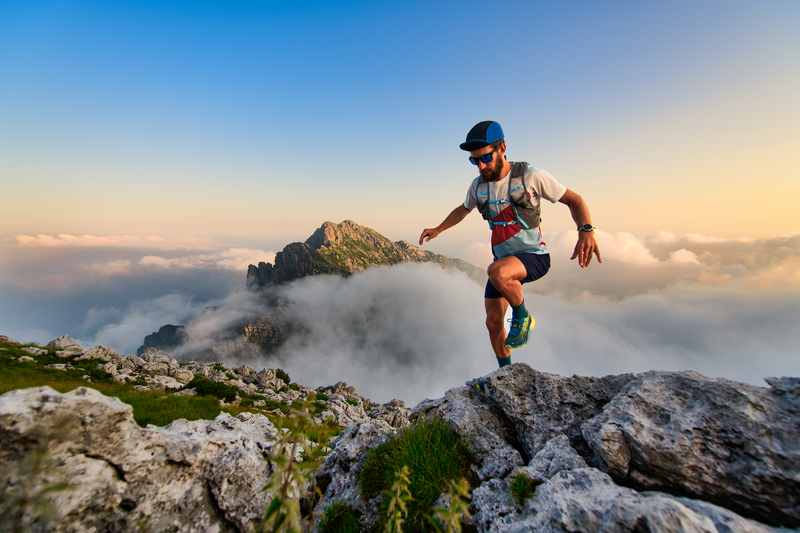
95% of researchers rate our articles as excellent or good
Learn more about the work of our research integrity team to safeguard the quality of each article we publish.
Find out more
REVIEW article
Front. Nutr. , 14 November 2024
Sec. Nutrition and Food Science Technology
Volume 11 - 2024 | https://doi.org/10.3389/fnut.2024.1496706
This article is part of the Research Topic Nutritional Bio-Active Compounds and Their Structural Modifications for Functional Foods View all 4 articles
Bioactive peptides hold significant potential for enhancing human health, however, their limited oral bioavailability poses a substantial barrier to their widespread use in the food and pharmaceutical industries. This article reviews the key factors influencing the absorption efficiency of oral bioactive peptides, including issues related to bitter taste perception, challenges in gastrointestinal environmental stability, and limitations in transmembrane transport. Furthermore, it highlights the latest technologies, such as osmotic technology, chemical modification, and advanced delivery systems, and discusses their advantages in enhancing the stability of bioactive peptides and facilitating intestinal absorption. In addition, the application and challenges of common delivery systems such as liposomes, emulsions, polymer nanoparticles, and hydrogels in oral bioactive peptide delivery are also discussed. This paper aims to provide a theoretical foundation for scientific research and practical applications of oral delivery of bioactive peptides, thereby promoting the further development of bioactive peptides in the context of human health.
Bioactive peptides are a class of compounds composed of natural amino acids arranged in various combinations, sequences, and spatial conformations. These peptides exhibit diverse physiological activities that are beneficial to the body’s functions. Typically, bioactive peptides range in size from 2 to 20 amino acid residues and have smaller molecular weights compared to proteins, but their bioactivity is often greater than that of proteins (1). Traditional protein digestion theory suggests that proteins can only be absorbed and utilized after being broken down into amino acids upon entering the body (2). However, recent studies have demonstrated that small-molecule peptides are absorbed more readily than proteins. Absorption channels for bioactive peptides exist in the small intestine, allowing these peptides to be directly absorbed and utilized by the body, with an absorption rate that surpasses that of proteins and amino acids. The bioactivity of bioactive peptides is reflected in various aspects, exhibiting regulatory functions such as antihypertensive, antihyperlipidemic, antihyperglycemic, anti-cholesterol, antiviral, and anticancer effects (3).
Although bioactive peptides have the potential to become functional foods and even drugs, their low bioavailability and low activity caused by oral administration are an urgent problem to be solved. The biological activity of a bioactive peptide depends largely on its chemical structure, including amino acid composition, molecular weight, amino acid sequence, and peptide spatial conformation (4). Oral administration of bioactive peptides need to overcome multiple barriers (such as complex enzymatic decomposition in the gastrointestinal tract, changes in pH, adsorption of small intestinal mucus, obstruction of small intestinal mucosal cells, etc.) before they can be absorbed and utilized by the human body. These barriers may cause changes in the sequence and spatial structure of bioactive peptides, resulting in the loss of biological activity of bioactive peptides. Furthermore, these barriers can hinder the absorption and utilization of bioactive peptides, significantly decreasing the amount that enters systemic circulation and performs biological functions in targeted areas.
Currently, various strategies have been developed to enhance the bioavailability of bioactive peptides in the human body. These strategies include chemical structure modifications, permeation enhancers, and colloidal delivery systems, such as liposomes, emulsions, biopolymer nanoparticles, and hydrogels (132). Each of these approaches has its own advantages and disadvantages. For instance, chemical modifications can significantly improve the stability of bioactive peptides; however, they may alter the original chemical structure of the peptides, potentially affecting their biological activity and even leading to the production of harmful substances (5). Although intestinal permeation enhancers (PEs) show good absorption-promoting effects, excessive use can compromise the integrity of the intestinal barrier, and the stability of permeation enhancers in the gastrointestinal tract also requires careful consideration by researchers (6). Encapsulating bioactive peptides using colloidal delivery systems is considered the most promising approach, as it can mask bitterness and overcome many challenges encountered during oral administration, but there are still some problems such as low encapsulation efficiency, poor stability, and poor targeting (7).
In summary, improving the bioavailability of orally delivered bioactive peptides requires a thorough analysis of the advantages and limitations of current delivery strategies. Unfortunately, to date, there remains a lack of systematic collation and comprehensive reviews addressing these issues in the relevant literature. Therefore, this review comprehensively examines the challenges associated with the oral delivery of bioactive peptides, introduces the advantages and disadvantages of existing oral delivery systems, and summarizes the future development trends of these systems. The aim of this review is to provide a valuable reference for subsequent studies on bioactive peptide delivery systems through this in-depth analysis.
The oral delivery of bioactive peptides presents several challenges. First, some bioactive peptides may possess a pronounced bitter taste, which can significantly impact patients’ acceptance of oral administration. Second, the digestive tolerance of bioactive peptides within the gastrointestinal tract poses another major challenge for their oral delivery. The variable pH gradient and the complex digestive enzyme system of the gastrointestinal tract can severely affect both the structural integrity and the functional stability of bioactive peptides. Additionally, the intricate defense system formed by the mucus layer, epithelial cells, and microbial community in the gastrointestinal tract is a critical factor limiting the oral bioavailability of these peptides. Furthermore, the unique physicochemical and structural properties of bioactive peptides can also significantly influence their efficacy in oral delivery.
Bioactive peptides from natural sources are very limited, so most bioactive peptides are produced by enzymatic hydrolysis of proteins. However, proteolysis can not only produce biologically active peptides, but also produce some peptides with a pronounced bitter taste. Generally speaking, bitter taste in food products is not accepted by consumers. The bitterness produced by the hydrolysis process limits the application of active peptides in the food industry, so how to reduce the bitterness is an extremely important issue. The bitter taste of peptides is related to hydrophobic amino acids (8) and their relative molecular masses (9). As early as 1997, Kuhfeld et al. (10) extracted peptides with molecular weights less than 4,000 Da from dried sausages, graded the extracts for sensory evaluation, and found that the higher the intensity of bitterness, the higher the concentration of hydrophobic amino acids in the extracts. Henriksen et al. (11) extracted bitter peptides from commercially available soy protein hydrolysates. The analysis showed that the bitterness of soy peptides was mainly associated with the presence of medium molecular weight peptides in the range of 1,000–4,000 Da, and the bitterness of peptide fractions less than 1,000 Da was lower than that of high molecular weight fractions.
Since the middle of the 20th century, the research on the removal of the bitterness of short protein peptides has gradually increased, and the most common method is masking. Fan et al. (12) used a variety of masking agents for removing bitterness from soy protein hydrolysates, among which xylitol, sucrose, and α-maltodextrin had significant debittering effects. In addition, bitterness can also be removed by destroying the structure of bitter peptides by enzymatic hydrolysis (13), which is widely used in industry because of its high efficiency and no loss of nitrogen. Saha et al. (14) used aminopeptidase to hydrolyze soybean protein isolate with a bitterness value of 3.6 to reduce its bitterness value to 0.4 reducing its bitterness value to 0.4. It is worth noting that the plastein reaction, the reaction in which protease promotes the formation of a gel-like substance from high-concentration protein hydrolyzate under suitable conditions, is an effective debittering method (15, 16). Peptide condensation during plastein reactions can help reduce the bitterness intensity of polypeptides. However, the plastein reaction is not yet applied in industry and needs further exploration.
Two major types of biochemical barriers exist for orally administered peptides: variable pH and gastrointestinal proteases (Figure 1). Orally administered bioactive peptides travel through the oral cavity to the stomach, then to the duodenum, jejunum, ileum, and finally to the colon and rectum (17). Although digestion begins in the oral cavity, due to the extremely short oral action time, the oral cavity not typically cited as a major factor hindering the absorption and utilization of orally administered bioactive peptides. The main factors affecting the absorption and utilization of oral bioactive peptides mainly come from the stomach and small intestine. The first thing to overcome when taking bioactive peptides orally is the variable pH of the gastrointestinal tract. The pH value of gastric juice is 1.5–3.5, that of the duodenum is about 5–6, and that of the jejunum and terminal ileum rises to 7–8 (18). Variable pH gradients have a great impact on the physiological efficacy of some bioactive peptides. The antioxidant activity of the pentapeptide ATSHH from whitefish protein will show a significant decrease trend under acidic conditions (pH = 2) (19).
