and pathways regulating defensive enzymes also demonstrate a close relationship between carbonyl and oxidative stresses. For instance, sulforaphane effectively prevents AGE formation through the Nrf2 activation that in turn induces glyoxalase 1, the key enzyme of glyoxalase system that detoxifies α-dicarbonyl compounds (135–137). Besides glyoxalase 1, among numerous targets of the Nrf2 transcription factor are both antioxidant and antiglycation enzymes: superoxide dismutase, catalase, glutathione peroxidase, peroxiredoxin, thioredoxin reductase, 𝛾-glutamylcysteine synthase, glutathione reductase, thioredoxine reductase, glutathione S-transferases, glutathione reductase (2, 8). In addition, sulforaphane has recently been found to modulate immune response by inhibiting the NF-κB and mitogen-activated protein kinase (MAPK) signaling pathways (138). Similar to sulforaphane, resveratrol, ellagitannins, oleanolic and ascorbic acids also protect cells and tissues against damaging by either oxidants or glycation agents through Nrf2-depending signaling (104, 139–141).
It is well demonstrated that widely used biguanide drug metformin, known for reducing blood glucose levels, also demonstrates the inhibitory effect at the early, intermediate, and late stages of glycation, and induces glyoxalase I activity, thereby reducing the level of α-dicarbonyl compounds and AGEs (120, 142, 143). In vitro and in vivo experiments have demonstrated that metformin reversed methylglyoxal-induced downregulation of Nrf2, and therefore prevented methylglyoxal-initiated damage, apoptosis, and inflammation (144).
Reduced glutathione, carnosine, and aminoguanidine also act as scavengers of α-dicarbonyls and other RCS (32, 117, 128). In addition, glutathione and carnosine are well-known antioxidants that prevent the formation of advanced products of nonenzymatic oxidation and glycation (32, 125–128).
Together with the mentioned above defensive mechanisms, lysosomal and proteasomal systems are also important (15, 111), since to some extent, they are involved in the clearance of poorly degraded AGEs (Figure 5, red arrows 6). Unfortunately, both protective systems decrease with age and under pathologies. Elevated nonenzymatic oxidation and/or glycation can inhibit proteolytic activity of the proteasome pathway due to the accumulation of damaged proteins that inhibit proteasomes (133, 145). Interestingly, some studies demonstrate a positive correlation between regular moderate exercise and activity of glyoxalase 1, as well as lysosomal and proteasomal systems (72, 146).
Strategy #3 (suppression of RAGE-mediated signaling)
Inhibition of RAGE could also be applied toward the modulation of AGE-RAGE axis (Figure 5, red arrows 7). Regarding this strategy, using inhibitors of either RAGE or its downstream targets, as well as RAGE antagonists, appear to be the most effective approaches.
Various compounds have been tested both in vitro and in vivo to block the AGE binding sites of RAGE and to act as competitive inhibitors of RAGE interaction with intracellular effectors nessesary for signal transduction (91, 147, 148). Many of synthetic substances demonstrate effective inhibition of RAGE-dependent signaling and consequent molecular events. Nonetheless, supporters of mild approaches prompt to modulate AGE-RAGE pathway by using natural compounds instead of artificial inhibitors, as the latter may cause side effects.
An increasing number of recent studies demonstrate that bioactive compounds derived from different natural sources effectively block AGE-RAGE formation and reduce RAGE signaling (119, 149, 150). For example, scalarin, a marine product, inhibits RAGE (151) and apigenin, a natural flavone, downregulates RAGE/NF-κB expression (152). The mentioned above food-derived scavengers of RCS/AGEs, and in particular sulforaphane, resveratrol, and curcumin, can also downregulate RAGE expression. Sulforaphane reduces expression of RAGE and effectively suppresses the RAGE-depending JNK and MAPK signaling cascades, which in turn inhibits NF-κB pathway and the production of pro-inflammatory cytokines (35, 136, 138, 153, 154).
Interestingly, a recent study reported that ascorbic acid significantly reduced the level of circulating HMGB1 (high mobility group box 1), a ligand of RAGE (141). Similarly, oleanolic acid demonstrated an inhibitory effect on HMGB1 release (139). Therefore, we suggest that such ubiquitous compounds as ascorbic and oleanolic acids also lead to mild reduction of RAGE activity by decreasing the concentration of ligands for RAGE other than AGEs. Moreover, as effective antioxidants, both acids can break glycation-oxidation vicious cycle that leads to AGE formation. In this regard, compounds such as sulforaphane, as well as ascorbic and oleanolic acids, that can control RAGE-mediated events at various points in the glycation-RAGE network, are valuable and promising bioactive components of functional foods for protecting against inflammation, aging and related disorders.
Physical training is another attractive approach for moderate modulation of AGE-RAGE axis. Indeed, in animals studies, exercise has been shown to attenuate the impact of glycation by elevated activity of glyoxalase 1 with simultaneous reduced activity of aldose reductase and decreased total RCS/AGE levels (155). Also, rergular moderate exercise has been reported to decrease the expression of the RAGE/NF-κB pathway (156). However, human studies often demonstrate contradictory results. As observed, the expression of RAGE, and sRAGE in particular, can vary significantly depending very much on the type and intensity of physical activity as well as the physiological state of the individuals.
Growing body of research reveals that circulating soluble sRAGE acts as “decoy” for RAGE ligands and can bind to AGEs but does not initiate intracellular signal transduction cascade; thereby upregulation of sRAGE is suggested to elicit beneficial protective effects via reduction of the AGE-RAGE interaction. Animal studies have shown that increasing sRAGE levels can prevent RAGE-mediated dysfunctions and even reverse some detrimental RAGE-related consequences (157–161). These findings confirm that elevating sRAGE could be an effective approach toward the modulating immune system and treating inflammatory diseases. In this regard, numerous studies look for relationship between human lifestyle improvement and sRAGE increase.
However, the impact of physical activity on sRAGE levels is complicated. Some studies reported significant increases in serum sRAGE levels due to physical training, while others found no effect or even a decrease in sRAGE levels under certain conditions (132, 162–164). Combinational approach to improve diet and optimize physical activity also sometimes may have unpredictable effects on metabolic parameters (72, 162). Clearly, the effect of physical training on sRAGE levels depends on multiple factors, including the type, duration, and intensity of activity, as well as diet, lifestyle, age, and the individual’s physiological state.
Moreover, sRAGE is a heterogenous group that includes at least the cRAGE and esRAGE isoforms. The mechanisms responsible for the balancing these isoforms in different cases are not well understood. For example, cRAGE and esRAGE amounts differently change, dependently on the intensity of physical activity (163, 164). Interestingly, total sRAGE level, and cRAGE in particular, decline with age, while esRAGE level does not change during aging, suggesting different associations of sRAGE isoforms with age-related diseases (100).
Administration of sRAGE, either peripherally or directly to target organs, is one of the most attractive and intensively investigated approaches. Accumulating evidence has revealed that in animal models sRAGE administration was associated with reduction in the development of pathological conditions and the improvement in tissue functioning (165). However, some reports indicate that sRAGE elevation correlates with inflammatory conditions (166, 167). It is likely that benefits of sRAGE elevation might be offset by its disadvantages or vice versa, depending on the chronic or acute character of disorder, stages of disease, physiological state of organism, etc. More research is needed to fully understand the effects of sRAGE administration.