In addition, after the bioactive peptides reach the stomach, they will stimulate the gastric mucosa to secrete pepsin from the gastric lining cells. Pepsin can hydrolyze the polypeptide with aromatic residues such as phenylalanine, tryptophan, and tyrosine. Bioactive peptides hydrolyzed by pepsin will lose their inherent biological activity. After the bioactive peptide enters the small intestine through the stomach, the trypsin and chymotrypsin present in the small intestine will also specifically hydrolyze the peptide chain (20). The hydrolysis of the above enzymes will change the structure and activity of the bioactive peptide. Li et al. (21) performed in vitro simulated digestion experiments on rice protein hydrolyzate and found that the anti-hypertensive IC50 (half maximal inhibitory concentration) value of rice protein increased from 140 to 180 μg/mL in the presence of digestive enzymes (pepsin and pancreatic enzymes), indicating that the anti-hypertensive activity of rice protein hydrolyzate was significantly reduced. In addition, after the bioactive peptides reach the stomach, they will stimulate the gastric mucosa to secrete pepsin from the gastric lining cells. Pepsin can hydrolyze the polypeptide with aromatic residues such as phenylalanine, tryptophan, and tyrosine. Bioactive peptides hydrolyzed by pepsin will lose their inherent biological activity. After the bioactive peptide enters the small intestine through the stomach, the trypsin and chymotrypsin present in the small intestine will also specifically hydrolyze the peptide chain (20). The hydrolysis of the above enzymes will change the structure and activity of the bioactive peptide. Li et al. (21) performed in vitro simulated digestion experiments on rice protein hydrolyzate and found that the anti-hypertensive IC50 (half maximal inhibitory concentration) value of rice protein increased from 140 to 180 μg/mL in the presence of digestive enzymes (pepsin and pancreatic enzymes), indicating that the anti-hypertensive activity of rice protein hydrolyzate was significantly reduced.
After bioactive peptides are digested in the stomach and successfully reach the small intestine, the intestinal mucus layer covering the intestinal surface is one of the main factors limiting the bioavailability of oral bioactive peptides. The intestinal mucus layer is a kind of intelligent hydrogel with high viscoelasticity and adhesiveness, which contains highly branched polysaccharides and negatively charged mucin (22). The intestinal mucus layer plays a protective role by forming a sieve-like structure on itself. This structure can effectively prevent 10–200 nm particles from passing through the mesh, and has the function of selectively transmitting nutrients (23). Mucin, glycolipids, and glycoproteins in the mucus layer act as both barriers and transmit signals (24). When bioactive peptides reach the intestinal mucus layer, their further diffusion may be affected by mucin adhesion.
After bioactive peptides pass through the mucus layer and reach the surface of epithelial cells, the epithelial cells located under the mucus are another major factor limiting the bioavailability of oral bioactive peptides. The small intestine epithelial cells are a continuous monolayer that separates the intestinal lumen from the underlying lamina propria. There is a tight junction (TJ) between adjacent epithelial cells, which only allows small molecules such as water and ions to pass through. In addition, the small intestine cell membrane acts as a barrier to prevent extracellular substances from freely entering and exiting the cells by selectively absorbing nutrients (25). Based on the above reasons, the small intestinal epithelium is impermeable. Bioactive peptides need to pass through the TJ or intestinal epithelial cell membrane to reach the bloodstream and ultimately bind to the target to exert physiological activity. However, most bioactive peptides cannot effectively penetrate intestinal epithelial cells due to the lack of targeted carrier proteins on the intestinal epithelial cell membrane, which seriously affects the bioavailability of bioactive peptides.
The physicochemical properties of peptides are one of the important factors affecting the bioavailability of orally delivered active peptides. The molecular weight and structural characteristics of the peptides can affect their absorption. Compared with short-chain peptides with smaller molecular weights, long-chain peptides are more sensitive to gastrointestinal proteases, which results in long-chain peptides being more easily degraded and absorbed by gastrointestinal digestive enzymes (26, 131). Research by Chen and Li (27) showed that the stability of casein-derived peptides with different molecular weights varies in simulated gastrointestinal tracts. Peptides with a molecular weight greater than 3 kDa are more likely to be degraded during gastric digestion than peptides with molecular weights less than 3 kDa (27). In addition, studies have shown that some short peptides with smaller molecular weights can be transported across intestinal cells through peptide transporters expressed in the intestine, while oligopeptides can be passively transported and absorbed into the body through hydrophobic regions or tight junctions of membrane epithelial cells (28, 130). However, long-chain peptides typically need to be absorbed through endocytosis. Therefore, short-chain peptides are more easily absorbed and utilized by the body.
In addition, the structural characteristics of peptides also play a crucial role in the stability of oral bioactive peptides. The amino acids sequence and structure of bioactive peptides can affect the stability of peptides during digestion, thereby affecting their bioavailability. Savoie et al. (29) found that high levels of proline and glutamic acid in peptide sequences can enhance the resistance of peptides to pepsin and trypsin. Udenigwe (30) research showed that bioactive peptides with a higher β-sheet structure ratio are more sensitive to heat treatment. In addition, the charge of the peptide has been shown to affect the transport of peptides. For example, peptides with neutral amino acid residues can be preferentially recognized by oligopeptide transporter 1 (PepT1) (31). PepT1 is a transporter protein present on the brush like border membrane of the small intestine epithelium. The research of Wang and Li (32) showed that in addition to PepT1 mediated transport pathway, bioactive peptides can also cross small intestinal epithelial cells through endocytic transport and paracellular transport. For example, positively charged hydrophobic antioxidant casein peptides can be transported via endocytosis, whereas negatively charged hydrophilic peptides need to be transported via paracellular pathways.
After successfully overcoming multiple obstacles such as the variable pH environment of the gastrointestinal tract, enzymatic hydrolysis by gastrointestinal digestive enzymes, and adhesion/pre-cleavage of the intestinal mucus layer, bioactive peptides still need to overcome the obstruction of the small intestinal epithelial cells to enter the blood circulation system, which is the prerequisite for the physiological functions of bioactive peptides in vivo. There are three main modes of transmembrane transport of bioactive peptides (Figure 2): vector transport, cell bypass transport, and endocytosis transport (33).
The carrier-mediated transport pathway primarily relies on oligopeptide transporters (34). The important feature of transporters is that they can select peptides. Transporters have been found to recognize and transport over 8,000 different peptides (35). There are two main types of transporters: PepT1 and PepT2. Both PepT1 and PepT2 can be used for the transport of dipeptides and tripeptides (36). Currently, there are more studies on PepT1 than PepT2 on the transport of polypeptides. PepT1 is mainly expressed in intestinal epithelial cells and is responsible for the transport and absorption of bioactive peptides. As mentioned in the section on the physicochemical properties of peptides, the charge of peptides affects the mode of transport, and PepT1 preferentially recognizes peptides with neutral charge and high hydrophobicity, and preferentially binds residues rich in non-polar amino acids. Fan et al. (37) studied the transport modes of IW, IWH, and IWHHT peptides in Caco-2 cells, which further verified that PepT1 preferred to select small peptides with high hydrophobicity. Table 1 summarizes the transport pathways of different bioactive peptides through the Caco-2 cell model, aiming to provide a solid experimental basis for subsequent research and product development.
The paracellular transport pathway is currently the most reported passive absorption pathway for bioactive peptides with more than tripeptides (38). The driving force for oligopeptide transport comes from the electrochemical gradient formed by protons as high-energy electrons are transferred along the respiratory chain, and the diffusion process does not require a carrier or energy consumption (39). The paracellular transport pathway is mediated through the TJ between epithelial cells, a tight biological barrier with selective permeability (40). It has been shown that TJ tends to transport negatively charged peptides and is selective for positively charged peptides (41), and bioactive peptides with small hydrophilic molecular weights are more inclined to this transport mode (42). In general, when the molecular diameter of a bioactive peptide exceeds 15 Å, the peptide cannot undergo paracellular transported. However, it is still possible for bioactive peptides with larger molecular sizes to diffuse through TJ if their structures have high conformational flexibility (43). Chiasma has successfully developed an oral formulation of octreotide, named Mycapssa®, utilizing its innovative Transient Permeation Enhancer (TPE™) technology. In this approach, sodium caprate serves as an osmotic enhancer, inducing the reversible opening of tight junctions between intestinal epithelial cells to facilitate the paracellular transport of peptides. The successful development of Mycapssa® not only strongly confirms the feasibility of the paracellular transport strategy for the oral delivery of peptide drugs but also paves the way for further research into the oral delivery of bioactive peptides (44).
Endocytic transport is an energy-dependent transcellular transport pathway and is the main transport pathway for long-chain peptides. In this pathway, bioactive peptides are transported into cells through the formation of vesicles formed by invagination of the cell membrane (45). Bioactive peptides with smaller molecules can enter the blood circulation through carrier transport and paracellular pathways, while most large molecule peptides need to be transported through endocytosis. The study by Xu et al. (46) showed that 17-peptide (casein 193–209) can be completely absorbed by the Caco-2 cell monolayer model, and its absorption process is mainly carried out through endocytosis transport. The first step in endocytic transport is the interaction of polypeptides with the cell membranes. Since the cell membrane is composed of a lipid bilayer, endocytic transport is considered an ideal pathway for the transport of lipophilic peptides. The anti-oxidant peptide YWDHNNPQIR is transported across the Caco-2 cell monolayer via endocytosis, primarily because it is composed of hydrophobic amino acids (47). Xiao et al. have innovatively designed and prepared a hybrid liposome system named mExos@DSPE-Hyd-PMPC. This system significantly improves drug encapsulation efficiency and enhances endocytic transport efficacy by effectively integrating functional liposomes with milk-derived exosomes (mExos). Notably, this hybrid liposome exhibits adaptive surface characteristics, enabling it to intelligently adjust its physicochemical properties based on the pH microenvironment of the intestinal mucosal surface. This adaptability facilitates a more efficient endocytic transport process (48).
Notably, research has demonstrated that the hydrophilicity and charge state of bioactive peptides play a significant role in their transport within the body (49). The charge can influence the interactions of bioactive peptides with cell membranes, transport carriers, and other molecules in the gastrointestinal environment. Table 2 summarizes the relationship between various transport mechanisms and the properties of peptides. However, it is important to emphasize that hydrophilicity and charge state are not the only factors determining the transport pathways of bioactive peptides. The transport pathways are also influenced by several other factors, including molecular weight, peptide structure, hydrophobicity, the gastrointestinal environment, and the selection of transport carriers (133).
As mentioned above, the oral administration of bioactive peptides encounters numerous barriers in the human body, which significantly diminish their bioavailability. Therefore, the development of effective oral delivery systems to enhance the bioavailability of bioactive peptides is imperative. An ideal oral delivery system should ensure that the bioactive peptide maintains its integrity before reaching the site of absorption and promotes targeted release at the desired site of absorption. Currently, several prominent oral delivery technologies have been extensively studied and applied to overcome the barriers associated with bioactive peptides delivery in the human body. These oral delivery technologies include permeation promotion technologies, chemical structural modifications, colloidal delivery systems, etc.