Conclusions and perspectives
Over recent decades, the impact of fructose on human health has attracted growing attention from the scientific community. The “fructose hypothesis” suggests that fructose plays a causative role in various health problems. Indeed, compelling evidence indicates that long-term intake of excessive fructose can have deleterious side effects, which are believed to be attributed to glycation and fructose-derived AGEs. It is well established that AGEs are involved in upregulation of the specific receptor for AGEs (RAGE), amplifying RAGE-mediated signaling related to inflammation, metabolic disorders, chronic diseases, and aging. In contrast, short-term consumption of low/moderate doses of fructose are found to be beneficial, as it contributes to decrease of blood pressure, body mass index, the levels of triglycerides and HbA1c, while also improving glucose tolerance. Fructose is a valuable nutrient under certain pathophysiological conditions, particularly due to inducing mild carbonyl/oxidative stress, which in turn stimulates protective mechanisms.
Recent advances in studying the AGE-RAGE axis have significantly extended our understanding of its crucial role in human health. Nevertheless, several key issues remain to be addressed. Unfortunately, there are no standardized conventional methods to measure dietary AGEs and other glycotoxins, which limits inter-laboratory comparisons of the data obtained. This limitation hinders developing a unified dietary AGE/glycotoxin database. Standardized methods also could help determine whether there are differences between fructose-derived AGEs and those generated by other dietary carbohydrates, such as glucose or galactose. Understanding these distinctions may provide insights into the specific characteristics of AGE-RAGE interactions.
Additional intriguing questions arise regarding the beneficial effects of low doses of fructose. Specifically, does this impact involve AGE-RAGE signaling? If so, which RAGE isoforms are responsible for the benefits associated with fructose? Furthermore, is it possible to optimize the RAGE/sRAGE ratio by using appropriate doses of fructose and other natural compounds in our diets?
While the AGE-RAGE axis downregulation appears to be a promising approach for attenuating various disease conditions linked to RAGE-mediated inflammation, it is crucial to ensure that strategies aimed at counteracting RAGE signaling do not diminish its beneficial effects. Although the structure of the AGE-RAGE network is relatively well-studied, further research is needed to expand our understanding of the mechanisms underlying the operation of the AGE-RAGE axis and their relationship to human health.
Author contributions
HS: Writing – review & editing, Writing – original draft.
Funding
The author(s) declare that financial support was received for the research, authorship, and/or publication of this article. This work was partially supported by a grant from the Ministry of Education and Science of Ukraine (#0122 U000894).
Acknowledgments
The author dedicates this article to Ukrainian warriors who defend our Motherland.
Conflict of interest
The author declares that the research was conducted in the absence of any commercial or financial relationships that could be construed as a potential conflict of interest.
Publisher’s note
All claims expressed in this article are solely those of the authors and do not necessarily represent those of their affiliated organizations, or those of the publisher, the editors and the reviewers. Any product that may be evaluated in this article, or claim that may be made by its manufacturer, is not guaranteed or endorsed by the publisher.
Abbreviations
RCS, Reactive carbonyl species; ROS, Reactive oxygen species; AGEs, advanced glycoxidation end products; RAGE, receptor for AGEs
References
1. Scheckhuber, CQ . Studying the mechanisms and targets of glycation and advanced glycation end-products in simple eukaryotic model systems. Int J Biol Macromol. (2019) 127:85–94. doi: 10.1016/j.ijbiomac.2019.01.032
2. Garaschuk, O, Semchyshyn, HM, and Lushchak, VI. Healthy brain aging: interplay between reactive species, inflammation and energy supply. Ageing Res Rev. (2018) 43:26–45. doi: 10.1016/j.arr.2018.02.003
3. Lushchak, VI . Adaptive response to oxidative stress: Bacteria, fungi, plants and animals. Comp Biochem Physiol C Toxicol Pharmacol. (2011) 153:175–90. doi: 10.1016/j.cbpc.2010.10.004
4. Semchyshyn, H . Is carbonyl/AGE/RAGE stress a hallmark of the brain aging? Pflugers Arch. (2021) 473:723–34. doi: 10.1007/s00424-021-02529-y
5. Forman, HJ, Fukuto, JM, Miller, T, Zhang, H, Rinna, A, and Levy, S. The chemistry of cell signaling by reactive oxygen and nitrogen species and 4-hydroxynonenal. Arch Biochem Biophys. (2008) 477:183–95. doi: 10.1016/j.abb.2008.06.011
6. Forman, HJ . Redox signaling: an evolution from free radicals to aging. Free Radic Biol Med. (2016) 97:398–407. doi: 10.1016/j.freeradbiomed.2016.07.003
7. Semchyshyn, H . Reactive carbonyl species in vivo: generation and dual biological effects. Sci World J. (2014) 2014:417842. doi: 10.1155/2014/417842
8. Lushchak, VI, and Storey, KB. Oxidative stress concept updated: definitions, classifications, and regulatory pathways implicated. EXCLI J. (2021) 20:956–67. doi: 10.17179/excli2021-3596
9. Yoshikawa, T, and You, F. Oxidative stress and bio-regulation. Int J Mol Sci. (2024) 25:3360. doi: 10.3390/ijms25063360
10. Tessier, FJ . The Maillard reaction in the human body. The main discoveries and factors that affect glycation. Pathol Biol (Paris). (2010) 58:214–9. doi: 10.1016/j.patbio.2009.09.014
11. Semchyshyn, HM, and Lushchak, VI. Interplay between oxidative and carbonyl stresses: molecular mechanisms, biological effects and therapeutic strategies of protection In: VI Lushchak and HM Semchyshyn, editors. Oxidative stress – Molecular mechanisms and biological effects. Rijeka, Croatia: InTech (2012). 15–46.
12. Trnkova, L, Drsata, J, and Bousova, I. Oxidation as an important factor of protein damage: implications for Maillard reaction. J Biosci. (2015) 40:419–39. doi: 10.1007/s12038-015-9523-7
13. Dyer, DG, Blackledge, JA, Katz, BM, Hull, CJ, Adkisson, HD, Thorpe, SR, et al. The Maillard reaction in vivo. Z Ernahrungswiss. (1991) 30:29–45. doi: 10.1007/BF01910730
14. Gordiienko, I . Carbonyl stress chemistry. Biointerface Res App Chem. (2024) 14:55. doi: 10.33263/BRIAC143.055
15. Ott, C, Jacobs, K, Haucke, E, Navarrete Santos, A, Grune, T, and Simm, A. Role of advanced glycation end products in cellular signaling. Redox Biol. (2014) 2:411–29. doi: 10.1016/j.redox.2013.12.016
16. Prasad, K, and Mishra, M. AGE-RAGE stress, stressors, and antistressors in health and disease. Int J Angiol. (2018) 27:1–12. doi: 10.1055/s-0037-1613678
17. Semchyshyn, H, Lozinska, LM, Miedzobrodzki, J, and Lushchak, VI. Fructose and glucose differentially affect aging and carbonyl/oxidative stress parameters in Saccharomyces cerevisiae cells. Carbohydr Res. (2011) 346:933–8. doi: 10.1016/j.carres.2011.03.005
18. Šebekova, K, and Brouder, ŠK. Dietary AGEs may have different effects in people with vegetarian versus omnivorous eating patterns In: J Uribarri , editor. Dietary AGEs and their role in health and disease. Boca Raton, FL: CRC Press (2017). 225–37.