One of the biggest obstacles to oral administration of bioactive peptides is the poor permeability of intestinal epithelial cells to bioactive peptides. Permeation enhancers (PEs) are substances that can temporarily increase intestinal permeability and promote the penetration of bioactive peptides through the intestinal epithelium (47). Currently, over 250 substances have been investigated in clinical research as PEs for the oral delivery of bioactive peptides, such as surfactants, fatty acids, bile salts, and cell-penetrating peptides (50). Based on their mechanisms of action, PEs are mainly divided into two categories (51). The first category mainly acts on the TJ between epithelial cells and achieves paracellular transport of bioactive peptides by opening the TJ between epithelial cells. The second category is to promote the transmembrane transport of bioactive peptides by increasing the permeability of the cell membrane. Table 3 lists some typical PEs and their respective mechanisms of action. It is worth noting that some specific PEs can act on both pathways at the same time, such as sodium decanoate, bile salts and chitosan. In addition, although PEs are generally considered safe and non-toxic, the additive dosage of PEs still needs to be strictly controlled when using them. Excessive use of PES can cause excessive changes in the permeability of intestinal epithelial cells, which will eventually induce local inflammation or long-term damage to intestinal epithelium (52). For example, calcium chelators can cause Ca2+ depletion in the body, thereby damaging actin filaments, altering adherens junctions and reducing cell adhesion (53).
Cell-penetrating peptides (CPPs), as an important branch of penetration enhancers, are mainly polypeptides ranging from 5 to 30 amino acids, which transport bioactive peptides across the membrane by penetrating the cell membrane or endocytosis (54). Currently, researchers have designed or identified more than 100 peptides that can effectively promote the transport of biological macromolecules across cell membranes. In practical applications, nucleotides, bioactive peptides, and other biologically active substances are prone to lose their activity in the systemic circulation. Encapsulating such substances in nanoparticles can greatly enhance their stability in vivo. However, the presence of the cell membrane hinders the uptake of bioactive substances by target cells. CPPs provide researchers with a new direction of exploration. Studies have shown that combining CPPs with nanoparticles can further enhance the transcellular delivery of bioactive peptides and effectively improve the uptake of bioactive substances by target cells. Knoll et al. (55) developed a new type of CPP-modified nanostructured lipid-based carrier, and experimental results demonstrated that this new type of coated nanocarrier can improve the uptake of bioactive substances by cells. The in vivo toxicity of CPPs is not yet fully understood, but a small number of published animal studies and several CPP formulations approved for clinical trials demonstrate the general safety profile of CPP molecules at study doses (56). Nevertheless, no CPP-encapsulated drugs have entered clinical trials, and further research is needed to evaluate their in vivo delivery effects (Table 4).
Bioactive peptides are a type of molecules that are relatively easy to modify in chemical structure. Chemical modification can significantly improve the stability of bioactive peptides. The more commonly used chemical modification methods are PEGylation and cyclization (57). PEGylation is a chemical modification technique that involves the covalent attachment of polyethylene glycol (PEG) molecules to biological macromolecules, such as proteins and peptides. This process aims to optimize the physicochemical properties and biological characteristics of these biomolecules. For bioactive peptides, the incorporation of PEG can significantly enhance their water solubility, thereby improving their solubility in physiological environments, which is essential for effective absorption and distribution. Furthermore, PEG, being an inert polymer, effectively protects peptide drugs from enzymatic degradation, leading to a substantial increase in the retention rate and bioavailability of bioactive peptides. Additionally, the increase in molecular weight resulting from PEGylation reduces the renal clearance rate of peptide drugs, thereby prolonging their half-life in the body and decreasing the frequency of administration (58). Zhou et al. (59) demonstrated that when the HM-3 peptide was modified with methoxy-PEG-aldehyde, its half-time was extended by 5.86 times in male SD rats. Wang (60) similarly showed that after pegylation, the CPU-HM peptide exhibited higher in vivo activity and a longer half-time.
Cyclization is another commonly used method for chemical modification of bioactive peptides. By creating a cyclic structure, cyclization eliminates the exposed N- and C-terminals in peptide molecules, rendering them less susceptible to enzymatic degradation (61). Desmopressin is an analog obtained by cyclization of vasopressin, which is more resistant to enzymatic degradation than vasopressin (62). Similarly, cyclized opioids exhibit longer half-life and higher metabolic stability (63). In addition, cyclic structural peptides have better permeability than linear structural peptides. The cyclic structure is more compact than the linear structure, which reduces the collision of the cyclic structure peptide in the solution and ultimately allows it to pass through the epithelial barrier faster (64).
In addition to debittering, the plastein reaction mentioned above also provides a feasible method for the modification of peptides. Studies have shown that plastein reactions can enhance the activity of angiotensin-converting enzyme (ACE) inhibitory peptides. Song et al. (65) used plastein reactions to modify hazelnut peptides, and the results showed that the ACE inhibition rate of the modified products was significantly improved. Similarly, Jiang et al. (66) employed plastein reaction to modify ACE inhibitory peptides derived from sea cucumbers, and found that the modified peptide showed significantly enhanced thermal stability, and the thermal transition temperature of the modified peptide increased from 120°C to 134°C. These studies indicate that plastein reaction is a promising strategy to induce structural modifications to improve the biological activity of peptides. However, the application of plastein reactions in peptide modification is not immature at present, and research on peptide sequence changes after plastein reactions is relatively limited. Regardless, when modifying the chemical structure of bioactive peptides to improve their bioavailability, it is necessary to pay attention that the modification process cannot affect the original functions of the bioactive peptides and to avoid the generation of harmful substances.
Due to the susceptibility of bioactive peptides to loss of physiological activity under different pH values and the action of digestive enzymes in the body, using a delivery system to encapsulate bioactive peptides can effectively eliminate the bitter taste while improving the stability of peptides in systemic circulation. Colloidal delivery systems have been widely applied in the delivery of bioactive peptides. Common colloidal delivery systems include liposomes, emulsions, polymer nanoparticles, and hydrogels, as illustrated in Figure 3.
Figure 3. Colloidal delivery system structure. Liposomes (a); Emulsions (b,c); Polymer nanoparticles (d); Hydrogels (e).
Liposomes are a kind of spherical closed vesicle formed by concentric phospholipid molecules linked end to end through hydrophobic interactions, which can protect the loaded materials from being broken down by enzymes and improve their bioavailability in the body (Figure 3a) (67). Gong et al. (68) the bioavailability of peanut peptides was effectively improved after being encapsulated in nanoliposomes. The main reason is that the nanoliposomes prepared in this study exhibited good stability under different pH conditions and different morphologies, which allows the peanut peptides encapsulated in the nanoliposomes to retain a relatively complete structure and high ACE inhibitory activity. Compared with other delivery systems, liposomes have the advantages of easy encapsulation, large encapsulation capacity, and minimal residual organic solvents. Liposomes can encapsulate both hydrophobic and hydrophilic bioactive peptides. Hydrophobic peptides can be embedded within the phospholipid bilayer, while hydrophilic peptides can be encapsulated in the aqueous core (69).
However, liposomes also have some limitations. Firstly, the phospholipid membrane of liposomes is sensitive to adverse factors such as high temperature, enzymes, and ionic strength. These adverse factors may cause the liposomes to decompose during storage or before reaching the small intestine, causing the bioactive peptides wrapped inside to leak out in advance (70). To overcome this limitation, researchers have found that surface modification of liposomes with polymers such as chitosan, pectin, and polyethylene glycol can effectively improve the stability and sustained release ability of liposomes (71). Ramezanzade et al. (72) developed a novel composite nano-carrier of triphosphorus sodium cross-linked chitosan coated liposomes, and differential scanning calorimetry showed that this composite nano-carrier had better thermal stability than ordinary liposomes. Wu et al. (73) used sodium alginate (SA) to coated liposomes containing DPP-IV inhibitory collagen peptides and found that compared with uncoated liposomes, SA-coated collagen peptide liposomes exhibited higher storage stability, gastrointestinal stability and transcellular permeability. Secondly, due to the large size structure of liposomes, they may not be absorbed by intestinal epithelial cells, and the penetration mechanism of liposomes is not yet clear. Therefor the best approach is to choose vesicles as small as possible for the delivery of active substances, with particle diameters below 100–200 nm (Figure 4) (74). Additionally, cationic charged liposomes are often chosen to deliver bioactive substances because they are more easily attracted to the negatively charged mucus layer. Cuomo et al. (75) employed liposomes for the oral delivery of all-trans-retinoic acid and observed that cationic liposomes could interact with saliva in the oral cavity, which carries a net negative charge. Importantly, when cationic liposomes were coated with mucoproteins from oral saliva, the charge on the cationic surface interaction changed from positive to negative. This prevented the liposomes from being attracted to the negatively charged mucus layer during other stages of digestion, providing further protection for the loaded molecules.
An emulsion is a thermodynamically unstable colloidal dispersion formed by two immiscible liquids (usually oil and water), in which one liquid is dispersed as small droplets in the other liquid (76). According to their structural characteristics, emulsions can be divided into single-layer emulsions (water-in-oil, oil-in-water) (Figure 3b) and multi-layer emulsions (water-in-oil-in-water, oil-in-water-in-oil) (Figure 3c) (77). As a complex multi-phase system, multi-layer emulsion has various system types, among which W1/O/W2 is the most commonly used in food. The main structural state of W1/O/W2 type emulsions is that small water droplets (internal water phase, W1 phase) are trapped in larger oil droplets, and are subsequently dispersed in the external water phase (W2 phase). Multi-layer emulsions are complex multiphase systems. W1/O/W2 type is more common in food, where small water droplets (inner aqueous phase, W1 phase) are trapped in larger oil droplets, which are then dispersed in the outer aqueous phase (W2 phase) (78). Like singlelayer emulsions, the formation of multilayer emulsions also requires the addition of emulsifiers. Previous studies have found that the type of emulsifier can affect the stability of multilayer emulsions. Jo et al. (79) found that the hydrophilic and lipophilic balance value of the emulsifier can significantly affect the stability of W1/O/W2 emulsion loaded with collagen peptides, and emulsifiers with significant amphiphilicity can make W1/O/W2 emulsion more stable. Ying et al. (80) used polyglycerol ricinoleate and modified starch as emulsifiers to successfully prepare an emulsion system with a soybean peptide encapsulation rate of more than 80%. The results of in vitro simulated gastrointestinal digestion showed that the emulsion system showed strong resistance to the decomposition of pepsin, and the retention rate of soybean peptide was higher than 70% after simulated gastric digestion. In some cases, even with the addition of emulsifiers, the properties of multilayer emulsions are still not stable enough. This is because the system has two interfaces with a large interfacial area, making the multiphase structure prone to destruction during storage (81). Currently, there are various methods to stabilize the structure of multiple emulsions. One effective method to improve the stability of multiple emulsions is to add proteins or polysaccharides to limit the movement of components. For example, the addition of gelatin to multiple emulsions could significantly improves their stability (82). Furthermore, studies have shown that emulsion delivery systems not only improve the gastrointestinal stability of peptides, but also have the characteristics of masking the bitter taste of bioactive peptides (79). Gao et al. (83) used water-in-oil high internal phase emulsions (W/O HIPE) to encapsulate bitter peptides and found that W/O HIPE had a significant masking effect on the bitter taste of peptides.
Although both single-layer emulsions and multi-layer emulsions need to be stabilized by adding emulsifiers, some synthetic low molecular weight surfactants still need to be considered for their potential harm to the human body (84). Specifically, surfactants with a high HLB (Hydrophilic–Lipophilic Balance) value may disrupt the skin barrier due to their strong interfacial activity, which can increase the skin’s permeability to harmful substances, leading to skin irritation and even triggering allergic reactions and skin inflammation. Secondly, during the preparation of emulsions, although surfactants are renowned for their emulsifying properties, there is also a risk of causing emulsion instability, such as phase separation, coalescence, or creaming. These instability phenomena not only affect the appearance and texture of the product but may also compromise its actual efficacy. Moreover, the interactions between surfactants and bioactive ingredients may lead to structural changes in the bioactive components, resulting in the loss of their original functions, which is crucial for maintaining the integrity of bioactive ingredients. Surfactants may interfere with the permeability and retention time of bioactive components, thereby affecting their distribution and metabolism within the organism, ultimately reducing their bioavailability and therapeutic effects (84). Therefore, researchers have been on the way to seek other safer methods to stabilize the emulsion structure. At this time, a special emulsion, Pickering emulsion, came into the attention of researchers. Cai et al. (85) found that the natural Pickering emulsion system formed by composite nanoparticles that interacted/conjugated antimicrobial peptide Parasin I with chitosan significantly improved the stability and antibacterial activity of Parasin I. The solid particles in Picorling emulsions are irreversibly adsorbed on the surface of the emulsion droplets and play a role in stabilizing the emulsion system. This characteristic of Picorling emulsion avoids the use of surfactants, so its advantage is that there is no need to consider the safety of surfactants in food systems (86). In view of the characteristics and high safety of Pickering emulsions, it has a large application space in the field of bioactive substance delivery, but its specific mechanism of action and application characteristics still require further extensive research.
Polymer nanoparticles are solid colloidal particles with an average particle size ranging from 10 to 1,000 nm (Figure 3d). Polymer nanoparticle delivery system is a kind of system that uses natural, semi-synthetic or synthetic polymer nanoparticles as delivery carriers to load bioactive substances through non-covalent methods such as electrostatic adsorption, hydrophobic interaction, hydrogen bonding and so on (87). Compared to lipid-based carriers and emulsions, polymer nanoparticles have a simple preparation process, smaller system size, better stability which can protect bioactive peptides from being decomposed in harsh gastrointestinal environments (88), thereby improving the oral bioavailability of bioactive peptides. Additionally, high lipid intake may in due obesity and cardiovascular diseases (89), while the commonly used materials of polymer nanoparticles are proteins, polysaccharides and their composite derivatives, such as gelatin, sodium alginate, chitosan, and their derivatives, etc. Thus, polymer nanoparticles are more healthier and easilier to be accepted by consumers. Currently, various polymer nanoparticle delivery systems have been designed and applied to bioactive peptides delivery. Zhu et al. (90) used lysozyme-xanthan gum nanoparticles as carriers of selenium-containing peptides and prepared lysozyme-xanthan gum-selenopeptide composite nanoparticles. In vitro release test results showed that the composite nanoparticles successfully delayed the release of selenium-containing peptides and improved their in vitro antioxidant activity. Uhl et al. (91) developed a surface-modified PLA nanoparticles that can be loaded with liraglutide, which increased the oral bioavailability of liraglutide by 4.5-fold.
Some polymers can reversibly open TJs between intestinal epithelial cells, help bioactive peptides to be transported through the paracellular pathway, and promote the penetration and absorption of bioactive peptides, such as chitosan and its derivatives (92). In addition, chitosan also has good degradability and is one of the commonly used materials for constructing polymer nanoparticle delivery systems (93). Auwal et al. (94) used sodium tripolyphosphate cross-linked chitosan nanoparticles as the carrier to encapsulate ACE-inhibitory peptides, and found that not only the physical and chemical stability of the peptides was significantly improved in vitro, but also the ACE inhibitory effect of the peptides was significantly improved after simulated gastrointestinal digestion. Han et al. (95) prepared a pH-sensitive complex through the electrostatic self-assembly of chitosan derivative N-trimethyl chitosan, peanut peptide, and sodium alginate. This complex exhibited a regular spherical shape with good stability, and the highest entrapment efficiency for peanut peptide reached 91%.
Hydrogel is a highly cross-linked hydrophilic polymer with a three-dimensional network structure and abundant pores that can absorb and retain a large amount of water (96) (Figure 3e). A hydrogel system is a very effective delivery system for bioactive peptides, which can be prepared by mixing bioactive peptides with a solution containing biopolymer molecules before gel formation, or also by loading bioactive peptides into a microgel after microgel formation (97). Ma et al. (98) developed a novel type of fish skin gelatin-based hydrogel that successfully loaded codfish peptides after gel formation and exhibited good mechanical properties and biocompatibility. Because different types of materials have greatly different molecular and physicochemical properties, the physical and chemical differences of materials have a greater impact on the encapsulation effect of the system. Therefore, when preparing hydrogels, materials need to be selected according to specific purposes and applications. Protein and polysaccharide are commonly used materials for the preparation of ingestible food-grade microgels. Huang et al. (99) used the emulsion template method to successfully loaded ACE inhibitory peptides into biopolymer microgels composed of chitosan and alginate, which effectively reduced the in vitro release rate of ACE-inhibitory peptides. Ma et al. (100) used hydrogel made of alginate and chitosan to contain sericin with anti-inflammatory activity, and animal experiments showed that sericin loaded by hydrogel could more effectively alleviate ulcerative colitis in mice. These experimental results indicate that hydrogels have great potential in oral delivery systems.
In addition, pH, temperature and other stimuli will lead to the morphological changes of some polymer hydrogels, which will eventually lead to the phase transition of hydrogels (101). The hydrogels with this phenomenon are called smart hydrogels, which can respond to environmental stimuli, also known as environmentally responsive hydrogels. Environmentally responsive hydrogels can make corresponding shrinkage and swelling changes when single or multiple changes occur in external temperature, pH, light, electric field, salinity and other conditions, ultimately achieving targeted release of bioactive peptides (102). The environmental responsiveness of smart hydrogels shows important application potential and value in the field of substance delivery. Specifically, some temperature responsive smart hydrogels can exhibit different morphologies through corresponding phase transitions at elevated or low temperatures depending on the ambient temperature. This temperature responsiveness allows the hydrogel to adjust the position and rate of drug release in response to fluctuations in body temperature or environmental temperature, resulting in precise delivery of internal embedding. For example, Chuang et al. (103) cleverly designed a thermosensitive hydrogel based on the fact that tumor tissue is slightly hotter than normal tissue. This hydrogel will precisely undergo phase transition and release the embedded drug in the high temperature environment of the tumor site, allowing effective tumor treatment with minimal drug damage to normal tissues. In addition, there are some pH-responsive smart hydrogels that can adjust their morphology or properties according to changes in environmental pH, a property that enables the embedded material to respond to release in a specific pH environment, such as the slightly acidic environment of tumor tissue or the acidic environment of the stomach. Xie et al. (104) designed a pH-sensitive hydrogel that expands and releases drugs in the acidic environment of the stomach, which could facilitate precision treatment of gastric ulcer sites. In addition to the temperature and pH response, some smart hydrogels can undergo morphological changes upon the induction of light, which are called photoresponsive hydrogels. In the treatment of skin diseases, Huiwen et al. (105) use photosensitive hydrogels to deliver drugs precisely to lesions, which can significantly reduce the damage of drugs to surrounding normal tissues and improve the accuracy and safety of treatment.
Due to their unique environmental responsiveness, smart hydrogels have the ability to precisely regulate the drug release process, which makes them show broad application prospects in the field of drug delivery. Similarly, with appropriate design and preparation strategies, smart hydrogels are also suitable for quantitative, timed, and site-directed delivery of bioactive peptides. Ye et al. (106) found that the pH-responsive carboxymethyl cellulose/polyvinyl alcohol hydrogel effectively prevented the release of soy peptides in the stomach and could basically achieve the directional release of soy peptides in the intestine. This precise delivery strategy not only enhances the retention rate of bioactive peptides but also significantly improves their bioavailability, thereby optimizing therapeutic effects. In addition, it needs to be acknowledged that although smart hydrogels can effectively control the directional release of bioactive peptides, because the human body environment is complex and changeable, the changes and safety of smart hydrogels in the body need to be further studied.
Another, it needs to be acknowledged that hydrogels also have some disadvantages that are difficult to avoid. Typically, hydrogels are very porous and have weak structural strength, which allows bioactive peptides (especially small peptides) to easily diffuse out of them. At present, some studies have shown that improving the capture rate of bioactive peptides by hydrogels by ensuring that the pores are small enough or enhancing the interaction between bioactive peptides and the biopolymer network within the microgel (107). Two polymers with complementary properties can form a double crosslinked hydrogel to increase the stability of the hydrogel (108). Chen et al. (109) successfully prepared strong gelatin hydrogels by dual-crosslinking gelatin with transglutaminase and carrageenan, which improved the mechanical properties and thermal stability of gelatin hydrogel. In addition, since hydrogels are mostly hydrophilic substances, they have certain limitations when embedding hydrophobic substances. Studies have found that polymerizing hydrogels with nanoparticles, micelles and cyclodextrins can significantly improve the encapsulation rate of hydrophobic substances in hydrogels. Shabkhiz et al. (110) successfully encapsulated a β-cyclodextrin inclusion complex containing glycyrrhizic acid and thyme essential oil into alginate hydrogel beads, increasing the peptide encapsulation rate to 89%. However, there are few reports on the use of this technology in bioactive peptide entrapment, and further investigation is required. In summary, with the further development of smart hydrogel delivery systems, more innovative breakthroughs will be achieved in the application of smart hydrogels in the delivery of bioactive peptides.
Bioactive peptides have garnered significant attention from researchers due to their diverse physiological activities. However, the bioavailability of orally delivered bioactive peptides is severely restricted by the natural barriers of the gastrointestinal digestive system, as well as the physical and chemical properties of the peptides themselves. To enhance the stability and bioavailability of oral bioactive peptides within the gastrointestinal environment, various strategies have been explored, including chemical structure modification, the use of penetration enhancers, and colloidal delivery systems (such as liposomes, emulsions, biopolymer nanoparticles, and hydrogels). Nevertheless, each strategy presents distinct limitations in practical applications.