19. Mortera, RR, Bains, Y, and Gugliucci, A. Fructose at the crossroads of the metabolic syndrome and obesity epidemics. Front Biosci (Landmark Ed). (2019) 24:186–211. doi: 10.2741/4713
20. Ting, KKY . John Yudkin's hypothesis: sugar is a major dietary culprit in the development of cardiovascular disease. Front Nutr. (2024) 11:1407108. doi: 10.3389/fnut.2024.1407108
21. Semchyshyn, H . Fructation in vivo: detrimental and protective effects of fructose. Biomed Res Int. (2013) 2013:343914. doi: 10.1155/2013/343914
22. Gugliucci, A . Formation of fructose-mediated advanced glycation end products and their roles in metabolic and inflammatory diseases. Adv Nutr. (2017) 8:54–62. doi: 10.3945/an.116.013912
23. Semchyshyn, H . Is part of the fructose effects on health related to increased AGE formation? In: J Uribarri , editor. Dietary AGEs and their role in health and disease. Boca Raton, FL: CRC Press (2017). 103–11.
24. Sotokawauchi, A, Matsui, T, Higashimoto, Y, and Yamagishi, SI. Fructose causes endothelial cell damage via activation of advanced glycation end products-receptor system. Diab Vasc Dis Res. (2019) 16:556–61. doi: 10.1177/1479164119866390
25. Rai, AK, Jaiswal, N, Maurya, CK, Sharma, A, Ahmad, I, Ahmad, S, et al. Fructose-induced AGEs-RAGE signaling in skeletal muscle contributes to impairment of glucose homeostasis. J Nutr Biochem. (2019) 71:35–44. doi: 10.1016/j.jnutbio.2019.05.016
26. Takeuchi, M, Sakasai-Sakai, A, Takata, T, Takino, JI, and Koriyama, Y. Effects of toxic AGEs (TAGE) on human health. Cells. (2022) 11:2178. doi: 10.3390/cells11142178
27. Valeri, F, Boess, F, Wolf, A, Göldlin, C, and Boelsterli, UA. Fructose and tagatose protect against oxidative cell injury by iron chelation. Free Radic Biol Med. (1997) 22:257–68. doi: 10.1016/S0891-5849(96)00331-0
28. Frenzel, J, Richter, J, and Eschrich, K. Fructose inhibits apoptosis induced by reoxygenation in rat hepatocytes by decreasing reactive oxygen species via stabilization of the glutathione pool. Biochim Biophys Acta. (2002) 1542:82–94. doi: 10.1016/s0167-4889(01)00169-0
29. Spasojević, I, Bajić, A, Jovanović, K, Spasić, M, and Andjus, P. Protective role of fructose in the metabolism of astroglial C6 cells exposed to hydrogen peroxide. Carbohydr Res. (2009) 344:1676–81. doi: 10.1016/j.carres.2009.05.023
30. Semchyshyn, H, and Lozinska, L. Fructose protects baker’s yeast against peroxide stress: potential role of catalase and superoxide dismutase. FEMS Yeast Res. (2012) 12:761–73. doi: 10.1111/j.1567-1364.2012.00826.x
31. Thornalley, PJ . Dicarbonyl intermediates in the Maillard reaction. Ann N Y Acad Sci. (2005) 1043:111–7. doi: 10.1196/annals.1333.014
32. Semchyshyn, H, Miedzobrodzki, J, Bayliak, MM, Lozinska, LM, and Homza, BV. Fructose compared with glucose is a more potent glycoxidation agent in vitro, but not under carbohydrate-induced stress in vivo: potential role of antioxidant and antiglycation enzymes. Carbohydr Res. (2014) 384:61–9. doi: 10.1016/j.carres.2013.11.015
33. Peng, X, Ma, J, Chen, F, and Wang, M. Naturally occurring inhibitors against the formation of advanced glycation end-products. Food Funct. (2011) 2:289–301. doi: 10.1039/c1fo10034c
34. Turk, Z . Glycotoxins, carbonyl stress and relevance to diabetes and its complications. Physiol Res. (2010) 59:147–56. doi: 10.33549/physiolres.931585
35. Angeloni, C, Malaguti, M, and Hrelia, S. Antiglycative activity of sulforaphane: a new avenue to counteract neurodegeneration? Neural Regen Res. (2015) 10:1750–1. doi: 10.4103/1673-5374.169613
36. Twarda-Clapa, A, Olczak, A, Białkowska, AM, and Koziołkiewicz, M. Advanced glycation end-products (AGEs): formation, chemistry, classification, receptors, and diseases related to AGEs. Cells. (2022) 11:1312. doi: 10.3390/cells11081312
37. Halliwell, B, and Gutteridge, JMC. Free radicals in biology and medicine. 2nd ed. Oxford, UK: Clarendon Press (1989). 543 p.
38. Uchida, K . Role of reactive aldehyde in cardiovascular diseases. Free Radic Biol Med. (2000) 28:1685–96. doi: 10.1016/s0891-5849(00)00226-4
39. Pamplona, R . Advanced lipoxidation end-products. Chem Biol Interact. (2011) 192:14–20. doi: 10.1016/j.cbi.2011.01.007
40. Monnier, VM, and Cerami, A. Nonenzymatic browning in vivo: possible process for aging of long-lived proteins. Science. (1981) 211:491–3. doi: 10.1126/science.6779377
41. Vlassara, H, Brownlee, M, and Cerami, A. High-affinity receptor-mediated uptake and degradation of glucose-modified proteins: a potential mechanism for the removal of senescent macromolecules. Proc Natl Acad Sci USA. (1985) 82:5588–92. doi: 10.1073/pnas.82.17.5588
42. Harman, D . Aging: a theory based on free radical and radiation chemistry. J Gerontol. (1956) 11:298–300. doi: 10.1093/geronj/11.3.298
43. Kalapos, MP . Methylglyoxal and glucose metabolism: a historical perspective and future avenues for research. Drug Metabol Drug Interact. (2008) 23:69–92. doi: 10.1515/dmdi.2008.23.1-2.69
44. Anderson, MM, Hazen, SL, Hsu, FF, and Heinecke, JW. Human neutrophils employ the myeloperoxidase-hydrogen peroxide-chloride system to convert hydroxy-amino acids into glycolaldehyde, 2-hydroxypropanal, and acrolein. A mechanism for the generation of highly reactive alpha-hydroxy and alpha,b-unsaturated aldehydes by phagocytes at sites of inflammation. J Clin Invest. (1997) 99:424–32. doi: 10.1172/JCI119176
45. Moghaddam, AE, Gartlan, KH, Kong, L, and Sattentau, QJ. Reactive carbonyls are a major Th2-inducing damage-associated molecular pattern generated by oxidative stress. J Immunol. (2011) 187:1626–33. doi: 10.4049/jimmunol.1003906
46. Beavers, WN, and Skaar, EP. Neutrophil-generated oxidative stress and protein damage in Staphylococcus aureus. Pathog Dis. (2016) 74:ftw060. doi: 10.1093/femspd/ftw060
47. Chung, SS, Ho, EC, Lam, KS, and Chung, SK. Contribution of polyol pathway to diabetes-induced oxidative stress. J Am Soc Nephrol. (2003) 14:S233–6. doi: 10.1097/01.asn.0000077408.15865.06
48. Xu, J, Begley, P, Church, SJ, Patassini, S, McHarg, S, Kureishy, N, et al. Elevation of brain glucose and polyol-pathway intermediates with accompanying brain-copper deficiency in patients with Alzheimer’s disease: metabolic basis for dementia. Sci Rep. (2016) 6:27524. doi: 10.1038/srep27524
49. Levi, B, and Werman, MJ. Fructose triggers DNA modification and damage in an Escherichia coli plasmid. J Nutr Biochem. (2001) 12:235–41. doi: 10.1016/s0955-2863(00)00158-3
50. Levi, B, and Werman, MJ. Fructose and related phosphate derivatives impose DNA damage and apoptosis in L5178Y mouse lymphoma cells. J Nutr Biochem. (2003) 14:49–60. doi: 10.1016/s0955-2863(02)00254-1
51. Sakai, M, Oimomi, M, and Kasuga, M. Experimental studies on the role of fructose in the development of diabetic complications. Kobe J Med Sci. (2002) 48:125–36.