Although chemical modification can effectively enhance the stability of bioactive peptides, alterations in their chemical structure may reduce biological activity or even result in the formation of harmful substances. PEs possess a strong ability to promote absorption; however, inappropriate use can compromise the integrity of the intestinal barrier and significantly impact intestinal health. Liposomes, which mimic the structure of biological membranes, facilitate interactions with cell membranes, thereby offering substantial advantages in improving drug bioavailability and targeting. Nevertheless, liposomes exhibit poor structural stability and are susceptible to external factors that can lead to rupture, fusion, and leakage of their contents. Additionally, the drug loading capacity of liposomes is often suboptimal due to limitations related to molecular size, charge, and hydrophobicity. Emulsions can effectively enhance the solubility and stability of drugs, but they face challenges such as poor dispersion stability and low bioavailability. Polymeric nanoparticles have garnered considerable attention due to their controllable particle size, excellent stability, and biocompatibility. However, improvements are still needed in their drug loading capacity, drug release efficiency, and targeting capabilities. Smart hydrogels exhibit high environmental responsiveness; however, their stability within the digestive system and the controlled release of embedded materials restrict their practical applications.
Recent research indicates that a single delivery system is insufficient to overcome all delivery challenges. As a result, hybrid delivery systems that combine various delivery methods are anticipated to emerge as a major research focus in oral delivery moving forward. With consumers increasingly prioritizing safety and health, the main research emphasis for the oral delivery of bioactive peptides will be on discovering natural, edible, and biocompatible materials that have low toxicity to serve as delivery carriers. Moreover, current design approaches for oral delivery systems mainly concentrate on overcoming the gastrointestinal barrier, while the targeting features of these systems have not been thoroughly investigated. As a result, a key area of research in the oral delivery of bioactive peptides will focus on creating targeted homeostasis within these systems. Additionally, most existing data on the oral delivery of bioactive peptides has come from in vitro or animal studies, with a lack of relevant clinical data. To effectively evaluate the impact of oral delivery systems for bioactive peptides on human health, clinical studies are necessary to determine if prolonged use of these systems could result in unexpected side effects in vivo. With ongoing technological advancements, it is expected that new hybrid delivery systems will be developed, leading to improved delivery of bioactive peptides.
XW: Writing – original draft. ZY: Writing – original draft. WZ: Conceptualization, Writing – review & editing. LX: Software, Supervision, Writing – review & editing. RL: Methodology, Visualization, Writing – review & editing. SC: Conceptualization, Methodology, Writing – review & editing.
The author(s) declare that financial support was received for the research, authorship, and/or publication of this article. This study was funded by the National Natural Science Foundation of China (32460557) and the Key Research and Development Project of Ningxia (Talent Introduction Project) (2022BSB03063).
The authors declare that the research was conducted in the absence of any commercial or financial relationships that could be construed as a potential conflict of interest.
All claims expressed in this article are solely those of the authors and do not necessarily represent those of their affiliated organizations, or those of the publisher, the editors and the reviewers. Any product that may be evaluated in this article, or claim that may be made by its manufacturer, is not guaranteed or endorsed by the publisher.
1. Manikkam, V, Vasiljevic, T, Donkor, ON, and Mathai, ML. A review of potential marine-derived hypotensive and anti-obesity peptides. Crit Rev Food Sci. (2016) 56:92–112. doi: 10.1080/10408398.2012.753866
2. Siklos, M, BenAissa, M, and Thatcher, GRJ. Cysteine proteases as therapeutic targets: does selectivity matter? A systematic review of calpain and cathepsin inhibitors. Acta Pharm Sin B. (2015) 5:506–19. doi: 10.1016/j.apsb.2015.08.001
3. Peighambardoust, SH, Karami, Z, Pateiro, M, and Lorenzo, JM. A review on health-promoting, biological, and functional aspects of bioactive peptides in food applications. Biomol Ther. (2021) 11:631. doi: 10.3390/biom11050631
4. Miner-Williams, WM, Stevens, BR, and Moughan, PJ. Are intact peptides absorbed from the healthy gut in the adult human? Nutr Res Rev. (2014) 27:308–29. doi: 10.1017/S0954422414000225
5. Geng, Y, Zheng, Y, Zhou, R, and Ma, M. Effect of supercritical carbon dioxide on protein structure modification and antimicrobial peptides production of Mongolian cheese and its in vitro digestion. Food Res Int. (2024) 191:114714. doi: 10.1016/j.foodres.2024.114714
6. Jiang, Z, Liu, S, Zhang, H, Li, Y, and Yuan, S. Contribution of chemical permeation enhancers to the process of transdermal drug delivery: adsorption, microscopic interactions, and mechanism. Colloids Surf B: Biointerfaces. (2024) 243:114138. doi: 10.1016/j.colsurfb.2024.114138
7. Han, J, Meade, J, Devine, D, Sadeghpour, A, Rappolt, M, and Goycoolea, FM. Chitosan-coated liposomal systems for delivery of antibacterial peptide LL17-32 to Porphyromonas gingivalis. Heliyon. (2024) 10:e34554. doi: 10.1016/j.heliyon.2024.e34554
8. Zhu, QG, Chen, ZJ, Paul, PK, Lu, Y, Wu, W, and Qi, JP. Oral delivery of proteins and peptides: challenges, status quo and future perspectives. Acta Pharm Sin B. (2021) 11:2416–48. doi: 10.1016/j.apsb.2021.04.001
9. Zhou, YJ, Zhang, Y, Hong, H, Luo, YK, Li, B, and Tan, YQ. Mastering the art of taming: reducing bitterness in fish by-products derived peptides. Food Res Int. (2023) 173:113241. doi: 10.1016/j.foodres.2023.113241
10. Kuhfeld, RF, Eshpari, H, Atamer, Z, and Dallas, DC. A comprehensive database of cheese-derived bitter peptides and correlation to their physical properties. Crit Rev Food Sci. (2023) 64:10105–19. doi: 10.1080/10408398.2023.2220792
11. Henriksen, AP, and Stahnke, LH. Sensory and chromatographic evaluations of water soluble fractions from dried sausages. J Agric Food Chem. (1997) 45:2679–84. doi: 10.1021/jf960792+
12. Fan, WW, Tan, XY, Xu, XB, Li, GD, Wang, ZY, and Du, M. Relationship between enzyme, peptides, amino acids, ion composition, and bitterness of the hydrolysates of Alaska Pollock frame. J Food Biochem. (2019) 43:e12801. doi: 10.1111/jfbc.12801
13. Bertelsen, AS, Laursen, A, Knudsen, TA, Moller, S, and Kidmose, U. Bitter taste masking of enzyme-treated soy protein in water and bread. J Sci Food Agr. (2018) 98:3860–9. doi: 10.1002/jsfa.8903
14. Saha, BC, and Hayashi, K. Debittering of protein hydrolyzates. Biotechnol Adv. (2001) 19:355–70. doi: 10.1016/S0734-9750(01)00070-2
15. Lei, FF, Zhao, QZ, Sun-Waterhouse, DX, and Zhao, MM. Characterization of a salt-tolerant aminopeptidase from marine Bacillus licheniformis SWJS33 that improves hydrolysis and debittering efficiency for soy protein isolate. Food Chem. (2017) 214:347–53. doi: 10.1016/j.foodchem.2016.07.028
16. Fu, Y, Chen, JR, Bak, KH, and Lametsch, R. Valorisation of protein hydrolysates from animal by-roducts: perspectives on bitter taste and debittering methods: a review. Int J Food Sci Tech. (2019) 54:978–86. doi: 10.1111/ijfs.14037
17. Dubey, SK, Parab, S, Dabholkar, N, Agrawal, M, Singhvi, G, Alexander, A, et al. Oral peptide delivery: challenges and the way ahead. Drug Discov Today. (2021) 26:931–50. doi: 10.1016/j.drudis.2021.01.001
18. Koziolek, M, Grimm, M, Becker, D, Iordanov, V, Zou, H, Shimizu, J, et al. Investigation of pH and temperature profiles in the GI tract of fasted human subjects using the Intellicap system. J Pharm Sci. (2015) 104:2855e63. doi: 10.1002/jps.24274
19. Jang, HL, Liceaga, AM, and Yoon, KY. Purification, characterisation and stability of an antioxidant peptide derived from sandfish (Arctoscopus japonicus) protein hydrolysates. J Funct Foods. (2016) 20:433–42. doi: 10.1016/j.jff.2015.11.020
20. Pei, JY, Gao, XC, Pan, DD, Hua, Y, He, J, Liu, Z, et al. Advances in the stability challenges of bioactive peptides and improvement strategies. Curr Res Food Sci. (2022) 5:2162–70. doi: 10.1016/j.crfs.2022.10.031
21. Li, GH, Qu, MR, Wan, JZ, and You, JM. Antihypertensive effect of rice protein hydrolysate with in vitro angiotensin I-converting enzyme inhibitory activity in spontaneously hypertensive rats. Asia Pac J Clin Nutr. (2006) 16:275–80.