52. Bengmark, S . Dietary intake of AGEs and ALEs and inflammation: nutritional aspects In: J Uribarri , editor. Dietary AGEs and their role in health and disease. Boca Raton, FL: CRC Press (2017). 309–27.
53. Livesey, G, and Taylor, R. Fructose consumption and consequences for glycation, plasma triacylglycerol, and body weight: meta-analyses and meta-regression models of intervention studies. Am J Clin Nutr. (2008) 88:1419–37. doi: 10.3945/ajcn.2007.25700
54. Rizkalla, SW . Health implications of fructose consumption: a review of recent data. Nutr Metab (Lond). (2010) 7:82. doi: 10.1186/1743-7075-7-82
55. Keskin, E, and Yoldas, IH. Fructose consumption correlates with triglyceride-glucose index and glycemic status in healthy adults. Clin Nutr ESPEN. (2022) 52:184–9. doi: 10.1016/j.clnesp.2022.11.008
56. Küçük, U, Taşdelen, B, and Pesenli, K. Does increased fructose consumption increase atherosclerosis burden in patients with NSTEMI? Int J Cardiovasc Acad. (2024) 10:7–12. doi: 10.4274/ijca.2024.24008
57. Moore, MC, Cherrington, AD, Mann, SL, and Davis, SN. Acute fructose administration decreases the glycemic response to an oral glucose tolerance test in normal adults. J Clin Endocrinol Metab. (2000) 85:4515–9. doi: 10.1210/jcem.85.12.7053
58. Sievenpiper, JL, Chiavaroli, L, de Souza, RJ, Mirrahimi, A, Cozma, AI, Ha, V, et al. ‘Catalytic’ doses of fructose may benefit glycaemic control without harming cardiometabolic risk factors: a small meta-analysis of randomised controlled feeding trials. Br J Nutr. (2012) 108:418–23. doi: 10.1017/S000711451200013X
59. Noronha, J, Braunstein, C, Blanco Mejia, S, Khan, TA, Kendall, CWC, Wolever, TMS, et al. The effect of small doses of fructose and its epimers on glycemic control: a systematic review and meta-analysis of controlled feeding trials. Nutrients. (2018) 10:1805. doi: 10.3390/nu10111805
60. Jafari, A, Faghfouri, AH, and Nikpayam, O. The effect of low-fructose diet on anthropometric and metabolic factors: a systematic review and meta-analysis. Nutr Metab Cardiovasc Dis. (2024) 34:281–93. doi: 10.1016/j.numecd.2023.10.025
61. US Department of Agriculture and US Department of Health and Human Services . Dietary guidelines for Americans, 2020–2025. 9th ed. Washington, DC: US Government Publishing Office (2020).
62. Marriott, BP, Cole, N, and Lee, E. National estimates of dietary fructose intake increased from 1977 to 2004 in the United States. J Nutr. (2009) 139:1228S–35S. doi: 10.3945/jn.108.098277
63. Vlassara, H, and Uribarri, J. Glycoxidation and diabetic complications: modern lessons and a warning? Rev Endocr Metab Disord. (2004) 5:181–8. doi: 10.1023/B:REMD.0000032406.84813.f6
64. Goldberg, T, Cai, W, Peppa, M, Dardaine, V, Baliga, BS, Uribarri, J, et al. Advanced glycoxidation end products in commonly consumed foods. J Am Diet Assoc. (2004) 104:1287–91. doi: 10.1016/j.jada.2004.05.214
65. Prasad, C, Davis, KE, Imrhan, V, Juma, S, and Vijayagopal, P. Advanced glycation end products and risks for chronic diseases: intervening through lifestyle modification. Am J Lifestyle Med. (2017) 13:384–404. doi: 10.1177/1559827617708991
66. Monteiro-Alfredo, T, and Matafome, P. Gut metabolism of sugars: formation of glycotoxins and their intestinal absorption. Diabetology. (2022) 3:596–605. doi: 10.3390/diabetology3040045
67. Uribarri, J, Woodruff, S, Goodman, S, Cai, W, Chen, X, Pyzik, R, et al. Advanced glycation end products in foods and a practical guide to their reduction in the diet. Am Diet Assoc. (2010) 110:911–16.e12. doi: 10.1016/j.jada.2010.03.018
68. Henle, T . AGEs in foods: Do they play a role in uremia? Kidney Int Suppl. (2003) 63:S145–7. doi: 10.1046/j.1523-1755.63.s84.16.x
69. Garay-Sevilla, ME, Rojas, A, Portero-Otin, M, and Uribarri, J. Dietary AGEs as exogenous boosters of inflammation. Nutrients. (2021) 13:2802. doi: 10.3390/nu13082802
70. Huang, Y, Chen, Z, Chen, B, Li, J, Yuan, X, Li, J, et al. Dietary sugar consumption and health: umbrella review. BMJ. (2023) 381:e071609. doi: 10.1136/bmj-2022-071609
71. Portero-Otin, M, de la Maza, MP, and Uribarri, J. Dietary advanced glycation end products: their role in the insulin resistance of aging. Cells. (2023) 12:1684. doi: 10.3390/cells12131684
72. Uribarri, J, del Castillo, MD, de la Maza, MP, Filip, R, Gugliucci, A, Luevano-Contreras, C, et al. Dietary advanced glycation end products and their role in health and disease. Adv Nutr. (2015) 6:461–73. doi: 10.3945/an.115.008433
73. Zhang, Q, Li, H, Zheng, R, Cao, L, Zhang, S, Zhang, S, et al. Comprehensive analysis of advanced glycation end-products in commonly consumed foods: presenting a database for dietary AGEs and associated exposure assessment. Food Sci Human Wellness. (2024) 13:1917–28. doi: 10.26599/FSHW.2022.9250159
74. Inan-Eroglu, E, Ayaz, A, and Buyuktuncer, Z. Formation of advanced glycation endproducts in foods during cooking process and underlying mechanisms: a comprehensive review of experimental studies. Nutr Res Rev. (2020) 33:77–89. doi: 10.1017/S0954422419000209
75. Uribarri, J, Cai, W, Sandu, O, Peppa, M, Goldberg, T, and Vlassara, H. Diet-derived advanced glycation end products are major contributors to the body’s AGE pool and induce inflammation in healthy subjects. Ann N Y Acad Sci. (2005) 1043:461–6. doi: 10.1196/annals.1333.052
76. Koschinsky, T, He, CJ, Mitsuhashi, T, Bucala, R, Liu, C, Buenting, C, et al. Orally absorbed reactive glycation products (glycotoxins): an environmental risk factor in diabetic nephropathy. Proc Natl Acad Sci USA. (1997) 94:6474–9. doi: 10.1073/pnas.94.12.6474
77. Yamagishi, S, Ueda, S, and Okuda, S. Food-derived advanced glycation end products (AGEs): a novel therapeutic target for various disorders. Curr Pharm Des. (2007) 13:2832–6. doi: 10.2174/138161207781757051
78. Krajcovicová-Kudlácková, M, Sebeková, K, Schinzel, R, and Klvanová, J. Advanced glycation end products and nutrition. Physiol Res. (2002) 51:313–6. doi: 10.33549/physiolres.930200
79. Pérez-Burillo, S, Pastoriza, S, and Rufian-Henares, JÁ. Effects of dietary AGEs in the gut microbiota composition In: J Uribarri , editor. Dietary AGEs and their role in health and disease. Boca Raton, FL: CRC Press (2017). 239–45.