22. Chai, JJ, Jiang, P, Wang, PJ, Jiang, YM, Li, D, Bao, WE, et al. The intelligent delivery systems for bioactive compounds in foods: physicochemical and physiological conditions, absorption mechanisms, obstacles and responsive strategie. Trends Food Sci Tech. (2018) 78:144–54. doi: 10.1016/j.tifs.2018.06.003
23. Wagner, CE, Wheeler, KM, and Ribbeck, K. Mucins and their role in shaping the functions of mucus barrier. Annu Rev Cell Dev Biol. (2018) 34:189–215. doi: 10.1146/annurev-cellbio-100617-062818
24. Bansil, R, and Turner, BS. The biology of mucus: composition, synthesis and organizatio. Adv Drug Deliv Rev. (2018) 124:3–15. doi: 10.1016/j.addr.2017.09.023
25. Capaldo, CT, Powell, DN, and Kalman, D. Layered defense: how mucus and tight junctions seal the intestinal Barrie. Mol Med. (2017) 95:927e34. doi: 10.1007/s00109-017-1557-x
26. Bougle, D, and Bouhallab, S. Dietary bioactive peptides: human studies. Crit Rev Food Sci Nut. (2017) 57:335–43. doi: 10.1080/10408398.2013.873766
27. Chen, M, and Li, B. The effect of molecular weights on the survivability of casein-derived antioxidant peptides after the simulated gastrointestinal digestion. Innov Food Sci Emerg. (2012) 16:341–8. doi: 10.1016/j.ifset.2012.07.009
28. Shen, W, and Matsui, T. Intestinal absorption of small peptides: a review. Int J Food Sci Tech. (2019) 54:1942–8. doi: 10.1111/ijfs.14048
29. Savoie, L, Agudelo, RA, Gauthier, SF, Marin, J, and Pouliot, Y. In vitro determination of the release kinetics of peptides and free amino acids during the digestion of food proteins. J AOAC Int. (2005) 88:935–48. doi: 10.1093/jaoac/88.3.935
30. Udenigwe, CC, and Fogliano, V. Food matrix interaction and bioavailability of bioactive peptides: two faces of the same coin? J Funct Foods. (2017) 35:9–12. doi: 10.1016/j.jff.2017.05.029
31. Vig, BS, Stouch, TR, Timoszyk, JK, Quan, Y, Wall, DA, Smith, RL, et al. Human PEPT1 pharmacophore distinguishes between dipeptide transport and binding. J Med Chem. (2006) 49:3636–44. doi: 10.1021/jm0511029
32. Wang, B, and Li, B. Charge and hydrophobicity of casein peptides influence transepithelial transport and bioavailabilit. Food Chem. (2018) 245:646–52. doi: 10.1016/j.foodchem.2017.09.032
33. Xu, QB, Hong, H, Wu, JP, and Yan, XH. Bioavailability of bioactive peptides derived from food proteins across the intestinal epithelial membrane: a review. Trends Food Sci Tech. (2019) 86:399–411. doi: 10.1016/j.tifs.2019.02.050
34. Bo, W, Ningning, X, and Bo, L. Influence of peptide characteristics on their stability, intestinal transport, and in vitro bioavailability: a review. J Food Biochem. (2018) 43:e12571. doi: 10.1111/jfbc.12571
35. Parker, JL, Mindell, JA, and Newstead, S. One transporter, two mechanisms. Thermodynamic evidence for a dual transport mechanism in a POT family peptide transporter. eLife. (2014) 3:3. doi: 10.7554/eLife.04273
36. Brandsch, M. Transport of drugs by proton-coupled peptide transporters: pearls and pitfalls. Expert Opin Drug Met. (2009) 5:887–905. doi: 10.1517/17425250903042292
37. Fan, HB, Xu, QB, Hong, H, and Wu, JP. Stability and transport of spent hen-derived ACE-inhibitory peptides IWHHT, IWH, and IW in human intestinal Caco-2 cell monolayers. J Agric Food Chem. (2018) 66:11347–54. doi: 10.1021/acs.jafc.8b03956
38. Linnankoski, J, Mäkelä, J, Palmgren, J, Mauriala, T, Vedin, C, Ungell, AL, et al. Paracellular porosity and pore size of the human intestinal epithelium in tissue and cell culture models. J Pharm Sci. (2010) 99:2166–75. doi: 10.1002/jps.21961
39. Bakke, S, Jordal, A, Gómez-Requeni, P, Verri, T, Kousoulaki, K, Aksnes, A, et al. Dietary protein hydrolysates and free amino acids affect the spatial expression of peptide transporter PepT1 in the digestive tract of Atlantic cod (Gadus morhua). Comp Biochem Physiol B Biochem Mol Biol. (2010) 156:48–55. doi: 10.1016/j.cbpb.2010.02.002
40. Pedretti, A, De Luca, L, Marconi, C, Negrisoli, G, Aldini, G, and Vistoli, G. Modeling of the intestinal peptide transporter hPepT1 and analysis of its transport capacities by docking and pharmacophore mapping. ChemMedChem. (2008) 3:1913–21. doi: 10.1002/cmdc.200800184
41. Salamat-Miller, N, and Johnston, TP. Current strategies used to enhance the paracellular transport of therapeutic polypeptides across the intestinal epithelium. Int J Pharm. (2005) 294:201–16. doi: 10.1016/j.ijpharm.2005.01.022
42. Wang, CY, Liu, S, Xie, XN, and Tan, ZR. Regulation profile of the intestinal peptide transporter 1 (PepT1). Drug Des Dev Ther. (2017) 11:3511–7. doi: 10.2147/DDDT.S151725
43. Marchiando, AM, Graham, WV, and Turner, JR. Epithelial barriers in homeostasis and disease. Annu Rev Pathol Mech. (2010) 5:119–44. doi: 10.1146/annurev.pathol.4.110807.092135
44. Karaś, M. Influence of physiological and chemical factors on the absorption of bioactive peptides. Int J Food Sci Tech. (2019) 54:1486–96. doi: 10.1111/ijfs.14054
45. Regazzo, D, Molle, D, Gabai, G, Tome, D, Dupont, D, Leonil, G, et al. The (193–209) 17-residues peptide of bovine β-casein is transported through Caco-2monolayer. Mol Nutr Food Res. (2010) 54:1428–35. doi: 10.1002/mnfr.200900443
46. Xu, F, Wang, LF, Ju, XR, Zhang, J, Yin, S, Shi, JY, et al. Transepithelial transport of YWDHNNPQIR and its metabolic fate with cytoprotection against oxidative stress in human intestinal Caco-2 cells. J Agric Food Chem. (2017) 65:2056–65. doi: 10.1021/acs.jafc.6b04731
47. Aungst, BJ. Absorption enhancers: applications and advances. AAPS J. (2012) 14:10–8. doi: 10.1208/s12248-011-9307-4
48. Ben-Shlomo, A, Liu, N-A, and Melmed, S. Somatostatin and dopamine receptor regulation of pituitary somatotroph adenomas. Pituitary. (2017) 20:93–9. doi: 10.1007/s11102-016-0778-2
49. Brayden, DJ, Hill, TA, Fairlie, DP, Maher, S, and Mrsny, RJ. Systemic delivery of peptides by the oral route: formulation and medicinal chemistry approaches. Adv Drug Deliv Rev. (2020) 157:2–36. doi: 10.1016/j.addr.2020.05.007
50. Maher, S, Mrsny, RJ, and Brayden, DJ. Intestinal permeation enhancers for oral peptide delivery. Adv Drug Deliv Rev. (2016) 106:277–319. doi: 10.1016/j.addr.2016.06.005
51. Welling, SH, Hubálek, F, Jacobsen, J, Brayden, DJ, Rahbek, UL, and Buckley, ST. Approaches for enhancing oral bioavailability of peptides and proteins. Int J Pharm. (2013) 447:75–93. doi: 10.1016/j.ijpharm.2013.02.030
52. Aguirre, TAS, Teijeiro-Osorio, D, Rosa, M, Coulter, IS, Alonso, MJ, and Brayden, DJ. Current status of selected oral peptide technologies in advanced preclinical development and in clinical trials. Adv Drug Deliver Rev. (2016) 106:223–41. doi: 10.1016/j.addr.2016.02.004
53. Sood, A, and Panchagnula, R. Peroral route: an opportunity for protein and peptide drug delivery. Chem Rev. (2001) 101:3275–304. doi: 10.1021/cr000700m
54. Liu, Y, Zhao, Z, and Li, M. Overcoming the cellular barriers and beyond: recent progress on cell penetrating peptide modified nanomedicine in combating physiological and pathological barriers. Asian J Pharm Sci. (2022) 17:523–43. doi: 10.1016/j.ajps.2022.05.002
55. Knoll, P, Hörmann, N, Le, NN, Wibel, R, Gust, R, and Bernkop-Schnürch, A. Charge converting nanostructured lipid carriers containing a cell-penetrating peptide for enhanced cellular uptake. J Colloid Interf Sci. (2022) 628:463–75. doi: 10.1016/j.jcis.2022.07.160
56. Dinca, A, Chien, WM, and Chin, MT. Intracellular delivery of proteins with cell-penetrating peptides for therapeutic uses in human disease. Int J Mol Sci. (2016) 17:263. doi: 10.3390/ijms17020263
57. Yao, JF, Yang, H, Zhao, YZ, and Xue, M. Metabolism of peptide drugs and strategies to improve their metabolic stability. Curr Drug Metab. (2018) 19:892–901. doi: 10.2174/1389200219666180628171531
58. Maack, T, Johnson, V, Kau, ST, Figueiredo, J, and Sigulem, D. Renal filtration, transport, and metabolism of low-molecular-weight proteins: a review. Kidney Int. (1979) 16:251–70. doi: 10.1038/ki.1979.128
59. Zhou, K, Zheng, XQ, Xu, HM, Zhang, J, Chen, YQ, Xi, T, et al. Studies of poly (ethylene glycol) modification of HM-3 polypeptides. Bioconjug Chem. (2009) 20:932–6. doi: 10.1021/bc900070r
60. Wang, B. Studies on the pegylation conditions of polypeptide CPU-HM and pharmacodynamics of modified products in vivo. Chinese J Pharm Biotechnol. (2016) 23:313–7.