80. Chen, Y, and Guo, TL. Dietary advanced glycation end-products elicit toxicological effects by disrupting gut microbiome and immune homeostasis. J Immunotoxicol. (2021) 18:93–104. doi: 10.1080/1547691X.2021.1959677
81. Daffu, G, del Pozo, CH, O'Shea, KM, Ananthakrishnan, R, Ramasamy, R, and Schmid, AM. Radical roles for RAGE in the pathogenesis of oxidative stress in cardiovascular diseases and beyond. Int J Mol Sci. (2013) 14:19891–910. doi: 10.3390/ijms141019891
82. Neeper, M, Schmidt, AM, Brett, J, Yan, SD, Wang, F, Pan, Y-CE, et al. Cloning and expression of a cell surface receptor for advanced glycosylation end products of proteins. J Biol Chem. (1992) 267:14998–5004. doi: 10.1016/S0021-9258(18)42138-2
83. Schmidt, AM, Vianna, M, Gerlach, M, Brett, J, Ryan, J, Kao, J, et al. Isolation and characterization of two binding proteins for advanced glycosylation end products from bovine lung which are present on the endothelial cell surface. J Biol Chem. (1992) 267:14987–97. doi: 10.1016/S0021-9258(18)42137-0
84. Schmidt, AM, Yan, SD, Yan, SF, and Stern, DM. The multiligand receptor RAGE as a progression factor amplifying immune and inflammatory responses. J Clin Invest. (2001) 108:949–55. doi: 10.1172/JCI14002
85. Fritz, G . RAGE: a single receptor fits multiple ligands. Trends Biochem Sci. (2011) 36:625–32. doi: 10.1016/j.tibs.2011.08.008
86. Ottosen, J, Smit, P, and Guo, WA. Advanced glycation end products and their receptors in aspiration-induced acute respiratory distress syndrome In: J Uribarri , editor. Dietary AGEs and their role in health and disease. Boca Raton, FL: CRC Press (2017). 203–11.
87. Koch, M, Chitayat, S, Dattilo, BM, Schiefner, A, Diez, J, Chazin, WJ, et al. Structural basis for ligand recognition and activation of RAGE. Structure. (2010) 18:1342–52. doi: 10.1016/j.str.2010.05.017
88. Sparvero, LJ, Asafu-Adjei, D, Kang, R, Tang, D, Amin, N, Im, J, et al. RAGE (receptor for advanced glycation Endproducts) RAGE ligands, and their role in cancer and inflammation. J Transl Med. (2009) 7:17. doi: 10.1186/1479-5876-7-17
89. Sitkiewicz, E, Tarnowski, K, Poznański, J, Kulma, M, and Dadlez, M. Oligomerization interface of RAGE receptor revealed by MS-monitored hydrogen deuterium exchange. PLoS One. (2013) 8:e76353. doi: 10.1371/journal.pone.0076353
90. Park, H, and Boyington, JC. The 1.5 Å crystal structure of human receptor for advanced glycation endproducts (RAGE) ectodomains reveals unique features determining ligand binding. J Biol Chem. (2010) 285:40762–70. doi: 10.1074/jbc.M110.169276
91. Manigrasso, MB, Pan, J, Rai, V, Zhang, J, Reverdatto, S, Quadri, N, et al. Small molecule inhibition of ligand-stimulated RAGE-DIAPH1 signal transduction. Sci Rep. (2016) 6:22450. doi: 10.1038/srep22450
92. Dong, H, Zhang, Y, Huang, Y, and Deng, H. Pathophysiology of RAGE in inflammatory diseases. Front Immunol. (2022) 13:931473. doi: 10.3389/fimmu.2022.931473
93. Kinscherf, NA, and Pehar, M. Role and therapeutic potential of RAGE signaling in neurodegeneration. Curr Drug Targets. (2022) 23:1191–209. doi: 10.2174/1389450123666220610171005
94. Yonekura, H, Yamamoto, Y, Sakurai, S, Petrova, RG, Abedin, MJ, Li, H, et al. Novel splice variants of the receptor for advanced glycation end-products expressed in human vascular endothelial cells and pericytes, and their putative roles in diabetes-induced vascular injury. Biochem J. (2003) 370:1097–109. doi: 10.1042/BJ20021371
95. Takeuchi, A, Yamamoto, Y, Munesue, S, Harashima, A, Watanabe, T, Yonekura, H, et al. Low molecular weight heparin suppresses receptor for advanced glycation end products-mediated expression of malignant phenotype in human fibrosarcoma cells. Cancer Sci. (2013) 104:740–9. doi: 10.1111/cas.12133
96. Ding, Q, and Keller, JN. Splice variants of the receptor for advanced glycosylation end products (RAGE) in human brain. Neurosci Lett. (2005) 373:67–72. doi: 10.1016/j.neulet.2004.09.059
97. Wu, F, Afanasyeva, Y, Zeleniuch-Jacquotte, A, Zhang, J, Schmidt, AM, and Chen, Y. Temporal reliability of serum soluble and endogenous secretory receptors for advanced glycation end-products (sRAGE and esRAGE) in healthy women. Cancer Causes Control. (2018) 29:901–5. doi: 10.1007/s10552-018-1066-4
98. Prasad, K . Low levels of serum soluble receptors for advanced glycation end products, biomarkers for disease state: myth or reality. Int J Angiol. (2014) 23:11–6. doi: 10.1055/s-0033-1363423
99. Schalkwijk, CG, Stehouwer, CD, and van Hinsbergh, VW. Fructose-mediated non-enzymatic glycation: sweet coupling or bad modification. Diabetes Metab Res Rev. (2004) 20:369–82. doi: 10.1002/dmrr.488
100. Scavello, F, Zeni, F, Tedesco, CC, Mensa, E, Veglia, F, Procopio, AD, et al. Modulation of soluble receptor for advanced glycation end-products (RAGE) isoforms and their ligands in healthy aging. Aging (Albany NY). (2019) 11:1648–63. doi: 10.18632/aging.101860
101. Senatus, L, MacLean, M, Arivazhagan, L, Egaña-Gorroño, L, López-Díez, R, Manigrasso, MB, et al. Inflammation meets metabolism: Roles for the receptor for advanced glycation end products axis in cardiovascular disease. Immunometabolism. (2021) 3:e210024. doi: 10.20900/immunometab20210024