61. Gentilucci, L, De Marco, R, and Cerisoli, L. Chemical modifications designed to improve peptide stability: incorporation of non-natural amino acids, pseudo-peptide bonds, and cyclization. Curr Pharm Des. (2010) 16:3185–203. doi: 10.2174/138161210793292555
62. Matsui, K, Kimura, T, Ota, K, Iitake, K, Shoji, M, Inoue, M, et al. Resistance of 1-Deamino [8-D-Argininei]-vasopressin to in vitro degradation as compared with arginine vasopressin. Endocrinol Japon. (1985) 32:547–57. doi: 10.1507/endocrj1954.32.547
63. Piekielna, J, Perlikowska, R, Gach, K, and Janecka, A. Cyclization in opioid peptides. Curr Drug Targets. (2013) 14:798–816. doi: 10.2174/1389450111314070008
64. Wang, CK, and Craik, DJ. Cyclic peptide oral bioavailability: lessons from the past. Biopolymers. (2016) 106:901–9. doi: 10.1002/bip.22878
65. Song, WT, Fu, JX, Zeng, Q, Lu, HY, Wang, J, Fang, L, et al. Improving ACE inhibitory activity of hazelnut peptide modified by plastein: physicochemical properties and action mechanism. Food Chem. (2023) 402:134498. doi: 10.1016/j.foodchem.2022.134498
66. Jiang, SS, Zhao, YH, Shen, QQ, Zhu, XJ, Dong, SY, Liu, ZY, et al. Modification of ACE-inhibitory peptides from Acaudina molpadioidea using the plastein reaction and examination of its mechanism. Food Biosci. (2018) 26:1–7. doi: 10.1016/j.fbio.2018.08.008
67. McClements, DJ. Encapsulation, protection, and delivery of bioactive proteins and peptides using nanoparticle and microparticle systems: a review. Adv Colloid Interface. (2018) 253:1–22. doi: 10.1016/j.cis.2018.02.002
68. Gong, KJ, Shi, AM, Liu, HZ, Liu, L, Hu, H, Yang, Y, et al. Preparation of nanoliposome loaded with peanut peptide fraction: stability and bioavailability. Food Funct. (2016) 7:2034–42. doi: 10.1039/C5FO01612F
69. Ajeeshkumar, KK, Aneesh, PA, Raju, N, Suseela, M, Ravishankar, CN, and Benjakul, S. Advancements in liposome technology: preparation techniques and applications in food, functional foods, and bioactive delivery: a review. Comp Rev Food Sci Food Saf. (2021) 20:1280–306. doi: 10.1111/1541-4337.12725
70. Taylor, TM, Davidson, PM, Bruce, BD, and Weiss, J. Liposomal nanocapsules in food science and agriculture. Crit Rev Food Sci. (2005) 45:587–605. doi: 10.1080/10408390591001135
71. Shishir, MRI, Karim, N, Gowd, V, Zheng, XD, and Chen, W. Liposomal delivery of natural product: a promising approach in health research. Trends Food Sci Tech. (2019) 85:177–200. doi: 10.1016/j.tifs.2019.01.013
72. Ramezanzade, L, Hosseini, SF, Akbari-Adergani, B, and Yaghmur, A. Cross-linked chitosan-coated liposomes for encapsulation of fish-derived peptide. Ciencia Tecnol Alime. (2021) 150:112057. doi: 10.1016/j.lwt.2021.112057
73. Wu, P, Chen, L, Chen, MS, Chiou, BS, Xu, FF, Liu, F, et al. Use of sodium alginate coatings to improve bioavailability of liposomes containing DPP-IV inhibitory collagen peptides. Food Chem. (2023) 414:135685. doi: 10.1016/j.foodchem.2023.135685
74. Dymek, M, and Sikora, E. Liposomes as biocompatible and smart delivery systems–the current state. Adv Colloid Interface. (2022) 309:102757. doi: 10.1016/j.cis.2022.102757
75. Cuomo, F, Ceglie, S, Miguel, M, Lindman, B, and Lopez, F. Oral delivery of all-trans retinoic acid mediated by liposome carriers. Colloid Surf B. (2021) 201:111655. doi: 10.1016/j.colsurfb.2021.111655
76. Lin, D, Kelly, AL, and Miao, S. Preparation, structure-property relationships and applications of different emulsion gels: Bulk emulsion gels, emulsion gel particles, and fluid emulsion gels. Trends Food Sci Technol. (2020) 102:123–37. doi: 10.1016/j.tifs.2020.05.024
77. Gurpreet, K, and Singh, SK. Review of nanoemulsion formulation and characterization techniques. Indian J Pharm Sci. (2018) 80:781–9.
78. Neumann, SM, Scherbej, I, Van der Schaaf, US, and Karbstein, HP. Investigations on the influence of osmotic active substances on the structure of water in oil emulsions for the application as inner phase in double emulsions. Colloid Surf A. (2018) 538:56–62. doi: 10.1016/j.colsurfa.2017.10.073
79. Jo, YJ, Karbstein, HP, and Van der Schaaf, US. Collagen peptide-loaded W 1/O single emulsions and W 1/O/W 2 double emulsions: influence of collagen peptide and salt concentration, dispersed phase fraction and type of hydrophilic emulsifier on droplet stability and encapsulation efficiency. Food Funct. (2019) 10:3312–23. doi: 10.1039/C8FO02467G
80. Ying, X, Gao, JX, Lu, J, Ma, CL, Lv, JP, Adhikari, B, et al. Preparation and drying of water-in-oil-in-water (W/O/W) double emulsion to encapsulate soy peptides. Food Res Int. (2021) 141:110148. doi: 10.1016/j.foodres.2021.110148
81. Ma, L, Wan, Z, and Yang, X. Multiple water-in-oil-in-water emulsion gels based on self-assembled saponin fibrillar network for photosensitive cargo protection. J Agric Food Chem. (2017) 65:9735–43. doi: 10.1021/acs.jafc.7b04042
82. Tang, XY, Wang, ZM, Meng, HC, Lin, JW, Guo, XM, Zhang, T, et al. Robust W/O/W emulsion stabilized by genipin-cross-linked sugar beet pectin-bovine serum albumin nanoparticles: co-encapsulation of betanin and curcumin. J Agric Food Chem. (2021) 69:1318–28. doi: 10.1021/acs.jafc.0c05212
83. Gao, Y, Wu, XL, McClements, DJ, Cheng, C, Xie, YF, Liang, RH, et al. Encapsulation of bitter peptides in water-in-oil high internal phase emulsions reduces their bitterness and improves gastrointestinal stability. Food Chem. (2022) 386:132787. doi: 10.1016/j.foodchem.2022.132787
84. Chassaing, B, Koren, O, Goodrich, JK, Poole, AC, Srinivasan, S, Ley, RE, et al. Dietary emulsifiers impact the mouse gut microbiota promoting colitis and metabolic syndrome. Nature. (2015) 519:92–6. doi: 10.1038/nature14232
85. Cai, L, Cao, M, and Regenstein, J. Slow-release and nontoxic Pickering emulsion platform for antimicrobial peptide. J Agric Food Chem. (2020) 68:7453–66. doi: 10.1021/acs.jafc.0c00874
86. Cheon, J, Haji, F, Baek, J, Wang, Q, and Tam, KC. Pickering emulsions for functional food systems. J Agric Food Res. (2023) 11:100510. doi: 10.1016/j.jafr.2023.100510
87. Mitchell, MJ, Billingsley, MM, Haley, RM, Wechsler, ME, Peppas, NA, and Langer, R. Engineering precision nanoparticles for drug delivery. Nat Rev Drug Discov. (2020) 20:101–24. doi: 10.1038/s41573-020-0090-8
88. Alexander, AEA, Ajazuddin, M, Swarna, M, Sharma, M, and Tripathi, DK. Polymers and permeation enhancers: specialized components of Mucoadhesives. J Pharm Sci. (2011) 4:91–5. doi: 10.3329/sjps.v4i1.8878
89. Kharat, M, and McClements, DJ. Recent advances in colloidal delivery systems for nutraceuticals: a case study–delivery by design of curcumin. J Colloid Interf Sci. (2019) 557:506–18. doi: 10.1016/j.jcis.2019.09.045
90. Zhu, YQ, Sun, XY, Luo, XQ, Ding, J, Fan, FJ, Li, P, et al. Encapsulation of selenium-containing peptides in xanthan gum-lysozyme nanoparticles as a powerful gastrointestinal delivery system. Food Res Int. (2022) 156:111351. doi: 10.1016/j.foodres.2022.111351
91. Uhl, P, Grundmann, C, Sauter, M, Storck, P, Tursch, A, Özbek, S, et al. Coating of PLA-nanoparticles with cyclic, arginine-rich cell penetrating peptides enables oral delivery of liraglutide. Nanomed Nanotechnol. (2020) 24:102132. doi: 10.1016/j.nano.2019.102132
92. Sonaje, K, Chuang, EY, Lin, KJ, Yen, TC, Su, FY, Tseng, MT, et al. Opening of epithelial tight junctions and enhancement of paracellular permeation by chitosan: microscopic, ultrastructural, and computed-tomographic observations. Mol Pharm. (2012) 9:1271–9. doi: 10.1021/mp200572t
93. Wu, DJ, Zhu, LX, Li, Y, Zhang, XL, Xu, SM, Yang, GS, et al. Chitosan-based colloidal polyelectrolyte complexes for drug delivery: a review. Carbohyd Polym. (2020) 238:116126. doi: 10.1016/j.carbpol.2020.116126
94. Auwal, SM, Zarei, M, Tan, CP, Basri, M, and Saari, N. Enhanced physicochemical stability and efficacy of angiotensin I-converting enzyme (ACE)-inhibitory biopeptides by chitosan nanoparticles optimized using Box-Behnken design. Sci Rep. (2018) 8:10411. doi: 10.1038/s41598-018-28659-5
95. Han, CT, Fang, L, Song, SX, and Min, WH. Polysaccharides-based delivery system for efficient encapsulation and controlled release of food-derived active peptides. Carbohyd Polym. (2022) 291:119580. doi: 10.1016/j.carbpol.2022.119580
96. Cascone, S, and Lamberti, G. Hydrogel-based commercial products for biomedical applications: a review. Int J Pharm. (2020) 573:118803. doi: 10.1016/j.ijpharm.2019.118803
97. Perry, SL, and McClements, DJ. Recent advances in encapsulation, protection, and oral delivery of bioactive proteins and peptides using colloidal systems. Molecules. (2020) 25:1161. doi: 10.3390/molecules25051161
98. Ma, WC, Yang, ML, Wu, D, Li, Y, Wang, LS, El-Seedi, HR, et al. Fish skin gelatin-based adhesive hydrogels loading cod peptides with osteogenic activity for bone tissue engineering. Colloid Surf A. (2023) 673:131695. doi: 10.1016/j.colsurfa.2023.131695
99. Huang, GQ, Xiao, JX, Hao, LQ, and Yang, J. Microencapsulation of an angiotensin I-converting enzyme inhibitory peptide VLPVP by membrane emulsification. Food Bioprocess Tech. (2017) 10:2005–12. doi: 10.1007/s11947-017-1953-9
100. Ma, Y, Tong, XL, Huang, YM, Zhou, X, Yang, CH, Chen, JC, et al. Oral administration of hydrogel-embedding silk sericin alleviates ulcerative colitis through wound healing, anti-inflammation, and anti-oxidation. ACS Biomater Sci Eng. (2019) 5:6231–42. doi: 10.1021/acsbiomaterials.9b00862