102. Chuah, YK, Basir, R, Talib, H, Tie, TH, and Nordin, N. Receptor for advanced glycation end products and its involvement in inflammatory diseases. Int J Inflamm. (2013) 2013:403460. doi: 10.1155/2013/403460
103. Ohtsu, A, Shibutani, Y, Seno, K, Iwata, H, Kuwayama, T, and Shirasuna, K. Advanced glycation end products and lipopolysaccharides stimulate interleukin-6 secretion via the RAGE/TLR4-NF-κB-ROS pathways and resveratrol attenuates these inflammatory responses in mouse macrophages. Exp Ther Med. (2017) 14:4363–70. doi: 10.3892/etm.2017.5045
104. Petriv, N, Neubert, L, Vatashchuk, M, Timrott, K, Suo, H, Hochnadel, I, et al. Increase of α-dicarbonyls in liver and receptor for advanced glycation end products on immune cells are linked to nonalcoholic fatty liver disease and liver cancer. Onco Targets Ther. (2021) 10:1874159. doi: 10.1080/2162402X.2021.1874159
105. Uribarri, J, Peppa, M, Cai, W, Goldberg, T, Lu, M, He, C, et al. Restriction of dietary glycotoxins reduces excessive advanced glycation end products in renal failure patients. J Am Soc Nephrol. (2003) 14:728–31. doi: 10.1097/01.asn.0000051593.41395.b9
106. de Courten, B, de Courten, MP, Soldatos, G, Dougherty, SL, Straznicky, N, Schlaich, M, et al. Diet low in advanced glycation end products increases insulin sensitivity in healthy overweight individuals: a double-blind, randomized, crossover trial. Am J Clin Nutr. (2016) 103:1426–33. doi: 10.3945/ajcn.115.125427
107. Schwarz, JM, Noworolski, SM, Erkin-Cakmak, A, Korn, NJ, Wen, MJ, Tai, VW, et al. Effects of dietary fructose restriction on liver fat, de novo lipogenesis, and insulin kinetics in children with obesity. Gastroenterology. (2017) 153:743–52. doi: 10.1053/j.gastro.2017.05.043
108. Erkin-Cakmak, A, Bains, Y, Caccavello, R, Noworolski, SM, Schwarz, JM, Mulligan, K, et al. Isocaloric fructose restriction reduces serum d-lactate concentration in children with obesity and metabolic syndrome. J Clin Endocrinol Metab. (2019) 104:3003–11. doi: 10.1210/jc.2018-02772
109. Matafome, P . Another player in the field: involvement of glycotoxins and glycosative stress in insulin secretion and resistance. Diabetology. (2020) 1:24–36. doi: 10.3390/diabetology1010004
110. Puchalska, P, and Crawford, PA. Multi-dimensional roles of ketone bodies in fuel metabolism, signaling, and therapeutics. Cell Metab. (2017) 25:262–84. doi: 10.1016/j.cmet.2016.12.022
111. Aldini, G, Vistoli, G, Stefek, M, Chondrogianni, N, Grune, T, Sereikaite, J, et al. Molecular strategies to prevent, inhibit, and degrade advanced glycoxidation and advanced lipoxidation end products. Free Radic Res. (2013) 47:93–137. doi: 10.3109/10715762.2013.792926
112. Jannapureddy, S, Sharma, M, Yepuri, G, Schmidt, AM, and Ramasamy, R. Aldose reductase: an emerging target for development of interventions for diabetic cardiovascular complications. Front Endocrinol (Lausanne). (2021) 12:636267. doi: 10.3389/fendo.2021.636267
113. Bhattacharya, R, Alam, MR, Kamal, MA, Seo, KJ, and Singh, LR. AGE-RAGE axis culminates into multiple pathogenic processes: a central road to neurodegeneration. Front Mol Neurosci. (2023) 16:1155175. doi: 10.3389/fnmol.2023.1155175
114. Saraswat, M, Muthenna, P, Suryanarayana, P, Petrash, JM, and Reddy, GB. Dietary sources of aldose reductase inhibitors: prospects for alleviating diabetic complications. Asia Pac J Clin Nutr. (2008) 17:558–65.
115. Grewal, AS, Thapa, K, Kanojia, N, Sharma, N, and Singh, S. Natural compounds as source of aldose reductase (AR) inhibitors for the treatment of diabetic complications: a mini review. Curr Drug Metab. (2020) 21:1091–116. doi: 10.2174/1389200221666201016124125
116. Neves, D . AGEs and erectile dysfunction: any role of dietary AGEs? In: J Uribarri , editor. Dietary AGEs and their role in health and disease. Boca Raton, FL: CRC Press (2017). 171–88.
117. Radoi, V, Lixandru, D, Mohora, M, and Virgolici, B. Advanced glycation end products in diabetes mellitus: mechanism of action and focused treatment. Proc Rom Acad Series B. (2012) 1:9–19.
118. Kousar, S, Sheikh, MA, and Asghar, M. Antiglycation activity of thiamin-HCl and benfotiamine in diabetic condition. J Pak Med Assoc. (2012) 62:1033–8.
119. Song, Q, Liu, J, Dong, L, Wang, X, and Zhang, X. Novel advances in inhibiting advanced glycation end product formation using natural compounds. Biomed Pharmacother. (2021) 140:111750. doi: 10.1016/j.biopha.2021.111750
120. Ahmad, S, Shahab, U, Baig, MH, Khan, MS, Srivastava, AK, Saeed, M, et al. Inhibitory effect of metformin and pyridoxamine in the formation of early, intermediate and advanced glycation end-products. PLoS One. (2013) 8:e72128. doi: 10.1371/journal.pone.0072128
121. Caengprasath, N, Ngamukote, S, Mäkynen, K, and Adisakwattana, S. The protective effects of pomelo extract (Citrus grandis L. Osbeck) against fructose-mediated protein oxidation and glycation. EXCLI J. (2013) 12:491–502.
122. Tang, Y, and Chen, A. Curcumin eliminates the effect of advanced glycation end-products (AGEs) on the divergent regulation of gene expression of receptors of AGEs by interrupting leptin signalling. Lab Investig. (2014) 94:503–16. doi: 10.1038/labinvest.2014.42
123. Ramis, R, Ortega-Castro, J, Caballero, C, Casasnovas, R, Cerrillo, A, Vilanova, B, et al. How does pyridoxamine inhibit the formation of advanced glycation end products? The role of its primary antioxidant activity. Antioxidants (Basel). (2019) 8:344. doi: 10.3390/antiox8090344
124. Forman, HJ, Zhang, H, and Rinna, A. Glutathione: overview of its protective roles, measurement, and biosynthesis. Mol Asp Med. (2009) 30:1–12. doi: 10.1016/j.mam.2008.08.006
125. Lushchak, VI . Glutathione homeostasis and functions: potential targets for medical interventions. J Amino Acids. (2012) 2012:736837. doi: 10.1155/2012/736837
126. Freund, MA, Chen, B, and Decker, EA. The inhibition of advanced glycation end products by carnosine and other natural dipeptides to reduce diabetic and age-related complications. Compr Rev Food Sci Food Saf. (2018) 17:1367–78. doi: 10.1111/1541-4337.12376
127. Kawahara, M, Tanaka, K, and Kato-Negishi, M. Zinc, carnosine, and neurodegenerative diseases. Nutrients. (2018) 10:147. doi: 10.3390/nu10020147
128. Aldini, G, de Courten, B, Regazzoni, L, Gilardoni, E, Ferrario, G, Baron, G, et al. Understanding the antioxidant and carbonyl sequestering activity of carnosine: direct and indirect mechanisms. Free Radic Res. (2021) 55:321–30. doi: 10.1080/10715762.2020.1856830
129. Shukla, K, Pal, PB, Sonowal, H, Srivastava, SK, and Ramana, KV. Aldose reductase inhibitor protects against hyperglycemic stress by activating Nrf2-dependent antioxidant proteins. J Diabetes Res. (2017) 2017:6785852. doi: 10.1155/2017/6785852
130. Farhangi, MA, Dehghan, P, and Namazi, N. Prebiotic supplementation modulates advanced glycation end products (AGEs), soluble receptor for AGEs (sRAGE), and cardiometabolic risk factors through improving metabolic endotoxemia: a randomized controlled clinical trial. Eur J Nutr. (2020) 59:3009–21. doi: 10.1007/s00394-019-02140-z
131. Park, HY, Lee, HB, Lee, SY, Oh, MJ, Ha, SK, Do, E, et al. Lactococcus lactis KF140 reduces dietary absorption of Nε-(Carboxymethyl)lysine in rats and humans via β-galactosidase activity. Front Nutr. (2022) 9:916262. doi: 10.3389/fnut.2022.916262
132. Legaard, GE, Lyngbaek, MPP, Almdal, TP, Durrer, CG, Nystrup, U, Larsen, EL, et al. Effects of different doses of exercise in adjunct to diet-induced weight loss on the AGE-RAGE axis in patients with short-standing type 2 diabetes: secondary analysis of the DOSE-EX multi-arm, parallel-group, randomized trial. Free Radic Biol Med. (2023) 208:52–61. doi: 10.1016/j.freeradbiomed.2023.07.031
133. Grillo, MA, and Colombatto, S. Advanced glycation endproducts (AGEs): involvement in aging and in neurodegenerative diseases. Amino Acids. (2008) 35:29–36. doi: 10.1007/s00726-007-0606-0
134. Tkachenko, V, Kovalchuk, Y, Bondarenko, N, Bondarenko, O, Ushakova, G, and Shevtsova, A. The cardio- and neuroprotective effects of corvitin and 2-oxoglutarate in rats with pituitrin-isoproterenol-induced myocardial damage. Biochem Res Int. (2018) 2018:9302414. doi: 10.1155/2018/9302414
135. Xue, M, Rabbani, N, Momiji, H, Imbasi, P, Anwar, MM, Kitteringham, N, et al. Transcriptional control of glyoxalase 1 by Nrf2 provides a stress-responsive defence against dicarbonyl glycation. Biochem J. (2012) 443:213–22. doi: 10.1042/BJ20111648
136. Subedi, L, Cho, K, Park, YU, Choi, HJ, and Kim, SY. Sulforaphane-enriched broccoli sprouts pretreated by pulsed electric fields reduces neuroinflammation and ameliorates scopolamine-induced amnesia in mouse brain through its antioxidant ability via Nrf2-HO-1 activation. Oxidative Med Cell Longev. (2019) 2019:3549274. doi: 10.1155/2019/3549274
137. Reynaert, NL, Vanfleteren, LEGW, and Perkins, TN. The AGE-RAGE axis and the pathophysiology of multimorbidity in COPD. J Clin Med. (2023) 12:3366. doi: 10.3390/jcm12103366
138. Múnera-Rodríguez, AM, Leiva-Castro, C, Sobrino, F, López-Enríquez, S, and Palomares, F. Sulforaphane-mediated immune regulation through inhibition of NF-kB and MAPK signaling pathways in human dendritic cells. Biomed Pharmacother. (2024) 177:117056. doi: 10.1016/j.biopha.2024.117056
139. Kawahara, K, Hashiguchi, T, Masuda, K, Saniabadi, AR, Kikuchi, K, Tancharoen, S, et al. Mechanism of HMGB1 release inhibition from RAW264.7 cells by oleanolic acid in Prunus mume Sieb. Et Zucc. Int J Mol Med. (2009) 23:615–20. doi: 10.3892/ijmm_00000172
140. Mateș, L, Banc, R, Zaharie, FA, Rusu, ME, and Popa, D-S. Mechanistic Insights into the Biological Effects and Antioxidant Activity of Walnut (Juglans regia L.) Ellagitannins: A Systematic Review. Antioxidants. (2024) 13:974. doi: 10.3390/antiox13080974
141. Kim, SR, Ha, YM, Kim, YM, Park, EJ, Kim, JW, Park, SW, et al. Ascorbic acid reduces HMGB1 secretion in lipopolysaccharide-activated RAW 264.7 cells and improves survival rate in septic mice by activation of Nrf2/HO-1 signals. Biochem Pharmacol. (2015) 95:279–89. doi: 10.1016/j.bcp.2015.04.007
142. Zhou, Z, Tang, Y, Jin, X, Chen, C, Lu, Y, Liu, L, et al. Metformin inhibits advanced glycation end products-induced inflammatory response in murine macrophages partly through AMPK activation and RAGE/NFκB pathway suppression. J Diabetes Res. (2016) 2016:4847812. doi: 10.1155/2016/4847812
143. Brings, S, Fleming, T, Freichel, M, Muckenthaler, MU, Herzig, S, and Nawroth, PP. Dicarbonyls and advanced glycation end-products in the development of diabetic complications and targets for intervention. Int J Mol Sci. (2017) 18:1153. doi: 10.3390/ijms18050984
144. Wang, G, Wang, Y, Yang, Q, Xu, C, Zheng, Y, Wang, L, et al. Metformin prevents methylglyoxal-induced apoptosis by suppressing oxidative stress in vitro and in vivo. Cell Death Dis. (2022) 13:29. doi: 10.1038/s41419-021-04478-x
145. Limanaqi, F, Biagioni, F, Gambardella, S, Familiari, P, Frati, A, and Fornai, F. Promiscuous roles of autophagy and proteasome in neurodegenerative proteinopathies. Int J Mol Sci. (2020) 21:3028. doi: 10.3390/ijms21083028
146. Loeffler, DA . Influence of normal aging on brain autophagy: a complex scenario. Front Aging Neurosci. (2019) 11:49. doi: 10.3389/fnagi.2019.00049