101. Suzuki, A, and Tanaka, T. Phase transition in polymer gels induced by visible light. Nature. (1990) 346:345–7. doi: 10.1038/346345a0
102. Suljovrujic, E, and Micic, M. Smart poly (oligo (propylene glycol) methacrylate) hydrogel prepared by gamma radiation. Nucl Instrum Meth B. (2015) 342:206–14. doi: 10.1016/j.nimb.2014.10.008
103. Chuang, S-H, Chen, K-J, Cheng, Y-T, Chen, Y-S, Lin, S-Y, Chou, H-Y, et al. A thermo-responsive chemically crosslinked long-term-release chitosan hydrogel system increases the efficiency of synergy chemo-immunotherapy in treating brain tumors. Int J Biol Macromol. (2024) 280:135894. doi: 10.1016/j.ijbiomac.2024.135894
104. Xie, R, Yan, X, Jing, Y, Shen, K, Zhang, M, Li, M, et al. pH-responsive bioadhesive with robust and stable wet adhesion for gastric ulcer healing. Biomaterials. (2024) 309:122599. doi: 10.1016/j.biomaterials.2024.122599
105. Huiwen, H, Sun, H, Jiang, Z, Wang, S, Liu, C, Zou, M, et al. Study on repair of abdominal wall defect rats with hernia mesh coated with chitosan-based photosensitive hydrogel. Carbohydr Polym. (2022) 291:119577. doi: 10.1016/j.carbpol.2022.119577
106. Ye, J, Liu, L, Lan, W, and Xiong, J. Targeted release of soybean peptide from CMC/PVA hydrogels in simulated intestinal fluid and their pharmacokinetics. Carbohyd Polym. (2023) 310:120713. doi: 10.1016/j.carbpol.2023.120713
107. McClements, DJ. Recent progress in hydrogel delivery systems for improving nutraceutical bioavailability. Food Hydrocolloid. (2017) 68:238–45. doi: 10.1016/j.foodhyd.2016.05.037
108. Liu, K, Chen, YY, Zha, XQ, Li, QM, Pan, LH, and Luo, JP. Research progress on polysaccharide/protein hydrogels: preparation method, functional property and application as delivery systems for bioactive ingredients. Food Res Int. (2021) 147:110542. doi: 10.1016/j.foodres.2021.110542
109. Chen, HR, Wu, D, Ma, WC, Wu, C, Liu, J, and Du, M. Strong fish gelatin hydrogels double crosslinked by transglutaminase and carrageenan. Food Chem. (2022) 376:131873. doi: 10.1016/j.foodchem.2021.131873
110. Shabkhiz, MA, Pirouzifard, MK, Pirsa, S, and Mahdavinia, GR. Alginate hydrogel beads containing Thymus daenensis essential oils/Glycyrrhizic acid loaded in β-cyclodextrin. Investigation of structural, antioxidant/antimicrobial properties and release assessment. J Mol Liq. (2021) 344:117738. doi: 10.1016/j.molliq.2021.117738
111. Bejjani, S, and Wu, J. Transport of IRW, an ovotransferrin-derived antihypertensive peptide, in human intestinal epithelial caco-2 cells. J Agric Food Chem. (2013) 61:1487–92. doi: 10.1021/jf302904t
112. Gleeson, JP, Brayden, DJ, and Ryan, SM. Evaluation of PepT1 transport of food-derived antihypertensive peptides, Ile-pro-pro and Leu-Lys-pro using in vitro, ex vivo and in vivo transport models. Eur J Pharm Biopharm. (2017) 115:276–84. doi: 10.1016/j.ejpb.2017.03.007
113. Satake, M, Enjoh, M, Nakamura, Y, Takano, T, Kawamura, Y, Arai, S, et al. Transepithelial transport of the bioactive tripeptide, val-pro-pro, in human intestinal Caco-2 cell monolayers. Biosci Biotech Bioch. (2002) 66:378–84. doi: 10.1271/bbb.66.378
114. Xu, QB, Fan, HB, Yu, WL, Hong, H, and Wu, JP. Transport study of egg-derived antihypertensive peptides (LKP and IQW) using Caco-2 and HT29 coculture monolayers. J Agric Food Chem. (2017) 65:7406–14. doi: 10.1021/acs.jafc.7b02176
115. Lin, QL, Xu, QB, Bai, J, Wu, W, Hong, H, and Wu, JP. Transport of soybean protein-derived antihypertensive peptide LSW across Caco-2 monolayers. J Funct Foods. (2017) 39:96–102. doi: 10.1016/j.jff.2017.10.011
116. Miguel, M, Dávalos, A, Manso, MA, de la Peña, G, Lasunción, MA, and López-Fandiño, R. Transepithelial transport across Caco-2 cell monolayers of antihypertensive egg-derived peptides. PepT1-mediated flux of tyr-pro-ile. Mol Nutr Food Res. (2008) 52:1507–13. doi: 10.1002/mnfr.200700503
117. Ding, L, Wang, LY, Zhang, Y, and Liu, JB. Transport of antihypertensive peptide RVPSL, ovotransferrin 328–332, in human intestinal Caco-2 cell monolayers. J Agric Food Chem. (2015) 63:8143–50. doi: 10.1021/acs.jafc.5b01824
118. Sun, HY, Liu, D, Li, SM, and Qin, ZY. Transport of val-leu-pro-val-pro in human intestinal epithelial (Caco-2) cell monolayers. J Agric Food Chem. (2008) 56:3582–6. doi: 10.1021/jf703640p
119. Quiros, A, Dávalos, A, Lasunción, MA, Ramos, M, and Recio, I. Bioavailability of the antihypertensive peptide LHLPLP: transepithelial flux of HLPLP. Int Dairy J. (2008) 18:279–86. doi: 10.1016/j.idairyj.2007.09.006
120. Yu, SF, Wang, WJ, Bu, TT, Zhao, RA, Niu, RH, Liu, L, et al. Digestion, absorption, and transport properties of soy-fermented douchi hypoglycemic peptides VY and SFLLR under simulated gastrointestinal digestion and Caco-2 cell monolayers. Food Res Int. (2023) 164:112340. doi: 10.1016/j.foodres.2022.112340
121. Pei, JY, Liu, Z, Pan, DD, Zhao, YF, Dang, YL, and Gao, XC. Transport, stability, and in vivo hypoglycemic effect of a broccoli-derived DPP-IV inhibitory peptide VPLVM. J Agric Food Chem. (2022) 70:4934–41. doi: 10.1021/acs.jafc.1c08191
122. Gao, JJ, Guo, YX, and Pan, DD. Intestinal absorption of milk-derived ace inhibitory peptides LL and LPEW using Caco-2 cell model. Food Sci. (2017) 38:214–9.
123. Ding, L, Wang, LY, Zhang, T, Yu, ZP, and Liu, JB. Hydrolysis and transepithelial transport of two corn gluten derived bioactive peptides in human Caco-2 cell monolayers. Food Res Int. (2018) 106:475–80. doi: 10.1016/j.foodres.2017.12.080
124. Eckert, E, Zambrowicz, A, Pokora, M, Setner, B, Dabrowska, A, Szoltysik, M, et al. Egg-yolk protein by-product as a source of ACE-inhibitory peptides obtained with using unconventional proteinase from Asian pumpkin (Cucurbita ficifolia). J Proteome. (2014) 110:107–16. doi: 10.1016/j.jprot.2014.08.003
125. Welling, SH, Hubálek, F, Jacobsen, J, Brayden, DJ, Rahbek, UL, and Buckley, ST. The role of citric acid in oral peptide and protein formulations: relationship between calcium chelation and proteolysis inhibition. Eur J Pharm Biopharm. (2014) 86:544–51. doi: 10.1016/j.ejpb.2013.12.017
126. Twarog, C, Fattah, S, Heade, J, Maher, S, Fattal, E, and Brayden, DJ. Intestinal permeation enhancers for oral delivery of macromolecules: a comparison between salcaprozate sodium (SNAC) and sodium caprate (C10). Pharmaceutics. (2019) 11:78. doi: 10.3390/pharmaceutics11020078
127. Niu, MM, Tan, YN, Guan, PP, Hovgaard, L, Lu, Y, Qi, JP, et al. Enhanced oral absorption of insulin-loaded liposomes containing bile salts: a mechanistic study. Int J Pharm. (2014) 460:119–30. doi: 10.1016/j.ijpharm.2013.11.028
128. Gleeson, JP, Frías, JM, Ryan, SM, and Brayden, DJ. Sodium caprate enables the blood pressure-lowering effect of Ile-pro-pro and Leu-Lys-pro in spontaneously hypertensive rats by indirectly overcoming PepT1 inhibition. Eur J Pharm Biopharm. (2018) 128:179–87. doi: 10.1016/j.ejpb.2018.04.021
129. Thanou, M, Verhoef, JC, and Junginger, HE. Oral drug absorption enhancement by chitosan and its derivatives. Adv Drug Deliver Rev. (2001) 52:117–26. doi: 10.1016/S0169-409X(01)00231-9
130. Shen, W, and Matsui, T. Current knowledge of intestinal absorption of bioactive peptides. Food Funct. (2017) 8:4306–14. doi: 10.1039/C7FO01185G
131. Liu, C, Kou, YQ, Zhang, X, Cheng, HB, Chen, XZ, and Mao, SR. Strategies and industrial perspectives to improve oral absorption of biological macromolecules. Expert Opin Drug Del. (2018) 15:223–33. doi: 10.1080/17425247.2017.1395853
132. Kandemir, K, Tomas, M, McClements, DJ, and Capanoglu, E. Recent advances on the improvement of quercetin bioavailability. Trends Food Sci Tech. (2022) 119:192–200. doi: 10.1016/j.tifs.2021.11.032
Keywords: bioactive peptides, oral administration, bioavailability, oral delivery systems, peptide transport
Citation: Wang X, Yang Z, Zhang W, Xing L, Luo R and Cao S (2024) Obstacles, research progress, and prospects of oral delivery of bioactive peptides: a comprehensive review. Front. Nutr. 11:1496706. doi: 10.3389/fnut.2024.1496706
Received: 15 September 2024; Accepted: 23 October 2024;
Published: 14 November 2024.
Edited by:
Jayani Chandrapala, RMIT University, AustraliaReviewed by:
Xianglu Zhu, East China University of Science and Technology, ChinaCopyright © 2024 Wang, Yang, Zhang, Xing, Luo and Cao. This is an open-access article distributed under the terms of the Creative Commons Attribution License (CC BY). The use, distribution or reproduction in other forums is permitted, provided the original author(s) and the copyright owner(s) are credited and that the original publication in this journal is cited, in accordance with accepted academic practice. No use, distribution or reproduction is permitted which does not comply with these terms.
*Correspondence: Songmin Cao, c29uZ21pbl9jYW9Abnh1LmVkdS5jbg==
†These authors share first authorship
Disclaimer: All claims expressed in this article are solely those of the authors and do not necessarily represent those of their affiliated organizations, or those of the publisher, the editors and the reviewers. Any product that may be evaluated in this article or claim that may be made by its manufacturer is not guaranteed or endorsed by the publisher.
Research integrity at Frontiers
Learn more about the work of our research integrity team to safeguard the quality of each article we publish.