147. Zeng, S, Feirt, N, Goldstein, M, Guarrera, J, Ippagunta, N, Ekong, U, et al. Blockade of receptor for advanced glycation end product (RAGE) attenuates ischemia and reperfusion injury to the liver in mice. Hepatology. (2004) 39:422–32. doi: 10.1002/hep.20045
148. Singh, H, and Agrawal, DK. Therapeutic potential of targeting the HMGB1/RAGE axis in inflammatory diseases. Molecules. (2022) 27:7311. doi: 10.3390/molecules27217311
149. Vijaykrishnaraj, M, and Wang, K. Dietary natural products as a potential inhibitor towards advanced glycation end products and hyperglycemic complications: a phytotherapy approach. Biomed Pharmacother. (2021) 144:112336. doi: 10.1016/j.biopha.2021.112336
150. Valle-Sánchez, SL, Rodríguez-Ramírez, R, Ávila-Villa, LA, Villa-Lerma, AG, Davidov-Pardo, G, Wall-Medrano, A, et al. Chapter 9 - natural inhibitory compounds of advanced glycation end products (AGEs) from the Maillard reaction. Stud Nat Prod Chem. (2023) 79:341–81. doi: 10.1016/B978-0-443-18961-6.00018-4
151. Guzmán, EA, Pitts, TP, Diaz, MC, and Wright, AE. The marine natural product Scalarin inhibits the receptor for advanced glycation end products (RAGE) and autophagy in the PANC-1 and MIA PaCa-2 pancreatic cancer cell lines. Investig New Drugs. (2019) 37:262–70. doi: 10.1007/s10637-018-0635-4
152. Zhou, Q, Cheng, KW, Gong, J, Li, ETS, and Wang, M. Apigenin and its methylglyoxal-adduct inhibit advanced glycation end products-induced oxidative stress and inflammation in endothelial cells. Biochem Pharmacol. (2019) 166:231–41. doi: 10.1016/j.bcp.2019.05.027
153. Maeda, S, Matsui, T, Ojima, A, Takeuchi, M, and Yamagishi, S. Sulforaphane inhibits advanced glycation end product-induced pericyte damage by reducing expression of receptor for advanced glycation end products. Nutr Res. (2014) 34:807–13. doi: 10.1016/j.nutres.2014.08.010
154. Subedi, L, Lee, JH, Gaire, BP, and Kim, SY. Sulforaphane inhibits MGO-AGE-mediated neuroinflammation by suppressing NF-κB, MAPK, and AGE-RAGE signaling pathways in microglial cells. Antioxidants (Basel). (2020) 9:792. doi: 10.3390/antiox9090792
155. Gu, Q, Wang, B, Zhang, X-F, Ma, Y-P, Liu, J-D, and Wang, X-Z. Contribution of receptor for advanced glycation end products to vasculature-protecting effects of exercise training in aged rats. Eur J Pharmacol. (2014) 741:186–94. doi: 10.1016/j.ejphar.2014.08.017
156. Falone, S, D’Alessandro, A, Mirabilio, A, Petruccelli, G, Cacchio, M, Di Ilio, C, et al. Long-term running biphasically improves methylglyoxal-related metabolism, redox homeostasis and neurotrophic support within adult mouse brain cortex. PLoS One. (2012) 7:e31401. doi: 10.1371/journal.pone.0031401
157. Song, F, Hurtado del Pozo, C, Rosario, R, Zou, YS, Ananthakrishnan, R, Xu, X, et al. RAGE regulates the metabolic and inflammatory response to high-fat feeding in mice. Diabetes. (2014) 63:1948–65. doi: 10.2337/db13-1636
158. Olekson, MP, Faulknor, RA, Hsia, HC, Schmidt, AM, and Berthiaume, F. Soluble receptor for advanced glycation end products improves stromal cell-derived factor-1 activity in model diabetic environments. Adv Wound Care (New Rochelle). (2016) 5:527–38. doi: 10.1089/wound.2015.0674
159. Derk, J, MacLean, M, Juranek, J, and Schmidt, AM. The receptor for advanced glycation endproducts (RAGE) and mediation of inflammatory neurodegeneration. J Alzheimers Dis Parkinsonism. (2018) 8:421. doi: 10.4172/2161-0460.1000421
160. Lee, S, Piao, C, Kim, G, Kim, JY, Choi, E, and Lee, M. Production and application of HMGB1-derived recombinant RAGE antagonist peptide for anti-inflammatory therapy in acute lung injury. Eur J Pharm Sci. (2018) 114:275–84. doi: 10.1016/j.ejps.2017.12.019
161. Hurtado Del Pozo, C, Ruiz, HH, Arivazhagan, L, Aranda, JF, Shim, C, Daya, P, et al. A receptor of the immunoglobulin superfamily regulates adaptive thermogenesis. Cell Rep. (2019) 28:773–91.e7. doi: 10.1016/j.celrep.2019.06.061
162. Macías-Cervantes, MH, Rodríguez-Soto, JM, Uribarri, J, Díaz-Cisneros, FJ, Cai, W, and Garay-Sevilla, ME. Effect of an advanced glycation end product-restricted diet and exercise on metabolic parameters in adult overweight men. Nutrition. (2015) 31:446–51. doi: 10.1016/j.nut.2014.10.004
163. Sponder, M, Campean, IA, Emich, M, Fritzer-Szekeres, M, Litschauer, B, Graf, S, et al. Long-term physical activity leads to a significant increase in serum sRAGE levels: a sign of decreased AGE-mediated inflammation due to physical activity? Heart Vessel. (2018) 33:893–900. doi: 10.1007/s00380-018-1125-5
164. Miranda, ER, Mey, JT, Blackburn, BK, Chaves, AB, Fuller, KNZ, Perkins, RK, et al. Soluble RAGE and skeletal muscle tissue RAGE expression profiles in lean and obese young adults across differential aerobic exercise intensities. J Appl Physiol. (2023) 135:849–62. doi: 10.1152/japplphysiol.00748.2022
165. Egaña-Gorroño, L, López-Díez, R, Yepuri, G, Ramirez, LS, Reverdatto, S, Gugger, PF, et al. Receptor for advanced glycation end products (RAGE) and mechanisms and therapeutic opportunities in diabetes and cardiovascular disease: insights from human subjects and animal models. Front Cardiovasc Med. (2020) 7:37. doi: 10.3389/fcvm.2020.00037
166. Nakamura, K, Yamagishi, S, Adachi, H, Kurita-Nakamura, Y, Matsui, T, Yoshida, T, et al. Serum levels of sRAGE, the soluble form of receptor for advanced glycation end products, are associated with inflammatory markers in patients with type 2 diabetes. Mol Med. (2007) 13:185–9. doi: 10.2119/2006-00090.Nakamura
Keywords: fructose, nonenzymatic processes, reactive species, glycation products, receptor for advanced glycation end products, diet optimization, sulforaphane, physical activity
Citation: Semchyshyn H (2024) Fructose-mediated AGE-RAGE axis: approaches for mild modulation. Front. Nutr. 11:1500375. doi: 10.3389/fnut.2024.1500375
Edited by:
Gustavo Ferreira Alves, University of Camerino, ItalyReviewed by:
Farnoosh Shemirani, The University of Iowa, United StatesMarius Emil Rusu, University of Medicine and Pharmacy Iuliu Hatieganu, Romania
Copyright © 2024 Semchyshyn. This is an open-access article distributed under the terms of the Creative Commons Attribution License (CC BY). The use, distribution or reproduction in other forums is permitted, provided the original author(s) and the copyright owner(s) are credited and that the original publication in this journal is cited, in accordance with accepted academic practice. No use, distribution or reproduction is permitted which does not comply with these terms.
*Correspondence: Halyna Semchyshyn, aGFseW5hLnNlbWNoeXNoeW5AcG51LmVkdS51YQ==